Corrigendum: The significance of cephalopod beaks as a research tool: An update
- 1Department of Life Sciences, Marine and Environmental Sciences Centre/ ARNET–Aquatic Research Network, University of Coimbra, Coimbra, Portugal
- 2British Antarctic Survey, Natural Environment Research Council, Cambridge, United Kingdom
- 3GEOMAR Helmholtz Centre for Ocean Research Kiel, Kiel, Germany
- 4Instituto Español de Oceanografía (IEO,CSIC), Santa Cruz de Tenerife, Spain
- 5CONACYT -Tecnológico Nacional de México/I. T., Chetumal, Quintana Roo, Mexico
- 6Mola di Bari, Italy
- 7Littoral Environnement et Sociétés (LIENSs), UMR 7266 CNRS-La Rochelle Université, La Rochelle, France
- 8Institut Universitaire de France (IUF), Paris, France
- 9Center of Marine Sciences, University of Algarve, Campus de Gambelas, Faro, Portugal
- 10Department of Marine Science, Coastal Carolina University, Conway, SC, United States
- 11Instituto de Investigaciones Marinas (CSIC), Vigo, Spain
- 12IU-ECOAQUA, University of Las Palmas de Gran Canaria, Edf. Ciencias Básicas, Campus de Tafira, Las Palmas de Gran Canaria, Spain
- 13Atlantic Naturalist Association, Horta, Portugal
- 14National Museum of Nature and Science, Tokyo, Japan
- 15Centre for Environment, Fisheries and Aquaculture Science (CEFAS), Lowestoft, United Kingdom
- 16HCMR, Hellenic Centre for Marine Research, Athens, Greece
- 17Laboratory for Ecology and Morphology of Marine Invertebrates, A.N. Severtsov Institute of Ecology and Evolution of the Russian Academy of Sciences, Moscow, Russia
- 18Department of Ecology and Animal Biology, Faculty of Marine Sciences, University of Vigo, Vigo, Spain
- 19College of Marine Sciences, Shanghai Ocean University, Shanghai, China
- 20MARE—Marine and Environmental Sciences Centre/ARNET–Aquatic Research Network, Laboratório Marítimo da Guia, Faculdade de Ciências, Universidade de Lisboa, Cascais, Portugal
- 21Centre Technique de Recherche et de Valorisation des Milieux Aquatiques (CITEB), Le Port, Île de la Réunion, France
- 22Centre de Recherche en Paléontologie-Paris (CR2P), CNRS, Sorbonne Université, Paris, France
- 23University of South Florida, College of Marine Science, St. Petersburg, FL, United States
- 24Institut de Ciènces del Mar, CSIC, Psg. Marítim de la Barceloneta, Barcelona, Spain
- 25Universidade Aberta, Rua Escola Politécnica, Lisboa, Portugal
- 26Department of Mechanical Engineering, Faculty of Engineering Science, University College London, London, United Kingdom
- 27Center for Marine Studies—Federal University of Parana (UFPR), Pontal do Paraná, PR, Brazil
- 28Centre d’Etudes Biologiques de Chizé, UMR 7372 du CNRS-La Rochelle Université, Villiers-en-Bois, France
The use of cephalopod beaks in ecological and population dynamics studies has allowed major advances of our knowledge on the role of cephalopods in marine ecosystems in the last 60 years. Since the 1960’s, with the pioneering research by Malcolm Clarke and colleagues, cephalopod beaks (also named jaws or mandibles) have been described to species level and their measurements have been shown to be related to cephalopod body size and mass, which permitted important information to be obtained on numerous biological and ecological aspects of cephalopods in marine ecosystems. In the last decade, a range of new techniques has been applied to cephalopod beaks, permitting new kinds of insight into cephalopod biology and ecology. The workshop on cephalopod beaks of the Cephalopod International Advisory Council Conference (Sesimbra, Portugal) in 2022 aimed to review the most recent scientific developments in this field and to identify future challenges, particularly in relation to taxonomy, age, growth, chemical composition (i.e., DNA, proteomics, stable isotopes, trace elements) and physical (i.e., structural) analyses. In terms of taxonomy, new techniques (e.g., 3D geometric morphometrics) for identifying cephalopods from their beaks are being developed with promising results, although the need for experts and reference collections of cephalopod beaks will continue. The use of beak microstructure for age and growth studies has been validated. Stable isotope analyses on beaks have proven to be an excellent technique to get valuable information on the ecology of cephalopods (namely habitat and trophic position). Trace element analyses is also possible using beaks, where concentrations are significantly lower than in other tissues (e.g., muscle, digestive gland, gills). Extracting DNA from beaks was only possible in one study so far. Protein analyses can also be made using cephalopod beaks. Future challenges in research using cephalopod beaks are also discussed.
Introduction
The important role of cephalopods (Mollusca: Cephalopoda) in many marine ecosystems has been widely acknowledged (Boyle and Rodhouse, 2005). They are commercially exploited around the World (Rodhouse et al., 2014; Arkhipkin et al., 2015; Doubleday et al., 2016; Arkhipkin et al., 2021; Sauer et al., 2021), are predators on numerous prey and are preyed by predators (Santos et al., 2001; Boyle and Rodhouse, 2005; Bello et al., 2011; Hoving et al., 2014; Xavier and Cherel, 2021), whose predator-prey interactions has been helping the development of a conservation framework for some of these predators (Luna et al., 2021). As cephalopod flesh gets quickly digested in predator stomachs, cephalopod beaks (synonym of jaws and mandibles) can resist digestion for as long as several months (Xavier et al., 2005; Barrett et al., 2007). Malcolm Clarke revolutionised the way cephalopod beaks could be used in ecological research, by providing evidence that many of them have unique shapes at species level (Clarke, 1962; Clarke, 1980; Clarke, 1986). Clarke and colleagues also developed the currently used terminology for different parts of the upper and lower beaks (Clarke, 1986) (Figure 1). Such initial efforts helped many other colleagues to develop beak identification guides (Imber, 1978; Pérez-Gándaras, 1983; Wolff, 1984; Kubodera and Furuhashi, 1987; Lu and Ickeringill, 2002; Xavier and Cherel, 2021; Pedà et al., 2022) and supported studies to understand the importance of cephalopods in the diet of different predator taxa (Clarke, 1996; Croxall and Prince, 1996; Klages, 1996; Smale, 1996; Cherel and Klages, 1998; Ménard et al., 2013; Abreu et al., 2019; Romanov et al., 2020; Queirós et al., 2021b; Cherel, 2021; Guímaro et al., 2021). This is particularly important as much information cannot be obtained by other means (e.g., scientific nets are too slow to catch faster cephalopods and catch far fewer species and narrower range of sampled sizes) (Clarke, 1977; Santos et al., 2001; Staudinger et al., 2013; Hoving et al., 2014; Cherel, 2020). Cephalopod beaks can also provide considerable information on a wide range of physiological, biological and ecological traits, including cephalopod availability, consumption of cephalopods, migrations, competition between cephalopod predators, levels of cephalopod scavenging by predators, distribution, age, growth, cohorts, life-events, stress, thermal changes, reproduction, feeding ecology, behavior, spawning areas, post-spawning mortality and sexual dimorphism [e.g., see review in Xavier et al. (2016) and Arkhipkin et al. (2018); Table 1]. More recently, new emergent techniques for work on beaks (e.g., stable isotope and trace elements analyses, geometric morphometrics and microstructure analysis) have provided further information on habitat and trophic position, composition, contamination, response of cephalopods to climate variability at individual and/or population levels, embryonic morphogenesis, paralarval ontogeny, ecology and age estimation (Cherel and Hobson, 2005; Perales-Raya et al., 2014b; Franco-Santos and Vidal, 2014; Xavier et al., 2016; Perales-Raya et al., 2018; Queirós et al., 2018; Golikov et al., 2019a; Golikov et al., 2019b; Northern et al., 2019; Abreu et al., 2020; Queirós et al., 2020a; Armelloni et al., 2020; Queirós et al., 2020b; Franco-Santos and Vidal, 2020; Golikov et al., 2020; Perales-Raya et al., 2020; Fang et al., 2021b; Lishchenko and Jones, 2021). Consequently, the importance of cephalopod beaks in ecological studies continues to attract attention and recognition, with various workshops being organised (Clarke, 1986; Xavier et al., 2007a; Jackson et al., 2007; Xavier et al., 2015). A workshop on cephalopod hard structures including beaks was held in Florida (United States), prior to the 2018 Cephalopod International Advisory Council (CIAC) Conference, results of which should be published in the near future. Most recently, a workshop focused on cephalopod beaks was held in Sesimbra (Portugal) at the 2022 CIAC Conference (Figures A1, A2) in order to review the latest scientific advances on the use of cephalopod beaks in marine ecological studies and discuss future challenges in this and other related research fields. The individual sections below concern key topics in cephalopod research based on the beak analysis discussed during this 2022 CIAC workshop.
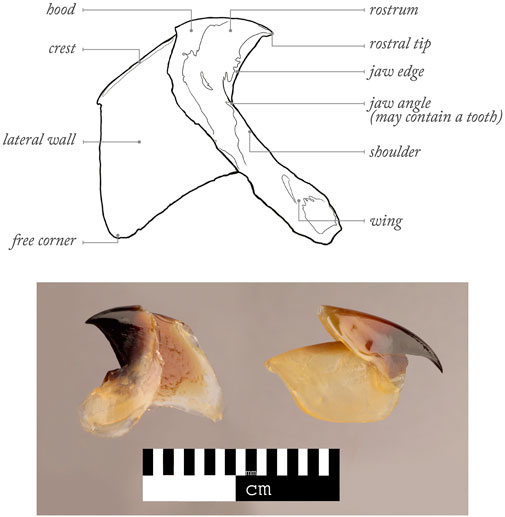
FIGURE 1. Examples of profiles of lower (on the left) and upper (on the right) beaks of cephalopods and the principal terms used to characterize decapod beaks (Xavier and Cherel (2021) following Clarke (1986)).
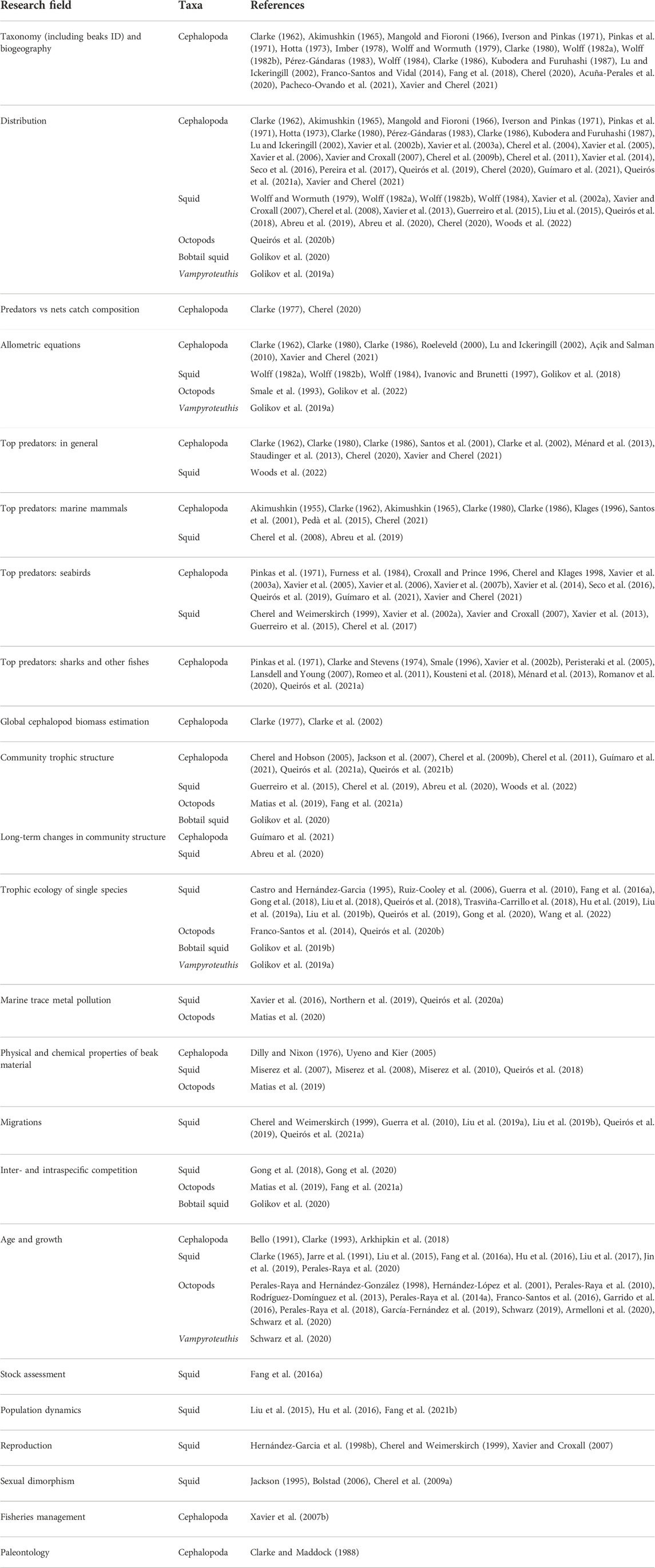
TABLE 1. A non-exhaustive overview of the use of cephalopod beaks in ecological studies and related research.
Advances in taxonomy, beak morphology, microstructure and paleontology
Taxonomy and beak morphology
Taxonomic identification is a critical issue of every investigation using accumulated cephalopod beaks from food samples (Table 1). Erroneous identifications can propagate along the studies through the years spreading and proliferating information, not only on predator-prey relationships but also on every subsequent analysis on beaks, regardless the nature of the analyses (e.g., species occurrence/distribution, stable isotopes, trace elements, growth increments) (Cherel, 2020, 2021; Xavier and Cherel, 2021). Substantial efforts have been directed to facilitate the identification of these hard structures through drawings, photographs (from different angles), 3-D videos, measurements, and by materials being accessible through regular publications or on the internet [e.g., Tree of Life web project–http://tolweb.org/articles/?article_id=5274 (Young, 2009); https://www.kahaku.go.jp/research/db/zoology/Beak-E/intro.htm (Kubodera et al., 2005)] (Clarke, 1986; Cherel, 2020; Xavier and Cherel, 2021). Nevertheless, the need for more research experts (e.g., boost/support a new generation of early career scientists in this field) on cephalopod beaks, as well as updated collections and more comprehensive guides will continue to be a necessity in the future (Xavier et al., 2007a; Xavier et al., 2015; Cherel, 2020; Xavier and Cherel, 2021).
Several methods applicable to cephalopod beak shape analysis were developed to date (Fang et al., 2018; Lishchenko and Jones, 2021). Nowadays, the group of methods which can be called “traditional morphometrics” (measurements of the linear distances, or indices, based on these measurements), is criticised for leading to a significant loss of information due to the complexity of studied structures and the multicollinearity between measurements (Adams et al., 2004; Volpedo and Vaz-dos-Santos, 2015). To some extent, this criticism is justified, although these analyses allowed the development of the first steps of beak shape analysis relevant for identification (Mangold and Fioroni, 1966; Wolff, 1982b; Wolff, 1984; Ogden et al., 1998; Fang et al., 2018). Moreover, they are the least laborious among morphometric methods and the richest in terms of available data for comparison.
An alternative approach is represented by two groups of geometric morphometric methods. The first group is based on obtaining Cartesian coordinates of biologically definable points, also called landmarks (Bookstein, 1991; Cadrin, 2014). In addition, sliding semi-landmarks, which are defined by equidistant points between two landmarks are also used to represent curves and surfaces of structures (Bookstein, 1997; Gunz et al., 2005; Gunz and Mitteroecker, 2013). These landmarks and semi-landmarks may be two- or three-dimensional, and generally are discrete and homologous (Zelditch et al., 2004). The coordinates obtained are modified using Procrustes analysis to avoid impact of the position and size of the studied object.
The second group of methods includes those methods which describe the structure’s outline as a whole. The most applied methods in this group are Fourier transform (where outlines are expressed as a function of equally spaced radii or of the tangent angle to the outline or of the curvilinear abscissa) and wavelet transform (with outlines expressed by a set of functions representing the dilations and translations of a single unique function). Application of both approaches to analyse the shape of cephalopod beaks have benefits and limitations (Lishchenko and Jones, 2021). Specifically, landmark-based methods allow selecting the points of interest which presumably have some biological or taxonomic meaning (e.g., rostral tip and wing fold, whose position reflects the length and curvature of the rostrum). On another hand, landmark selection inevitably leads to the loss of information, which may be crucial if the points of interest were chosen incorrectly. Additionally, process of landmark selection is particularly laborious, time consuming and demands a certain level of qualification of the researcher. The outline-based methods of the shape analysis do not have these drawbacks and allow detailed description of a beak’s contour on the image. However, the latter methods are associated with a very specific issue, which is called a “pixel noise” by some authors (Haines and Crampton, 2000; Harbitz and Albert, 2015) (i.e., “pixel noise” stands for the excessive and meaningless set of information which hampers the analysis of the structure’s shape).
Despite these limitations, the potential of these approaches is substantial, supported by the results of recent studies. Most commonly, geometric morphometrics methods were successfully applied for taxonomic classification, species identification or stocks (Neige and Dommergues, 2002; Crespi-Abril et al., 2010; Tanabe et al., 2015b; Fang et al., 2017; Jin et al., 2017; Fang et al., 2018; Pacheco-Ovando et al., 2021; Díaz-Santana-Iturrios et al., 2022). At the beginning of the millennium, the application of these approaches to a wide diversity of species was scarce (Neige and Dommergues, 2002; Tanabe et al., 2015b) and provided limited resolution of identification. Their findings suggested that the 2-D lateral shapes of beaks (lateral wall, hood and wing contours) are clustered at high taxonomic levels (orders, suborders) and that the upper and lower parts of the beak carry a slightly different information. By showing that to be true, quantitative analysis of beak shape might assist identification at high taxonomic levels (at least, to the level of family). However, in the last decade, geometric morphometric methods began to flourish. Different authors applied either 2D landmark-based methods (Fang et al., 2017; Fang et al., 2018; Pacheco-Ovando et al., 2021; Díaz-Santana-Iturrios et al., 2022) or outline-based methods (Jin et al., 2017). Both approaches showed high level of identification accuracy, up to 100% in classification of the genera (Pacheco-Ovando et al., 2021), up to 93% in species identification (Fang et al., 2018), and up to 70% correct classifications of stock units (Fang et al., 2017). These results point to the potential to engineer automated identification programs.
Several studies revealed the potential of beak shape analysis in ecological studies (Fernández-Álvarez et al., 2020; Pacheco-Ovando et al., 2021; Roscian et al., 2022). Specifically, Fernández-Álvarez et al. (2020) studied impacts of developmental malformations of the buccal mass on the trophic position of Eledone cirrhosa and found that the habitat and the trophic position were not significantly affected by the malformations. Other authors found significant differences in the beak shapes of pelagic and benthic species in relation to their trophic levels (Roscian et al., 2022), between species living in coastal and oceanic habitats (Pacheco-Ovando et al., 2021) and between species/populations living in different feeding habitats (Pacheco-Ovando et al., 2021). New phylogenomic techniques applied to cephalopods (Anderson and Lindgren, 2021; Sanchez et al., 2021; Fernández-Álvarez et al., 2022) may help to assess which morphological characters of the beaks are determined by phylogeny and which are explained by other drivers.
Geometric morphometric studies of cephalopod beaks have the greatest potential in the field of species identification, as part of both the routine monitoring process and as high-end studies. Application of outline-based methods allows it even without additional efforts. At present, an automated similar system has been used for the identification of fish using otolith contours (Lombarte et al., 2006). This approach shows that this system may allow accurate identification of animals even when there is only very basic information available about the subject of research and it is probably the least time-consuming method of them all. Development of such a system is a long-term process that needs close validation checks, but even at the early stage of development, it could substantially expand our knowledge on cephalopods.
Beak morphological changes during the early life
Beaks of embryos and paralarvae are quite distinct from those of juveniles and adults (Franco-Santos et al., 2014; Franco-Santos and Vidal, 2014; Franco-Santos et al., 2016; Armelloni et al., 2020; Franco-Santos and Vidal, 2020). The beaks of hatchlings and smaller squid and octopus paralarvae studied so far, are very fragile and slightly pigmented, being nearly transparent. Growth rings might or not be clearly visible in the lateral wall of both the upper and lower beaks (Franco-Santos and Vidal, 2014), and they might be visible in the anterior pigmented region of upper beaks that corresponds to the rostrum (Perales-Raya et al., 2014b; Franco-Santos et al., 2016; Arkhipkin et al., 2018; Perales-Raya et al., 2018). Paralarval beaks in several cephalopod families (E.g., Family Ommastrephidae, family Octopodidae) have teeth that might be present in both beaks or only in the lower one (Boletzky, 1971; Wakabayashi et al., 2002; Uchikawa et al., 2009; Franco-Santos et al., 2014; Franco-Santos and Vidal, 2014; Franco-Santos and Vidal, 2020). In the upper beak of many species, the rostrum has not yet protruded, and has a typical sagittal slit between the two-halves that could be slightly or very pronounced (Figure 2). In addition, conspicuous features of the beaks, such as the hood and lateral walls might not be developed yet in paralarvae of some families, such as Ommastrephidae (Franco-Santos and Vidal, 2020), giving the beak a more rounded shape. A study with late embryonic hatching stages of Octopus vulgaris embryos has shown that the upper beak is rudimentary and lacks the hood, but the teeth are already visible and these stages represent the beginning of the pigmentation process. The hood and shoulder develop along with the exposure of the dentition on the rostrum just prior to hatching (Armelloni et al., 2020), suggesting that beak development is intensified afterwards during the paralarval phase (Franco-Santos and Vidal, 2014; Franco-Santos and Vidal, 2020).
