- 1Center for Advanced Studies and Technology, University G. d’Annunzio of Chieti-Pescara, Chieti, Italy
- 2Department of Medicine and Aging Sciences, University G. d’Annunzio of Chieti-Pescara, Chieti, Italy
- 3Department of Neuroscience, Imaging and Clinical Sciences, University G. d’Annunzio of Chieti-Pescara, Chieti, Italy
Introduction: Ca2+ levels in adult skeletal muscle fibers are mainly controlled by excitation-contraction (EC) coupling, a mechanism that translates action potentials in release of Ca2+ from the sarcoplasmic reticulum (SR) release channels, i.e. the ryanodine receptors type-1 (RyR1). Calsequestrin (Casq) is a protein that binds large amounts of Ca2+ in the lumen of the SR terminal cisternae, near sites of Ca2+ release. There is general agreement that Casq is not only important for the SR ability to store Ca2+, but also for modulating the opening probability of the RyR Ca2+ release channels.
The initial studies: About 20 years ago we generated a mouse model lacking Casq1 (Casq1-null mice), the isoform predominantly expressed in adult fast twitch skeletal muscle. While the knockout was not lethal as expected, lack of Casq1 caused a striking remodeling of membranes of SR and of transverse tubules (TTs), and mitochondrial damage. Functionally, CASQ1-knockout resulted in reduced SR Ca2+ content, smaller Ca2+ transients, and severe SR depletion during repetitive stimulation.
The myopathic phenotype of Casq1-null mice: After the initial studies, we discovered that Casq1-null mice were prone to sudden death when exposed to halogenated anaesthetics, heat and even strenuous exercise. These syndromes are similar to human malignant hyperthermia susceptibility (MHS) and environmental-exertional heat stroke (HS). We learned that mechanisms underlying these syndromes involved excessive SR Ca2+ leak and excessive production of oxidative species: indeed, mortality and mitochondrial damage were significantly prevented by administration of antioxidants and reduction of oxidative stress. Though, how Casq1-null mice could survive without the most important SR Ca2+ binding protein was a puzzling issue that was not solved.
Unravelling the mystery: The mystery was finally solved in 2020, when we discovered that in Casq1-null mice the SR undergoes adaptations that result in constitutively active store-operated Ca2+ entry (SOCE). SOCE is a mechanism that allows skeletal fibers to use external Ca2+ when SR stores are depleted. The post-natal compensatory mechanism that allows Casq1-null mice to survive involves the assembly of new SR-TT junctions (named Ca2+ entry units) containing Stim1 and Orai1, the two proteins that mediate SOCE.
The role of Calsequestrin in skeletal muscle function
Calsequestrin, a member of the macromolecular complex that regulates EC coupling. Calcium ions (Ca2+) are powerful second messengers in all cell types. The electrochemical gradient existing between the cytoplasm and the extracellular space or intracellular stores (either the endoplasmic or sarcoplasmic reticulum; ER and SR) allows release channels to generate elevations in cytoplasmic Ca2+ concentrations (transient or prolonged) that may control gene transcription, cellular differentiation, communication between different intracellular organelles, cellular metabolism, etc. (Dolmetsch, 2003; Gerke et al., 2005). In muscles, rapid increases in intracellular Ca2+ ([Ca2+]i) regulate contractile function and are generally caused by a large and rapid Ca2+ release from internal stores, i.e., sarcoplasmic reticulum (SR), that follows the depolarization of exterior membranes and that may be supplemented, especially in cardiac and smooth muscle, by Ca2+ entry from the extracellular space. The mechanism that links depolarization of the sarcolemma to Ca2+ release, known as excitation-contraction (EC) coupling (Sandow et al., 1965; Schneider et al., 1973; Rios et al., 1991), is mediated by a coordinated interaction between proteins localized in specialized intracellular junctions named Ca2+ release units (CRUs). CRUs are formed by the association of two separate and well-organized membrane systems that come in close contact with one another (Schneider, 1994; Protasi et al., 2002): on one side the sarcolemma and/or the transverse tubule (TT), on the other the internal terminal cisternae of the SR. In skeletal muscle fibers, EC coupling relies on the physical interaction between voltage gated L-type Ca2+ channel of TTs, the dihydropyridine receptors (DHPR) also known as CaV1.1, and the SR Ca2+ release channel (ryanodine receptor type 1, RyR1). DHPRs and RyRs are the two key elements in the EC coupling machinery (MacLennan and Wong, 1971; Lai et al., 1988). DHPRs are organized in the TT membrane in groups of four, named tetrads, which are physically coupled to the 4 identical subunits of RyR1. Several RyR1, which are visible as feet in electron microscopy (EM), are clustered in ordered arrays forming usually double rows in the junctional face of SR (Franzini-Armstrong, 1970; Saito et al., 1984; Block et al., 1988; Protasi et al., 1997; Protasi et al., 1998; Protasi et al., 2000; Paolini et al., 2004). DHPRs and RyR1-feet are not the only players in skeletal EC coupling as several other complementary proteins play key roles in EC coupling. Among them, Calsequestrin (Casq) located in the SR lumen of the junctional SR domains (also named terminal cisternae), hence in proximity to RyR1 arrays, plays a key role in providing releasable Ca2+ to activate muscle contraction.
The dual role of Calsequestrin as SR Ca2+ buffer and RyR modulator. Casq is an acidic protein that binds Ca2+ within the SR lumen with high capacity, but medium-low affinity (Meissner, 1975; MacLennan and de Leon, 1983). Casq main function is to provide a large pool of rapidly-releasable Ca2+ during EC coupling, mainly acting as a Ca2+ buffer in the SR lumen, i.e. a sponge that accumulates ions in proximity of sites of release (MacLennan and Wong, 1971). To play this role Casq is not evenly distributed throughout the SR lumen, but markedly concentrated in correspondence of SR terminal cisternae, in proximity of the junctional face bearing RyR arrays (MacLennan and Wong, 1971; Campbell et al., 1983; Saito et al., 1984). In deep-etch EM studies, Casq appeared as an electron-dense matrix anchored by thin strands, or anchoring filaments, to the SR membrane (Franzini-Armstrong et al., 1987). These strands are likely accessory proteins, i.e. triadin and junctin, that probably allow communication between Casq and RyRs (Kobayashi et al., 2000; Shin et al., 2000; Boncompagni et al., 2012, 2009; Dulhunty et al., 2009; Dulhunty et al., 2009; Wei et al., 2009; Li et al., 2015). In support of this view, Casq over-expression in cardiac muscle resulted in swelling of SR terminal cisternae (Jones et al., 1998; Tijskens et al., 2003), while its knockout caused disappearance of the electron-dense matrix (Knollmann et al., 2006). As active Ca2+ transport in the SR lumen is limited by the intraluminal SR free Ca2+ concentration (Makinose and Hasselbach, 1965; Weber, 1966; Inesi and de Meis, 1989), Casq presence maintains intraluminal concentrations of free Ca2+ low (Beard et al., 2005), allowing for more efficient inward transport by the sarco-endoplasmic reticulum Ca2+ ATP-ase (SERCA) pumps. This is especially important in fast-twitch fibers, where the amount of Ca2+ cycled in and out of the SR is greater than in slow fibers (Fryer and Stephenson, 1996).
Two isoforms of Casq are expressed in mammalian muscles, products of two different genes: CASQ1 and CASQ2 (Scott et al., 1988; Zarain-Herzberg et al., 1988; Fujii et al., 1990; Arai et al., 1991). Cardiac muscle expresses exclusively Casq2, independently of the developmental stage. On the other hand, in skeletal muscle both isoforms are present: Casq2 is the most abundant isoform in all types of developing skeletal fibers and is still abundantly expressed in adult slow-twitch muscle (Damiani et al., 1990). In fast-twitch fibers, on the other hand, Casq2 is progressively replaced by Casq1, which eventually will remain the only isoform expressed in adult fibers (Sacchetto et al., 1993).
Although evidence from ablation of Casq in Caenorhabditis (C.) Elegans wall muscles (which express a single Casq isoform) and in mammalian striated muscles suggested that Casq is not absolutely essential for muscle function (Knollmann et al., 2006; Paolini et al., 2007), there is now agreement that Casq beside being important for the SR ability to store Ca2+ (Wang et al., 1998) also modulates the activity of the SR Ca2+ release channels, i.e. the RyRs (Ikemoto et al., 1989; Gilchrist et al., 1992; Beard et al., 2002; Beard et al., 2005; Dulhunty et al., 2006). Whereas the specific regulatory function of Casq on the activity of RyR was initially unclear as both activation and inhibition of Ca2+ release were reported (Kawasaki and Kasai, 1994; Ohkura et al., 1995; Szegedi et al., 1999; Herzog et al., 2000; Beard et al., 2002; Beard et al., 2005; Dulhunty et al., 2006), there is now general agreement that Casq reduces the opening probability of RyRs.
Structural and functional consequences of Casq1 ablation in mice
Ablation of Casq1: the initial findings. Will the deletion of CASQ1 be lethal, as the knockouts of RYR1 and α1DHPR (the 2 key proteins of EC coupling) proved to be (Knudson et al., 1989; Takekura et al., 1995)? Is Casq essential for skeletal muscle function, or the initial findings in C. Elegans (Cho et al., 2000) would be also confirmed in mammalian muscle? To address these specific questions, F. Protasi decided in collaboration with his Principal Investigator (Dr. P. D. Allen) and with Lexicon (OmniBank Library, Lexicon Genetics) to start a venture that led to the generation of CASQ1 knockout mice (or Casq1-null). Unexpectedly, Casq1-null mice were viable and fertile, and apparently developed normally under standard housing conditions, raising initially the reasonable doubt that the knockout procedure was unsuccessful. However, western blot (WB) analysis soon confirmed the absence of Casq1 in hindlimb skeletal muscle. In 2002, F. Protasi started the experiments that brough to the publication in 2007 of a first scientific report about Casq1-null mice in J. Physiol. (London). The absence of Casq1 was confirmed by both WB analysis and immunocytochemistry in extensor digitorum longus (EDL) and Soleus muscle, respectively, a predominantly-fast and a predominantly-slow twitch muscle (Paolini et al., 2007). As expected, Casq2 was still present, but its expression was not upregulated to compensate for the lack of Casq1, as initially hypothesized to explain the apparently normal phenotype of knockout mice (Paolini et al., 2007). Casq2 was abundant in Soleus, confined to type I and IIA fibers, but very little Casq2 was found in EDL muscle, mostly composed of fast twitch IIB and IIX fibers. Though, EDL was still functional with most fibers lacking any detectable Casq expression. These initial observations deserved additional investigation focusing on the structural and functional consequences of Casq1 ablation in the EC coupling system.