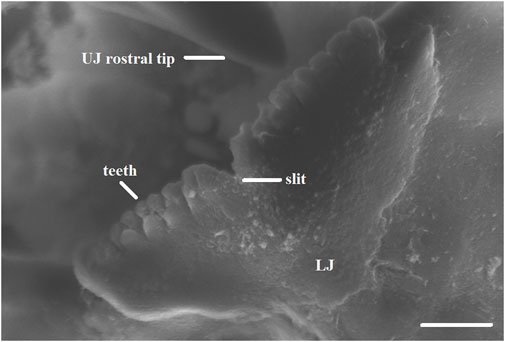
FIGURE 2. Close-up of a scanning electron microscope image indicating (lines) the teeth and slit in the lower beak (LJ) and the rostral tip in the upper beak (UJ) of an Illex argentinus paralarva of 4.0 mm ML. Scale bar 10 μm ((Franco-Santos and Vidal, 2020); copyright permission obtained).
The beak morphology of smaller paralarvae suggests a weak bite force. Thus, at this stage the beak has not yet assumed the functions of biting flesh and masticating the hard exoskeleton of crustaceans (Franco-Santos and Vidal, 2014). Indeed, beaks of smaller paralarvae seem to be adapted for a specialised feeding mode that involves external pre-digestion and suction of body fluids of crustacean prey (Franco-Santos et al., 2014; Franco-Santos and Vidal, 2014; Franco-Santos and Vidal, 2020). This is supported by observations of the feeding behaviour of O. vulgaris and loliginid squid paralarvae (Hernández-García et al., 2000; Franco-Santos and Vidal, 2014).
As paralarvae grow, the teeth are eroded and disappear, and the hood and lateral walls grow rapidly as they are important sites for buccal musculature attachment (Uyeno and Kier, 2007). The sagittal slit progressively closes and gives way to the rostrum. The pigmented area increases through the deposition of sclerotized layers (i.e., not chitin during the darkening process), particularly in the rostrum, which protrudes very fast in both beaks (Franco-Santos and Vidal, 2014; Franco-Santos and Vidal, 2020). Beak darkening is a continuous process, through which beaks become harder and more robust (Hernández-García et al., 1998a, b; Miserez et al., 2007). Fully pigmented beaks were considered to be “mature beaks” by Clarke (1986).
These studies have shown that the underdeveloped beaks of paralarvae are rapidly transformed, giving way to the prominent structures present in juvenile and adult beaks. Such studies have provided the foundation for inferences about rostrum functionality and its relationship with feeding strategy and prey selection during the first stages of the life cycle, about which little is known (Franco-Santos and Vidal, 2014; Nande et al., 2017; Vidal and Salvador, 2019; Franco-Santos and Vidal, 2020). In addition, beaks of O. vulgaris paralarvae have been used to validate daily growth increments in the early stages, for comparison of wild and cultured specimens to understand massive mortalities previous to the juvenile phase (Garrido et al., 2016; García-Fernández et al., 2019) and to solidify our understanding of the significant influence of temperature on increment deposition (Perales-Raya et al., 2018). The accuracy of age estimation inferred from growth marks in the upper beak rostrum has been also confirmed in late-stage embryos (Armelloni et al., 2020). In addition, it was suggested that these growth marks might be used as biomarkers for stress during rearing of O. vulgaris paralarvae (Franco-Santos et al., 2016).
Microstructure: Age, growth and record of life extreme events
Although statoliths are the most frequently used material for age determination in cephalopods, it has been suggested that beaks could provide additional/complementary data, especially when it is impossible to obtain access to age data from statoliths (Liu et al., 2014). This is the case for octopods, which lack visible increments in the microstructure of their statoliths (Clarke, 1978). In addition, statoliths of adult cephalopods need to be ground on both sides, which is time-consuming and labour-intensive, whereas beaks do not need to be ground on both sides (Arkhipkin et al., 2018). Beaks are present throughout the life cycle of all extant cephalopod species, and can be easily extracted and preserved (Clarke, 1986). Beak growth process takes place along the posterior border, where the most recent chitinized and hydrated material is deposited, so that the oldest and most pigmented material is found in the anterior tip (Miserez et al., 2008; Perales-Raya et al., 2014a). Moreover, growth increments have been observed and validated along several parts of cephalopod beaks [e.g., lateral wall surfaces, rostrum sagittal sections (Perales-Raya et al., 2010)]. Perales-Raya et al. (2010) recommended counting growth increments in the lateral wall surface of beaks of Octopus vulgaris as fewer increments were detected in rostrum sagittal sections, probably due to erosion of the rostral tip when the animal is feeding. In some cephalopods (including O. vulgaris) these increments have been validated as being deposited daily (see below for further discussion). Thus, counts of growth increments on these structures can potentially provide absolute age estimates and growth data in any ontogenetic phase (Figure 3). Comparison with other structures in which deposition of growth increments is thought to be daily, as it has been validated for statoliths in several species of squid (E.g., Illex illecebrosus, Loliolus noctiluca, Loligo chinensis, Loligo vulgaris reynaudii) and cuttlefish (E.g., Sepia officinalis) (Hurley et al., 1985; Jackson, 1990; Lipinski et al., 1998; Bettencourt and Guerra, 2001), can be used to infer the periodicity of increment deposition in the beaks of newly studied species or when validation experiments are not feasible. Nevertheless, validation experiments involving mark-recapture or captive rearing of known-age or chemically-marked specimens are needed to ensure absolute age determination in the species (Campana, 2001). Even then, and considering that a circadian rhythm has been proved in many species (Cobb et al., 1995; Meisel et al., 2003), the fact that growth increments are shown to be deposited daily does not prove that this will always be the case to all cephalopod species/populations as increment deposition may depend on various factors (e.g., food availability, water temperature) (Bettencourt and Guerra, 2000; Zumholz et al., 2006; Canali et al., 2011).
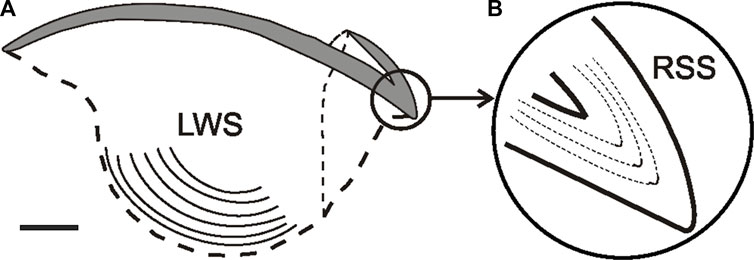
FIGURE 3. Lateral view of the upper beak in Octopus vulgaris (A) Sagittal section showing the inner lateral wall surface (LWS) bearing increments (lines). (B) Rostrum sagittal section magnified and showing the daily increments. From Perales-Raya et al. (2014a); copyright permission obtained. Bar: approx. 2 mm.
Since growth increments in beaks were first reported in the 1960s for the squid Moroteuthopsis longimana (misidentified as Moroteuthis ingens by Clarke (Clarke, 1965; Cherel, 2020), many attempts have been made to use these structures to estimate cephalopod age (Tables 1, 2). Indeed, the growth increments in cephalopod beaks, initially observed by Clarke (1965), in the surface of lateral walls did not show a suitable sequence of increments to estimate the age in the species examined. The use of beak microstructure for age estimation was re-assessed in the 1990’s in octopuses (Perales-Raya and Hernández-González, 1998), due to the absence of evident increments in their statoliths. Perales-Raya and Hernández-González (1998) observed a sequence of thin increments in sagittal sections of the rostrum of O. vulgaris beaks, and suggested that their deposition should be related to an individual’s age. Successful analysis of lateral wall surfaces of O. vulgaris beaks was then performed by Hernández-López et al. (2001) and daily deposition was confirmed in paralarvae. Then, both techniques were compared and improved by Perales-Raya et al. (2010). Validation studies were subsequently performed to confirm daily deposition also in octopuses (E.g., Octopus vulgaris, Octopus maya) (Canali et al., 2011; Rodríguez-Domínguez et al., 2013; Bárcenas et al., 2014). Finally, daily deposition was validated in the full ontogenetic range for O. vulgaris on both the rostrum sagittal sections and the lateral wall surfaces by Perales-Raya et al. (2014a). These authors used the recording of specific events in beaks of O. vulgaris taken into captivity (e.g., capture, temperature changes) as dated marks to validate the temporal deposition of increments. Consequently, the analysis of beaks as life event recorders has been applied in wild populations to understand the effects of environmental variations, biological events, stress of capture (Perales-Raya et al., 2014b), and to improve the welfare of early stages in reared populations (Franco-Santos et al., 2016).
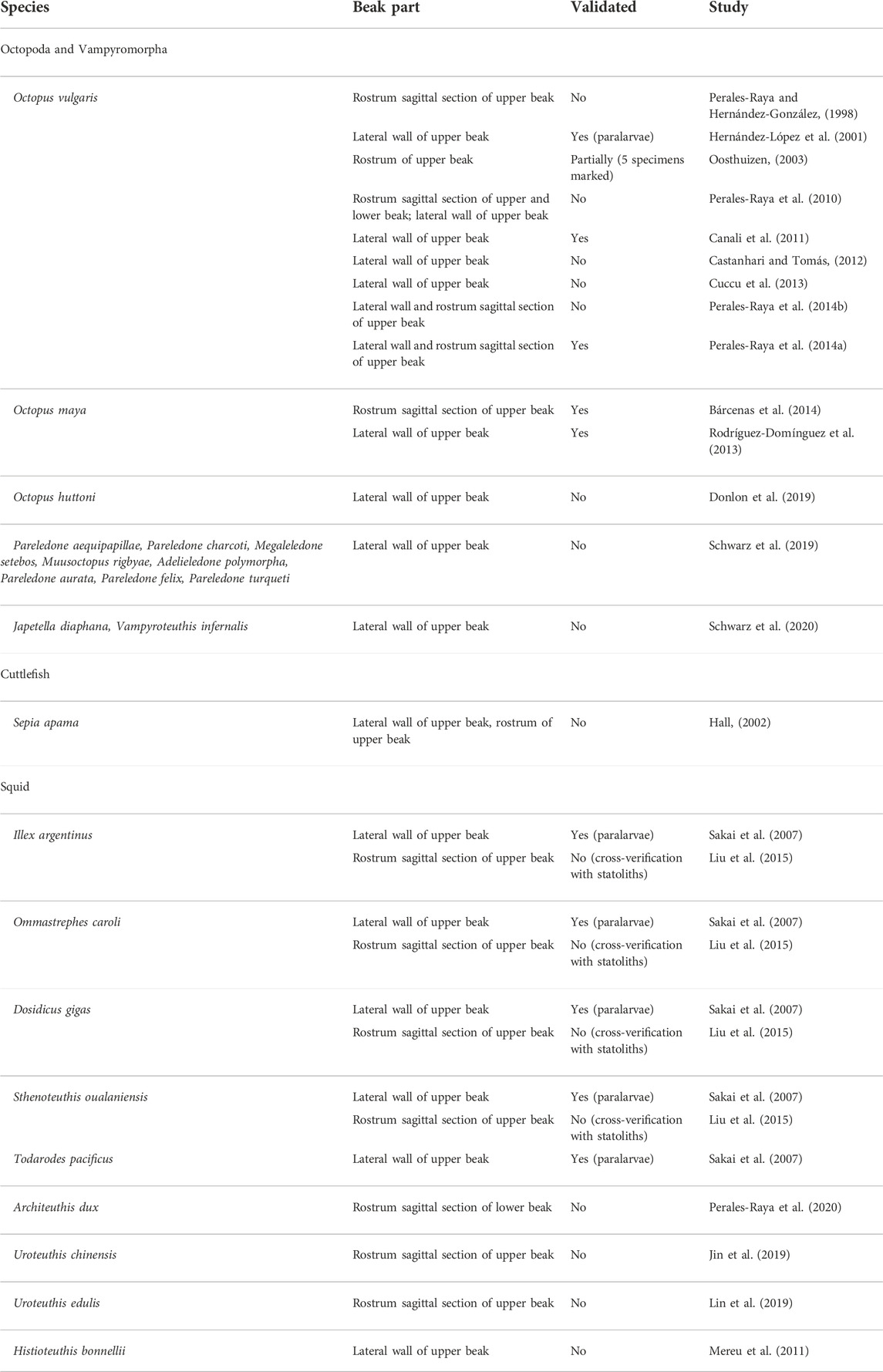
TABLE 2. Detailed information on cephalopod species that have been attempted using beak increment analysis.
Since the daily deposition was validated in O. vulgaris (Figure 4), the beak microstructure has been used in age and growth studies of several species of squid, cuttlefish and octopods, providing key information on their life history and population dynamics (Cuccu et al., 2013; Fang et al., 2016a; Liu et al., 2017; Batista et al., 2021). Validation studies have been performed in species such as Sepia officinalis (Lishchenko, unpublished data) and Octopus insularis, based on the growth increments in the rostrum sagittal sections or lateral wall surface of the beak (see references in Table 2).
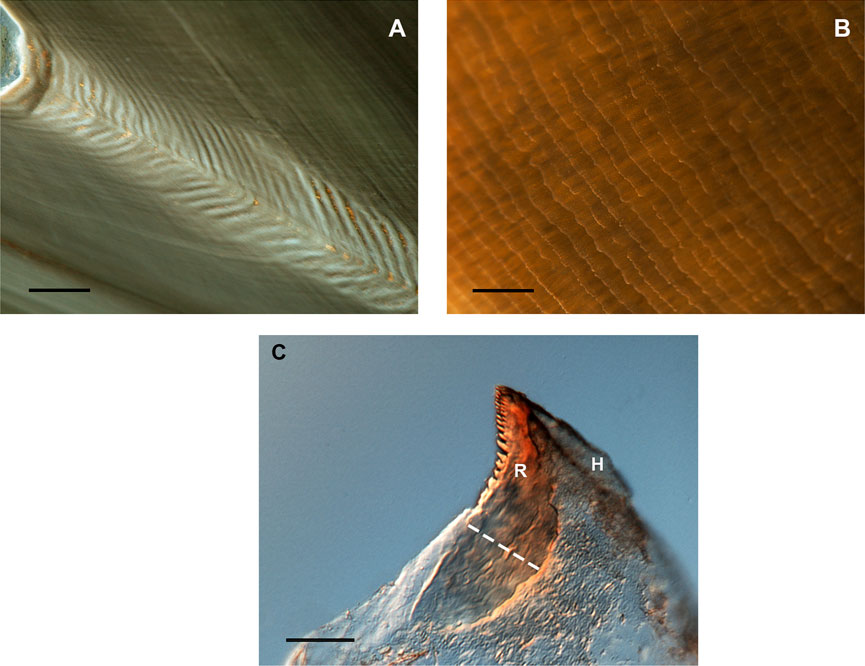
FIGURE 4. Microstructure of beaks showing growth increments in cephalopod beaks (Octopus vulgaris). (A) Increments in the rostrum sagittal sections (RSS). Bar: 100 µm. (B) Increments in the inner lateral wall surface of the upper beak. Bar: 200 µm. (C) Increments in the rostrum surface of the upper beak in early stages. R = rostrum; H = hood; dotted white line = reading area. From Arkhipkin et al. (2018) and Perales-Raya et al. (2018); copyright permission obtained). Bar: 50 µm.
In relation to using beaks for age determination in cephalopods, it was found that the tip erosion during the feeding process may bias increment counts in the anterior region of the beak but counting the oldest increments in the dorsal area of the section prevents age underestimation (Arkhipkin et al., 2018). A simple method has been recently developed to quantify the tip erosion using the number of increments in the dorsal non-eroded region and the width of increment of the central reading region (Perales-Raya et al., 2020). Moreover, the beak microstructure of emblematic deep-sea species such as the giant squid Architeuthis dux (Perales-Raya et al., 2020) (Figure 5) provided age data and a maximum lifespan estimation of around 3 years, based on rostrum sagittal sections from the lower beaks. The same technique was used in the beaks of the warty squid M. longimana from the stomach contents of Antarctic toothfish to estimate the age of this Southern Ocean species (Queirós, Bartolomé, Xavier, Perales-Raya, unpublished data). In both studies, the authors tested the lateral wall surface of upper beaks and the rostrum sagittal sections of upper and lower beaks but only the latter showed a suitable sequence of increments for age estimation. On the contrary, the lateral wall surface has been the only beak region explored in Antarctic incirrate octopods from the families Megaleledonidae and Enteroctopodidae (Schwarz, 2019; Schwarz et al., 2019). These authors suggested lifespans exceeding 3 years and the possibility that deposition of growth increments in beaks of Antarctic octopods is not daily.
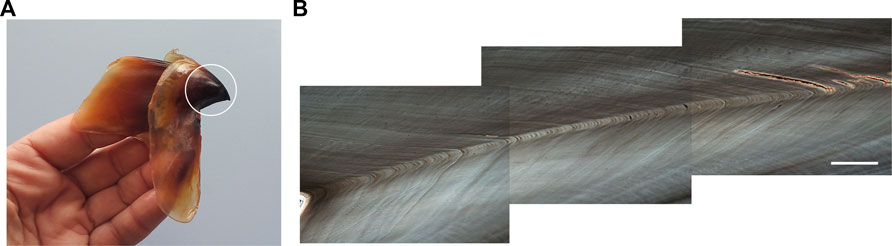
FIGURE 5. Lower beak of the giant squid Architeuthis dux. A white circle highlights the rostrum (A). Image composition of the sagittal section of the lower beak rostrum showing growth increments (B). From Perales-Raya et al. (2020); copyright permission obtained). Bar: 200 µm.
Growth increments (usually a few dozen) are also known on the surface of ammonite aptychi (calcitic coverings of the lower beak). The major growth increments were hypothesised to represent fortnightly tidal cycles or months, and the minor increments to correspond to days or semi-diurnal tidal cycles. If this assumption is correct, the ammonite life span would last between 1 and 6 years and the growth would be sigmoidal, with slowing down at maturity (Hewitt et al., 1993; Machalski, 2021).
Palaeontology: Fossil beaks
The nautiloid and ammonoid beaks are the cephalopod structures that fossilise most effectively after the shells. They are most often preserved in 2D but 3D fossils occasionally occur (Nixon, 2015). Ammonoid lower beaks are the most abundant in the fossil record, particularly from the Lower Jurassic (Toarcian) where a bivalve calcareous covering appears on the lower beak (Tanabe et al., 2015a). Similarly, the rostrum of the upper beaks of nautiluses, consisting of a hard, pointed calcareous tip (rhyncholite), is quite common in the fossil record from the Triassic onwards. Entirely organic beaks such as those of coleoids are very rare in the fossil record but are nevertheless known from exceptionally well-preserved deposits, the so-called Lägersttätten. Numerous examples are found in ammonoids dating back to the Devonian (Tanabe et al., 2015a; Klug et al., 2016). They are rarer in the Nautilida, but a few specimens are usable for shape analysis (Klug et al., 2021a). In Coleoids, beaks are found from the end of the Lower Jurassic (Nixon, 2015; Klug et al., 2021b). Fairly well-preserved 3D specimens have been related to Sepiolida (Harzhauser, 1999), Oegopsida (Tanabe et al., 2006), Cirrata (Tanabe et al., 2008), Vampyromorphida (Tanabe and Hikida, 2010), or undetermined teuthid taxa (Tanabe et al., 2015b).
All these fossils are still underused because of the shortage of comparative analyses between current and fossil beaks. The identification of beak shape adaptations to habitats or prey selection in modern species could inform our understanding of fossil shapes, thus complementing the classical paleoecological inferences from shells, gladius, soft tissues or fossil deposits (Fuchs and Iba, 2015; Fuchs et al., 2016). For example, investigating habitat shifts from shallow neritic to mesopelagic or bathyal environments (Hoving et al., 2014; Košťák et al., 2021) or vice versa (Arkhipkin et al., 2012) is of importance for understanding the evolution of modern coleoid lineages and for interpreting radiation events during cephalopod evolutionary history.
Currently, the data support a significant diversification event in Octopodiformes and Decapodiformes in relation to the so-called Marine Mesozoic Revolution, characterised by a major remodelling of shallow ecosystems, the rise of durophagous predators, and the resulting ecological “arms race” between predators and prey (Salamon et al., 2012). In this context, the diversification of coleoid cephalopods (stem Decabrachia, Spirulida) would have been driven by competition with predatory fish (Packard, 1972; Tanner et al., 2017; López-Córdova et al., 2022). The lineages of modern coleoid species are thought to have originated from one or more radiation pulses before the Cretaceous-Paleogene extinction (K-Pg crisis) (Fuchs and Lukeneder, 2014; López-Córdova et al., 2022). After the K-Pg crisis, a radiation pulse was stimulated by the disappearance of many predators and the availability of ecological space. Finally, another radiation pulse occurred during the mid-Cenozoic, controlled by climate change and low predator pressure (Fuchs and Lukeneder, 2014; López-Córdova et al., 2022). New data contributing to the inference of habitat, trophic level or predatory abilities of fossil species will be useful to assess the proposed evolutionary scenarios. To achieve this goal, thorough analysis of the relationship between beak shape and species ecology in current cephalopods, followed by integration of the results with the fossil species would be an excellent step forward.
Advances in analysis if the composition of cephalopods beaks relevant to ecological studies
Cephalopod beaks are secreted by a single layer of cells in the buccal tissue, the beccublast cells, which are tall columnar epithelial cells (Dilly and Nixon, 1976; Tan et al., 2015). Beaks grow without replacement throughout the life of cephalopods (Perales-Raya et al., 2014a). Therefore, performing chemical analyses on entire beaks yields an average value for the entire life of the individual, whereas dividing the beak into different sections allows us to study different periods within the life cycle (Cherel and Hobson, 2005; Cherel et al., 2009a; Guerra et al., 2010; Xavier et al., 2016; Queirós et al., 2020a).
Beak chemical composition as a challenge to the application of chemical analyses
Beaks are composed of chitin-protein complexes (Miserez et al., 2007). There are differences in the composition among cephalopod taxa, with beaks of octopods being composed exclusively of α chitin, whereas those from squid, while mainly composed by α chitin, also contain β chitin (Miserez et al., 2007; Matias et al., 2019). However, these variations are not expected to result in significant differences when performing chemical analyses on these structures. In contrast, the ratio between chitin and protein varies along the beak, which can have significant influences on the analyses (e.g., lowering of δ15N values when the amount of chitin is higher) (Miserez et al., 2008; Tan et al., 2015). These differences in the chitin: protein ratio can be easily observed in the pigmentation, with the untanned parts of the beak having a higher content of chitin than the fully tanned portion, which has a higher protein content (Miserez et al., 2008).
Stable isotopes
Stable isotopes, particularly of δ13C and δ15N, are widely used in ecological studies (Peterson and Fry, 1987; Bearhop et al., 2004; Newsome et al., 2007). Using δ13C values, it is possible to determine the carbon source at the base of the food chain and, ultimately, the feeding habitat of individuals (Cherel and Hobson, 2005). In marine systems, δ13C values are known to vary with latitude (lower towards the poles), with inshore–offshore gradient (lower values towards offshore waters) and between benthic and pelagic environments (lower in pelagic organisms) (Cherel and Hobson, 2005; Newsome et al., 2007; Magozzi et al., 2017). In addition, anthropogenic CO2 in the atmosphere has resulted in a decrease in δ13C (as well as in 14C) in both the atmosphere and the oceans, due to fossil fuels being relatively depleted in heavier carbon isotopes, the so-called Suess effect (Keeling, 1979; Gruber et al., 1999; Sonnerup et al., 1999). Regarding δ15N values, these are used to study the trophic position of the individuals, based on the principle that predators are enriched in 15N in relation to their prey (Peterson and Fry, 1987).
Bulk stable isotopic analyses (SIA) on beaks have been routinely perfomed, with δ13C and δ15N measured in cephalopods from all ocean basins (Hobson and Cherel, 2006; Cherel et al., 2009b; Guerra et al., 2010; Navarro et al., 2013; Golikov et al., 2019a; Queirós et al., 2020b; Fang et al., 2021a). They have been used: 1) in ecological and biogeographical studies to determine the foraging habitat and the role of cephalopods in food webs (Ruiz-Cooley et al., 2006; Guerra et al., 2010; Golikov et al., 2018; Staudinger et al., 2019; Fang et al., 2021a), 2) in fisheries to study stocks’ distribution, contributing also to the implementation of ecosystem-based management (Fang et al., 2016b; Queirós et al., 2019), and 3) to study impacts of climate change and environmental fluctuations in these organisms (Golikov et al., 2019a; Hu et al., 2019; Abreu et al., 2020). Furthermore, SIA on beaks can also be used to study the foraging ecology of their predators (Guerreiro et al., 2015; Abreu et al., 2019; Guímaro et al., 2021). SIA of the entire beak gives us an average value for the life of the individual, and when applied to different sections of the beak (using the methodology specifically applied for SIA analyses (Guerra et al., 2010; Queirós et al., 2018)), it enables the study of ontogenetic changes throughout the lifespan (Cherel and Hobson, 2005; Guerra et al., 2010; Queirós et al., 2018; Wang et al., 2022). More recently, compound specific stable isotopes on amino acids (CSIA-AA) have also been studied in cephalopod beaks, mainly to delete the chitin effect that lowers the bulk δ15N values of beaks when compared to other tissues (Cherel et al., 2019; Woods et al., 2022) (see below).
The SIA of beaks is a well-established technique with several advantages when compared to the use of other tissues. Cephalopod beaks recovered from predators’ stomachs have comparable stable isotopic compositions regardless the time they were subjected to digestive processes and independently of the time-period during which they have been kept in collections (Cherel and Hobson, 2005; Abreu et al., 2020). The preservation method (e.g., dried, frozen, in ethanol, in formalin) also does not affect SI values/ratios, enabling its application to museum beak collections and retrospective investigations (Ruiz-Cooley et al., 2011). However, when using SIA, there are some caveats to be aware of (see those listed below). Some of these can be overcome using CSIA-AA but due to the higher costs of this technique, its wider use has been so far limited. In addition, there are common problems (e.g., amount of sample required, knowledge of the trophic enrichment factor) associated with both CSIA-AA and bulk SIA. Below, we summarise the main problems associated with SIA, how to detect them and possible solutions. First and foremost, the amount of sample (usually ∼0.35 mg but it is dependent of the equipment/methodology/analyzer used) that is necessary for both SIA and CSIA-AA (and other analyses) can be a limitation, especially if the study aims for a sequential analysis along the beak or concerns smaller species/specimens whose entire beaks do not make up the necessary mass for the analyses. In this situation, a possible solution is to pool the minimum number of different beaks necessary to perform the analyses. However, it must be guaranteed that beaks belong to similar individuals (i.e., similar size, collected in the same location and at the same time) to ensure they belong to the same school/cohort (e.g., Guímaro et al., 2021; Queirós et al., 2021a). Interpretation of SIA results from cephalopod beaks (and other tissues) is dependent on baseline stable isotopic values (i.e., to determine the habitat and trophic level). It is advisable to determine the δ13C values of Particulate Organic Matter (POM) from the region and/or the δ15N values of an organism with a known trophic position (e.g., δ15N value of a filter-feeder species that it is in the second trophic level). To overcome the necessity of analysing POM stable isotopic values, it is possible to use values obtained from previous studies, or studies that modelled isotopic variation around the world’s oceans (Somes et al., 2010; Farmer et al., 2021; St John Glew et al., 2021). However, when using values from previous studies or when comparing values obtained in beaks collected in different periods, it is necessary to account for the temporal variation of isotopes in the environment. To solve this, the obtained δ13C values need to be corrected considering the Suess effect when values span across decades or consider modelled past δ13C values (Farmer et al., 2021). For the δ15N, the use of values obtained by previous modelling studies is so far the best option (Somes et al., 2010; Farmer et al., 2021; Verwega et al., 2021). If the capture location of the individual is known, the isotopic value of the near-death beak segment [i.e., the end of the hood and crest in the upper beak and the end of the hood and crest and wing in the lower beak (Queirós et al., 2018)], can be related to the local isotopic values. This may not be appropriate when using beaks sampled from predators’ stomachs, since it needs to also take into consideration the daily (and long-time scale) movements of the predator and the time the beak spent in the stomach and these will vary depending on predator and cephalopod species involved (Xavier et al., 2003b; Xavier and Cherel, 2021). Nevertheless, for bulk SIA, without measuring baselines at the time of collection, it is impossible to estimate the trophic position of the individual. In contrast, a baseline is not necessary when determining the trophic position using CSIA-AA because the use of so-called “source amino acids” (e.g., phenylalanine) provides a baseline for the cephalopod beak (Cherel et al., 2019). However, using bulk SIA it is still possible, by comparing different beaks or different sections of the beaks (Queirós et al., 2018), to identify changes in habitat or trophic position. One limitation when studying changes in habitat is that changes in δ13C values can be related to latitudinal, inshore-offshore, or benthic-pelagic changes (Cherel and Hobson, 2007; Newsome et al., 2007); thus results should be considered carefully and conclusions should be supported by previous knowledge of the ecology of the species. Furthermore, it is important to note that δ13C also increases by around ∼1‰ per trophic level through the food web, so that a significant correlation between δ13C and δ15N values could be due to a habitat change or diet change. Determining the slope of a regression between δ13C and δ15N values may however enable these two possibilities to be distinguished.
Regarding trophic position changes, when comparing δ15N values, it is important to know the relevant trophic enrichment factor (TEF) (trophic discrimination factor (TDF) in CSIA-AA) (i.e., the differences between the δ15N values of predator and its diet). Previous studies using S. officinalis raised in captivity showed that for beaks, this value is ∼3.4‰ (Hobson and Cherel, 2006). However, recent studies showed that the TEF tends to decrease with increasing trophic position, requiring the use of the “scale δ15N framework” (Hussey et al., 2014a; b). This δ15N framework also needs to be region specific (Hussey et al., 2014a; b). In CSIA-AA, the δ15N framework is not applicable and a TDF of ∼7.6‰ is used universally (O’Connell, 2017; Whiteman et al., 2019), although no cephalopod-specific studies exist to date. Also, CSIA-AA requires information on the difference between δ15N values of «trophic» and «source amino acids » in producers (trophic level 1), commonly referred to as β, to estimate consumer’s trophic position. A value of β of −3.4‰ is universally used in aquatic ecosystems (O’Connell, 2017; Whiteman et al., 2019). It should be borne in mind that δ15N values do not change only with the trophic position or prey species, but can be related to other changes in the diet of the predators such as the proportions of different prey that are eaten (Bearhop et al., 2004) and to changes in the diet of the prey species.
Currently neither bulk nor compound-specific SIA allows the identification of specific prey species. Even if the measurement of δ13C and δ15N values in a specific target prey species is performed, it is difficult to confirm that the tested species was eaten rather than another species with similar diet and trophic level (Fogel and Tuross, 2003; Naito et al., 2013; Pinkerton et al., 2014; Golikov et al., 2020). So-called mixed models are commonly used to infer predator diet composition from bulk SIA data. They require information on the δ13C and δ15N values of all putative prey but, even then, the natural variation around average values needs to be accounted for and, fundamentally, using values of only two variables (δ13C and δ15N values) to determine the values of multiple model parameters (the dietary importance of N putative prey types) is difficult (Phillips et al., 2014). In this sense, CSIA-AA offers a way forward since δ13C and δ15N values are potentially available for multiple trophic amino acids, greatly increasing the theoretical discriminatory power of the data to allow diet composition to be determined.
The δ13C and δ15N values obtained from bulk SIA and CSIA-AA are not directly comparable, and even if using the estimated trophic position allows the two types of δ15N measurement to be compared indirectly, there is currently no such method for δ13C. One key limitation when applying SIA to beaks is their chitin content. Chitin is a polymer of N-acetyl-glucosamine (i.e., a complex sugar that contains N atoms that are impoverished in 15N when compared to amino acids). Thus, δ15N values are not only lower in beaks than in other tissues, but also vary between different sections of the same beak due to the varying chitin: protein ratio (as mentioned above). It is necessary to carefully evaluate the C:N mass ratios obtained in the results, with higher ratios suggesting a higher amount of chitin (Cherel et al., 2009a). It may be possible to define a C:N ratio beyond which SIA results should be discarded, or to calculate a correction factor based on previous studies [e.g., Post et al. (2007)]. Results are usually considered unusable if C:N ratio values are above 4.0 but it may be appropriate to use results from part of the beak (i.e., the more heavily pigmented part) or to relax the rule if the study is on a rare species. This is particularly important when analysing beaks of juvenile cephalopods for which much of the structure is transparent, thus with higher chitin concentration than in the fully tanned beaks of adults (Clarke, 1986; Cherel et al., 2009a). This limitation can be overcome by removing the transparent part of the beak (Matias et al., 2019; Staudinger et al., 2019) or by using CSIA-AA which is not dependent on the amount of chitin in the beaks (Cherel et al., 2019; Woods et al., 2022). Regarding the use of different sections of the beaks for SIA, it should be noted that values obtained from the tip of the rostrum, sometimes considered as a proxy for juvenile life, result from a mixture of beak material deposited in early life stages and new beak material deposited over the life of the individual (Queirós et al., 2018). This suggests that in species that migrate and increase their trophic position throughout their life, δ13C values are higher or lower depending on the habitat occupied by the adult, and δ15N values are higher due to the higher trophic position in the later life-stage (Queirós et al., 2018).
Trace elements
Trace elements occur naturally in the environment, yet their concentrations are increasing due to anthropogenic activities (Sen and Peucker-Ehrenbrink, 2012). These elements can be essential (e.g., copper, iron, zinc) or non-essential (e.g., cadmium, mercury, lead), with both being potentially toxic at a given concentration (Jakimska et al., 2011). They may bio-accumulate throughout the life of individuals and some of them can bio-magnify through the food webs, with the main uptake being by prey ingestion (Szynkowska et al., 2018). Because of their importance in the food web, trace element concentrations have been extensively studied in cephalopods (Bustamante et al., 2000; Seixas et al., 2005; Pierce et al., 2008; Lischka et al., 2020; Seco et al., 2020). However, they have only recently been measured in cephalopod beaks (Fang et al., 2019; Lin et al., 2019; Northern et al., 2019). While numerous studies measured the concentration of mercury in beaks (Xavier et al., 2016; Matias et al., 2019; Queirós et al., 2020a), studies analyzing the concentration of other trace elements are rarer. Northern et al. (2019) measured the trace element concentrations in three different sections of Moroteuthopsis ingens lower beaks using both solution based inductively coupled plasma mass spectrometry (SB-ICP-MS) and laser ablation inductively coupled plasma mass spectrometry (LA-ICP-MS) methodologies. Both techniques were able to measure the concentrations of at least 23 elements, though LA-ICP-MS was able to detect three additional elements (i.e., Be, Y and Zr), that SB-ICP-MS did not detect, and also found more variability between beak sections (Northern et al., 2019). More studies are needed to understand whether this inequality is related to the methodology or to differences between the studied specimens.
For trace elements analyses, in contrast to SIA, the tip of the rostrum cannot be considered a proxy for the juvenile life phase as results obtained in this section are similar to those obtained in the end of the hood (Queirós et al., 2020a). Here, the anterior section of the hood is the most appropriate to study this life phase (Queirós et al., 2020a). Analyses of trace elements on beaks are useful for: 1) ecotoxicological studies (Xavier et al., 2016; Queirós et al., 2020a), 2) biogeographical studies to determine the distribution and migration of individuals (Fang et al., 2019; Northern et al., 2019), 3) ecological studies to evaluate the individuals trophic ecology and diet changes during ontogeny (Matias et al., 2019), and 4) the identification of cryptic species (Fang et al., 2021c). As cephalopods are usually widely distributed all around the world, measuring the concentrations of the different trace elements in beaks can also be used for biomonitoring the variability of contamination across ocean basins and, by using beaks preserved in collections, it may allow an evaluation of how concentrations of these elements have changed over time. Moreover, as performed in other taxa, such as bivalves and fish (Campana et al., 1994; Gomes et al., 2016), the analysis of trace elements over the life of individuals in beaks can be used to study the connectivity between different areas to help in the conservation of species and management of cephalopod fisheries.
It is worth noting that, when analysing trace element concentrations in cephalopod beaks, the resulting concentrations are significantly lower than those in other tissues such as muscle, digestive gland, or gills (Xavier et al., 2016; Matias et al., 2019; Matias et al., 2020). Although a previous study found a relationship between mercury concentrations in beaks and muscle in one Antarctic octopod species, which would suggest the former as a potential proxy for the mercury concentration of the flesh, no such relationship was found in other species (Matias et al., 2020). However, the analyses of trace element concentrations in different sections of the beaks may allow to determine differences (i.e., ratios) of elements concentrations in different life stages (Queirós et al., 2020a). For example, mercury concentrations on beaks suggest that adults of M. longimana have twice as more mercury than juveniles, which also may happen in the muscle (Queirós et al., 2020a). Ultimately, element concentrations measured in beaks can be used to estimate the concentration of the element that might be transferred from the prey to the predator.
Measuring trace element concentrations in cephalopod beaks does have some limitations. As beaks tend to have lower concentrations of the target elements, the detection limit of the techniques can be a problem, especially when looking for minor elements and when analysing subsections of the beaks for trace metal analyses. This should not be a problem when analysing entire beaks (except if they are from small species or very young individuals). When a specific equipment is available for an element, e.g., Advanced mercury analyser to mercury, its use should be prioritised as it is more sensitive to the element and enables the measurement of lower concentrations. Otherwise, LA-ICP-MS is probably the best option as it has a lower detection limit than other techniques (Fang et al., 2019; Northern et al., 2019; Fang et al., 2021c), and is thus suitable to determine concentrations of minor elements or to measure the concentrations of trace elements in small sections of the beaks. The amount of sample needed to perform some trace element analyses can also be a problem for both entire small beaks and/or beak sections. Here, as for SIA, pooling beaks with similar size, origin and time of sampling is a possible solution (Queirós et al., 2020a; Guímaro et al., 2021). These limitations also apply to the emerging compound-specific trace element analyses (Weiss et al., 2008; Wiederhold, 2015). Emerging compound-specific trace element analyses, such as mercury stable isotopes, can offer a great opportunity to delineate the vertical habitat of cephalopods, as already shown in sharks (Le Croizier G. et al., 2020; Le Croizier G.l. et al., 2020; Besnard et al., 2021) and seabirds (Renedo et al., 2018). Indeed, when using trace elements to study individual migrations, it is important to know if there is any ontogenetic change in the diet or trophic position, because as trace elements are mostly taken up by diet (Szynkowska et al., 2018), we need to be sure to be sure that changes in trace element concentrations are in fact related to changes of habitat and not with the trophic position of cephalopods.
Beak composition should always be considered in any of the analyses mentioned here. As some trace elements have a higher affinity for proteins, e.g., mercury (Bustamante et al., 2006), the changing protein:chitin ratio along the beak can potentially influence the result. Hence, it is important to be careful when analysing the results, especially when comparing different beak sections or beaks from different species for trace elements, sizes and maturation states (Queirós et al., 2020a). As with SIA, this limitation can be overcome with the compound specific trace elements analysis (Renedo et al., 2018; Cherel et al., 2019; Whiteman et al., 2019; Besnard et al., 2021).
DNA analyses
Genetic analysis plays an important role in the study of cephalopod systematics and evolution (Boyle and Rodhouse, 2005; Strugnell et al., 2011; Allcock et al., 2015; Bolstad et al., 2018). It has also been used to identify cephalopod flesh found in the stomachs of predators as well to study the role of cephalopods as predators (Deagle et al., 2005; Braley et al., 2010; Hoving et al., 2014; Olmos-Pérez et al., 2017; Fernández-Álvarez et al., 2018; Queirós et al., 2021b). These previous studies used the flesh, both muscle and buccal mass, of individuals from collections, or that were captured or washed up on the shore (Bolstad et al., 2018; Queirós et al., 2020b). As some cephalopods, in particular oceanic squids, tend to easily avoid capture and many predators prey/scavenge on them, extracting DNA from the beaks would be an important tool as it would facilitate the identification of some species whose beaks are very similar [e.g., Histioteuthis eltaninae and H. atlantica (Xavier and Cherel, 2021)], the identification of beaks belonging to undescribed species [e.g., Oegopsida sp. A, Taonius sp. (Clarke), Onychoteuthis sp. B (Imber) (Cherel et al., 2004; Cherel et al., 2011; Cherel, 2020)], and to study the phylogeny and taxonomy of these hard-to-catch species.
As far as we know, only one study was able to extract DNA from cephalopod beaks (Vecchione et al., 2009). These authors extracted DNA from a beak of Muusoctopus thielei collected from a whole specimen. Another successful tentative trial was made in the beaks of Architeuthis dux but the amount of extracted DNA was not enough to follow up with further research at the time (Tom Gilbert, personal communication). In contrast, Xavier et al. (2016) did not succeed in extracting DNA from beaks of M. longimana and Filippovia knipovitchi. When comparing the studies of both Vecchione et al. (2009) and Xavier et al. (2016) to understand what could influence DNA extraction, one primary difference is that the former study used fresh beaks retrieved from the individual, while the latter used non-fresh beaks from predators’ diet. After spending time in the stomach, the beaks lost their transparent part which is the area closer to the beccublast cells that synthesise the beak proteins (Tan et al., 2015). Further differences can also be found in the methodology used in both studies (Vecchione et al., 2009; Xavier et al., 2016). We believe that it is worthwhile to continue to attempt the extraction of DNA from cephalopod beaks, although we suggest that studies should focus on the transparent parts of the beaks, rather than the entire, fully sclerotized, beak. Nevertheless, the use of the transparent part of the beak could be a limitation for these methodologies in smaller species in which the transparent part is reduced, as well as in beaks from predators’ stomachs since the transparent part disappears with the action of the gastric acids (Clarke, 1986; Duffy and Jackson, 1986). Furthermore, we suggest trying different methodologies, including approaches that have been used to successfully extracted DNA from other difficult-to-handle issues e.g., bones or fossils (Vecchione et al., 2009; Xavier et al., 2016; Campos and Gilbert, 2019; Modi et al., 2021).
Structural analysis
The rostrum of cephalopod beaks is among the hardest and stiffest fully organic materials on Earth, while the lateral walls and wing areas of these beaks are generally soft and flexible (Miserez et al., 2008). Indeed, the beak rostrum can be harder than engineering polymers and present an intermediate response to blunt abrasion (Miserez et al., 2007). Because of these characteristics, beaks are seen as an inspiration for new engineering protein-based and environmentally load-bearing polymers (i.e., polymers that can support great amounts of weight) that can replicate properties of living organisms (Miserez et al., 2010; Linder, 2015; Sun et al., 2020). Furthermore, there is also an interest in chitosan, a biopolymer obtained from chitin (Miserez et al., 2008), which has applications in the food industry, pharmaceuticals, textiles and biotechnology (Morin-Crini et al., 2019). However, for such compounds to be replicated, it is important to understand how they are formed and what gives the beaks their unique properties since they do not contain metal ions, minerals, or halogens, which typically confer hardness and stiffness to biomaterials [e.g., Zinc (Zn) ions on polychaete jaws (Miserez et al., 2007; Linder, 2015)]. The determination of beak characteristics can also be used in the study the trophic ecology (by providing insights into what kind of prey could be eaten) (Matias et al., 2019).