The structural adaptations of the EC coupling system to lack of Casq1. The EM analysis of Casq1-null EDL and Soleus fibers revealed a significant remodeling of the internal membranes involved in EC coupling, i.e. SR and TTs, though different in the two muscles (Figure 1). In Soleus the most evident alteration in EC coupling sites (i.e. CRUs) was a significant reduction in size of SR terminal cisternae, a shrinkage that was also present in EDL fibers (Paolini et al., 2007). In EDL, though, the structural impact of Casq1 ablation was not limited to the reduction in size of the SR lumen in terminal cisternae, but a striking remodeling of CRUs caused the formation of SR-TT-SR junctions often formed by multiple elements (5, 7, even 9) (Paolini et al., 2007), while in control muscle CRUs are usually formed by only three elements (2 SR terminal cisternae flanking a central TT) which are named triads. Furthermore, the Ca2+ release channels (RyRs) in these multi-layered CRUs were not organized in two rows as in normal triads but formed by multiple rows similar the arrays of RyR in dyads of cardiomyocytes (Sun et al., 1995; Protasi et al., 1996; Franzini-Armstrong et al., 2005). These findings suggested that multi-layered junctions bearing multiple rows of RyR-feet should contain more RyR1 than normal wild type (WT) fibers, a fact that was promptly confirmed by counting RyR-feet in EM and by ryanodine-binding studies (Paolini et al., 2007).
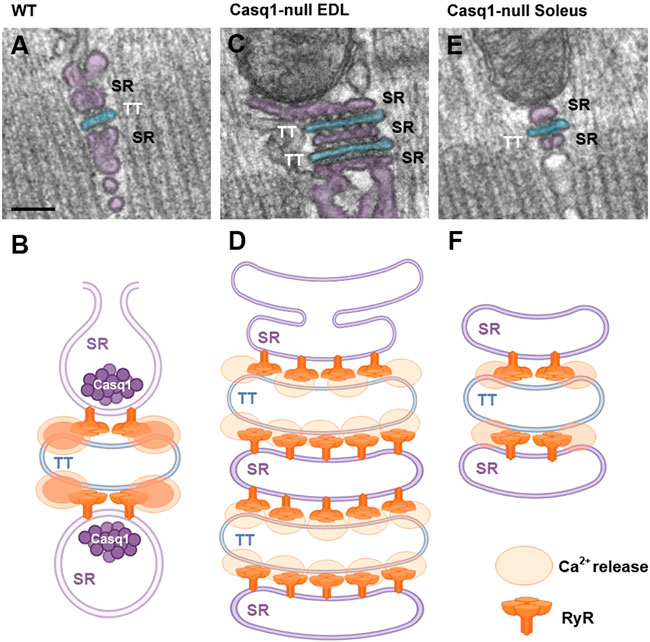
FIGURE 1. Representative EM images and schematic cartoons depicting CRUs in WT and Casq1-null fibers. (A,B) In WT fibers, CRUs are formed by three elements (2 SR terminal cisternae flanking a central TT), SR has a wide profile and contains a dark matrix representing Casq. RyRs usually form two rows spanning the junctional gap between SR and TT. (C–F) In Casq1-null fibers SR terminal cisternae are significantly narrower than in WT as they lack Casq-matrix; in EDL fibers CRUs are often formed by multiple elements decorated by multiple rows of RyRs. Scale bars: (A, C, E), 0.1 μm.
The shrinkage of terminal cisternae in both Soleus and EDL fibers could be quite easily explained by the lack of Casq1 in the SR lumen of terminal cisternae. Indeed, C. Franzini-Armstrong in a series of elegant papers demonstrated that: a) the SR terminal cisternae is filled with an electron-dense matrix (anchored to the SR membrane by thin strands) and proposed that this matrix was polymerized Casq (Franzini-Armstrong et al., 1987); b) the amount of Casq expressed in muscle fibers can influence size and architecture of the junctional SR (Jones et al., 1998; Tijskens et al., 2003; Knollmann et al., 2006; Franzini-Armstrong, 2009). Hence, in Casq1-null fibers the reduction in size of the SR terminal cisternae is very likely the direct effect of lack of Casq1 in the SR lumen. In support of this hypothesis came studies of re-expression of Casq1 in Casq1-null fibers, which restored the width of junctional SR (Tomasi et al., 2012).
On the other hand, why the membranes of CRUs of EDL fibers proliferate to form multilayered junctions remains to be determined. One hypothesis is that the lack of Casq1 causes a reduction of releasable Ca2+ (a fact that was indeed confirmed in functional studies; see below), which in turn would trigger compensatory mechanisms aiming to increase junctional SR volume and/or the number of release sites (i.e. more RyR1 channels available for Ca2+ release). Multilayered CRUs do not form in Soleus fibers even when both Casq1 and 2 were missing (Paolini et al., 2011), suggesting that their formation is not the direct result of lack of Casq, but possibly an adaptation of EDL fast-twitch fibers which need more and larger transients than slow-twitch fibers to supply Ca2+ ions to the contractile apparatus.
Other structural modifications caused by Casq1 ablation in skeletal fibers. While studying the EC coupling machinery (Paolini et al., 2007), we noticed that EDL fibers from Casq1-null mice exhibited areas of partial disarray of the contractile apparatus and swollen/damaged mitochondria. In Paolini et al., 2015, the authors studied more in depth these fiber damage using histological sections, and electron/confocal microscopy. Authors found that Casq1-null mice, beside the remodeling of membranes involved in EC coupling previously described (Paolini et al., 2007), also developed a mild myopathy characterized by mitochondrial damage (starting in adulthood), that eventually caused the formation of structural and contracture cores at later ages (Paolini et al., 2015). Misalignment of contractile elements, Z-line streaming, and regions in which myofibrils were over-contracted progressively increased from 4–6 months to 14–24 months of age (Paolini et al., 2015). Interestingly, similar alterations were described previously in biopsies from patients diagnosed with Central Core Disease (CCD) and in RYR1-knockin mice carrying human mutations associated to CCD (Boncompagni et al., 2009b; Zvaritch et al., 2009). Muscle damage was accompanied by a significant decrease in body weight across all ages (compared to age-matched WT mice), which at least in part could be ascribed to a reduction of muscle mass (Paolini et al., 2015). Cross-sectional area (CSA) of skeletal fibers (Paolini et al., 2015) was on average reduced: one possible mechanism underlying the atrophy of muscle fibers from Casq1-null mice was up-regulation of four atrogenes (CathepsinL, Bnip3, Psmd1, and Atrogin1) that belong to the autophagy and ubiquitin-proteasome pathways, as assessed by microarray analyses of EDL muscles (Paolini et al., 2015). Even though, the molecular analysis found increased expression of PGC-1α, a transcriptional co-activator that stimulates mitochondrial biogenesis and promotes the remodeling of muscle tissue toward a more oxidative metabolism (Lin et al., 2005; Liang and Ward, 2006), histological analyses of EDL muscles from Casq1-null mice did not find fiber-type switching. In turn, the overexpression of PGC-1α could be mediated by the increased cytosolic [Ca2+] in Casq1-null fibers (Dainese et al., 2009).
Why ablation of Casq1 leads to mitochondrial damage and formation of cores is still unclear. Though, we know that in fast-twitch fibers of mice most mitochondria are positioned in proximity to CRUs, linked to them by tethers (Boncompagni et al., 2009a; Protasi et al., 2021b). It is also well established that mitochondrial function depends on Ca2+ release during EC coupling (Rudolf et al., 2004; Rossi et al., 2011) as Ca2+ entry into the mitochondrial matrix functions as a signal that activates aerobic ATP production (Jouaville et al., 1999; De Stefani et al., 2011; Gherardi et al., 2020). The proximity between mitochondria and CRUs may be crucial for proper Ca2+ signaling between the two organelles (Boncompagni et al., 2009a; Rossi et al., 2009; Rossi et al., 2011). This considered, one could hypothesize that Casq1 ablation causes SR Ca2+ leak (due to lack of Casq1 inhibition of RyR1 opening probability) (Beard et al., 2002; Beard et al., 2005), which in turn could: a) increase energy demand for Ca2+ re-uptake; and/or b) promote mitochondriogenesis to provide additional energy and/or also cytosolic Ca2+ buffering capacity: the volume of mitochondria in Casq1-null fibers was indeed increased (Paolini et al., 2007), possibly as a result of increased expression of PGC-1α; c) cause excessive Ca2+ entry that could damage mitochondria: studies conducted in single fibers from double Casq-null mice - which lack both Casq1 and 2 (dCasq-null) - revealed that the mitochondrial [Ca2+]free at rest was higher compared to WT (Scorzeto et al., 2013).
The increased structural damage of mitochondria could be the result of a combined action of increased cytosolic Ca2+ and also of a redox imbalance. Indeed, the decrease in GSH/GSSG ratio and increase in mSOF activity in muscle from Casq1-null mice were both indicative of increased oxidative stress (Wang et al., 2008; Dainese et al., 2009; Michelucci et al., 2015; Paolini et al., 2015). Mitochondria exposed to excessive cytosolic Ca2+ may not function properly and, thus, produce excessive oxidative species of oxygen (ROS), leading to a dangerous feed-forward mechanism that may underline mitochondrial and cellular damage. Membrane structures of the cells can be affected by increased oxidative stress which is known to cause lipid peroxidation (Davies et al., 1982). This vicious cycle resembles that described in mouse model which carries a RYR1Y522S/WT mutation linked to MHS in humans (Durham et al., 2008; Boncompagni et al., 2009b; Wei et al., 2011).
How the mitochondrial damage will later lead to formation of structural cores also remains to be defined. Mitochondrial damage could lead to release of their content, which is known to be a proapoptotic signal (Liu et al., 1996; Zou et al., 1997; Goldstein et al., 2000; Wang, 2001; Garrido et al., 2006; Munoz-Pinedo et al., 2006; Green and Llambi, 2015). In fact, when mitochondria are damaged, cytochrome-c, a protein which in healthy cells is found only in the mitochondrial intermembrane space, is released from the mitochondrial electron transfer chain and initiates caspase activation (Liu et al., 1996; Garrido et al., 2006). In addition, increase in cytosolic Ca2+ alone may independently of mitochondria activate the proteolytic machinery, which may break down myofibrils and other intracellular components (Gissel, 2005).