Several studies analysed the structure and mechanical properties of cephalopod beaks, most of them using those of the jumbo squid Dosidicus gigas (Miserez et al., 2007; Miserez et al., 2008; Miserez et al., 2010; Tan et al., 2015). To our knowledge, apart from these studies, only Matias et al. (2019) have studied the mechanical properties of cephalopod beaks, using two Antarctic octopod species, Pareledone turqueti and Adelieledone polymorpha. The methodology used by all these studies was very similar [i.e., optical and scanning electron microscopy and high-resolution microcomputed tomography to determine the microstructure and structural features, x-ray diffraction to study the density, nanoindentation test to determine the beak mechanical properties and single-edge notched tension (SENT) to determine the fracture toughness]. In contrast to previous methodologies, only one limitation was found, and it was related to the size of the beak and its suitability for some tests (e.g., SENT test). This is why the authors decided to use very large D. gigas beaks (Miserez et al., 2007). The similarity between the techniques used to date suggests that future studies could use the same approach to study the structural properties of beaks, facilitating comparisons, providing insights into the ecology of the species and inspiring the engineering of new materials. Indeed, different results obtained for three species showed different hardness in the following sequence, from hardest to least hard: D. gigas > P. turqueti > A. polymorpha (Miserez et al., 2007; Matias et al., 2019).
Proteomics
The study of an organisms’ proteome can assume a major role in understanding how species will react to climate change, pollutants and other environmental stressors (Nunn and Timperman, 2007; Braconi et al., 2011; Tomanek, 2014). Indeed, previous studies showed that temperature, toxic trace elements, food limitation, or hormones can all affect the proteins in molluscs, as well as their amino acid pool (Veldhoen et al., 2012; Clark et al., 2017). Furthermore, an organisms’ proteome can help in species’ identification (Mazzeo and Siciliano, 2016). A proteomic approach has been used in cephalopods to understand their colours, toxins, host-parasite relationships and their immune system (Gestal and Castellanos-Martínez, 2015; Roumbedakis et al., 2018; Albertin and Simakov, 2020; Gonçalves and Costa, 2021). These studies used tissues such as skin (Crookes et al., 2004), slime (Caruana et al., 2016), saliva (Cornet et al., 2014), and in cuttlefish cuttlebones (Pabic et al., 2017).
In a pioneering study, Miserez et al. (2008) carried out the first analysis of the proteins in cephalopod beaks. Using beaks of the jumbo squid D. gigas, they found differences in the amino acids composition between the tanned and untanned areas (Miserez et al., 2008) and showed that the stiffness of the beaks was linked to the amount of certain proteins, identifying l-3,4-dihydroxyphenylalanine-histidine (dopa-His) as providing mechanical strength to the beak material. The authors subsequently found many cross-links were actually based on (His)-4-methylcatechol and not dopa-His (Miserez et al., 2008; Miserez et al., 2010). Following these studies, Tan et al. (2015) combined different transcriptomic (using mRNA from beccublast) and proteomic techniques to study the proteins in cephalopod beaks. They found the presence of two major families of proteins: the chitin-binding proteins (DgCBPs) and the histidine-rich beak proteins (DgHBPs), the former new to science (Tan et al., 2015). However, the precise distribution of each protein in the beak remains unknown, and only estimations using its wet mass are available (Tan et al., 2015). Despite this major step, it is still true that very little is known about the proteome of cephalopod beaks, especially because these studies focused only on one species and, as proteins are related to stiffness and beaks of different species have different stiffness structural analyses (Miserez et al., 2008), it is important to know if these proteins are the same for all species. Additionally, the presence of stress marks during the formation of beaks suggests changes during their formation that can be related to proteins (Perales-Raya et al., 2014a).
The study of cephalopod beak proteins is important to protein engineering as they can be a model for liquid-liquid phase separation (Sun et al., 2020). Another advantage of studying beak proteins, as with other techniques, is their availability in collections and the possibility of using predators as biological samplers. This suggests that cephalopod beaks can be used to study environmental changes through time but also be useful to study impacts of environmental stressors in species that are challenging to sample. As proteomics can also be used in evolutionary and ecological studies (Diz et al., 2012), it also has the potential to help in the study of cephalopod evolution. However, there are known limitations to studying beak proteins, and major uncertainties since this is a very new field of study. The major limitation found by Tan et al. (2015) is that classic protein extraction protocols do not work on beaks. They overcame this limitation by using non-enzymatic reagents that cleave peptide bonds, releasing them from the beak structure (Tan et al., 2015). However, other strategies are needed to extract beak proteins without destroying them. Furthermore, it is still unknown whether different methods of preservation have different effects on proteins and whether proteins are still present in beaks from museum collections.
Future challenges in research in cephalopods beaks
Regarding the use of beaks for taxonomy, some relevant key features, limitations and perspectives are outlined below. Most previous investigations used lower beaks only (Clarke, 1986; Xavier et al., 2007a; Xavier et al., 2015). However, discarding upper beaks is a potential loss of information that was highlighted in subsequent investigations (Cherel et al., 2000; Cherel et al., 2017). Hence, both lower and uppers beaks should be included in future studies (Xavier et al., 2011). Also, care is needed when using names of species assigned to beaks in older publications, due to a combination of past misidentifications and subsequent improvements in both beak identification and cephalopod taxonomy over the last few decades (Cherel, 2020, 2021). Also, beaks are routinely used in the studies of trophic ecology of predatory species to estimate prey length and mass. However, regression formulas of relationships between beak size and length and mass of cephalopods are lacking for the majority of species. Extensive collection and routine publishing of such data is an essential task in cephalopod research in the future. Another challenge is that, despite the recent global revision of some families (e.g., Onychoteuthidae) (Bolstad, 2010; Bolstad et al., 2018), cephalopod taxonomy is still problematic: the chaotic state of some taxa precludes identifying beaks to the species level with confidence in many cases (e.g., Brachioteuthidae, Chiroteuthidae). Improvement in beak identification requires that both taxonomic revision and the description of new species include drawings and/or photos of the lower and upper beaks, ideally from early stages to mature adults. It also requires exploring new methods to extract DNA from biological samples in poor condition. In most cases, as reported above, conventional procedures fail to extract DNA from partly digested cephalopod flesh, thus preventing the use of buccal masses from food samples to confirm/inform and thus improve cephalopod identification based on the corresponding beaks. This loss of information is unfortunate because most oceanic squids are notably difficult to catch using traditional means, while some species form a significant part of predators’ diet. In a few cases, the reverse is true, with beaks helping to solve systematic issues. For example, conventional examination of squid morphology and anatomy failed to find differences between Histioteuthis bonnellii bonnellii and H. b. corpuscula (Voss et al., 1998) [presently considered synonym: H. bonnellii (MolluscaBase, 2022)], while both beak morphology and size clearly indicate that they belong to different taxa (Clarke, 1980), a finding that merits further genetic investigation using the new generation of efficient DNA tools. Finally, identifying beaks from their morphology is time-consuming and needs expertise. We thus recommend getting expert advice before attributing a species name to a beak (Xavier and Cherel, 2021). Unfortunately, the most important bottleneck of the method now is the low and decreasing number of experts, meaning that efforts must be made to train early career researchers to identify cephalopod beaks from their morphology. The value of conventional photographic guides of cephalopods beaks for training and species identification should be recognized. Existing beak identification guides have covered only minor part of cephalopods diversity and their regional morphological variability. Therefore, the development of new extended guides or web-based solutions (see examples above) are still essential. Additionally, simultaneous collaborative efforts focused on collection of beaks, their photographs and DNA samples deposited as public web-based resource may also serve as important reference database for cephalopod identification in the future, as it already occurs with fish (i.e., AFORO as an example for collaborative otoliths database (http://aforo.cmima.csic.es/) (Lombarte et al., 2006).
A promising alternative or supplement to identification of cephalopods based on the morphology of their beaks is the development of a software for geometric morphometric-based automatic identification of the beaks. However, this approach also has some limitations. The first issue to solve is the development of efficient methods of acquisition of images for processing. At the moment, there is no agreement about the equipment to use, from which angles to record images, and how to take photos of the beaks. Some authors used 2-D images of the beak’s lateral view (Fang et al., 2017; Jin et al., 2017; Tan et al., 2021), others used a complex system of mirrors to obtain combined 2-D images of frontal and lateral views (Crespi-Abril et al., 2010), and others used a combination of underwater photogrammetry and CT scanning to obtain 3-D images of beaks (Roscian et al., 2022). As results of studies based on 2-D images suggest, this is enough for routine identification of abundant species (Tan et al., 2021), although in-depth ecological, paleontological, or taxonomic studies may demand more complex method (Roscian et al., 2022). We argue that the use of beaks for identification purposes demands a simple approach, which can be adopted both in the field and in the laboratory. From this point of view, acquisition of 2-D images of the beak’s lateral view seems more promising (Fang et al., 2017; Tan et al., 2021), but further studies on the loss of information and accuracy, when in using images of only the lateral view, are still needed.
Another issue is the selection of methods for analysis of images. Landmark-based methods of analysis have plenty of advantages in this regard, specifically when it comes to preserving ecologically or taxonomically meaningful information, but approach requires experienced users. On another hand, outline-based methods more suitable for automation of the process, since they do not require the user to select the points of interest or otherwise fine-tune the analysis. Thus, the software for automatic identification of the cephalopods based on the shape of their beaks may be based on Fourier or wavelet transformation, similar to the system developed for fish identification (Lombarte et al., 2006). It should be noted that accuracy of such an approach to identification may be improved if supplemented by the analysis of beak’s pigmentation (Fang et al., 2017), or if only the pigmented part is analysed (Lishchenko and Jones, 2021).
Further studies on a large number of taxa, testing the phylogenetic signal carried by beak shape will be necessary to establish the level of accuracy that could be achieved in identification. Moreover, as 3-D reconstruction is time-consuming and more complex than 2-D analyses, recent work using 3-D geometric morphometrics, which allows the complexity of beak shape to be better captured, has shown that the phylogenetic signal of upper and lower beaks is significant but moderate and that it cannot explain all the morphological variation in beaks on its own (Roscian et al., 2022). On the other hand, cephalopod beaks remain complex to image in three dimensions without damaging them. The use and improvement of advanced imaging technologies such as X-ray tomography and underwater photogrammetry on small objects will allow most species to be digitized (Roscian et al., 2021; Ziegler and Sagorny, 2021; Roscian et al., 2022). Nevertheless, the digitization of specimens is a long task and the accumulation and accessibility of the data to the community is a major challenge in achieving a comprehensive sampling of cephalopod 3-D model beaks. These 3-D models could indeed allow us to thoroughly renew our understanding of the signal carried by the shape of the beaks and be very complementary to 2-D analyses. Recently, Roscian et al. (2022) showed, using 3-D geometric morphometrics, that there is a likely link between ecological parameters such as habitat and trophic level and beak shape. These outcomes are in accordance with those obtained for squid and octopod paralarvae indicating a relationship between beak shape variation and diet shifts (Franco-Santos et al., 2014; Franco-Santos and Vidal, 2014; Franco-Santos and Vidal, 2020).
The study of developmental features of the beak during the early ontogeny of cephalopods is a nearly unexplored field of study. Much remains to be investigated in relation to the developmental pattern, shape and chemical composition of the beak and its relationship with the feeding ecology during early life. These studies encourage further research into the analysis of beak shape in relation to the ecology of the taxa, to test whether adaptive traits can be identified in these structures at any phase of the life cycle. To achieve this goal, it will be necessary not only to have many 3-D models but also to collect more data on the diets, habitats and trophic positions of species for which information remains scarce or poorly known, such as some deep-sea octopods or squids (i.e., some members of the genus Opisthoteuthis, the pelagic Vitreledonella richardi or Asperoteuthis lui). This approach will open new opportunities to study the evolutionary history of cephalopods, as it will be possible to integrate fossil forms. Although the contribution of fossil beaks to the reconstruction of the evolutionary history of coleoids is challenging due to the deformations of the fossil record and their rarity, methods are now available to overcome these problems (Hughes and Jell, 1992; Schlager et al., 2018; Demuth et al., 2022). Quantitative comparative analyses of modern and fossil beaks are now achievable and should produce significant advances in paleoecological interpretation of selected fossil forms.
Regarding using beaks in age and growth studies, some key relevant features, limitations and perspectives of the field are outlined below. When working on age determination of a species for the first time, exploration of both lateral wall surfaces and rostrum sagittal sections is mandatory. Increments do not always present regular pattern in the lateral wall surfaces, but they usually do so in the rostrum sagittal sections. Moreover, both upper and lower beaks need also to be examined to select the most suitable for the species of interest. Some species exhibit high erosion and irregular increment sequences in lateral wall surfaces of upper beaks (e.g., Architeuthis and Moroteuthopsis), whereas in others (e.g., Octopus vulgaris) the upper beaks are the most suitable for age estimation. When using beaks from cephalopod predators (stomach contents), the loss of material from the external border of these beaks might be substantial, presenting problems if the lateral wall surfaces are used for age estimation, therefore the information on how long it takes for the transparent part of lateral walls to disappear would help to prevent age underestimations. On the other hand, it is necessary to know how long the beak has been in the stomach to have a proxy of the death day of the specimen. These issues are covered in depth further down since further research is desirable. Age validation experiments are scarce in beaks, but they are required to confirm the periodicity of deposition of the growth increments in the species of interest. Mark-recapture methods in wild populations are expensive but others such as captive experiments, using marking or known-age specimens, are suitable for age validation when aquaculture facilities are accessible and the species can live in captivity for some time. It is also important to validate the age of the first increment, not only the temporal deposition of increments, to obtain reliable age estimations. When the species of interest is unsuitable for validation experiments (e.g., deep-water species), cross-verification by comparing with other validated structures (e.g., statoliths in squids or vestigial shells in octopuses), is an alternative.
The observation, counting and analysis of daily increments is time-consuming. The life-mode approach to performing semi-automated counts is advisable but not usually available in current image analysis systems. Artificial intelligence could provide a useful tool to save time and improve the detection and count of increments since it could “learn” from the images previously analysed by experienced readers. Finally, the cumulative width of the daily increments could be a potential tool to estimate growth in the wild, before capture (e.g., Perales-Raya et al. (2020) for Architeuthis dux; Queirós, Bartolomé, Xavier and Perales-Raya, unpublished for M. longimana). Preliminary results on reared O. vulgaris used correlations between cumulative widths and body mass to estimate the growth in the wild, before capture (Perales-Raya, Bartolomé, Márquez, Felipe and Almansa, unpublished data). Moreover, future research should also further evaluate beak growth under warming (e.g., climate change scenarios) under laboratory conditions, which is known to cause thermal stress in cephalopod beaks (E.g., in Octopus vulgaris) (Perales-Raya et al., 2014a) (Perales-Raya et al., 2014b), in order to validate the magnitude of such beak marks and their ecological implications.
The application of different chemical and structural analyses on cephalopod beaks to study different aspects of the species and individual life-cycle is increasing, though with some techniques being well-established in cephalopods (e.g., stable isotopic analyses), while others are still in development (e.g., proteomics). Nevertheless and independently of whether a method is “established,” all methods present issues and challenges that should be addressed in the future. Stable isotopic analysis, as is the case for most of the techniques applied on beaks, is dependent on the chitin:protein ratio, which varies throughout the beak. Future studies should evaluate whether the variation of the chitin:protein ratio is similar across species. Furthermore, as a higher proportion of chitin results in lower δ15N values, a correction factor for chitin, similar to those used in stable isotopic analyses on muscle for lipids (Hobson and Cherel, 2006), should be found to enable the direct comparison of δ15N values between different sections of the beak and different life-stages and to compare with other tissues. Several studies applied SIA on beaks from predators’ stomachs, with most of these determining the habitat using known gradients of δ13C values in the environment (Cherel and Hobson, 2005; Guerreiro et al., 2015; Abreu et al., 2020), especially when using different sections of the beak (Guerra et al., 2010; Queirós et al., 2018). If the capture location is known, the δ13C value of the last formed beak material could be used as a baseline that helps to determine the movement of the individual. However, to have an idea of the capture location if the sample came from stomach contents, it is important to know how long the beak remained in the stomach. Although there is some information on the amount of time a beak can stay in a predator stomach (Ashmole and Ashmole, 1967; Clarke, 1980; Jackson and Ryan, 1986; Gales and Cheal, 1992; Xavier et al., 2011), future experimental studies are needed to understand the average time a beak takes to be egested in relation to the type of predator and how long it takes for transparent parts of a beak to disappear, depending on both its own size and darkening stage as well as on the predator biology. Consequently, it is essential for diet studies to distinguish the beaks that are from recently eaten prey (i.e., beaks recently consumed by predators that still have flesh attached, beaks in buccal masses or from complete or partially digested specimens) from those which have been in the stomach a long time and may be eroded (i.e., beaks without transparent parts or flesh attached).
SIA is also dependent on a baseline value and, despite some previous studies determining POM isotopic values for the different areas or modelling these values, future studies should update these values, partly because they may change over time, as well as using beaks from individuals with known capture location to create an isoscape specifically for cephalopod beaks. Apart from the baselines, future studies should focus on establishing a specific trophic enrichment factor for cephalopod beaks (ideally for each cephalopod species in a given region) that enables the study of the trophic level of an individual using an enrichment factor adapted for these structures rather than general enrichment factors that are common for all marine organisms.
Regarding the trace elements analyses, further studies should investigate in detail whether the differences found between results from different techniques used in previous studies (i.e., SB-ICP-MS and LA-ICP-MS) (Northern et al., 2019), are a consequence of the technique or simply a function of which beaks were used. It is also important that future studies investigate the relationship between element concentrations in the beaks and those found in other tissues of the individual. Due to the different chitin:protein ratio along the beak and the different affinity that some elements have with proteins, it is important that future studies evaluate how the change in beak composition can affect the concentrations of the different elements, and look in detail at the rostrum as this part of the beak may be prone to accumulate high levels of some elements, not working as a proxy for the juvenile life-phase as it does in SIA (Queirós et al., 2020a). Additionally, it is important to evaluate the usefulness of beaks as proxy of environmental pollution, such as studying these trace elements in beaks related to pollution (e.g., increased mercury levels due to anthropogenic sources), to provide a proxy of pollution in cephalopods.
Concerning the most recent techniques applied to beak structure and protein composition, we suggest that the various available methodologies are tested in order to find which are the most efficient. Considering previous studies, we suggest that future research aiming to extract DNA from (fresh) beaks from predators’ stomachs that still have their transparent parts, as well as the use of techniques that have been shown to recover DNA material from fossils or bones. Regarding structure analyses, we suggest that future studies should aim to develop an understanding of the interspecific variability in the beak structure and how it can influence, for example, the diet of each species. The study of the beak proteins is, as far as we know, one of the most recent techniques that has been applied to these structures. Based on its importance for beak composition and its influence on the ecology of the species, we suggest that new techniques should be explored: techniques used in the past able to retrieve proteins, though destroying the beaks, should be applied in other species to understand the inter-species variability. Future studies should also explore the effect of the different preservation methods on the beak proteins to evaluate whether beaks in museum collections can be used in these studies.
Author contributions
The workshop was planned and organized by JX, AG, JQ, CP-R, RR-L, and YC who produced the initial framework of the manuscript. The final structure of the manuscript and text was based on the contribution of all authors during and after the workshop. These contributions were initially gathered by JX, JQ, and CP-R and then reviewed and accepted by all authors.
Acknowledgments
This study benefited from the strategic program of the Marine and Environmental Sciences Centre (MARE), financed by the FCT (UIDB/704292/2020). This project was also supported through funding from the European Union’s Horizon 2020 research and innovation programme under the Marie Skłodowska-Curie grant agreement No. 101065960 granted to AG. JQ is supported by FCT PhD scholarship co-financed by FSE (SFRH/BD/144320/2019). CP-R would like to thank the support of the project OCTOMICS (AGL 201789475-C2-1-R) and the EU-FEDER funds. ÁG also thank the support of the project ECOSUMA (PID 2019-110088RB-I00). The IUF (Institut Universitaire de France) is acknowledged for its support to PB as a senior member. VD was supported by NSF REU Site EAR-1062692, as well as through a Natural History Research Experiences summer internship at the Smithsonian Institution. AG-M was supported by a PhD-fellowship (PIFULPGC-2017-CIENCIAS-2) from the University of Las Palmas de Gran Canaria. Participation of ER in the workshop on cephalopod beaks and studies of cephalopods in Reunion Island was supported by the Project DECAPOT funded by EU FEDER and la Région Réunion. AS-M was supported by a PhD-fellowship (ref. PRE 2021-099558) associated to the project BITER (PID 2020-114732RB-C31, Ministerio de Ciencia e Innovación, Spanish Government). LS was supported by a Human Frontier Science Program Long-term fellowship (LT000476/2021-L). EV would like to thank support from the Brazilian National Research Council (CNPq # 316391/2021-2). Finally, the authors acknowledge the journals Marine Ecology Progress Series, Journal of Shellfish Research and Bulletin of Marine Science for their approval of our permission requests.
Conflict of interest
The authors declare that the research was conducted in the absence of any commercial or financial relationships that could be construed as a potential conflict of interest.
Publisher’s note
All claims expressed in this article are solely those of the authors and do not necessarily represent those of their affiliated organizations, or those of the publisher, the editors and the reviewers. Any product that may be evaluated in this article, or claim that may be made by its manufacturer, is not guaranteed or endorsed by the publisher.
References
Abreu J., Phillips R. A., Ceia F. R., Ireland L., Paiva V. H., Xavier J. C. (2020). Long-term changes in habitat and trophic level of Southern Ocean squid in relation to environmental conditions. Sci. Rep. 10, 15215. doi:10.1038/s41598-020-72103-6
Abreu J., Staniland I. J., Rodrigues C. F., Queirós J. P., Pereira J. M., Xavier J. C. (2019). Squid in the diet of antarctic fur seals: Potential links to oceanographic conditions and antarctic krill abundance. Mar. Ecol. Prog. Ser. 628, 211–221. doi:10.3354/meps13100
Açik S., Salman A. (2010). Estimation of body size from the beaks of eight sepiolid species (Cephalopoda: Sepiolidae) from the Eastern Mediterranean Sea. Molluscan Res. 30, 154–164.
Acuña-Perales N., Córdova-Zavaleta F., Arrese-Dávila V., Torrejón-Zegarra R., Rodríguez-Salazar J., González-Pestana A., et al. (2020). Guía de identificación de picos de algunas especies de cefalópodos obtenidos en el Mar Peruano. ProDelphinus, Lima, Peru. 26.