The functional consequence of lack of Casq1 in the SR. Despite the striking morphological remodelling of intracellular membrane systems involved in EC coupling (possibly a postnatal attempt of muscle to compensate for the lack of Casq1), Casq1-null fibers were still functionally impaired. Studies in whole muscles showed that Casq1 ablation caused compromised Ca2+ release and reuptake by the SR, i.e. prolonged time-to-peak and half-relaxation time. Both parameters were more pronounced in EDL than in Soleus (Paolini et al., 2007), a fact that can be easily explained by the greater dependence of EDL on Casq1, as slow-twitch muscles contain more Casq2. The direct measurement of Ca2+ efflux from the SR in isolated single flexor digitorum brevis (FDB) fibers (Paolini et al., 2007) revealed: a) significantly smaller Ca2+ transients evoked by electrical stimulation in Casq1-null fibers compared to WT; b) greatly reduced amount of Ca2+ stored in the SR, as shown by application of 20 mM caffeine in permeabilized fibers.
A few years later two other papers were published and added additional information toward the full understanding of the mechanisms underlying Ca2+ handling in fibers lacking Casq1. Using Ca2+dyes of new generation that allowed independent measurements of [Ca2+]free in myoplasm and SR lumen:
a) Canato and coll. (2010) demonstrated that CASQ1-knockout results in deep SR depletion under high-frequency stimulation. These findings were explained by the absence of Casq1 in proximity of release sites which would lower the amount of releasable Ca2+ during repetitive EC coupling activation. The authors also underlined the fact that in Casq1-null fibers the intermediate step of reuptake, directly dependent on the SR [Ca2+]free, is the mostly affected, both in terms of amplitude and rate constant (Canato et al., 2010).
b) Royer and coll. (2010), using fibers from dCasq-null mice (lacking both Casq1 and 2), while confirming SR depletion in dCasq-null fibers, added elegant information regarding the rate-velocity of Ca2+ efflux from the SR in presence or absence of Casq (Royer et al., 2010). In their description of a new parameter named evacuality (E), they stated that: a) E is directly proportional to SR Ca2+ permeability and inversely to its Ca2+ buffering capacity; and b) E starts low and increases progressively as the SR is depleted in WT fibers, while E starts high and decreases upon SR Ca2+ depletion in dCasq-null fibers.
Studies of contraction kinetics in dCasq-null mice were also used to investigate possible differences between fast- and slow-twitch muscles (Paolini et al., 2011). Analysis of grip-strength test in dCasq-null mice (compared to Casq1-null) indicated that muscular function of mice is mainly dependent on the Casq isoform. This is not surprising as most muscle groups of mice are mainly fast-twitch, hence dependant mainly on Casq1. Even in Soleus muscles (a slow twitch muscle containing a considerable amount of Casq2) during high-frequency and prolonged stimulation (2 s pulses), tetanic tension showed only a modest decline, and - contrary to EDL - not a great drop in force.
Re-expression of exogenous Casq1 in skeletal muscle fibers of Casq1-null mice restored almost completely, beside the structure of CRUs (see above), also the functional parameter of Ca2+ transient. The corrected (structural and) functional parameters were ascribed only to Casq1 re-introduction, as all other SR proteins that could participate in Ca2+ homeostasis did not vary their expression level after re-expression of exogenous Casq1 (Tomasi et al., 2012).
The discovery of the MHS/HS phenotype of Casq1-null mice
Increased spontaneous mortality rate in Casq1-null mice. During the years that brought to the first publication in J. Physiol. (Paolini et al., 2007), we noticed a significantly increased rate of spontaneous mortality in Casq1-null mice, particularly after 3 months of age. Initially we did not pay enough attention to this phenomenon, but then realized that most of those deaths were registered in male mice while they were housed in reproductive cages (Dainese et al., 2009). This observation suggested a possible contribution of stress due to mating to mortality rate. Reviewing the existing literature, we discovered that a similar phenotype was reported in animals carrying a defect in EC coupling. Indeed, in porcine stress syndrome (PSS), pigs carrying a mutation in RYR1 causing SR Ca2+ leak were prone to sudden death during emotional stress due to transport to market, mating, administration of anesthesia, prolonged exposure to elevated ambient temperature and even during vigorous exercise (Jones et al., 1972; Nelson et al., 1972; Fujii et al., 1991). The PSS is similar to malignant hyperthermia susceptibility (MHS), an abnormal reaction caused in humans by administration of halogenated anaesthetics which affects individuals carrying specific mutations in RYR1 (Denborough and Lovell, 1960; Denborough, et al., 1962; Denborough et al., 1970a; Denborough et al., 1970b; Galli et al., 2006; Wu et al., 2006). Though, MH susceptible individuals may trigger hyperthermic episodes also when exposed to high environmental temperature and/or strenuous exercise: some of these crises and exercise-induced rhabdomyolysis may occur in subjects with a family history of MHS (Wyndham, 1977; Pamukcoglu, 1988; Ryan and Tedeschi, 1997; Tobin et al., 2001; Wappler et al., 2001; Davis et al., 2002; Capacchione et al., 2010; Dlamini et al., 2013). In these cases, hyperthermic reactions are not referred to MHS, but as episodes of exertional-environmental heat stroke (HS).
Based on the above knowledge, we developed the hypothesis that ablation of Casq1 in mice could have caused a pathological phenotype that somehow resembled human MHS or HS. This syndrome would have been responsible of sudden death in male mice during mating. In support of this hypothesis, two additional pieces of information:
1. In cardiac muscle, gain-of-function mutations in RYR2 and loss-of-function mutations in CASQ2 cause a similar phenotype, i.e. catecholaminergic polymorphic ventricular tachycardia (CPVT), which is caused by excessive SR Ca2+ leak (Knollmann et al., 2006).
2. Casq1 was proposed to inhibit the RyR1 opening probability (Beard et al., 2002; Beard et al., 2005), suggesting that Casq1 ablation could in principle cause SR Ca2+ leak, as gain-of-function mutations in RYR1.
Hence, we decided to test the possibility that Casq1-null mice were susceptible to trigger MH/HS episodes in response to halothane and heat.
The discovery of Casq1-null mice being susceptible to MH and HS episodes. In Dainese et al., 2009, we first reported the increased mortality rate of male Casq1-null mice and then tested MH/HS susceptibility exposing mice to halothane (2%, 1 h) and heat (41°C, 30 min). Both protocols were previously used to test the phenotype of RYR1-knockin mice expressing human mutations linked to MH (Chelu et al., 2006; Yang et al., 2006; Durham et al., 2008). During both protocols male Casq1-null mice exhibited a high mortality rate (about 80% of male Casq1-null mice died), while females were only slightly more susceptible than WT (Dainese et al., 2009). MH/HS episodes were characterized by whole body contracture, hyperthermia, and rhabdomyolysis. Importantly, both crises induced by halothane and by heat could be completely prevented by prior intraperitoneal injection of dantrolene (Dainese et al., 2009), the only drug approved for acute treatment of MH in humans (Wedel et al., 1995). Regarding the gender difference in susceptibility, a difference in mortality rate between males and females was also reported in many epidemiologic studies of MH in humans (Strazis and Fox, 1993; Sidman and Gallagher, 1995; Brandom and Larach, 2002; Burkman et al., 2007; Migita et al., 2007). EDL muscles dissected after the exposure to the heat challenge were severely damaged, presenting regions of contractures and overstretched sarcomeres, Z-line misalignment, loss of M-line, and SR swelling (Dainese et al., 2009). As muscle damage suggested rhabdomyolysis (a classic feature of MH and HS crises), we isolated intact EDL muscles and subjected them to an in-vitro contracture test (IVCT), the gold standard protocol used to diagnose MH in humans (1984). Muscles of Casq1-null mice exhibited sensitivity to both temperature and caffeine and developed contractures more easily than muscles from WT mice (Dainese et al., 2009). Also, intracellular Ca2+ levels were increased at physiological temperatures, both in adult FDB fibers and myotubes of Casq1-null mice (Dainese et al., 2009).
Together, these results suggested that the anesthetic and heat sensitivity of Casq1-null mice closely resemble that of pigs affected by PSS and of mouse models of MH/HS carrying gain-of-function mutations in the RYR gene (Ording et al., 1985; Fujii et al., 1991; Yang et al., 2006; Durham et al., 2008). Furthermore, our results reinforced the correlation between MHS and HS and supported the hypothesis that these two syndromes may share a common pathogenic mechanism (Durham et al., 2008; Dainese et al., 2009; Michelucci et al., 2015; Michelucci et al., 2017b). Recently, we have also shown how oxygen consumption and basal metabolic rate may be used as markers susceptibility of mice to MH and HS (Serano et al., 2022).
Role of oxidative stress in SR Ca2+ leak and in hyperthermic crises. In 2008, it was proposed that a key molecular event leading to hyperthermic crises is the excessive production of oxidative species of oxygen and nitrogen (ROS and NOS) by mitochondria, which in turn would exacerbate SR Ca2+ leak via RyR1 nitrosylation (Durham et al., 2008). In Michelucci et al., 2015 the efficacy of two different antioxidant compounds in preventing hyperthermic reactions was tested in-vivo in Casq1-null mice. N-acetylcysteine (NAC) and Trolox were respectively administrated in drinking water or by intraperitoneal injection. Both treatments were quite successful in reducing the mortality rate of Casq1-null mice in response to exposure to heat (41°C, 1 h) and halothane (2%, 1 h at 32°C): from about ∼80% to only ∼20%. Animals treated with antioxidant also showed a decreased rise in core temperature during the heat challenge and a delayed onset of lethal crises (Michelucci et al., 2015). In addition, NAC treatment also: a) reduced muscle damage during exposure to heat stress (assessed by quantifying: the number of damaged fibers in histology, serum CK levels, and plasma K+ and Ca2+ concentrations); b) rescued structural alterations observed in Casq1-null mice at rest, reducing mitochondrial damage (Paolini et al., 2015); and finally c) normalized IVCT, lowering the caffeine sensitivity of resting tension in EDL muscles from Casq1-null muscles.
To confirm that antioxidants reduced oxidative stress, authors first measured mitochondrial superoxide flashes (mSOF) activity after the treatment with NAC in dissociated FDB muscle fibers (Wei et al., 2011; Michelucci et al., 2015; Cheng et al., 2018): mSOF frequency (elevated in knockout fibers) was significantly reduced by NAC both at 20° and 37°C (Michelucci et al., 2015). In addition, biochemical analyses performed in EDL muscles revealed that NAC treatment reduced the expression levels of superoxide dismutase-1 (SOD-1), 3-Nitro-l-Tyrosine (3-NT), and finally lowered reduced/oxidized glutathione ratio (GSH/GSSG) in comparison to muscle of untreated Casq1-null mice. As SOD-1 is one of the main antioxidant enzymes (McCord and Fridovich, 1969), 3-NT are direct products of oxidative damage on proteins (Ogino and Wang, 2007), and GSH/GSSG ratio reflect the overall cellular redox state, data collected indicated that NAC treatment reduced oxidative stress (Michelucci et al., 2015).