Adams D. C., Rohlf F. J., Slice D. E. (2004). Geometric morphometrics: Ten years of progress following the “revolution”. Ital. J. Zool. 71 (1), 5–16. doi:10.1080/11250000409356545
Akimushkin I. I. (1965). “Cephalopods of the seas of the USSR. Moscow: Nauka,” in Israel program for scientific translations Jerusalem: IPST. (Translated from Russian).
Albertin C. B., Simakov O. (2020). Cephalopod biology: At the intersection between genomic and organismal novelties. Annu. Rev. Anim. Biosci. 8, 71–90. doi:10.1146/annurev-animal-021419-083609
Allcock A. L., Lindgren A., Strugnell J. (2015). The contribution of molecular data to our understanding of cephalopod evolution and systematics: A review. J. Nat. Hist. 49 (21-24), 1373–1421. doi:10.1080/00222933.2013.825342
Anderson F. E., Lindgren A. R. (2021). Phylogenomic analyses recover a clade of large-bodied decapodiform cephalopods. Mol. Phylogenet. Evol. 156, 107038. doi:10.1016/j.ympev.2020.107038
Arkhipkin A. I., Bizikov V. A., Doubleday Z. A., Laptikhovsky V. V., Lishchenko F. V., Perales-Raya C., et al. (2018). Techniques for estimating the age and growth of molluscs: Cephalopoda. J. Shellfish Res. 37 (4), 783–792. doi:10.2983/035.037.0409
Arkhipkin A. I., Bizikov V. A., Fuchs D. (2012). Vestigial phragmocone in the gladius points to a deepwater origin of squid (Mollusca: Cephalopoda). Deep Sea Res. Part I Oceanogr. Res. Pap. 61, 109–122. doi:10.1016/j.dsr.2011.11.010
Arkhipkin A. I., Hendrickson L. C., Payá I., Pierce G. J., Roa-Ureta R. H., Robin J.-P., et al. (2021). Stock assessment and management of cephalopods: Advances and challenges for short-lived fishery resources. ICES J. Mar. Sci. 78 (2), 714–730. doi:10.1093/icesjms/fsaa038
Arkhipkin A. I., Rodhouse P. G. K., Pierce G. J., Sauer W., Sakai M., Allcock L., et al. (2015). World squid fisheries. Rev. Fish. Sci. Aquac. 23, 92–252. doi:10.1080/23308249.2015.1026226
Armelloni E. N., Lago-Rouco M. J., Bartolomé A., Felipe B. C., Almansa E., Perales-Raya C. (2020). Exploring the embryonic development of upper beak in Octopus vulgaris Cuvier, 1797: New findings and implications for age estimation. Fish. Res. 221, 105375. doi:10.1016/j.fishres.2019.105375
Ashmole N. P., Ashmole M. J. (1967). Comparative feeding ecology of seabirds of a tropical oceanic island. Bull. Peabody Mus. Nat. Hist. 24, 1–131.
Bárcenas G. V., Perales-Raya C., Bartolomé-Baraza A., Almansa E., Rosas C. (2014). Age validation in Octopus maya (Voss and Solís, 1966) by counting increments in the beak sections of known age individuals. Fish. Res. 152, 93–97. doi:10.1016/j.fishres.2013.08.007
Barrett R. T., Camphusysen C. J., Anker-Nilssen T., Chardine J. W., Furness R. W., Garthe S., et al. (2007). Diet studies of seabirds: A review and recommendations. ICES J. Mar. Sci. 64, 1675–1691. doi:10.1093/icesjms/fsm152
Batista B., Matthews-Cascon H., Marinho R., Kikuchi E., Haimovici M. (2021). The growth and population dynamics of Octopus insularis targeted by a pot longline fishery in north-eastern Brazil. J. Mar. Biol. Assoc. U. K. 101 (6), 935–946. doi:10.1017/S0025315421000898
Bearhop S., Adams C. E., Waldron S., Fuller R. A., MacLeod H. (2004). Determining trophic niche width: A novel approach using stable isotope analysis. J. Anim. Ecol. 73 (5), 1007–1012. doi:10.1111/j.0021-8790.2004.00861.x
Bello G. (1991). Role of cephalopods in the diet of the swordfish, Xiphias gladius, from the eastern Mediterranean Sea. Bull. Mar. Sci. 49, 312–324.
Bello G., Travaglini A., Bentivegna F. (2011). Histioteuthis bonnellii (Cephalopoda: Histioteuthidae): A new prey item of the leatherback turtle Dermochelys coriacea (reptilia: Dermochelidae). Mar. Biol. Res. 7 (3), 314–316. doi:10.1080/17451000.2010.492224
Besnard L., Le Croizier G., Galván-Magaña F., Point D., Kraffe E., Ketchum J., et al. (2021). Foraging depth depicts resource partitioning and contamination level in a pelagic shark assemblage: Insights from mercury stable isotopes. Environ. Pollut. 283, 117066. doi:10.1016/j.envpol.2021.117066
Bettencourt V., Guerra A. (2000). Growth increments and biomineralization process in cephalopod statoliths. J. Exp. Mar. Biol. Ecol. 248 (2), 191–205. doi:10.1016/S0022-0981(00)00161-1
Bettencourt V., Guerra A. (2001). Age studies based on daily growth increments in statoliths and growth lamellae in cuttlebone of cultured Sepia officinalis. Mar. Biol. 139 (2), 327–334. doi:10.1007/s002270100582
Boletzky S. V. (1971). Mandibules denticulées chez les larves des Teuthoidées et des Octopodes (Mollusca, Cephalopoda). Comptes Rendus l’Academie Sci. Paris, Série272, 2904–2906.
Bolstad K. S., Braid H. E., Strugnell J. M., Lindgren A. R., Lischka A., Kubodera T., et al. (2018). A mitochondrial phylogeny of the family Onychoteuthidae (Cephalopoda: Oegopsida). Mol. Phylogenet. Evol. 128, 88–97. doi:10.1016/j.ympev.2018.05.032
Bolstad K. S. (2006). Sexual dimorphism in the beaks of Moroteuthis ingens Smith, 1881 (Cephalopoda: Oegopsida: Onychoteuthidae). N. Z. J. Zool. 33, 317–327. doi:10.1080/03014223.2006.9518459
Bolstad K. S. (2010). Systematics of the Onychoteuthidae Gray, 1847 (Cephalopoda: Oegopsida). Zootaxa 2696, 1–186. doi:10.11646/zootaxa.2696.1.1
Bookstein F. L. (1997). Landmark methods for forms without landmarks: Morphometrics of group differences in outline shape. Med. Image Anal. 1 (3), 225–243. doi:10.1016/S1361-8415(97)85012-8
Bookstein F. L. (1991). Morphometric tools for landmark data: Geometry and biology. Cambridge, UK: Cambridge University Press.
Braconi D., Bernardini G., Santucci A. (2011). Linking protein oxidation to environmental pollutants: Redox proteomic approaches. J. Proteomics 74 (11), 2324–2337. doi:10.1016/j.jprot.2011.06.029
Braley M., Goldsworthy S. D., Page B., Steer M., Austin J. J. (2010). Assessing morphological and DNA-based diet analysis techniques in a generalist predator, the arrow squid Nototodarus gouldi. Mol. Ecol. Resour. 10 (3), 466–474. doi:10.1111/j.1755-0998.2009.02767.x
Bustamante P., Grigioni S., Boucher-Rodoni R., Caurant F., Miramand P. (2000). Bioaccumulation of 12 trace elements in the tissues of the nautilus Nautilus macromphalus from New Caledonia. Mar. Pollut. Bull. 40 (8), 688–696. doi:10.1016/S0025-326X(00)00005-9
Bustamante P., Lahaye V., Durnez C., Churlaud C., Caurant F. (2006). Total and organic Hg concentrations in cephalopods from the North Eastern Atlantic waters: Influence of geographical origin and feeding ecology. Sci. Total Environ. 368 (2), 585–596. doi:10.1016/j.scitotenv.2006.01.038
Cadrin S. X. (2014). “Morphometric landmarks,” in Stock identification methods. Editors S. X. Cadrin, L. A. Kerr, and S. Mariani (London: Elsevier), 109–128.
Campana S. E. (2001). Accuracy, precision and quality control in age determination, including a review of the use and abuse of age validation methods. J. Fish. Biol. 59 (2), 197–242. doi:10.1111/j.1095-8649.2001.tb00127.x
Campana S. E., Fowler A. J., Jones C. M. (1994). Otolith elemental fingerprinting for stock identification of Atlantic cod (Gadus morhua) using laser ablation ICPMS. Can. J. Fish. Aquat. Sci. 51 (9), 1942–1950. doi:10.1139/f94-196
Campos P. F., Gilbert M. T. P. (2019). “DNA extraction from keratin and chitin,” in Ancient DNA: Methods and protocols. Editors B. Shapiro, A. Barlow, P. D. Heintzman, M. Hofreiter, J. L. A. Paijmans, and A. E. R. Soares (New York, NY: Springer), 57–63.
Canali E., Ponte G., Belcari P., Rocha F., Fiorito G. (2011). Evaluating age in Octopus vulgaris: Estimation, validation and seasonal differences. Mar. Ecol. Prog. Ser. 441, 141–149. doi:10.3354/meps09399
Caruana N. J., Cooke I. R., Faou P., Finn J., Hall N. E., Norman M., et al. (2016). A combined proteomic and transcriptomic analysis of slime secreted by the southern bottletail squid, Sepiadarium austrinum (Cephalopoda). J. Proteomics 148, 170–182. doi:10.1016/j.jprot.2016.07.026
Castanhari G., Tomás A. R. G. (2012). Beak increment counts as a tool for growth studies of the common octopus Octopus vulgaris in southern Brazil. Bol. Inst. Pesca, São Paulo 38 (4), 323–331.
Castro J. J., Hernández-Garcia V. (1995). Ontogenetic changes in mouth structures, foraging behaviour and habitat use of Scomber japonicus and Illex coindetii. Sci. Mar. 59, 347–355.
Cherel Y. (2020). A review of Southern Ocean squids using nets and beaks. Mar. Biodivers. 50, 98. doi:10.1007/s12526-020-01113-4
Cherel Y., Bustamante P., Richard P. (2019). Amino acid δ13C and δ15N from sclerotized beaks: A new tool to investigate the foraging ecology of cephalopods, including giant and colossal squids. Mar. Ecol. Prog. Ser. 624, 89–102. doi:10.3354/meps13002
Cherel Y., Ducatez S., Fontaine C., Richard P., Guinet C. (2008). Stable isotopes reveal the trophic position and mesopelagic fish diet of female southern elephant seals breeding on the Kerguelen Islands. Mar. Ecol. Prog. Ser. 370, 239–247. doi:10.3354/meps07673
Cherel Y., Duhamel G., Gasco N. (2004). Cephalopod fauna of subantarctic islands: New information from predators. Mar. Ecol. Prog. Ser. 266, 143–156. doi:10.3354/meps266143
Cherel Y., Fontaine C., Jackson G. D., Jackson C. H., Richard P. (2009a). Tissue, ontogenic and sex-related differences in δ13C and δ15N values of the oceanic squid Todarodes filippovae (Cephalopoda: Ommastrephidae). Mar. Biol. 156, 699–708. doi:10.1007/s00227-008-1121-x
Cherel Y., Gasco N., Duhamel G. (2011). “Top predators and stable isotopes document the cephalopod fauna and its trophic relationships in Kerguelen waters,” in The kerguelen plateau: Marine ecosystem and fisheries. Editors G. Duhamel, and D. Welsford (Paris: Société Française d’Ichtyologie), 99–108.
Cherel Y., Hobson K. (2005). Stable isotopes, beaks and predators: A new tool to study the trophic ecology of cephalopods, including giant and colossal squids. Proc. Biol. Sci. 272, 1601–1607. doi:10.1098/rspb.2005.3115
Cherel Y., Hobson K. A. (2007). Geographical variation in carbon stable isotope signatures of marine predators: A tool to investigate their foraging areas in the Southern Ocean. Mar. Ecol. Prog. Ser. 329, 281–287. doi:10.3354/meps329281
Cherel Y., Klages N. (1998). “A review of the food of albatrosses,” in Albatross biology and conservation. Editors G. Robertson, and R. Gales (Chipping Norton, Australia: Surrey Beatty & Sons), 113–136.
Cherel Y. (2021). Revisiting taxonomy of cephalopod prey of sperm whales caught commercially in subtropical and Southern Ocean waters. Deep Sea Res. Part I Oceanogr. Res. Pap. 169, 103490. doi:10.1016/j.dsr.2021.103490
Cherel Y., Ridoux V., Spitz J., Richard P. (2009b). Stable isotopes document the trophic structure of a deep-sea cephalopod assemblage including giant octopod and giant squid. Biol. Lett. 5, 364–367. doi:10.1098/rsbl.2009.0024
Cherel Y., Weimerskirch H. (1999). Spawning cycle of onychoteuthid squids in the southern Indian ocean: New information from seabird predators. Mar. Ecol. Prog. Ser. 188, 93–104. doi:10.3354/meps188093
Cherel Y., Weimerskirch H., Trouve C., Bost C. (2000). Food and feeding ecology of the neritic-slope forager black-browed albatross and its relationships with commercial fisheries in Kerguelen waters. Mar. Ecol. Prog. Ser. 207, 183–199. doi:10.3354/meps207183
Cherel Y., Xavier J. C., de Grissac S., Trouvé C., Weimerskirch H. (2017). Feeding ecology, isotopic niche, and ingestion of fishery-related items of the wandering albatross Diomedea exulans at Kerguelen and Crozet Islands. Mar. Ecol. Prog. Ser. 565, 197–215. doi:10.3354/meps11994
Clark M. S., Sommer U., Sihra J. K., Thorne M. A., Morley S. A., King M., et al. (2017). Biodiversity in marine invertebrate responses to acute warming revealed by a comparative multi-omics approach. Glob. Chang. Biol. 23 (1), 318–330. doi:10.1111/gcb.13357
Clarke M. (1965). Growth rings in the beaks of the squid Moroteuthis ingens (Oegopsida: Onychoteuthidae). Malacologia 3, 287–307.
Clarke M. R. (1978). The cephalopod statolithan-introduction to its form. J. Mar. Biol. Assoc. U. K. 58 (3), 701–712. doi:10.1017/S0025315400041345
Clarke M. R. (1986). A handbook for the identification of cephalopod beaks. Oxford: Clarendon Press.
Clarke M. R. (1993). “Age determination and common sense - a free discussion on difficulties encountered by the author,” in Recent advances in cephalopod fisheries biology. Editors T. Okutani, R. K. O´Dor, and T. Kubodera (Tokyo: Tokai University Press), 752.
Clarke M. R., Allcock L., Santos M. B. (2002). Estimating cephalopod biomass: Workshop report. Bull. Mar. Sci. 71, 47–65.
Clarke M. R. (1980). “Cephalopoda in the diet of sperm whales of the southern hemisphere and their bearing on sperm whale biology,” in Discovery reports. Cambridge, UK: Cambridge University Press, 1–324.
Clarke M. R. (1996). Cephalopods as prey.III. Cetaceans. Phil. Trans. R. Soc. Lond. B 351, 1053–1065.
Clarke M. R., Maddock L. (1988). “Beaks of living coleoid Cephalopoda,” in Paleontology and neontology of cephalopods. Editors M. R. Clarke, and E. R. Trueman (London: Academic Press), 121–131. The Mollusca.
Clarke M. R., Stevens J. D. (1974). Cephalopods, blue sharks and migration. J. Mar. Biol. Assoc. U. K. 54, 949–957. doi:10.1017/s0025315400057672
Cobb C. S., Pope S. K., Williamson R. (1995). Circadian rhythms to light-dark cycles in the lesser octopus, Eledone clrrhosa. Mar. Freshw. Behav. Physiol. 26, 47–57. doi:10.1080/10236249509378927
Cornet V., Henry J., Corre E., Le Corguille G., Zanuttini B., Zatylny-Gaudin C. (2014). Dual role of the cuttlefish salivary proteome in defense and predation. J. Proteomics 108, 209–222. doi:10.1016/j.jprot.2014.05.019
Crespi-Abril A. C., Morsan E. M., Barón P. J. (2010). Analysis of the ontogenetic variation in body and beak shape of the Illex argentinus inner shelf spawning groups by geometric morphometrics. J. Mar. Biol. Assoc. U. K. 90 (3), 547–553. doi:10.1017/s0025315409990567
Crookes W. J., Ding L.-L., Huang Q. L., Kimbell J. R., Horwitz J., McFall-Ngai M. J. (2004). Reflectins: The unusual proteins of squid reflective tissues. Science 303 (5655), 235–238. doi:10.1126/science.1091288
Croxall J. P., Prince P. A. (1996). Cephalopods as prey: Seabirds. Philos. Trans. R. Soc. Lond. B 351, 1023–1043. doi:10.1098/rstb.1996.0091
Cuccu D., Mereu M., Cau A., Pesci P., Cau A. (2013). Reproductive development versus estimated age and size in a wild Mediterranean population of Octopus vulgaris (Cephalopoda: Octopodidae). J. Mar. Biol. Assoc. U. K. 93 (3), 843–849. doi:10.1017/S0025315412000203
Deagle B. E., Tollit D. J., Jarman S. N., Hindell M. A., Trites A. W., Gales N. J. (2005). Molecular scatology as a tool to study diet: Analysis of prey DNA in scats from captive steller sea lions. Mol. Ecol. 14, 1831–1842. doi:10.1111/j.1365-294X.2005.02531.x
Demuth O. E., Benito J., Tschopp E., Lautenschlager S., Mallison H., Heeb N., et al. (2022). Topology-based three-dimensional reconstruction of delicate skeletal fossil remains and the quantification of their taphonomic deformation. Front. Ecol. Evol. 125. doi:10.3389/fevo.2022.828006
Díaz-Santana-Iturrios M., Zepeda-Benitez V., Pacheco-Ovando R., Cornejo C. F., Cerda J. M., Salinas-Zavala C. A., et al. (2022). Northeastern Pacific Octopus beak shape for species-level detection. Malacologia 64 (2), 169–184. doi:10.4002/040.064.0202
Dilly P. N., Nixon M. (1976). The cells that secrete the beaks in octopods and squids (Mollusca, Cephalopoda). Cell. Tissue Res. 167 (2), 229–241. doi:10.1007/BF00224330
Diz A. P., Martínez-Fernández M., Rolán-Alvarez E. (2012). Proteomics in evolutionary ecology: Linking the genotype with the phenotype. Mol. Ecol. 21 (5), 1060–1080. doi:10.1111/j.1365-294X.2011.05426.x
Donlon E. M., Damsteegt E. L., McKinnon J., Higgins F. A., Lamare M. D. (2019). Growth and age of the midget octopus, Octopus huttoni. Aquat. Ecol. 53 (4), 689–706. doi:10.1007/s10452-019-09719-y
Doubleday Z. A., Prowse T. A. A., Arkhipkin A., Pierce G. J., Semmens J., Steer M., et al. (2016). Global proliferation of cephalopods. Curr. Biol. 26, R406–R407. doi:10.1016/j.cub.2016.04.002
Duffy D. C., Jackson S. (1986). Diet studies of seabirds: A review of methods. Colon. Waterbirds 9, 1–17. doi:10.2307/1521138
Fang Z., Chen X., Su H., Thompson K., Chen Y. (2017). Evaluation of stock variation and sexual dimorphism of beak shape of neon flying squid, Ommastrephes bartramii, based on geometric morphometrics. Hydrobiologia 784 (1), 367–380. doi:10.1007/s10750-016-2898-0
Fang Z., Fan J., Chen X., Chen Y. (2018). Beak identification of four dominant Octopus species in the East China Sea based on traditional measurements and geometric morphometrics. Fish. Sci. 84 (6), 975–985. doi:10.1007/s12562-018-1235-0
Fang Z., Han P., Shi S., Chen X. (2021a). Ontogenetic variation of trophic habitat for sympatric benthic octopods in East China Sea derived from isotopic analysis on beaks. Fish. Res. 238, 105902. doi:10.1016/J.FISHRES.2021.105902
Fang Z., Han P., Wang Y., Chen Y., Chen X. (2021b). Interannual variability of body size and beak morphology of the squid Ommastrephes bartramii in the North Pacific Ocean in the context of climate change. Hydrobiologia 848 (6), 1295–1309. doi:10.1007/s10750-021-04528-7
Fang Z., Jin Y., Chen X., Wang Y. (2021c). Differences in the concentrations of trace elements among different hard structures and their potential application in species identification: A case study on loliginidae cryptic species. Mar. Biol. Res. 17 (4), 350–361. doi:10.1080/17451000.2021.1957936
Fang Z., Li J., Thompson K., Hu F., Chen X., Liu B., et al. (2016a). Age, growth, and population structure of the red flying squid (Ommastrephes bartramii) in the North Pacific Ocean, determined from beak microstructure. Fish. Bull. Wash. 114 (1), 34–44. doi:10.7755/FB.114.1.3
Fang Z., Liu B., Chen X., Chen Y. (2019). Ontogenetic difference of beak elemental concentration and its possible application in migration reconstruction for Ommastrephes bartramii in the North Pacific Ocean. Acta Oceanol. Sin. 38 (10), 43–52. doi:10.1007/s13131-019-1431-5
Fang Z., Thompson K., Jin Y., Chen X., Chen Y. (2016b). Preliminary analysis of beak stable isotopes (δ13C and δ15N) stock variation of neon flying squid, Ommastrephes bartramii, in the North Pacific Ocean. Fish. Res. 177, 153–163. doi:10.1016/j.fishres.2016.01.011
Farmer J. R., Hertzberg J., Cardinal D., Fietz S., Hendry K., Jaccard S., et al. (2021). Assessment of C, N, and Si isotopes as tracers of past ocean nutrient and carbon cycling. Glob. Biogeochem. Cycles (35), e2020GB006775. doi:10.1029/2020GB006775
Fernández-Álvarez F. Á., Machordom A., García-Jiménez R., Salinas-Zavala C. A., Villanueva R. (2018). Predatory flying squids are detritivores during their early planktonic life. Sci. Rep. 8 (1), 3440. doi:10.1038/s41598-018-21501-y
Fernández-Álvarez F. Á., Taite M., Vecchione M., Villanueva R., Allcock A. L. (2022). A phylogenomic look into the systematics of oceanic squids (order Oegopsida). Zool. J. Linn. Soc. 194 (4), 1212–1235. doi:10.1093/zoolinnean/zlab069
Fernández-Álvarez F. Á., Farré M., Sánchez-Márquez A., Villanueva R., Escolar O., Navarro J. (2020). Potentially handicapped but otherwise functional: Malformations in prey capture tools show no impacts on octopus life. Ecol. Evol. 10 (23), 12685–12689. doi:10.1002/ece3.6903
Fogel M. L., Tuross N. (2003). Extending the limits of paleodietary studies of humans with compound specific carbon isotope analysis of amino acids. J. Archaeol. Sci. 30 (5), 535–545. doi:10.1016/S0305-4403(02)00199-1
Franco-Santos R., Iglesias J., Domingues P., Vidal E. A. G. (2014). Early beak development in Argonauta nodosa and Octopus vulgaris (Cephalopoda: Incirrata) paralarvae suggests adaptation to different feeding mechanisms. Hydrobiologia 725 (1), 69–83. doi:10.1007/s10750-013-1721-4
Franco-Santos R. M., Perales-Raya C., Almansa E., De Troch M., Garrido D. (2016). Beak microstructure analysis as a tool to identify potential rearing stress for Octopus vulgaris paralarvae. Aquac. Res. 47 (9), 3001–3015. doi:10.1111/are.12753
Franco-Santos R., Vidal E. (2014). Beak development of early squid paralarvae (Cephalopoda: Teuthoidea) may reflect an adaptation to a specialized feeding mode. Hydrobiologia 725 (1), 85–103. doi:10.1007/s10750-013-1715-2
Franco-Santos R. M., Vidal E. A. G. (2020). Tied hands: Synchronism between beak development and feeding-related morphological changes in ommastrephid squid paralarvae. Hydrobiologia 847 (8), 1943–1960. doi:10.1007/s10750-020-04223-z
Fuchs D., Iba Y. (2015). The gladiuses in coleoid cephalopods: Homology, parallelism, or convergence? Swiss J. Palaeontol. 134 (2), 187–197. doi:10.1007/s13358-015-0100-3
Fuchs D., Iba Y., Tischlinger H., Keupp H., Klug C. (2016). The locomotion system of Mesozoic Coleoidea (Cephalopoda) and its phylogenetic significance. Lethaia 49 (4), 433–454. doi:10.1111/let.12155
Fuchs D., Lukeneder A. (2014). Cenozoic coleoids (Cephalopoda) from Austria—A review of Schultz’s Catalogus Fossilium Austriae. Denisia 157, 23–32.