To verify if the mechanisms underlying the effect of antioxidants involved modulation of intracellular Ca2+ levels, the effect of NAC treatment on Ca2+ transients was also tested directly in isolated FDB fibers loaded with a ratiometric Ca2+ dye in experiments at rest and during prolonged high-frequency stimulation (60 Hz, 2 s) (Michelucci et al., 2015).
In two later studies published between 2017 and 2018, additional evidence pointing to a central role of oxidative stress in HS crises was collected (Michelucci et al., 2017a; Guarnier et al., 2018).
(a) In the first study to investigate the gender difference in the phenotype of Casq1-null mice (female mice being more protected than males during exposure to halothane or environmental heat), we changed the hormonal profile of mice (Michelucci et al., 2017a). Many studies in literature reported a higher percentage of MHS cases in males than females (about 70/80% vs females) (Strazis and Fox, 1993; Larach et al., 2001; Burkman et al., 2007; Migita et al., 2007). The potent cellular antioxidant properties of estrogens, female sex steroid hormones, are well documented in literature: (i) upregulation of several antioxidant enzymes, (ii) downregulation of ROS-generating enzymes, and (iii) direct free-radical scavenging properties (Sugioka et al., 1987; Mooradian, 1993; Gomez-Zubeldia et al., 2001; Wagner et al., 2001; Florian et al., 2004; Borras et al., 2005). To test if estrogens were protecting female mice from MH/HS crises, we treated for 1 month (3–4 months of age): (i) male Casq1-null mice with Premarin (a dose of 40 ng/g of body weight every day for 1 month; McCullough et al., 2001; Shen et al., 2008) and (ii) female Casq1-null mice with Leuprolide (a dose of 100 ng/g of body weight every day for 1 month), known to abolish estrogens production (Wilson et al., 2007).
(b) In the second paper published in 2018, as aerobic training is known to increase endogenous antioxidant protection (Venditti and Di Meo, 1997; de Sousa et al., 2017), we subjected Casq1-null mice to aerobic training on treadmill for 2 months at 60% of their maximal speed (1 h, 5 times/week).
In short, both estrogen administration (Michelucci et al., 2017a) and aerobic training (Guarnier et al., 2018) protected male Casq1-null mice from lethal episodes by reducing hyperthermia during exposure of mice to heat stress. The protective effect of these treatments was also visible in IVCT: the generation of tension of EDL muscles isolated from Casq1-null mice to increasing [caffeine] and/or temperature was reduced by administration of Premarin and also by aerobic exercise. Also, rhabdomyolysis of skeletal muscle fibers and mitochondrial damage were prevented/reduced by estrogens and training in EDL muscle from Casq1-null mice. Analysis of oxidative stress levels confirmed the antioxidant role of estrogens and of aerobic training in muscles of Casq1-null mice: (i) GSH/GSSG ratio, (ii) levels of nitrated proteins (3-NT) (Michelucci et al., 2017a), (iii) levels of carbonyl proteins and (of diene conjugates (Guarnier et al., 2018) were all normalized. Note that administration of Leuprolide to female Casq1-null mice, a treatment that reduces the production of estrogens (Michelucci et al., 2017a), increased the susceptibility of female mice to heat stress. Together, the results contained in these two studies (Michelucci et al., 2017a; Guarnier et al., 2018) reinforced: a) the hypothesis proposed by Durham and coll. (2008) that oxidative stress plays a key role in hyperthermic crises; b) that antioxidants could be in principle used as preventive measure in periods of intense environmental heat to reduce the risk of HS.
Exertional stress is also a factor of risk for hyperthermic crises in Casq1-null mice. Humans experience hyperthermic crises with very similar symptoms, either as a result of the administration of volatile anesthetics (malignant hyperthermia, MH), or when exposed to environmental heat or to intense physical/emotional stress (environmental/exertional HS) (Wyndham, 1977; Pamukcoglu, 1988; Ryan and Tedeschi, 1997; Tobin et al., 2001; Wappler et al., 2001; Davis et al., 2002; Capacchione et al., 2010; Dlamini et al., 2013). Some examples: a) muscle biopsies from two soldiers who have experienced episodes of exertional HS were positive to halothane during IVCT (Hopkins et al., 1991); b) MH patients were associated with either non-specific myopathies or congenital disorders in three separate families; two of these patients also showed evidence of exercise-induced rhabdomyolysis (Davis et al., 2002); c) life-threatening episodes triggered by either environmental heat or physical exertion are characterized by hyperthermia and rhabdomyolysis, a pathophysiologic fingerprint also typical of anesthetic-induced MH crises (Hopkins et al., 1991; Bouchama and Knochel, 2002; Davis et al., 2002; Capacchione et al., 2010; Lehmann-Horn et al., 2011).
While susceptibility to anesthetics and environmental heat was tested between 2006 and 2009 in knock-in mice carrying RYR1 mutations linked to MH in humans (Chelu et al., 2006; Yang et al., 2006; Durham et al., 2008) and in Casq1-null mice (Dainese et al., 2009), the susceptibility to physical exertion remained to be tested. For this reason, we subjected RYR1Y522S/WT and Casq1-null mice to an exertional-stress (ES) protocol, i.e. incremental running on a treadmill placed in an environmental chamber in which temperature and humidity were kept at 34°C and 40% respectively (Michelucci et al., 2017c). We also tested the efficacy of drugs commonly used in humans to block MH reactions triggered by administration of anesthetics. The main findings of this study were:
(a) Lethal crises occurred in about 80% of both RYR1Y522S/WT and Casq1-null mice exposed to the ES protocols, while no lethal episodes were registered in WT mice. Of note, the lethal crises during the ES protocol displayed clinical signs very similar to those experienced by the same animals when exposed to either halothane or environmental heat (i.e., whole-body contractures, difficulty in breathing, and impaired and spasmodic movements).
(b) Pretreatment of susceptible mice 60min prior the ES protocol with a single injection of Azumolene (a Dantrolene analog which is more water soluble) was effective in preventing exertional HS: the mortality rate was indeed almost completely abolished in both mouse models, and also core temperature was reduced in pre-treated animals.
c) Azumolene reduced also muscle damage during ES protocol, and normalized IVCT and intracellular Ca2+ levels during exposure to increasing [caffeine].
(d) Finally, as in MH crisis triggered by either exposure to halothane and heat ROS and RNS had a pivotal role in promoting a feed-forward mechanism that led to excessive SR Ca2+ release (Durham et al., 2008; Michelucci et al., 2015), we measured the levels of 3-NT, a product of nitration of tyrosine residues in proteins mediated by RNS (Ogino and Wang, 2007). Muscles of RYR1Y522S/WT and Casq1-null mice showed higher levels of 3-NT already in basal conditions (compared to WT), and even more following ES; though, pretreatment with Azumolene was effective in controlling the increase in 3-NT levels during exposure to the ES protocol.
The data collected in this study (Michelucci et al., 2017c) suggested: a) MH susceptible individuals could, in principle, be considered at risk for exertion-induced episodes; and b) drugs that are commonly used to treat classic anesthetic MH reactions (i.e., Dantrolene) could be considered as possible pharmacologic interventions for acute HS, a class of disorders that currently has no cure.
Why Casq1-null mice were viable? Finally solving the mystery
The role of Store Operated Ca2+ entry in skeletal muscle fibers. Ca2+ homeostasis in skeletal fibers is the result of a complex interplay between several mechanisms: release of Ca2+ by EC coupling, Ca2+ re-uptake by the SR, Ca2+ binding to sarcomeric and cytoplasmic proteins (Troponin, Parvalbumin, etc.), mitochondrial Ca2+ uptake, etc. (Calderon et al., 2014) In addition, during prolonged and repetitive stimulation, fibers may lose some Ca2+ in the extracellular space via plasma membrane Ca2+ ATP-ases (PMCA). In turn, this may result in a reduction of the total amount of Ca2+ stored in the SR, which is one the main mechanism underlying muscle fatigue (Lamb, 2002). Though, muscle fibers have developed systems to recover extracellular Ca2+ when the amount of Ca2+ in intracellular SR stores is too low to support normal muscle function: this condition is known as SR depletion.
Depletion of intracellular Ca2+ stores (ER and SR) in excitable and non-excitable cells is known to be the trigger for the activation of store-operated Ca2+ entry (SOCE). SOCE is a ubiquitous Ca2+ influx mechanism in which Stim1 (stromal interaction molecule-1), a Ca2+ sensor in the lumen of the ER/SR (Liou et al., 2005; Roos et al., 2005; Zhang et al., 2005), and Orai1, a Ca2+ release-activated Ca2+ (CRAC) channel of external membranes (Feske et al., 2006; Prakriya et al., 2006; Vig et al., 2006; Yeromin et al., 2006), are the two main players. Activation of SOCE is triggered by dimerization of Stim1 in the ER/SR when [Ca2+] is too low, which then activates Orai1 in the plasma membrane that allows Ca2+entry (Michelucci et al., 2018; Qiu and Lewis, 2019). In muscle, it has been demonstrated that SOCE is also modulated by Casq1: Wang and coll (Wang et al., 2015) reported a retrograde regulation of Stim1-Orai1 interaction (and SOCE) by Casq. Casq seems to exert its role in SOCE through the formation of a complex between the aspartate-rich segment of Casq1 and Stim1, which inhibits Stim1 aggregation (Zhang et al., 2016). As the physiological role of Casq1 would be to inhibit the SOCE mechanism, the deletion of CASQ should in principle upregulate SOCE. Indeed, accelerated activation of SOCE current in myotubes from Casq1-null mice was already reported (Yarotskyy et al., 2013).
SOCE activity in muscle is important to limit fatigue during sustained and repetitive activity (Wei-Lapierre et al., 2013; Carrell et al., 2016; Michelucci et al., 2018). As SOCE activation in muscle is apparently faster than in non-excitable cells (Launikonis et al., 2003; Launikonis and Rios, 2007), it was initially proposed that the interaction of Stim1 and Orai1 would occur in CRUs, the sites of EC coupling where TTs and SR (containing respectively Orai1 and Stim1) are already in contact with one another (Dirksen, 2009). However, later work by our group demonstrated that SOCE in muscle occurs in different junctions that are formed at the I band between stacks of SR membranes and extensions of TT, named Ca2+ Entry Units (CEUs) (Boncompagni et al., 2017; 2018; Protasi et al., 2021a). These junctions are few and small in muscle at rest, but become larger and more numerous during acute exercise (Figure 2). We demonstrated that CEUs contain Stim1 and Orai1 proteins, which increase their colocalization during exercise thank to elongation of TTs at the I band and remodeling of SR membranes in flat cisternae.
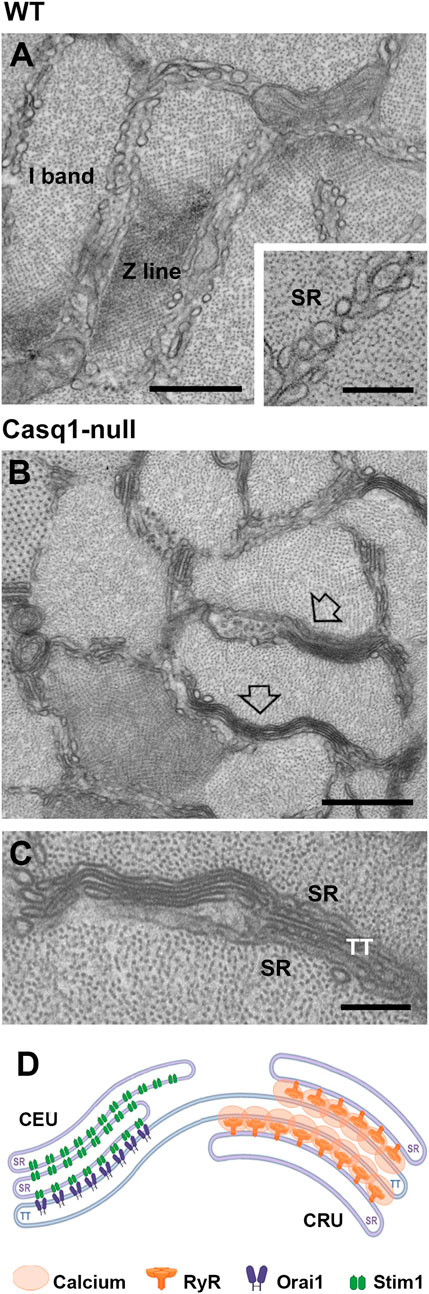
FIGURE 2. Representative EM images of SR at the I band of WT and Casq1-null fibers and schematic cartoon of SR remodeling in Casq1-null fibers. (A) SR at I band of WT EDL fibers appear as SR vesicles and small tubes. (B,C) In Casq1-null EDL fibers, SR at the I band is often remodeled in SR-stacks (pointed by empty arrows), one the two components of CEUs. (D) Cartoon showing a CEU and a CRU, which are both junctions between SR at TT, though they contain different proteins (see symbol legend). Scale bars: (A,B), 0.2 μm; inset in (A,C) 0.5 μm.
Assembly of CEUs during exercise have been correlated with: a) increased fatigue resistance in presence of external Ca2+, which is lost when extracellular Ca2+ is removed or Ca2+ entry blocked by supplementation in the extracellular solution with SOCE blockers (BTP-2 and 2-APB) (Boncompagni et al., 2017); b) reduced decline of Ca2+ transient amplitude during repetitive high-frequency stimulation; c) accelerated rate of Mn2+ quench, the gold standard technique used to assess SOCE function (Michelucci et al., 2019). We also reported that CEUs are dynamic structures as they promptly assemble during exercise, but also disassemble in the first hours during recovery, when TTs retract from SR-stacks preventing Orai1 from interacting with Stim1, hence inactivating the function of SOCE (Michelucci et al., 2019).
Casq1-null fibers contain constitutively assembled Ca2+ Entry Units. As Casq was considered one of the three key proteins on EC coupling (together with RyR1 and DHPR), when we planned to generate Casq1-null mice we expected a lethal phenotype. We were not expecting that muscle fibers could function in absence of Casq1, yet surprisingly mice were alive and developed quite normally to adulthood (see paragraph Structural and functional consequences of Casq1 ablation in mice). As detailed above, EM allowed us to discover that lack of Casq1 induced morphological adaptations to sites of EC coupling: CRUs were formed by multiple SR-TT-SR junctions and fibers contained an almost doubled amount of RyR1 (Paolini et al., 2007) (Figure 1). At the time we thought that these structural adaptations must have been sufficient to allow an almost normal function of skeletal fibers. Though, we did not have the foresight to see what we recently discovered (Michelucci et al., 2020).
Before our first publication in 2007, while performing EM to analyse the EC coupling machinery, we did not notice that lack of Casq1 induced also the formation of stacks of flat SR cisternae oriented longitudinally between myofibrils, mostly located at the I band (Figure 2). SR-stacks in Casq1-null fibers were first reported by Boncompagni et al., 2012. Structures virtually identical to these SR-stacks were reported in mice lacking junctophilin, and described as irregular triad junctions by Ko and coll. (Ko et al., 2011). After that, SR-stacks were also found in triadin/junctin knockout fibers (Boncompagni et al., 2012), in Casq2-null skeletal fibers (Valle et al., 2016), and in MAP6 knockout muscle (Sebastien et al., 2018).
Almost a decade later the initial observation of stacks in Casq1-null fibers (Boncompagni et al., 2012), and after discovering the sites of SOCE (Boncompagni et al., 2017; 2018; Michelucci et al., 2019), we finally realized that the stacks of flat SR cisternae found in Casq1-null fibers were one of the two main elements forming CEUs, the intracellular junctions that mediate SOCE. The data collected over several years in two separate projects (1. characterization of Casq1-null mice phenotype; 2. identification of SOCE-sites) led us to hypothesize that SOCE activation, through the assembly of CEUs, could be another mechanism used by Casq1-null mice to compensate for the lack of Casq1 in the SR.
Compelling evidence published in Michelucci et al., 2020 in J. Gen. Physiol. revealed that EDL muscles from sedentary Casq1-null mice (e.g. not subjected to exercise) contained preassembled junctions between SR-stacks and TT extensions within the I band virtually identical to the CEUs formed in WT mice during acute exercise (Boncompagni et al., 2017; 2018; Michelucci et al., 2019). Quantitative analysis by EM showed that CEUs in Casq1-null fibers were fully assembled as both components were in place: i) higher percentage of fibers with SR-stacks and more SR-stacks per unit area, compared to control muscle; and ii) increased TT extension at the I band and more contacts with SR-stacks, compared to WT muscle. Pre-assembly of CEUs in muscle of Casq1-null mice is likely mediated by increased expression of Stim1S, Stim1L, Orai1, and SERCA. The final proofs that these pre-assembled CEUs were functional came from studies showing: a) increased rate of Mn2+ quench in FDB fibers of Casq1-null compared to WT, both following a protocol of SR depletion and without SR depletion; b) increased SR refilling during repetitive stimulation, which helped to normalize Ca2+ transients (abolished by removal of external Ca2+ or when Ca2+ entry was reduced by presence of SOCE inhibitors, e.g. 0 Ca2+ or BTP-2).
The findings reported by Michelucci and others demonstrated that the SR-TT junctions within the I band of Casq1-null mice represent pre-assembled CEUs that provide an active pathway for constitutive and store-operated Ca2+ influx (Michelucci et al., 2020). Constitutively active SOCE potentially serves as a surrogate Ca2+ pool and compensates for the marked reduction in total SR Ca2+ store capacity caused by the lack of Casq1 (Paolini et al., 2007; Canato et al., 2010). Pre-assembled CEUs may result from a long-term post-natal adaptation which helps to maintain proper muscle function during prolonged periods of activity.
Final remarks
We had known for several years that the total amount of releasable Ca2+ from intracellular SR stores was markedly reduced in Casq1-null fibers (Paolini et al., 2007). Though, the resistance to fatigue of fibers and mice was not significantly impaired. The increased mitochondrial number/volume (Paolini et al., 2007), which in turn was possibly induced by the need of additional ATP to remove excessive accumulation of Ca2+ in the cytoplasm, could have been one factor that helped Casq1-null muscles to sustain prolonged activity. Yet our hypothesis was not correct.
Casq1-null fibers were using external Ca2+ to compensate for the low amount of Ca2+ stored in the SR. Now also the severe SR depletion of Casq1-null fibers when subjected to high frequency stimulation reported in Canato et al., 2010 makes more sense, as SR depletion is the key signal that activates SOCE, hence assembly of CEUs. In the end, the presence of constitutively active CEUs in muscle of Casq1-null mice could alone explain how these mice are able to survive despite the absence of a protein once considered fundamental for EC coupling and for skeletal muscle function.
Conclusion
Generation of knockout mice was once a real challenge. However, year-by-year it has become easier and easier to generate knockout and knockin mice to study the function of specific proteins. Often so easy that - my personal feeling - animal models generated with modern and fast techniques, maybe at a lower cost, are often not really investigated in depth. It is more appealing to most scientists to generate a new model, than deepen the investigation on a model that has been already published once or twice.
Our experience with Casq1-null mice has been a long journey of almost 20 years now, that demonstrated to us how the structural and functional changes induced by the ablation of a single protein may be quite complex, and definitely not easy to unravel with a single - not even a few - publications. First, we described morphological and functional changes induced by ablation of Casq1 in adult mice: remodeling of CRUs with more Ca2+ release channels and reduced SR Ca2+ content, which caused fast depletion under high frequency stimulation (Paolini et al., 2007; Canato et al., 2010). Then, spontaneous mortality of male mice pushed us to investigate these deaths and allowed the discovery of susceptibility of mice to trigger hyperthermic episodes when exposed to halothane, heat, and even strenuous exercise (Dainese et al., 2009; Protasi et al., 2009; Michelucci et al., 2017c). During these investigations we were also able to deepen our understanding of the molecular bases of these disorders (Ca2+ leak, oxidative stress; mitochondrial damage; etc.) and verified the protective effect of antioxidants and estrogens (Michelucci et al., 2015; Paolini et al., 2015; Michelucci et al., 2017a; Guarnier et al., 2018).
Then the discovery of the sites of SOCE (i.e. CEUs) - in an apparently unrelated project - allowed us to revisit some structural modifications induced by ablation of Casq1 (presence of SR-stacks at the I band; Boncompagni et al., 2012) and understand how Casq1-null mice use continuously external Ca2+ to compensate for the reduced amount of Ca2+ in the SR stores (Michelucci et al., 2020).
In the end, it was quite an exciting journey that was worth traveling. What the future will bring is still unknown. But one idea is already in our mind: IVCT, the gold standard procedure to assess susceptibility to MH (test in which biopsies are exposed to increasing halothane of caffeine), requires the presence of extracellular Ca2+ to determine which patients are at risk of MH reactions (Fletcher et al., 1990). Hence, Ca2+ entry plays a role in contracture of biopsies and MH susceptibility. We have also recently showed that assembly of CEUs during exercise contributes to the increase in body temperature during exertional stress (Girolami et al., 2022). One puzzling question to answer in our future work would be: the constitutively active SOCE in Casq1-null fibers may contribute to the MH/HS susceptibility of these mice?