Furness B. L., Laugksch R. C., Duffy D. C. (1984). Cephalopod beaks and studies of seabird diets. Auk 101, 619–620. doi:10.1093/auk/101.3.619
Gales N. J., Cheal A. J. (1992). Estimating diet composition of the Australian sea lion (Neophoca cinerea) from scat analysis: An unreliable technique. Wildl. Res. 19, 447–456. doi:10.1071/wr9920447
García-Fernández P., Prado-Álvarez M., Nande M., Perales-Raya C., Almansa E., Varó I., et al. (2019). Global impact of diet and temperature over aquaculture of Octopus vulgaris paralarvae from a transcriptomic approach. Sci. Rep. 9 (1), 10312–10317. doi:10.1038/s41598-019-46492-2
Garrido D., Navarro J. C., Perales-Raya C., Nande M., Martín M., Iglesias J., et al. (2016). Fatty acid composition and age estimation of wild Octopus vulgaris paralarvae. Aquaculture 464, 564–569. doi:10.1016/j.aquaculture.2016.07.034
Gestal C., Castellanos-Martínez S. (2015). Understanding the cephalopod immune system based on functional and molecular evidence. Fish. Shellfish Immunol. 46 (1), 120–130. doi:10.1016/j.fsi.2015.05.005
Golikov A. V., Artemev G. M., Blicher M. E., Gudmundsson G., Jørgensen L. L., Olafsdottir S. H., et al. (2022). Deep and cold: Are boreal and arctic finned octopods, Stauroteuthis syrtensis and Cirroteuthis muelleri (Cephalopoda, octopoda, Cirrata), ecological analogues? Deep Sea Res. Part I Oceanogr. Res. Pap. 181, 103706. doi:10.1016/j.dsr.2022.103706
Golikov A. V., Ceia F. R., Sabirov R. M., Ablett J. D., Gleadall I. G., Gudmundsson G., et al. (2019a). The first global deep-sea stable isotope assessment reveals the unique trophic ecology of Vampire Squid Vampyroteuthis infernalis (Cephalopoda). Sci. Rep. 9, 19099. doi:10.1038/s41598-019-55719-1
Golikov A. V., Ceia F. R., Sabirov R. M., Batalin G. A., Blicher M. E., Gareev B. I., et al. (2020). Diet and life history reduce interspecific and intraspecific competition among three sympatric Arctic cephalopods. Sci. Rep. 10 (1), 21506. doi:10.1038/s41598-020-78645-z
Golikov A. V., Ceia F. R., Sabirov R. M., Belyaev A. N., Blicher M. E., Arboe N. H., et al. (2019b). Food spectrum and trophic position of an Arctic cephalopod, Rossia palpebrosa (Sepiolida), inferred by stomach contents and stable isotope (δ13C and δ15N) analyses. Mar. Ecol. Prog. Ser. 632, 131–144. doi:10.3354/meps13152
Golikov A. V., Ceia F. R., Sabirov R. M., Zaripova Z. I., Blicher M. E., Zakharov D. V., et al. (2018). Ontogenetic changes in stable isotope (δ13C and δ15N) values in squid Gonatus fabricii (Cephalopoda) reveal its important ecological role in the Arctic. Mar. Ecol. Prog. Ser. 606, 65–78. doi:10.3354/meps12767
Gomes I., Peteiro L. G., Albuquerque R., Nolasco R., Dubert J., Swearer S. E., et al. (2016). Wandering mussels: Using natural tags to identify connectivity patterns among marine protected areas. Mar. Ecol. Prog. Ser. 552, 159–176. doi:10.3354/MEPS11753
Gonçalves C., Costa P. M. (2021). Cephalotoxins: A hotspot for marine bioprospecting? Front. Mar. Sci. 8, 647344. doi:10.3389/fmars.2021.647344
Gong Y., Li Y., Chen X., Yu W. (2020). Trophic niche and diversity of a pelagic squid (Dosidicus gigas): A comparative study using stable isotope, fatty acid, and feeding apparatuses morphology. Front. Mar. Sci. 7, 642. doi:10.3389/fmars.2020.00642
Gong Y., Ruiz-Cooley R. I., Hunsicker M. E., Li Y., Chen X. (2018). Sexual dimorphism in feeding apparatus and niche partitioning in juvenile jumbo squid Dosidicus gigas. Mar. Ecol. Prog. Ser. 607, 99–112. doi:10.3354/meps12768
Gruber N., Keeling C. D., Bacastow R. B., Guenther P. R., Lueker T. J., Wahlen M., et al. (1999). Spatiotemporal patterns of carbon-13 in the global surface oceans and the oceanic Suess effect. Glob. Biogeochem. cycles 13 (2), 307–335. doi:10.1029/1999GB900019
Guerra Á., Rodriguez-Navarro A. B., González A. F., Romanek C. S., Alvarez-Lloret P., Pierce G. J. (2010). Life-history traits of the giant squid Architeuthis dux revealed from stable isotope signatures recorded in beaks. ICES J. Mar. Sci. 67, 1425–1431. doi:10.1093/icesjms/fsq091
Guerreiro M., Richard A., Phillips R. A., Cherel Y., Ceia F. R., Alvito P., et al. (2015). Habitat and trophic ecology of Southern Ocean cephalopods from stable isotope analyses. Mar. Ecol. Prog. Ser. 530, 119–134. doi:10.3354/meps11266
Guímaro H. R., Thompson D. R., Paiva V. H., Ceia F. R., Cunningham D. M., Moors P. J., et al. (2021). Cephalopods habitat and trophic ecology: Historical data using snares penguin as biological sampler. Polar Biol. 44 (1), 73–84. doi:10.1007/s00300-020-02776-2
Gunz P., Mitteroecker P., Bookstein F. (2005). “Semilandmarks in 3D,” in Modern Morphometrics in physical anthropology developments in primatology: Progress and prospects (New York: Kluwer Academic Publishers-Plenum Publishers), 73–98.
Gunz P., Mitteroecker P. (2013). Semilandmarks: A method for quantifying curves and surfaces. Hystrix 24 (1), 103–109. doi:10.4404/hystrix-24.1-6292
Haines A. J., Crampton J. S. (2000). Improvements to the method of Fourier shape analysis as applied in morphometric studies. Palaeontology 43 (4), 765–783. doi:10.1111/1475-4983.00148
Hall K. C. (2002). The life history and fishery of a spawning aggregation of the giant Australian cuttlefish Sepia apama. PhD Thesis (Adelaide, Australia: University of Adelaide), 265.
Harbitz A., Albert O. T. (2015). Pitfalls in stock discrimination by shape analysis of otolith contours. ICES J. Mar. Sci. 72 (7), 2090–2097. doi:10.1093/icesjms/fsv048
Harzhauser M. (1999). “Filling a gap-beaks and hooks of Cenozoic coleoids (Cephalopoda),” in Annalen des Naturhistorischen Museums in Wien. Serie A für Mineralogie und Petrographie, Geologie und Paläontologie, Anthropologie und Prähistorie. Vienna: Natural History Museum of Vienna, 123–135.
Hernández-García V., Hernández-Lopez J. L., Castro J. J. (1998a). The octopus (Octopus vulgaris) in the small-scale trap fishery off the Canary Islands (Central-East Atlantic). Fish. Res. 35 (3), 183–189. doi:10.1016/s0165-7836(98)00080-0
Hernández-García V., Martin A. M., Castro J. J. (2000). Evidence of external digestion of crustaceans in Octopus vulgaris paralarvae. J. Mar. Biol. Assoc. U. K. 80, 559–560. doi:10.1017/S0025315400002320
Hernández-Garcia V., Piatkowski U., Clarke M. R. (1998b). “Development of the darkening of Todarodes sagittatus beaks and its relation to growth and reproduction,” in Cephalopod biodiversity, ecology and evolution. Editors A. I. L. Payne, M. R. Lipinski, and M. R. Clarke, 363–373. (Cape Town, South Africa). M. A. C. Roeleveld's research works. African Journal of Marine Science.
Hernández-López J. L., Castro-Hernández J. J., Hernández-García V. (2001). Age determined from the daily deposition of concentric rings on common octopus (Octopus vulgaris) beaks. Fish. Bull. 99, 679–684.
Hewitt R., Westermann G., Checa A. (1993). Growth rates of ammonites estimated from aptychi. Geobios 26, 203–208. doi:10.1016/S0016-6995(06)80374-8
Hobson K. A., Cherel Y. (2006). Isotopic reconstruction of marine food webs using cephalopod beaks: New insight from captively raised Sepia officinalis. Can. J. Zool. 84, 766–770. doi:10.1139/z06-049
Hotta H. (1973). Identification of squids and cuttlefish in the adjacent waters of Japan. Bull. Seikai Natl. Fish. Res. Inst. 43, 133–147.
Hoving H. J. T., Perez J. A. A., Bolstad K., Braid H., Evans A. B., Fuchs D., et al. (2014). The study of deep-sea cephalopods. Adv. Mar. Biol. 67, 235–359. doi:10.1016/B978-0-12-800287-2.00003-2
Hu G., Boenish R., Gao C., Li B., Chen X., Chen Y., et al. (2019). Spatio-temporal variability in trophic ecology of jumbo squid (Dosidicus gigas) in the southeastern Pacific: Insights from isotopic signatures in beaks. Fish. Res. 212, 56–62. doi:10.1016/j.fishres.2018.12.009
Hu G., Fang Z., Liu B., Yang D., Chen X., Chen Y. (2016). Age, growth and population structure of jumbo flying squid Dosidicus gigas off the Peruvian Exclusive Economic Zone based on beak microstructure. Fish. Sci. 82 (4), 597–604. doi:10.1007/s12562-016-0991-y
Hughes N. C., Jell P. A. (1992). A statistical/computer-graphic technique for assessing variation in tectonically deformed fossils and its application to Cambrian trilobites from Kashmir. Lethaia 25 (3), 317–330. doi:10.1111/j.1502-3931.1992.tb01401.x
Hurley G. V., Odense P. H., O'Dor R. K., Dawe E. G. (1985). Strontium labelling for verifying daily growth increments in the statolith of the short-finned squid (Illex illecebrosus). Can. J. Fish. Aquat. Sci. 42 (2), 380–383. doi:10.1139/f85-050
Hussey N. E., MacNeil M. A., McMeans B. C., Olin J. A., Dudley S. F., Cliff G., et al. (2014b). Rescaling the trophic structure of marine food webs. Ecol. Lett. 17 (2), 239–250. doi:10.1111/ELE.12226
Hussey N. E., MacNeil M. A., McMeans B. C., Olin J. A., Dudley S. F., Cliff G., et al. (2014a). Rescaling the trophic structure of marine food webs. Ecol. Lett. 17 (6), 239–250. doi:10.1111/ele.12226
Imber M. J. (1978). The squid families Cranchiidae and Gonatidae (Cephalopoda: Teuthoidea) in the New Zealand region. N. Z. J. Zool. 5, 445–484. doi:10.1080/03014223.1978.10428331
Ivanovic M. L., Brunetti N. E. (1997). Description of Illex argentinus beaks and rostral length relationships with size and weight of squids. Rev. Invest. Des. Pesq. 11, 135–144. http://hdl.handle.net/1834/1926.
Iverson I. L. K., Pinkas L. (1971). A pictorial guide to beaks of certain eastern Pacific cephalopods. Fish. Bull. 152, 83–105.
Jackson G. D. (1990). The use of tetracycline staining techniques to determine statolith growth ring periodicity in the tropical loliginid squids Loliolus noctiluca and Loligo chinensis. Veliger 33, 389–393.
Jackson G. D. (1995). The use of beaks as tools for biomass estimation in the deepwater squid Moroteuthis ingens (Cephalopoda: Onychoteuthidae) in New Zealand waters. Polar Biol. 15, 9–14. doi:10.1007/bf00236118
Jackson G. D., Bustamante P., Cherel Y., Fulton E. A., Grist E. P. M., Jackson C. H., et al. (2007). Applying new tools to cephalopod trophic dynamics and ecology: Perspectives from the Southern Ocean cephalopod workshop, February 2-3, 2006. Rev. Fish. Biol. Fish. 17, 79–99. doi:10.1007/s11160-007-9055-9
Jackson S., Ryan P. G. (1986). Differential digestion rates of prey by white-chinned petrels (Procellaria aequinoctialis). Auk 103, 617–619.
Jakimska A., Konieczka P., Skóra K., Namieśnik J. (2011). Bioaccumulation of metals in tissues of marine animals, Part I: The role and impact of heavy metals on organisms. Pol. J. Environ. Stud. 20 (5), 1117–1125.
Jarre A., Clarke M., Pauly D. (1991). Re-Examination of growth estimates in oceanic squids: The case of Kondakovia longimana (Onychoteuthidae). ICES J. Mar. Sci. 48, 195–200. doi:10.1093/icesjms/48.2.195
Jin Y., Lin F., Chen X., Liu B., Li J. (2019). Microstructure comparison of hard tissues (statoliths, beaks, and eye lenses) of Uroteuthis chinensis in the South China Sea. Bull. Mar. Sci. 95 (1), 13–26. doi:10.5343/bms.2017.1175
Jin Y., Liu B., Li J., Chen X. (2017). Identification of three common Loliginidae squid species in the South China Sea by analyzing hard tissues with geometric outline method. J. Ocean. Univ. China 16 (5), 840–846. doi:10.1007/s11802-017-3218-7
Keeling C. D. (1979). The suess effect: 13Carbon-14Carbon interrelations. Environ. Int. 2 (4-6), 229–300. doi:10.1016/0160-4120(79)90005-9
Klug C., Frey L., Korn D., Jattiot R., Rücklin M. (2016). The oldest Gondwanan cephalopod Mandibles (Hangenberg Black Shale, Late Devonian) and the mid-Palaeozoic rise of jaws. Palaeontology 59 (5), 611–629. doi:10.1111/pala.12248
Klug C., Pohle A., Roth R., Hoffmann R., Wani R., Tajika A. (2021a). Preservation of nautilid soft parts inside and outside the conch interpreted as central nervous system, eyes, and renal concrements from the Lebanese Cenomanian. Swiss J. Palaeontol. 140 (1), 15–11. doi:10.1186/s13358-021-00229-9
Klug C., Schweigert G., Fuchs D., De Baets K. (2021b). Distraction sinking and fossilized coleoid predatory behaviour from the German Early Jurassic. Swiss J. Palaeontol. 140 (1), 7–12. doi:10.1186/s13358-021-00218-y
Košťák M., Schlögl J., Fuchs D., Holcová K., Hudáčková N., Culka A., et al. (2021). Fossil evidence for vampire squid inhabiting oxygen-depleted ocean zones since at least the Oligocene. Commun. Biol. 4 (1), 216. doi:10.1038/s42003-021-01714-0
Kousteni V., Karachle P. K., Megalofonou P., Lefkaditou E. (2018). Cephalopod prey of two demersal sharks caught in the Aegean Sea (eastern Mediterranean). J. Mar. Biolog. Assoc. U. K. 98, 81–88. doi:10.1017/S002531541700159X
Kubodera T., Furuhashi M. (1987). Manual for the identification of cephalopods and myctophids in the stomach contents. Japan: The Fisheries Agency of Japan.
Kubodera T., Oizumi H., Imaizumi T., Jorgensen E. M. (2005). Manual for the identification of cephalopod beaks in the northwest pacific. Tokyo: National Museum of Nature and Science. https://www.kahaku.go.jp/research/db/zoology/Beak-E/intro.htm.
Lansdell M., Young J. (2007). Pelagic cephalopods from eastern Australia: species composition, horizontal and vertical distribution determined from the diets of pelagic fishes. Rev. Fish. Biol. Fish. 17, 125–138. doi:10.1007/s11160-006-9024-8
Le Croizier G. l., Lorrain A., Sonke J. E., Hoyos-Padilla E. M., Galván-Magaña F., Santana-Morales O., et al. (2020). The twilight zone as a major foraging habitat and mercury source for the great white shark. Environ. Sci. Technol. 54 (24), 15872–15882. doi:10.1021/acs.est.0c05621
Le Croizier G., Lorrain A., Sonke J. E., Jaquemet S., Schaal G., Renedo M., et al. (2020). Mercury isotopes as tracers of ecology and metabolism in two sympatric shark species. Environ. Pollut. 265, 114931. doi:10.1016/j.envpol.2020.114931
Lin J. Y., Liu B., Wang L. (2019). Age and growth of swordtip squids based on microstructure of beaks. J. East China Univ. Sci. Technol. 45 (5), 775–782.
Linder M. B. (2015). Biomaterials: Recipe for squid beak. Nat. Chem. Biol. 11 (7), 455–456. doi:10.1038/nchembio.1842
Lipinski M. R., Durholtz M. D., Underhill L. G. (1998). Field validation of age readings from the statoliths of chokka squid (Loligo vulgaris reynaudii d'Orbigny, 1845) and an assessment of associated errors. ICES J. Mar. Sci. 55 (2), 240–257. doi:10.1006/jmsc.1997.0274
Lischka A., Braid H., Cherel Y., Bolstad K., Lacoue-Labarthe T., Bustamante P. (2020). Influence of sexual dimorphism on stable isotopes and trace element concentrations in the greater hooked squid Moroteuthopsis ingens from New Zealand waters. Mar. Environ. Res. 159, 104976. doi:10.1016/j.marenvres.2020.104976
Lishchenko F., Jones J. B. (2021). Application of shape analyses to recording structures of marine organisms for stock discrimination and taxonomic purposes. Front. Mar. Sci. 8, 1301. doi:10.3389/fmars.2021.667183
Liu B. L., Chen X. J., Chen Y., Hu G. Y., Yu W., Wang J. T., et al. (2017). Periodic increments in the jumbo squid (Dosidicus gigas) beak: A potential tool for determining age and investigating regional difference in growth rates. Hydrobiologia 790 (1), 83–92. doi:10.1007/s10750-016-3020-3
Liu B. L., Chen X. J., Li J. H., Fang Z. (2014). Review on age and growth of cephalopods using their beaks. Chin. J. Shanghai Ocean. Univ. 23 (6), 930–936.