Author contributions
This is a review: each author (except the first author) wrote the first draft of a chapter. Then, FP collected the initial drafts and extensively edited the whole manuscript (and wrote cover letter). LP and CP assembled the Figures, while BG and MS collected the references. When the manuscript was complete, it was fully reviewed by each author. All authors contributed to revisions requested by the journal and approved the submitted version.
Funding
This research was funded by grants: (1) GGP19231 from the Italian Telethon ONLUS; and (2) subcontract of AR059646 to FP from the National Institutes of Health United States .
Conflict of interest
The authors declare that the research was conducted in the absence of any commercial or financial relationships that could be construed as a potential conflict of interest.
Publisher’s note
All claims expressed in this article are solely those of the authors and do not necessarily represent those of their affiliated organizations, or those of the publisher, the editors and the reviewers. Any product that may be evaluated in this article, or claim that may be made by its manufacturer, is not guaranteed or endorsed by the publisher.
Abbreviations
Casq, calsequestrin; CASQ, calsequestrin gene; CCD, Central Core Disease; CEU, Ca2+ entry unit; CPVT, catecholaminergic polymorphic ventricular tachycardia; CRU, Ca2+ release unit; DHPR, dihydropyridine receptor; EC coupling, excitation-contraction coupling; EDL, extensor digitorum longus; EM, electron microscopy; ER, endoplasmic reticulum; ES, exertional stress; FDB, flexor digitorum brevis; HS, heat stroke; IVCT, in vitro contracture test; mSOF, mitochondrial superoxide flashes; MHS, malignant hyperthermia susceptibility; NAC, N-acetylcysteine; PSS, porcine stress syndrome; ROS and NOS, oxidative species of oxygen and nitrogen; RyR, ryanodine receptor; SR, sarcoplasmic reticulum; SERCA, sarco-endoplasmic reticulum Ca2+ ATP-ase; SOCE, store-operated Ca2+ entry; TT, transverse tubule; WB, western blot; WT, wild type.
References
Arai M., Alpert N. R., Periasamy M. (1991). Cloning and characterization of the gene encoding rabbit cardiac calsequestrin. Gene 109 (2), 275–279. doi:10.1016/0378-1119(91)90621-h0378
Beard N. A., Casarotto M. G., Wei L., Varsanyi M., Laver D. R., Dulhunty A. F. (2005). Regulation of ryanodine receptors by calsequestrin: Effect of high luminal Ca2+ and phosphorylation. Biophys. J. 88 (5), 3444–3454. doi:10.1529/biophysj.104.051441
Beard N. A., Sakowska M. M., Dulhunty A. F., Laver D. R. (2002). Calsequestrin is an inhibitor of skeletal muscle ryanodine receptor calcium release channels. Biophys. J. 82 (1), 310–320. doi:10.1016/S0006-3495(02)75396-4
Block B. A., Imagawa T., Campbell K. P., Franzini-Armstrong C. (1988). Structural evidence for direct interaction between the molecular components of the transverse tubule/sarcoplasmic reticulum junction in skeletal muscle. J. Cell. Biol. 107 (2), 2587–2600. doi:10.1083/jcb.107.6.2587
Boncompagni S., Michelucci A., Pietrangelo L., Dirksen R. T., Protasi F. (2018). Addendum: Exercise-dependent formation of new junctions that promote STIM1-Orai1 assembly in skeletal muscle. Sci. Rep. 8 (1), 17463. doi:10.1038/s41598-018-33063-0
Boncompagni S., Michelucci A., Pietrangelo L., Dirksen R. T., Protasi F. (2017). Exercise dependent formation of new junctions that promote STIM1-Orai1 assembly in skeletal muscle. Sci. Rep. 7 (1), 14286. doi:10.1038/s41598-017-14134-0
Boncompagni S., Protasi F., Franzini-Armstrong C. (2012). Sequential stages in the age dependent gradual formation and accumulation of tubular aggregates in fast twitch muscle fibers: SERCA and calsequestrin involvement. Age (Dordr) 34 (1), 27–41. doi:10.1007/s11357-011-9211-y
Boncompagni S., Rossi A. E., Micaroni M., Beznoussenko G. V., Polishchuk R. S., Dirksen R. T., et al. (2009a). Mitochondria are linked to calcium stores in striated muscle by developmentally regulated tethering structures. Mol. Biol. Cell. 20 (3), 1058–1067. doi:10.1091/mbc.e08-07-0783
Boncompagni S., Rossi A. E., Micaroni M., Hamilton S. L., Dirksen R. T., Franzini-Armstrong C., et al. (2009b). Characterization and temporal development of cores in a mouse model of malignant hyperthermia. Proc. Natl. Acad. Sci. U. S. A. 106 (51), 2199621996–2199622001. doi:10.1073/pnas.0911496106
Boncompagni S., Thomas M., Lopez J. R., Allen P. D., Yuan Q., Kranias E. G., et al. (2012). Triadin/Junctin double null mouse reveals a differential role for Triadin and Junctin in anchoring CASQ to the jSR and regulating Ca(2+) homeostasis. PLoS One 7 (7), e39962. doi:10.1371/journal.pone.0039962
Borras C., Gambini J., Gomez-Cabrera M. C., Sastre J., Pallardo F. V., Mann G. E., et al. (2005). 17beta-oestradiol up-regulates longevity-related, antioxidant enzyme expression via the ERK1 and ERK2[MAPK]/NFkappaB cascade. Aging Cell. 4 (3), 113ACE151–118. doi:10.1111/j.1474-9726.2005.00151.x
Bouchama A., Knochel J. P. (2002). Heat stroke. N. Engl. J. Med. 346 (25), 1978–1988. doi:10.1056/NEJMra011089
Brandom B., Larach M. (2002). Reassessment of the safety and efficacy of dantrolene. Anesthesiology 96 (2), A1199. doi:10.1097/00000542-200209002-01199
Burkman J. M., Posner K. L., Domino K. B. (2007). Analysis of the clinical variables associated with recrudescence after malignant hyperthermia reactions. Anesthesiology 106 (5), 901–906. quiz 1077-1078. doi:10.1097/01.anes.0000265148.86566.68
Calderon J. C., Bolanos P., Caputo C. (2014). The excitation-contraction coupling mechanism in skeletal muscle. Biophys. Rev. 6 (1), 133–160. doi:10.1007/s12551-013-0135-x
Campbell K. P., MacLennan D. H., Jorgensen A. O., Mintzer M. C. (1983). Purification and characterization of calsequestrin from canine cardiac sarcoplasmic reticulum and identification of the 53, 000 Dalton glycoprotein. J. Biol. Chem. 258 (2), 1197–1204. doi:10.1016/s0021-9258(18)33178-8
Canato M., Scorzeto M., Giacomello M., Protasi F., Reggiani C., Stienen G. J. (2010). Massive alterations of sarcoplasmic reticulum free calcium in skeletal muscle fibers lacking calsequestrin revealed by a genetically encoded probe. Proc. Natl. Acad. Sci. U. S. A. 107 (51), 22326–22331. doi:10.1073/pnas.1009168108
Capacchione J. F., Sambuughin N., Bina S., Mulligan L. P., Lawson T. D., Muldoon S. M. (2010). Exertional rhabdomyolysis and malignant hyperthermia in a patient with ryanodine receptor type 1 gene, L-type calcium channel alpha-1 subunit gene, and calsequestrin-1 gene polymorphisms. Anesthesiology 112 (1), 239–244. doi:10.1097/ALN.0b013e3181c29504
Carrell E. M., Coppola A. R., McBride H. J., Dirksen R. T. (2016). Orai1 enhances muscle endurance by promoting fatigue-resistant type I fiber content but not through acute store-operated Ca2+ entry. FASEB J. 30 (12), 4109–4119. doi:10.1096/fj.201600621R
Chelu M. G., Goonasekera S. A., Durham W. J., Tang W., Lueck J. D., Riehl J., et al. (2006). Heat- and anesthesia-induced malignant hyperthermia in an RyR1 knock-in mouse. FASEB J. 20 (2), 329–330. doi:10.1096/fj.05-4497fje
Cheng G., Zielonka M., Dranka B., Kumar S. N., Myers C. R., Bennett B., et al. (2018). Detection of mitochondria-generated reactive oxygen species in cells using multiple probes and methods: Potentials, pitfalls, and the future. J. Biol. Chem. 293 (26), 10363–10380. doi:10.1074/jbc.RA118.003044
Cho J. H., Oh Y. S., Park K. W., Yu J., Choi K. Y., Shin J. Y., et al. (2000). Calsequestrin, a calcium sequestering protein localized at the sarcoplasmic reticulum, is not essential for body-wall muscle function in Caenorhabditis elegans. J. Cell. Sci. 113 (22), 3947–3958. doi:10.1242/jcs.113.22.3947
Dainese M., Quarta M., Lyfenko A. D., Paolini C., Canato M., Reggiani C., et al. (2009). Anesthetic- and heat-induced sudden death in calsequestrin-1-knockout mice. FASEB J. 23 (6), 1710–1720. doi:10.1096/fj.08-121335
Damiani E., Volpe P., Margreth A. (1990). Coexpression of two isoforms of calsequestrin in rabbit slow-twitch muscle. J. Muscle Res. Cell. Motil. 11 (6), 522–530. doi:10.1007/BF01745219
Davies K. J., Quintanilha A. T., Brooks G. A., Packer L. (1982). Free radicals and tissue damage produced by exercise. Biochem. Biophys. Res. Commun. 107 (4), 1198–1205. doi:10.1016/s0006-291x(82)80124-1
Davis M., Brown R., Dickson A., Horton H., James D., Laing N., et al. (2002). Malignant hyperthermia associated with exercise-induced rhabdomyolysis or congenital abnormalities and a novel RYR1 mutation in New Zealand and Australian pedigrees. Br. J. Anaesth. 88 (4), 508–515. doi:10.1093/bja/88.4.508
de Sousa C. V., Sales M. M., Rosa T. S., Lewis J. E., de Andrade R. V., Simoes H. G. (2017). The antioxidant effect of exercise: A systematic review and meta-analysis. Sports Med. 47 (2), 277–293. doi:10.1007/s40279-016-0566-1
De Stefani D., Raffaello A., Teardo E., Szabo I., Rizzuto R. (2011). A forty-kilodalton protein of the inner membrane is the mitochondrial calcium uniporter. Nature 476 (7360), 336nature10230–340. doi:10.1038/nature10230
Denborough M. A., Ebeling P., King J. O., Zapf P. (1970a). Myopathy and malignant hyperpyrexia. Lancet 1 (7657), 1138–1140. doi:10.1016/s0140-6736(70)91215-8
Denborough M. A., Forster J. F., Hudson M. C., Carter N. G., Zapf P. (1970b). Biochemical changes in malignant hyperpyrexia. Lancet 1 (7657), 1137–1138. doi:10.1016/s0140-6736(70)91214-6
Denborough M. A., Forster J. F., Lovell R. R., Maplestone P. A., Villiers J. D. (1962). Anaesthetic deaths in a family. Br. J. Anaesth. 34, 395–396. doi:10.1093/bja/34.6.395
Denborough M. A., Lovell R. R. H. (1960). Anæsthetic deaths in a family. Lancet 276 (7140), 45. doi:10.1016/S0140-6736(60)92690-8
Dirksen R. T. (2009). Checking your SOCCs and feet: The molecular mechanisms of Ca2+ entry in skeletal muscle. J. Physiol. 587 (13), 3139–3147. doi:10.1113/jphysiol.2009.172148
Dlamini N., Voermans N. C., Lillis S., Stewart K., Kamsteeg E. J., Drost G., et al. (2013). Mutations in RYR1 are a common cause of exertional myalgia and rhabdomyolysis. Neuromuscul. Disord. 23 (7), 540–548. doi:10.1016/j.nmd.2013.03.008
Dolmetsch R. (2003). Excitation-transcription coupling: Signaling by ion channels to the nucleus. Sci. STKE 2003 (166), PE4. doi:10.1126/stke.2003.166.pe4
Dulhunty A. F., Beard N. A., Pouliquin P., Kimura T. (2006). Novel regulators of RyR Ca2+ release channels: Insight into molecular changes in genetically-linked myopathies. J. Muscle Res. Cell. Motil. 27 (5-7), 351–365. doi:10.1007/s10974-006-9086-1
Dulhunty A., Wei L., Beard N. (2009). Junctin - the quiet achiever. J. Physiol. 587 (Pt 13), 3135–3137. doi:10.1113/jphysiol.2009.171959
Durham W. J., Aracena-Parks P., Long C., Rossi A. E., Goonasekera S. A., Boncompagni S., et al. (2008). RyR1 S-nitrosylation underlies environmental heat stroke and sudden death in Y522S RyR1 knockin mice. Cell. 133 (1), 53–65. doi:10.1016/j.cell.2008.02.042
Feske S., Gwack Y., Prakriya M., Srikanth S., Puppel S. H., Tanasa B., et al. (2006). A mutation in Orai1 causes immune deficiency by abrogating CRAC channel function. Nature 441 (7090), 179–185. doi:10.1038/nature04702
Fletcher J. E., Huggins F. J., Rosenberg H. (1990). The importance of calcium ions for in vitro malignant hyperthermia testing. Can. J. Anaesth. 37 (6), 695–698. doi:10.1007/BF03006495
Florian M., Freiman A., Magder S. (2004). Treatment with 17-beta-estradiol reduces superoxide production in aorta of ovariectomized rats. Steroids 69 (13-14), 779–787. doi:10.1016/j.steroids.2004.09.008
Franzini-Armstrong C. (2009). Architecture and regulation of the Ca2+ delivery system in muscle cells. Appl. Physiol. Nutr. Metab. 34 (3), 323h09–327.
Franzini-Armstrong C., Kenney L. J., Varriano-Marston E. (1987). The structure of calsequestrin in triads of vertebrate skeletal muscle: A deep-etch study. J. Cell. Biol. 105 (1), 49–56. doi:10.1083/jcb.105.1.49
Franzini-Armstrong C., Protasi F., Tijskens P. (2005). The assembly of calcium release units in cardiac muscle. Ann. N. Y. Acad. Sci. 1047, 76–85. doi:10.1196/annals.1341.007
Franzini-Armstrong C. (1970). Studies of the triad : I. Structure of the junction in frog twitch fibers. J. Cell. Biol. 47 (2), 488–499. doi:10.1083/jcb.47.2.488
Fryer M. W., Stephenson D. G. (1996). Total and sarcoplasmic reticulum calcium contents of skinned fibres from rat skeletal muscle. J. Physiol. 493 (2), 357–370. doi:10.1113/jphysiol.1996.sp021388
Fujii J., Otsu K., Zorzato F., de Leon S., Khanna V. K., Weiler J. E., et al. (1991). Identification of a mutation in porcine ryanodine receptor associated with malignant hyperthermia. Science 253 (5018), 448–451. doi:10.1126/science.1862346
Fujii J., Willard H. F., MacLennan D. H. (1990). Characterization and localization to human chromosome 1 of human fast-twitch skeletal muscle calsequestrin gene. Somat. Cell. Mol. Genet. 16 (2), 185–189. doi:10.1007/BF01233048
Galli L., Orrico A., Lorenzini S., Censini S., Falciani M., Covacci A., et al. (2006). Frequency and localization of mutations in the 106 exons of the RYR1 gene in 50 individuals with malignant hyperthermia. Hum. Mutat. 27 (8), 830. doi:10.1002/humu.9442
Garrido C., Galluzzi L., Brunet M., Puig P. E., Didelot C., Kroemer G. (2006). Mechanisms of cytochrome c release from mitochondria. Cell. Death Differ. 13 (9), 14231423–14231433. doi:10.1038/sj.cdd.4401950
Gerke V., Creutz C. E., Moss S. E. (2005). Annexins: Linking Ca2+ signalling to membrane dynamics. Nat. Rev. Mol. Cell. Biol. 6 (6), 449nrm1661–461. doi:10.1038/nrm1661
Gherardi G., Monticelli H., Rizzuto R., Mammucari C. (2020). The mitochondrial Ca(2+) uptake and the fine-tuning of aerobic metabolism. Front. Physiol. 11, 554904. doi:10.3389/fphys.2020.554904
Gilchrist J. S., Belcastro A. N., Katz S. (1992). Intraluminal Ca2+ dependence of Ca2+ and ryanodine-mediated regulation of skeletal muscle sarcoplasmic reticulum Ca2+ release. J. Biol. Chem. 267 (29), 20850–20856. doi:10.1016/s0021-9258(19)36766-3
Girolami B., Serano M., Michelucci A., Pietrangelo L., Protasi F. (2022). Store-operated Ca(2+) entry in skeletal muscle contributes to the increase in body temperature during exertional stress. Int. J. Mol. Sci. 23 (7), 3772. doi:10.3390/ijms23073772
Gissel H. (2005). The role of Ca2+ in muscle cell damage. Ann. N. Y. Acad. Sci. 1066, 166–180. doi:10.1196/annals.1363.013
Goldstein J. C., Waterhouse N. J., Juin P., Evan G. I., Green D. R. (2000). The coordinate release of cytochrome c during apoptosis is rapid, complete and kinetically invariant. Nat. Cell. Biol. 2 (3), 156–162. doi:10.1038/35004029
Gomez-Zubeldia M. A., Arbues J. J., Hinchado G., Nogales A. G., Millan J. C. (2001). Influence of estrogen replacement therapy on plasma lipid peroxidation. Menopause 8 (4), 274–280. doi:10.1097/00042192-200107000-00009
Green D. R., Llambi F. (2015). Cell death signaling. Cold Spring Harb. Perspect. Biol. 7 (12), a006080. doi:10.1101/cshperspect.a006080
Guarnier F. A., Michelucci A., Serano M., Pietrangelo L., Pecorai C., Boncompagni S., et al. (2018). Aerobic training prevents heatstrokes in calsequestrin-1 knockout mice by reducing oxidative stress. Oxid. Med. Cell. Longev. 2018, 4652480. doi:10.1155/2018/4652480
Herzog A., Szegedi C., Jona I., Herberg F. W., Varsanyi M. (2000). Surface plasmon resonance studies prove the interaction of skeletal muscle sarcoplasmic reticular Ca(2+) release channel/ryanodine receptor with calsequestrin. FEBS Lett. 472 (1), 73–77. doi:10.1016/s0014-5793(00)01431-9
Hopkins P. M., Ellis F. R., Halsall P. J. (1991). Evidence for related myopathies in exertional heat stroke and malignant hyperthermia. Lancet 338 (8781), 1491–1492. doi:10.1016/0140-6736(91)92304-k
Ikemoto N., Ronjat M., Meszaros L. G., Koshita M. (1989). Postulated role of calsequestrin in the regulation of calcium release from sarcoplasmic reticulum. Biochemistry 28 (16), 6764–6771. doi:10.1021/bi00442a033
Inesi G., de Meis L. (1989). Regulation of steady state filling in sarcoplasmic reticulum. J. Biol. Chem. 264 (10), 5929–5936. doi:10.1016/s0021-9258(18)83639-0
Jones E. W., Nelson T. E., Anderson I. L., Kerr D. D., Burnap T. K. (1972). Malignant hyperthermia of swine. Anesthesiology 36 (1), 42–51. doi:10.1097/00000542-197201000-00008
Jones L. R., Suzuki Y. J., Wang W., Kobayashi Y. M., Ramesh V., Franzini-Armstrong C., et al. (1998). Regulation of Ca2+ signaling in transgenic mouse cardiac myocytes overexpressing calsequestrin. J. Clin. Investig. 101 (7), 1385–1393. doi:10.1172/JCI1362
Jouaville L. S., Pinton P., Bastianutto C., Rutter G. A., Rizzuto R. (1999). Regulation of mitochondrial ATP synthesis by calcium: Evidence for a long-term metabolic priming. Proc. Natl. Acad. Sci. U. S. A. 96 (24), 13807–13812. doi:10.1073/pnas.96.24.13807
Kawasaki T., Kasai M. (1994). Regulation of calcium channel in sarcoplasmic reticulum by calsequestrin. Biochem. Biophys. Res. Commun. 199 (3), 1120–1127. doi:10.1006/bbrc.1994.1347-7
Knollmann B. C., Chopra N., Hlaing T., Akin B., Yang T., Ettensohn K., et al. (2006). Casq2 deletion causes sarcoplasmic reticulum volume increase, premature Ca2+ release, and catecholaminergic polymorphic ventricular tachycardia. J. Clin. Investig. 116 (9), 2510–2520. doi:10.1172/JCI29128
Knudson C. M., Chaudhari N., Sharp A. H., Powell J. A., Beam K. G., Campbell K. P. (1989). Specific absence of the alpha 1 subunit of the dihydropyridine receptor in mice with muscular dysgenesis. J. Biol. Chem. 264 (3), 1345–1348. doi:10.1016/s0021-9258(18)94191-8
Ko J. K., Choi K. H., Zhao X., Komazaki S., Pan Z., Weisleder N., et al. (2011). A versatile single-plasmid system for tissue-specific and inducible control of gene expression in transgenic mice. FASEB J. 25 (8), 2638–2649. doi:10.1096/fj.11-181560
Kobayashi Y. M., Alseikhan B. A., Jones L. R. (2000). Localization and characterization of the calsequestrin-binding domain of triadin 1. Evidence for a charged beta-strand in mediating the protein-protein interaction. J. Biol. Chem. 275 (23), 17639–17646. doi:10.1074/jbc.M002091200
Lai F. A., Erickson H. P., Rousseau E., Liu Q. Y., Meissner G. (1988). Purification and reconstitution of the calcium release channel from skeletal muscle. Nature 331 (6154), 315–319. doi:10.1038/331315a0
Lamb G. D. (2002). Excitation-contraction coupling and fatigue mechanisms in skeletal muscle: Studies with mechanically skinned fibres. J. Muscle Res. Cell. Motil. 23 (1), 81–91. doi:10.1023/a:1019932730457
Larach M. G., Rosenberg H., Gronert G. A., Allen G. C. (2001). Did anesthetics trigger cardiac arrests in patients with occult myopathies? Anesthesiology 94 (5), 933–935. doi:10.1097/00000542-200105000-00039
Launikonis B. S., Barnes M., Stephenson D. G. (2003). Identification of the coupling between skeletal muscle store-operated Ca2+ entry and the inositol trisphosphate receptor. Proc. Natl. Acad. Sci. U. S. A. 100 (5), 2941–2944. doi:10.1073/pnas.0536227100
Launikonis B. S., Rios E. (2007). Store-operated Ca2+ entry during intracellular Ca2+ release in mammalian skeletal muscle. J. Physiol. 583 (1), 81–97. doi:10.1113/jphysiol.2007.135046