Liu B. L., Chen X., Qian W., Jin Y., Li J. (2019a). δ13C and δ15N in Humboldt squid beaks: Understanding potential geographic population connectivity and movement. Acta Oceanol. Sin. 38 (10), 53–59. doi:10.1007/s13131-019-1487-2
Liu B. L., Fang Z., Chen X., Chen Y. (2015). Spatial variations in beak structure to identify potentially geographic populations of Dosidicus gigas in the Eastern Pacific Ocean. Fish. Res. 164 (0), 185–192. doi:10.1016/j.fishres.2014.12.001
Liu B. L., Jin Y., Chen X., Li J., Qian W., Liu N., et al. (2019b). High individual variability in beak stable isotopes of jumbo squid off Peruvian exclusive economic zone (EEZ) waters in the analysis of migratory and foraging ecology. J. Ocean. Univ. China 18 (1), 232–238. doi:10.1007/s11802-019-3749-1
Liu B. L., Lin J. Y., Chen X. J., Jin Y., Wang J. T. (2018). Inter-and intra-regional patterns of stable isotopes in Dosidicus gigas beak: Biological, geographical and environmental effects. Mar. Freshw. Res. 69 (3), 464–472. doi:10.1071/MF17144
Lombarte A., Chic Ò., Parisi-Baradad V., Olivella R., Piera J., García-Ladona E. (2006). A web-based environment for shape analysis of fish otoliths. The AFORO database. Sci. Mar. 70, 147–152. doi:10.3989/scimar.2006.70n1147
López-Córdova D. A., Avaria-Llautureo J., Ulloa P. M., Braid H. E., Revell L. J., Fuchs D., et al. (2022). Mesozoic origin of coleoid cephalopods and their abrupt shifts of diversification patterns. Mol. Phylogenet. Evol. 166, 107331. doi:10.1016/j.ympev.2021.107331
Lu C. C., Ickeringill R. (2002). Cephalopod beak identification and biomass estimation techniques: Tools for dietary studies of southern Australian finfishes. Mus. Vic. Sci. Rep. 6, 1–65. doi:10.24199/j.mvsr.2002.06
Luna A., Sánchez P., Chicote C., Gazo M. (2021). Cephalopods in the diet of Risso's dolphin (Grampus griseus) from the mediterranean sea: A review. Mar. Mamm. Sci. 38 (2), 725–741. doi:10.1111/mms.12869
Machalski M. (2021). Correlation of shell and aptychus growth provides insights into the palaeobiology of a scaphitid ammonite. Palaeontology 64 (2), 225–247. doi:10.1111/pala.12519
Magozzi S., Yool A., Vander Zanden H., Wunder M., Trueman C. (2017). Using ocean models to predict spatial and temporal variation in marine carbon isotopes. Ecosphere 8 (5), e01763. doi:10.1002/ecs2.1763
Mangold K., Fioroni P. (1966). Morphologie et biométrie des mandibules de quelques céphalopodes méditerranéens. Vie Milieu 17, 1139–1196.
Matias R. S., Gregory S., Ceia F. R., Baeta A., Seco J., Rocha M. S., et al. (2019). Show your beaks and we tell you what you eat: Different ecology in sympatric Antarctic benthic octopods under a climate change context. Mar. Environ. Res. 150, 104757. doi:10.1016/j.marenvres.2019.104757
Matias R. S., Seco J., Gregory S., Belchier M., Pereira M. E., Bustamante P., et al. (2020). Antarctic octopod beaks as proxy for mercury concentrations in soft tissues. Mar. Pollut. Bull. 158, 111447. doi:10.1016/j.marpolbul.2020.111447
Mazzeo M. F., Siciliano R. A. (2016). Proteomics for the authentication of fish species. J. Proteomics 147, 119–124. doi:10.1016/j.jprot.2016.03.007
Meisel D. V., Byrne R. A., Kuba M., Griebel U., Mather J. A. (2003). “Circadian rhythms in Octopus vulgaris,” in Coleoid cephalopods through time. Editors K. Warnke, H. Keupp, and S. v. Boletzky, 171–177.
Ménard F., Potier M., Jaquemet S., Romanov E., Sabatié R., Cherel Y. (2013). Pelagic cephalopods in the Western Indian Ocean: New information from diets of top predators. Deep Sea Res. Part II Top. Stud. Oceanogr. 95, 83–92. doi:10.1016/j.dsr2.2012.08.022
Mereu M., Stacca D., Cannas R., Cuccu D. (2011). On the growth rings on Histioteuthis bonnellii (Férussac, 1835) upper beaks. Biol. Mar. Mediterr. 18 (1), 124–127.
Miserez A., Li Y., Waite J. H., Zok F. (2007). Jumbo squid beaks: Inspiration for design of robust organic composites. Acta Biomater. 3, 139–149. doi:10.1016/j.actbio.2006.09.004
Miserez A., Rubin D., Waite J. H. (2010). Cross-linking chemistry of squid beak. J. Biol. Chem. 285 (49), 38115–38124. doi:10.1074/jbc.M110.161174
Miserez A., Schneberk T., Sun C., Zok F. W., Waite J. H. (2008). The transition from stiff to compliant materials in squid beaks. Science 319 (5871), 1816–1819. doi:10.1126/science.1154117
Modi A., Vergata C., Zilli C., Vischioni C., Vai S., Tagliazucchi G. M., et al. (2021). Successful extraction of insect DNA from recent copal inclusions: Limits and perspectives. Sci. Rep. 11 (1), 6851–6858. doi:10.1038/s41598-021-86058-9
MolluscaBase (2022). Histioteuthis bonnellii (Férussac, 1834). Accessed through: World Register of Marine Species at: https://www.marinespecies.org/aphia.php?p=taxdetails&id=140111 on 2022.07.30.
Morin-Crini N., Lichtfouse E., Torri G., Crini G. (2019). Applications of chitosan in food, pharmaceuticals, medicine, cosmetics, agriculture, textiles, pulp and paper, biotechnology, and environmental chemistry. Environ. Chem. Lett. 17 (4), 1667–1692. doi:10.1007/s10311-019-00904-x
Naito Y. I., Chikaraishi Y., Ohkouchi N., Yoneda M. (2013). Evaluation of carnivory in inland Jomon hunter–gatherers based on nitrogen isotopic compositions of individual amino acids in bone collagen. J. Archaeol. Sci. 40 (7), 2913–2923. doi:10.1016/J.JAS.2013.03.012
Nande M., Iglesias J., Domingues P., Pérez M. (2017). Effect of temperature on energetic demands during the last stages of embryonic development and early life of Octopus vulgaris (Cuvier, 1797) paralarvae. Aquac. Res. 48 (4), 1951–1961. doi:10.1111/are.13032
Navarro J., Coll M., Somes C. J., Olson R. J. (2013). Trophic niche of squids: Insights from isotopic data in marine systems worldwide. Deep Sea Res. Part II Top. Stud. Oceanogr. 95, 93–102. doi:10.1016/j.dsr2.2013.01.031
Neige P., Dommergues J.-L. (2002). Disparity of beaks and statoliths of some coleoids: A morphometric approach to depict shape differentiation. Abh. Geol. B-A 57, 393–399.
Newsome S. D., Rio C. M. D., Bearhop S., Phillips D. L. (2007). A niche for isotopic ecology. Front. Ecol. Environ. 5, 429–436. doi:10.1890/060150.1
Northern T. J., Smith A. M., McKinnon J. F., Bolstad K. S. (2019). Trace elements in beaks of greater hooked squid Onykia ingens: Opportunities for environmental tracing. Molluscan Res. 39 (1), 29–34. doi:10.1080/13235818.2018.1495604
Nunn B. L., Timperman A. T. (2007). Marine proteomics. Mar. Ecol. Prog. Ser. 332, 281–289. doi:10.3354/meps332281
O’Connell T. (2017). ‘Trophic’ and ‘source’ amino acids in trophic estimation: A likely metabolic explanation. Oecologia 184 (2), 317–326. doi:10.1007/s00442-017-3881-9
Ogden R. S., Allcock A., Watts P., Thorpe J. (1998). The role of beak shape in octopodid taxonomy. South Afr. J. Mar. Sci. 20, 29–36. doi:10.2989/025776198784126476
Olmos-Pérez L., Roura Á., Pierce G. J., Boyer S., González Á. F. (2017). Diet composition and variability of wild Octopus vulgaris and Alloteuthis media (Cephalopoda) paralarvae: A metagenomic approach. Front. Physiol. 8 (321), 321–21. doi:10.3389/fphys.2017.00321
Oosthuizen A. (2003). A development and management framework for a new Octopus vulgaris Fishery in South Africa. PhD thesis (Grahamstown, South Africa: Rhodes University), 183.
Pabic C. L., Marie A., Marie B., Percot A., Bonnaud-Ponticelli L., Lopez P. J., et al. (2017). First proteomic analyses of the dorsal and ventral parts of the Sepia officinalis cuttlebone. J. Proteomics 150, 63–73. doi:10.1016/j.jprot.2016.08.015
Pacheco-Ovando R., Granados-Amores J., González-Salinas B., Ruiz-Villegas J. M., Díaz-Santana-Iturrios M. (2021). Beak shape analysis and its potential to recognize three loliginid squid species found in the northeastern Pacific. Mar. Biodivers. 51 (5), 82–12. doi:10.1007/s12526-021-01220-w
Packard A. (1972). Cephalopods and fish: The limits of convergence. Biol. Rev. 47, 241–307. doi:10.1111/j.1469-185x.1972.tb00975.x
Pedà C., Battaglia P., Scuderi A., Voliani A., Mancusi C., Andaloro F., et al. (2015). Cephalopod prey in the stomach contents of odontocete cetaceans stranded in the western Mediterranean Sea. Mar. Biol. Res. 11, 593–602. doi:10.1080/17451000.2014.966724
Pedà C., Battaglia P., Romeo T., Stipa M. G., Longo F., Malara D., et al. (2022). Photographic atlas of cephalopod beaks from the Mediterranean Sea. Milan, Italy, 120. Eta Beta.
Perales-Raya C., Almansa E., Bartolomé A., Felipe B. C., Iglesias J., Sánchez F. J., et al. (2014a). Age validation in Octopus vulgaris beaks across the full ontogenetic range: Beaks as recorders of life events in Octopuses. J. Shellfish Res. 33, 481–493. doi:10.2983/035.033.0217
Perales-Raya C., Bartolomé A., García-Santamaría M. T., Pascual-Alayón P., Almansa E. (2010). Age estimation obtained from analysis of octopus (Octopus vulgaris Cuvier, 1797) beaks: Improvements and comparisons. Fish. Res. 106, 171–176. doi:10.1016/j.fishres.2010.05.003
Perales-Raya C., Bartolomé A., Hernández-Rodríguez E., Carrillo M., Martín V., Fraile-Nuez E. (2020). How old are giant squids? First approach to aging Architeuthis beaks. Bull. Mar. Sci. 96 (2), 357–374. doi:10.5343/bms.2019.0041
Perales-Raya C., Hernández-González C. (1998). “Growth lines within the beak microstructure of the octopus Octopus vulgaris Cuvier, 1797,” in Cephalopod biodiversity, ecology and evolution. Editors A. I. L. Payne, M. R. Lipinski, and M. R. Clarke, 135–142. (Cape Town, South Africa). M. A. C. Roeleveld's research works. African Journal of Marine Science.
Perales-Raya C., Jurado-Ruzafa A., Bartolomé A., Duque V., Carrasco M. N., Fraile-Nuez E. (2014b). Age of spent Octopus vulgaris and stress mark analysis using beaks of wild individuals. Hydrobiologia 725 (1), 105–114. doi:10.1007/s10750-013-1602-x
Perales-Raya C., Nande M., Roura A., Bartolomé A., Gestal C., Otero J. J., et al. (2018). Comparative study of age estimation in wild and cultured Octopus vulgaris paralarvae: Effect of temperature and diet. Mar. Ecol. Prog. Ser. 598, 247–259. doi:10.3354/meps12218
Pereira J. M., Paiva V. H., Xavier J. C. (2017). Using seabirds to map the distribution of elusive pelagic cephalopod species. Mar. Ecol. Prog. Ser. 567, 257–262. doi:10.3354/meps12020
Peristeraki P., Tserpes G., Lefkaditou E. (2005). What cephalopod remains from Xiphias gladius stomachs can imply about predator-prey interactions in the Mediterranean Sea? J. Fish Biol. 67, 549–554. doi:10.1111/j.0022-1112.2005.00742.x
Pérez-Gándaras G. (1983). Estudio de los cefalópodos ibéricos: Sistemática y binomia mediante el estudio morfométrico comparado de sus mandíbulas. Ph.D. Thesis (Madrid, Spain: Universidad Complutense de Madrid).
Peterson B. J., Fry B. (1987). Stable isotopes in ecosystem studies. Annu. Rev. Ecol. Syst. 18, 293–320. doi:10.1146/annurev.es.18.110187.001453
Phillips D. L., Inger R., Bearhop S., Jackson A. L., Moore J. W., Parnell A. C., et al. (2014). Best practices for use of stable isotope mixing models in food-web studies. Can. J. Zool. 92 (10), 823–835. doi:10.1139/cjz-2014-0127
Pierce G. J., Stowasser G., Hastie L., Bustamante P. (2008). Geographic, seasonal and ontogenetic variation in cadmium and mercury concentrations in squid (Cephalopoda: Teuthoidea) from UK waters. Ecotoxicol. Environ. Saf. 70 (3), 422–432. doi:10.1016/j.ecoenv.2007.07.007
Pinkas L., Oliphant M. S., Iversen I. L. K. (1971). Food habits of albacore, bluefin tuna, and bonito in California waters. Calif. Dep. Fish. Game Fish. Bull. 152 (105), 1–105.
Pinkerton M., Bury S., Brown J., Forman J., Kilimnik A. (2014). “Stable isotope analysis of tissue samples to investigate trophic linkages of Antarctic toothfish (Dissostichus mawsoni) in the Ross and Amundsen Sea regions,” in Commission for the conservation of antarctic marine living resources WG-EMM-14/50 (Hobart, AUS: CCAMLR), 30.
Post D. M., Layman C. A., Arrington D. A., Takimoto G., Quattrochi J., Montana C. G. (2007). Getting to the fat of the matter: Models, methods and assumptions for dealing with lipids in stable isotope analyses. Oecologia 152 (1), 179–189. doi:10.1007/s00442-006-0630-x
Queirós J. P., Bustamante P., Cherel Y., Coelho J. P., Seco J., Roberts J., et al. (2020a). Cephalopod beak sections used to trace mercury levels throughout the life of cephalopods: The giant warty squid Moroteuthopsis longimana as a case study. Mar. Environ. Res. 161, 105049. doi:10.1016/j.marenvres.2020.105049
Queirós J. P., Cherel Y., Ceia F. R., Hilário A., Roberts J., Xavier J. C. (2018). Ontogenic changes in habitat and trophic ecology in the Antarctic squid Kondakovia longimana derived from isotopic analysis on beaks. Polar Biol. 41 (12), 2409–2421. doi:10.1007/s00300-018-2376-4
Queirós J. P., Fenwick M., Stevens D. W., Cherel Y., Ramos J. A., Xavier J. C. (2020b). Ontogenetic changes in habitat and trophic ecology of the giant Antarctic octopus Megaleledone setebos inferred from stable isotope analyses in beaks. Mar. Biol. 167 (5), 56. doi:10.1007/s00227-020-3666-2
Queirós J. P., Hilário A., Thompson D., Ceia F. R., Elliott G., Walker K., et al. (2021a). From warm to cold waters: New insights into the habitat and trophic ecology of Southern Ocean squids throughout their life cycle. Mar. Ecol. Prog. Ser. 659, 113–126. doi:10.3354/meps13551
Queirós J. P., Phillips R. A., Baeta A., Abreu J., Xavier J. C. (2019). Habitat, trophic levels and migration patterns of the short-finned squid Illex argentinus from stable isotope analysis of beak regions. Polar Biol. 42 (12), 2299–2304. doi:10.1007/s00300-019-02598-x
Queirós J. P., Ramos J. A., Cherel Y., Franzitta M., Duarte B., Rosa R., et al. (2021b). Cephalopod fauna of the Pacific Southern Ocean using Antarctic toothfish (Dissostichus mawsoni) as biological samplers and fisheries bycatch specimens. Deep Sea Res. Part I Oceanogr. Res. Pap. 174, 103571. doi:10.1016/j.dsr.2021.103571
Renedo M., Amouroux D., Pedrero Z., Bustamante P., Cherel Y. (2018). Identification of sources and bioaccumulation pathways of MeHg in subantarctic penguins: A stable isotopic investigation. Sci. Rep. 8 (1), 8865. doi:10.1038/s41598-018-27079-9
Rodhouse P. G. K., Pierce G. J., Nichols O. C., Sauer W. H. H., Arkhipkin A. I., Laptikhovsky V. V., et al. (2014). Environmental effects on cephalopod population dynamics: Implications for management of fisheries. Adv. Mar. Biol. 67, 99–233. doi:10.1016/B978-0-12-800287-2.00002-0
Rodríguez-Domínguez A., Rosas C., Méndez-Loeza I., Markaida U. (2013). Validation of growth increments in stylets, beaks and lenses as ageing tools in Octopus maya. J. Exp. Mar. Biol. Ecol. 449, 194–199. doi:10.1016/j.jembe.2013.10.001
Roeleveld M. A. (2000). Giant squid beaks: Implications for systematics. J. Mar. Biol. Assoc. U. K. 80 (1), 185–187. doi:10.1017/S0025315499001769
Romanov E. V., Nikolic N., Dhurmeea Z., Bodin N., Puech A., Norman S., et al. (2020). Trophic ecology of albacore tuna Thunnus alalunga in the Western tropical Indian Ocean and adjacent waters. Mar. Freshw. Res. 71 (11), 1517–1542. doi:10.1071/MF19332
Romeo T., Battaglia P., Pedà C., Perzia P., Consoli P., Esposito V., et al. (2012). Pelagic cephalopods of the central Mediterranean Sea determined by the analysis of the stomach content of large fish predators. Helgol. Mar. Res. 66, 295–306. doi:10.1007/s10152-011-0270-3
Roscian M., Herrel A., Cornette R., Delapré A., Cherel Y., Rouget I. (2021). Underwater photogrammetry for close-range 3D imaging of dry-sensitive objects: The case study of cephalopod beaks. Ecol. Evol. 11 (12), 7730–7742. doi:10.1002/ece3.7607
Roscian M., Herrel A., Zaharias P., Cornette R., Fernandez V., Kruta I., et al. (2022). Every hooked beak is maintained by a prey: Ecological signal in cephalopod beak shape. Funct. Ecol. 36, 2015–2028. doi:10.1111/1365-2435.14098
Roumbedakis K., Drábková M., Tyml T., Di Cristo C. (2018). A perspective around cephalopods and their parasites, and suggestions on how to increase knowledge in the field. Front. Physiol. 9, 1573. doi:10.3389/fphys.2018.01573
Ruiz-Cooley R. I., Garcia K. Y., Hetherington E. D. (2011). Effects of lipid removal and preservatives on carbon and nitrogen stable isotope ratios of squid tissues: Implications for ecological studies. J. Exp. Mar. Biol. Ecol. 407 (1), 101–107. doi:10.1016/j.jembe.2011.07.002
Ruiz-Cooley R., Markaida U., Gendron D., Aguíñiga S. (2006). Stable isotopes in jumbo squid (Dosidicus gigas) beaks to estimate its trophic position: Comparison between stomach contents and stable isotopes. J. Mar. Biol. Assoc. U. K. 86 (2), 437–445. doi:10.1017/S0025315406013324
Sakai M., Brunetti N., Bower J., Elena B., Ichii T., Ivanovic M., et al. (2007). Daily growth increments in upper beak of five ommastrephid paralarvae, Illex argentinus, Ommastrephes bartramii, Dosidicus gigas, Sthenoteuthis oualaniensis, Todarodes pacificus. Behav. Brain Res. 9, 1–11. doi:10.1016/j.bbr.2007.03.016
Salamon M. A., Niedźwiedzki R., Gorzelak P., Lach R., Surmik D. (2012). Bromalites from the middle triassic of Poland and the rise of the mesozoic marine revolution. Palaeogeogr. Palaeoclimatol. Palaeoecol. 321-322, 142–150. doi:10.1016/j.palaeo.2012.01.029
Sanchez G., Fernández-Álvarez F. Á., Taite M., Sugimoto C., Jolly J., Simakov O., et al. (2021). Phylogenomics illuminates the evolution of bobtail and bottletail squid (order Sepiolida). Commun. Biol. 4 (1), 819–9. doi:10.1038/s42003-021-02348-y
Santos M. B., Clarke M. R., Pierce G. J. (2001). Assessing the importance of cephalopods in the diets of marine mammals and other top predators: Problems and solutions. Fish. Res. 52 (1-2), 121–139. doi:10.1016/s0165-7836(01)00236-3
Sauer W. H., Gleadall I. G., Downey-Breedt N., Doubleday Z., Gillespie G., Haimovici M., et al. (2021). World octopus fisheries. Rev. Fish. Sci. Aquac. 29 (3), 279–429. doi:10.1080/23308249.2019.1680603
Schlager S., Profico A., Di Vincenzo F., Manzi G. (2018). Retrodeformation of fossil specimens based on 3D bilateral semi-landmarks: Implementation in the R package “Morpho”. PloS one 13 (3), e0194073. doi:10.1371/journal.pone.0194073
Schwarz R. (2019). Assessing the lifespans of coldwater octopods (Cephalopoda: Octopodiformes). PhD thesis (Kiel, Germany: Kiel University), 147.
Schwarz R., Hoving H.-J., Noever C., Piatkowski U. (2019). Life histories of antarctic incirrate octopods (Cephalopoda: Octopoda). PloS one 14 (7), e0219694. doi:10.1371/journal.pone.0219694
Schwarz R., Piatkowski U., Robison B. H., Laptikhovsky V. V., Hoving H.-J. (2020). Life history traits of the deep-sea pelagic cephalopods Japetella diaphana and Vampyroteuthis infernalis. Deep Sea Res. Part I Oceanogr. Res. Pap. 164, 103365. doi:10.1016/j.dsr.2020.103365
Seco J., Roberts J., Ceia F., Baeta A., Ramos J., Paiva V., et al. (2016). Distribution, habitat and trophic ecology of antarctic squid Kondakovia longimana and Moroteuthis knipovitchi: Inferences from predators and stable isotopes. Polar Biol. 39, 167–175. doi:10.1007/s00300-015-1675-2
Seco J., Xavier J. C., Brierley A. S., Bustamante P., Coelho J. P., Gregory S., et al. (2020). Mercury levels in Southern Ocean squid: Variability over the last decade. Chemosphere 239, 124785. doi:10.1016/j.chemosphere.2019.124785
Seixas S., Bustamante P., Pierce G. (2005). Accumulation of mercury in the tissues of the common octopus Octopus vulgaris (L.) in two localities on the Portuguese coast. Sci. Total Environ. 340 (1-3), 113–122. doi:10.1016/j.scitotenv.2004.08.012
Sen I. S., Peucker-Ehrenbrink B. (2012). Anthropogenic disturbance of element cycles at the Earth’s surface. Environ. Sci. Technol. 46 (16), 8601–8609. doi:10.1021/es301261x
Smale M. J., Clarke M. R., Klages N. T. W., Roeleveld M. A. C. (1993). Octopod beak identification—resolution at a regional level (Cephalopoda, Octopoda: Southern Africa). S. Afr. J. Mar. Sci. 13 (1), 269–293. doi:10.2989/025776193784287338
Somes C. J., Schmittner A., Galbraith E. D., Lehmann M. F., Altabet M. A., Montoya J. P., et al. (2010). Simulating the global distribution of nitrogen isotopes in the ocean. Glob. Biogeochem. Cycles 24 (4). doi:10.1029/2009GB003767
Sonnerup R. E., Quay P. D., McNichol A. P., Bullister J. L., Westby T. A., Anderson H. L. (1999). Reconstructing the oceanic 13C Suess effect. Glob. Biogeochem. Cycles 13 (4), 857–872. doi:10.1029/1999GB900027
St John Glew K., Espinasse B., Hunt B. P., Pakhomov E. A., Bury S. J., Pinkerton M., et al. (2021). Isoscape models of the Southern Ocean: Predicting spatial and temporal variability in carbon and nitrogen isotope compositions of particulate organic matter. Glob. Biogeochem. Cycles 35 (9), e2020GB006901. doi:10.1029/2020GB006901
Staudinger M. D., Dimkovikj V. H., France C. A., Jorgensen E., Judkins H., Lindgren A., et al. (2019). Trophic ecology of the deep-sea cephalopod assemblage near bear seamount in the northwest atlantic ocean. Mar. Ecol. Prog. Ser. 629, 67–86. doi:10.3354/meps13121
Staudinger M., Juanes F., Salmon B., Teffer A. (2013). The distribution, diversity, and importance of cephalopods in top predator diets from offshore habitats of the Northwest Atlantic Ocean. Deep Sea Res. Part II Top. Stud. Oceanogr. 95, 182–192. doi:10.1016/j.dsr2.2012.06.004
Strugnell J. M., Cherel Y., Cooke I. R., Gleadall I. G., Hochberg F. G., Ibáñez C. M., et al. (2011). The Southern Ocean: Source and sink? Deep Sea Res. Part II Top. Stud. Oceanogr. 58 (1), 196–204. doi:10.1016/j.dsr2.2010.05.015
Sun Y., Lim Z. W., Guo Q., Yu J., Miserez A. (2020). Liquid–liquid phase separation of proteins and peptides derived from biological materials: Discovery, protein engineering, and emerging applications. MRS Bull. 45 (12), 1039–1047. doi:10.1557/mrs.2020.301
Szynkowska M. I., Pawlaczyk A., Maćkiewicz E. (2018). Bioaccumulation and biomagnification of trace elements in the environment. Recent Adv. Trace Elem. 251, 251–276. doi:10.1002/9781119133780.CH13
Tan H. Y., Goh Z. Y., Loh K.-H., Then A. Y.-H., Omar H., Chang S.-W. (2021). Cephalopod species identification using integrated analysis of machine learning and deep learning approaches. PeerJ 9, e11825. doi:10.7717/peerj.11825
Tan Y., Hoon S., Guerette P. A., Wei W., Ghadban A., Hao C., et al. (2015). Infiltration of chitin by protein coacervates defines the squid beak mechanical gradient. Nat. Chem. Biol. 11 (7), 488–495. doi:10.1038/nchembio.1833
Tanabe K., Hikida Y., Iba Y. (2006). Two coleoid jaws from the upper cretaceous of Hokkaido, Japan. J. Paleontol. 80 (1), 138–145. doi:10.1666/0022-3360(2006)080[0138:TCJFTU]2.0.CO;2
Tanabe K., Hikida Y. (2010). Jaws of a new species of Nanaimoteuthis (Coleoidea: Vampyromorphida) from the Tuironian of Hokkaido, Japan. Paleontological Res. 14 (2), 145–150. doi:10.2517/1342-8144-14.2.145
Tanabe K., Kruta I., Landman N. H. (2015a). “Ammonoid buccal mass and jaw apparatus,” in Ammonoid paleobiology: From anatomy to ecology. Editors C. Klug, D. Korn, K. De Baets, I. Kruta, and R. H. Mapes (Dordrecht: Springer), 429–484.
Tanabe K., Misaki A., Ubukata T. (2015b). Late Cretaceous record of large soft-bodied coleoids based on lower jaw remains from Hokkaido, Japan. Acta Palaeontol. Pol. 60 (1), 27–38. doi:10.4202/app.00057.2013
Tanabe K., Trask P., Ross R., Hikida Y. (2008). Late Cretaceous octobrachiate coleoid lower jaws from the North Pacific regions. J. Paleontol. 82 (2), 398–408. doi:10.1666/07-029.1
Tanner A. R., Fuchs D., Winkelmann I. E., Gilbert M. T. P., Pankey M. S., Ribeiro Â. M., et al. (2017). Molecular clocks indicate turnover and diversification of modern coleoid cephalopods during the Mesozoic Marine Revolution. Proc. Biol. Sci. 284 (1850), 20162818. doi:10.1098/rspb.2016.2818
Tomanek L. (2014). Proteomics to study adaptations in marine organisms to environmental stress. J. Proteomics 105, 92–106. doi:10.1016/j.jprot.2014.04.009
Trasviña-Carrillo L., Hernández-Herrera A., Torres-Rojas Y., Galván-Magaña F., Sánchez-González A., Aguíñiga-García S., et al. (2018). Spatial and trophic preferences of jumbo squid Dosidicus gigas (D'orbigny, 1835) in the central gulf of California: Ecological inferences using stable isotopes. Rapid Commun. Mass Spectrom. 32 (15), 1225–1236. doi:10.1002/rcm.8147
Uchikawa K., Sakai M., Wakabayashi T., Ichii T. (2009). The relationship between paralarval feeding and morphological changes in the proboscis and beaks of the neon flying squid Ommastrephes bartramii. Fish. Sci. 75 (2), 317–323. doi:10.1007/s12562-008-0036-2
Uyeno T. A., Kier W. M. (2007). Electromyography of the buccal musculature of octopus (Octopus bimaculoides): A test of the function of the muscle articulation in support and movement. J. Exp. Biol. 210 (1), 118–128. doi:10.1242/jeb.02600
Uyeno T. A., Kier W. M. (2005). Functional morphology of the cephalopod buccal mass: A novel joint type. J. Morphol. 264 (2), 211–222. doi:10.1002/jmor.10330
Vecchione M., Allcock A. L., Piatkowski U., Strugnell J. M. (2009). Benthoctopus rigbyae, n. sp., a new species of cephalopod (Octopoda: Incirrata) from near the Antarctic Peninsula. Malacologia 51, 13–28. doi:10.4002/040.051.0102
Veldhoen N., Ikonomou M. G., Helbing C. C. (2012). Molecular profiling of marine fauna: Integration of omics with environmental assessment of the world's oceans. Ecotoxicol. Environ. Saf. 76, 23–38. doi:10.1016/j.ecoenv.2011.10.005
Verwega M.-T., Somes C. J., Schartau M., Tuerena R. E., Lorrain A., Oschlies A., et al. (2021). Description of a global marine particulate organic carbon-13 isotope data set. Earth Syst. Sci. Data 13 (10), 4861–4880. doi:10.5194/essd-2021-159
Vidal E. A. G., Salvador B. (2019). The tentacular strike behavior in squid: Functional interdependency of morphology and predatory behaviors during ontogeny. Front. Physiol. 10, 1558. doi:10.3389/fphys.2019.01558
Volpedo A. V., Vaz-dos-Santos A. (2015). Métodos de estudios con otolitos: Principios y aplicaciones. Ciudad Autónoma de Buenos Aires: INPA CONICET UBA.
Voss N. A., Nesis K. N., Rodhouse P. G. (1998). “Systematics, biology and biogeography of the family Histioteuthidae (Oegopsida),” in Systematics and biogeography of cephalopods. Editors N. A. Voss, M. Vecchione, R. O. Toll, and M. J. Sweeney, 293–373. (Washington, USA: Smithsonian National Museum of Natural History). Smithsonian contribution to Zoology.
Wakabayashi T., Saito K., Tsuchiya K., Segawa S. (2002). Descriptions of Eucleoteuthis luminosa (Sasaki, 1915) and Ornithoteuthis volatilis (Sasaki, 1915) paralarvae in the northwestern Pacific. Venus 60 (4), 237–260. doi:10.18941/venus.60.4_237
Wang Y., Han P., Chen X., Fang Z. (2022). Interannual and ontogenetic stage differences on trophic ecology of neon flying squid Ommastrephes bartramii inferred from stable isotope analyses in beaks. Fish. Res. 249, 106252. doi:10.1016/J.FISHRES.2022.106252
Weiss D. J., Rehkdmper M., Schoenberg R., McLaughlin M., Kirby J., Campbell P. G., et al. (2008). Application of nontraditional stable-isotope systems to the study of sources and fate of metals in the environment. Environ. Sci. Technol. 42, 655–664. doi:10.1021/es0870855
Whiteman J. P., Elliott Smith E. A., Besser A. C., Newsome S. D. (2019). A guide to using compound-specific stable isotope analysis to study the fates of molecules in organisms and ecosystems. Divers. (Basel). 11 (1), 8. doi:10.3390/d11010008
Wiederhold J. G. (2015). Metal stable isotope signatures as tracers in environmental geochemistry. Environ. Sci. Technol. 49 (5), 2606–2624. doi:10.1021/es504683e
Wolff G. A. (1982a). A beak key for eight eastern tropical Pacific cephalopod species with relationships between their beak dimensions and size. Fish. Bull. 80 (2), 357–370.
Wolff G. A. (1982b). A study of feeding relationships in tuna and porpoise through the application of cephalopod beak analysis. Texas, USA, A&M University, College Station, 231. Final Tech. Rep. DAR-7924779.
Wolff G. A. (1984). Identification and estimation of size from the beaks of 18 species of cephalopods from the Pacific Ocean. NOAA Techincal Report NMFS. 17
Wolff G. A., Wormuth J. H. (1979). Biometric separation of the beaks of two morphologically similar species of the squd family Ommastrephidae. Bull. Mar. Sci. 29 (4), 587–592.
Woods B., Walters A., Hindell M., Revill A., Field I., McCormack S., et al. (2022). Trophic structure of Southern Ocean squid: A cross-basin analysis of stable isotopes in archived beaks from predator stomachs. Mar. Ecol. Prog. Ser. 685, 137–152. doi:10.3354/meps13990
Xavier J. C., Allcock L., Cherel Y., Lipinski M. R., Gomes-Pereira J. N., Pierce G., et al. (2015). Future challenges in cephalopod research. J. Mar. Biol. Assoc. U. K. 95, 999–1015. doi:10.1017/s0025315414000782
Xavier J. C., Cherel Y. (2021). Cephalopod beak guide for the Southern Ocean: An update on taxonomy. Cambridge, Cambridgeshire, England: British Antarctic Survey, 129.
Xavier J. C., Cherel Y., Roberts J., Piatkowski U. (2013). How do cephalopods become available to seabirds: Can fish gut contents from tuna fishing vessels be a major food source of deep-dwelling cephalopods? ICES J. Mar. Sci. 70, 46–49. doi:10.1093/icesjms/fss167
Xavier J. C., Clarke M. R., Magalhães M. C., Stowasser G., Blanco C., Cherel Y. (2007a). Current status of using beaks to identify cephalopods: III international workshop and training course on cephalopod beaks, Faial Island, Azores, April 2007. Arquipélago - Life Mar. Sci. 24, 41–48.
Xavier J. C., Croxall J. P., Cresswell K. A. (2005). Boluses: An effective method for assessing the proportions of cephalopods in the diet of albatrosses. Auk 122, 1182–1190. doi:10.1093/auk/122.4.1182
Xavier J. C., Croxall J. P. (2007). Predator-prey interactions: Why do larger albatrosses feed on bigger squid? J. Zool. 271, 408–417. doi:10.1111/j.1469-7998.2006.00224.x
Xavier J. C., Croxall J. P., Reid K. (2003a). Interannual variation in the diets of two albatross species breeding at South Georgia: Implications for breeding performance. Ibis 145, 593–610. doi:10.1046/j.1474-919X.2003.00196.x
Xavier J. C., Croxall J. P., Rodhouse P. G. K. (2002a). Unusual occurrence of Illex argentinus (Cephalopoda: Ommastrephidae) in the diet of the albatrosses breeding at bird island, south Georgia. Bull. Mar. Sci. 71, 1109–1112.
Xavier J. C., Croxall J. P., Trathan P. N., Rodhouse P. G. (2003b). Inter-annual variation in the cephalopod component of the diet of wandering albatrosses Diomedea exulans breeding at Bird Island, South Georgia. Mar. Biol. 142, 611–622. doi:10.1007/s00227-002-0962-y
Xavier J. C., Ferreira S., Tavares S., Santos N., Mieiro C. L., Trathan P. N., et al. (2016). The significance of cephalopod beaks in marine ecology studies: Can we use beaks for DNA analyses and mercury contamination assessment? Mar. Pollut. Bull. 103 (1–2), 220–226. doi:10.1016/j.marpolbul.2015.12.016
Xavier J. C., Geraint G. A., Croxall J. P. (2006). Determining large scale distribution of pelagic cephalopods, fish and crustaceans in the South Atlantic from wandering albatross (Diomedea exulans) foraging data. Ecography 29, 260–272. doi:10.1111/j.2006.0906-7590.04525.x
Xavier J. C., Phillips R. A., Cherel Y. (2011). Cephalopods in marine predator diet assessments: Why identifying upper and lower beaks is important. ICES J. Mar. Sci. 68, 1857–1864. doi:10.1093/icesjms/fsr103
Xavier J. C., Rodhouse P. G., Purves M. G., Daw T. M., Arata J., Pilling G. M. (2002b). Distribution of cephalopods recorded in the diet of Patagonian toothfish (Dissostichus eleginoides) around South Georgia. Polar Biol. 25, 323–330. doi:10.1007/s00300-001-0343-x
Xavier J. C., Walker K., Elliot G., Cherel Y., Thompson D. (2014). Cephalopod fauna of South Pacific waters: New information from breeding New Zealand wandering albatrosses. Mar. Ecol. Prog. Ser. 513, 131–142. doi:10.3354/meps10957
Xavier J. C., Wood A. G., Rodhouse P. G., Croxall J. P. (2007b). Interannual variations in cephalopod consumption by albatrosses at South Georgia: Implications for future commercial exploitation of cephalopods. Mar. Freshw. Res. 58, 1136–1143. doi:10.1071/mf06237
Young R. E. (2009). Links to cephalopod beaks on the tree of life. Available at: http://tolweb.org/notes/?note_id=5274 (Webpage Accessed July 30, 2022).
Zelditch M. L., Swiderski D. L., Sheets H. D. (2004). Geometric morphometrics for biologists: A primer. Oxford, UK: Academic press, Elsevier.
Ziegler A., Sagorny C. (2021). Holistic description of new deep sea megafauna (Cephalopoda: Cirrata) using a minimally invasive approach. BMC Biol. 19 (1), 81–14. doi:10.1186/s12915-021-01000-9
Appendix
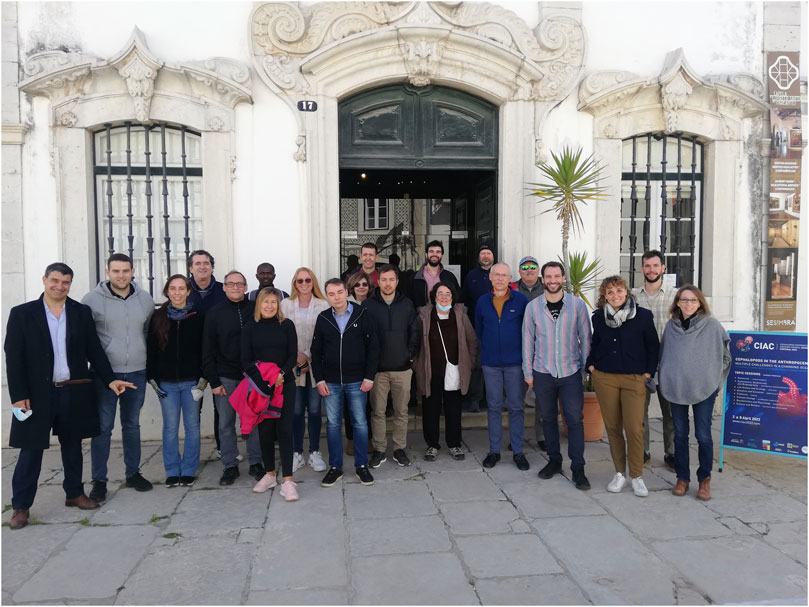
FIGURE A1. Group photo of the participants, in person, of the CIAC 2022 beak workshop (Sesimbra, Portugal).
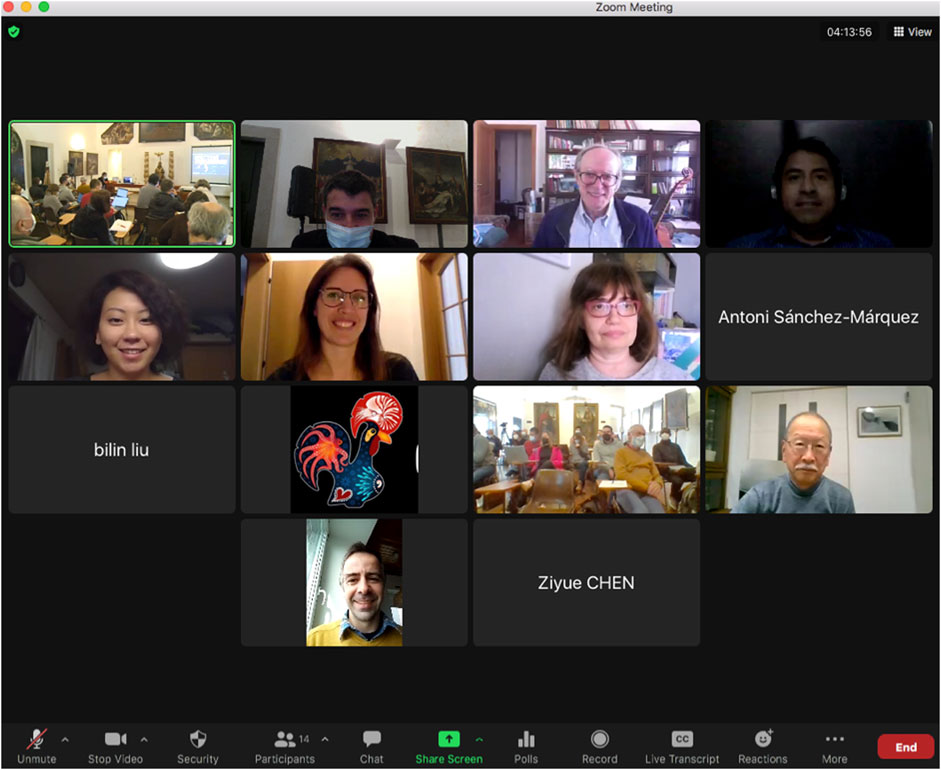
FIGURE A2. Group photo of the participants online of the CIAC 2022 beak workshop (Sesimbra, Portugal).
Keywords: cephalopod ecology, beak taxonomy/composition/morphology/microstructure/paleontology, cephalopod trophic dynamics, cephalopod population dynamics, cephalopod ecotoxicology
Citation: Xavier JC, Golikov AV, Queirós JP, Perales-Raya C, Rosas-Luis R, Abreu J, Bello G, Bustamante P, Capaz JC, Dimkovikj VH, González AF, Guímaro H, Guerra-Marrero A, Gomes-Pereira JN, Hernández-Urcera J, Kubodera T, Laptikhovsky V, Lefkaditou E, Lishchenko F, Luna A, Liu B, Pierce GJ, Pissarra V, Reveillac E, Romanov EV, Rosa R, Roscian M, Rose-Mann L, Rouget I, Sánchez P, Sánchez-Márquez A, Seixas S, Souquet L, Varela J, Vidal EAG and Cherel Y (2022) The significance of cephalopod beaks as a research tool: An update. Front. Physiol. 13:1038064. doi: 10.3389/fphys.2022.1038064
Received: 06 September 2022; Accepted: 25 October 2022;
Published: 16 November 2022.
Edited by:
Alfonso N. Maeda-Martinez, Unidad Nayarit del Centro de Investigaciones Biológicas del Noroeste, MexicoReviewed by:
Michael J. Kuba, Okinawa International University, JapanCarlos Rosas, National Autonomous University of Mexico, Mexico
Copyright © 2022 Xavier, Golikov, Queirós, Perales-Raya, Rosas-Luis, Abreu, Bello, Bustamante, Capaz, Dimkovikj, González, Guímaro, Guerra-Marrero, Gomes-Pereira, Hernández-Urcera, Kubodera, Laptikhovsky, Lefkaditou, Lishchenko, Luna, Liu, Pierce, Pissarra, Reveillac, Romanov, Rosa, Roscian, Rose-Mann, Rouget, Sánchez, Sánchez-Márquez, Seixas, Souquet, Varela, Vidal and Cherel. This is an open-access article distributed under the terms of the Creative Commons Attribution License (CC BY). The use, distribution or reproduction in other forums is permitted, provided the original author(s) and the copyright owner(s) are credited and that the original publication in this journal is cited, in accordance with accepted academic practice. No use, distribution or reproduction is permitted which does not comply with these terms.
*Correspondence: José C. Xavier, anhhdmllckB6b28udWMucHQ=