Lehmann-Horn F., Klingler W., Jurkat-Rott K. (2011). Nonanesthetic malignant hyperthermia. Anesthesiology 115 (5), 915–917. doi:10.1097/ALN.0b013e318232008f
Li L., Mirza S., Richardson S. J., Gallant E. M., Thekkedam C., Pace S. M., et al. (2015). A new cytoplasmic interaction between junctin and ryanodine receptor Ca2+ release channels. J. Cell. Sci. 128 (5), 951–963. doi:10.1242/jcs.160689
Liang H., Ward W. F. (2006). PGC-1alpha: A key regulator of energy metabolism. Adv. Physiol. Educ. 30 (4), 145–151. doi:10.1152/advan.00052.2006
Lin J., Handschin C., Spiegelman B. M. (2005). Metabolic control through the PGC-1 family of transcription coactivators. Cell. Metab. 1 (6), 361–370. doi:10.1016/j.cmet.2005.05.004
Liou J., Kim M. L., Heo W. D., Jones J. T., Myers J. W., Ferrell J. E., et al. (2005). STIM is a Ca2+ sensor essential for Ca2+-store-depletion-triggered Ca2+ influx. Curr. Biol. 15 (13), 1235–1241. doi:10.1016/j.cub.2005.05.055
Liu X., Kim C. N., Yang J., Jemmerson R., Wang X. (1996). Induction of apoptotic program in cell-free extracts: Requirement for dATP and cytochrome c. Cell. 86 (1), 147–157. doi:10.1016/s0092-8674(00)80085-9
MacLennan D. H., de Leon S. (1983). Biosynthesis of sarcoplasmic reticulum proteins. Methods Enzymol. 96, 570–579. doi:10.1016/s0076-6879(83)96049-4
MacLennan D. H., Wong P. T. (1971). Isolation of a calcium-sequestering protein from sarcoplasmic reticulum. Proc. Natl. Acad. Sci. U. S. A. 68 (6), 1231–1235. doi:10.1073/pnas.68.6.1231
Makinose M., Hasselbach W. (1965). The influence of oxalate on calcium transport of isolated sarcoplasmic reticular vesicles. Biochem. Z. 343 (4), 360–382.
McCord J. M., Fridovich I. (1969). The utility of superoxide dismutase in studying free radical reactions. J. Biol. Chem. 244 (22), 6056–6063. doi:10.1016/s0021-9258(18)63505-7
McCullough L. D., Alkayed N. J., Traystman R. J., Williams M. J., Hurn P. D. (2001). Postischemic estrogen reduces hypoperfusion and secondary ischemia after experimental stroke. Stroke 32 (3), 796–802. doi:10.1161/01.STR.32.3.796
Meissner G. (1975). Isolation and characterization of two types of sarcoplasmic reticulum vesicles. Biochim. Biophys. Acta 389 (1), 51–68. doi:10.1016/0005-2736(75)90385-5
Michelucci A., Boncompagni S., Canato M., Reggiani C., Protasi F. (2017a). Estrogens protect calsequestrin-1 knockout mice from lethal hyperthermic episodes by reducing oxidative stress in muscle. Oxid. Med. Cell. Longev. 2017, 6936897. doi:10.1155/2017/6936897
Michelucci A., Boncompagni S., Pietrangelo L., Garcia-Castaneda M., Takano T., Malik S., et al. (2019). Transverse tubule remodeling enhances Orai1-dependent Ca(2+) entry in skeletal muscle. Elife 8, e47576. doi:10.7554/eLife.47576
Michelucci A., Boncompagni S., Pietrangelo L., Takano T., Protasi F., Dirksen R. T. (2020). Pre-assembled Ca2+ entry units and constitutively active Ca2+ entry in skeletal muscle of calsequestrin-1 knockout mice. J. Gen. Physiol. 152 (10), e202012617. doi:10.1085/jgp.202012617)
Michelucci A., De Marco A., Guarnier F. A., Protasi F., Boncompagni S. (2017b2017). Antioxidant treatment reduces formation of structural cores and improves muscle function in RYR1(Y522S/WT) mice. Oxid. Med. Cell. Longev., 6792694. doi:10.1155/2017/6792694
Michelucci A., Garcia-Castaneda M., Boncompagni S., Dirksen R. T. (2018S0143). Role of STIM1/ORAI1-mediated store-operated Ca(2+) entry in skeletal muscle physiology and disease. Cell. Calcium 76, 101–115. doi:10.1016/j.ceca.2018.10.004
Michelucci A., Paolini C., Boncompagni S., Canato M., Reggiani C., Protasi F. (2017c). Strenuous exercise triggers a life-threatening response in mice susceptible to malignant hyperthermia. FASEB J. 31 (8), 3649–3662. doi:10.1096/fj.201601292R
Michelucci A., Paolini C., Canato M., Wei-Lapierre L., Pietrangelo L., De Marco A., et al. (2015). Antioxidants protect calsequestrin-1 knockout mice from halothane- and heat-induced sudden death. Anesthesiology 123 (3), 603–617. doi:10.1097/ALN.0000000000000748
Migita T., Mukaida K., Kawamoto M., Kobayashi M., Yuge O. (2007). Fulminant-type malignant hyperthermia in Japan: Cumulative analysis of 383 cases. J. Anesth. 21 (2), 285–288. doi:10.1007/s00540-006-0495-5
Mooradian A. D. (1993). Antioxidant properties of steroids. J. Steroid Biochem. Mol. Biol. 45 (6), 509–511. doi:10.1016/0960-0760(93)90166-t
Munoz-Pinedo C., Guio-Carrion A., Goldstein J. C., Fitzgerald P., Newmeyer D. D., Green D. R. (2006). Different mitochondrial intermembrane space proteins are released during apoptosis in a manner that is coordinately initiated but can vary in duration. Proc. Natl. Acad. Sci. U. S. A. 103 (31), 11573–11578. doi:10.1073/pnas.0603007103
Nelson T. E., Jones E. W., Venable J. H., Kerr D. D. (1972). Malignant hyperthermia of Poland China swine: Studies of a myogenic etiology. Anesthesiology 36 (1), 52–56. doi:10.1097/00000542-197201000-00009
Ogino K., Wang D. H. (2007). Biomarkers of oxidative/nitrosative stress: An approach to disease prevention. Acta Med. Okayama 61 (4), 181–189. doi:10.18926/AMO/32871
Ohkura M., Ide T., Furukawa K., Kawasaki T., Kasai M., Ohizumi Y. (1995). Calsequestrin is essential for the Ca2+ release induced by myotoxin alpha in skeletal muscle sarcoplasmic reticulum. Can. J. Physiol. Pharmacol. 73 (8), 1181–1185. doi:10.1139/y95-167
Ording H., Hald A., Sjontoft E. (1985). Malignant hyperthermia triggered by heating in anaesthetized pigs. Acta Anaesthesiol. Scand. 29 (7), 698–701. doi:10.1111/j.1399-6576.1985.tb02283.x
Pamukcoglu T. (1988). Sudden death due to malignant hyperthermia. Am. J. Forensic Med. Pathol. 9 (2), 161–162. doi:10.1097/00000433-198806000-00015
Paolini C., Protasi F., Franzini-Armstrong C. (2004). The relative position of RyR feet and DHPR tetrads in skeletal muscle. J. Mol. Biol. 342 (1), 145–153. doi:10.1016/j.jmb.2004.07.035
Paolini C., Quarta M., D'Onofrio L., Reggiani C., Protasi F. (2011). Differential effect of calsequestrin ablation on structure and function of fast and slow skeletal muscle fibers. J. Biomed. Biotechnol. 2011, 634075. doi:10.1155/2011/634075
Paolini C., Quarta M., Nori A., Boncompagni S., Canato M., Volpe P., et al. (2007). Reorganized stores and impaired calcium handling in skeletal muscle of mice lacking calsequestrin-1. J. Physiol. 583 (2), 767–784. doi:10.1113/jphysiol.2007.138024
Paolini C., Quarta M., Wei-LaPierre L., Michelucci A., Nori A., Reggiani C., et al. (2015). Oxidative stress, mitochondrial damage, and cores in muscle from calsequestrin-1 knockout mice. Skelet. Muscle 5, 10. doi:10.1186/s13395-015-0035-9
Prakriya M., Feske S., Gwack Y., Srikanth S., Rao A., Hogan P. G. (2006). Orai1 is an essential pore subunit of the CRAC channel. Nature 443 (7108), 230nature05122–233. doi:10.1038/nature05122
Protasi F., Franzini-Armstrong C., Allen P. D. (1998). Role of ryanodine receptors in the assembly of calcium release units in skeletal muscle. J. Cell. Biol. 140 (4), 831–842. doi:10.1083/jcb.140.4.831
Protasi F., Franzini-Armstrong C., Flucher B. E. (1997). Coordinated incorporation of skeletal muscle dihydropyridine receptors and ryanodine receptors in peripheral couplings of BC3H1 cells. J. Cell. Biol. 137 (4), 859–870. doi:10.1083/jcb.137.4.859
Protasi F., Paolini C., Dainese M. (2009). Calsequestrin-1: A new candidate gene for malignant hyperthermia and exertional/environmental heat stroke. J. Physiol. 587 (13), 3095–3100. doi:10.1113/jphysiol.2009.171967
Protasi F., Paolini C., Nakai J., Beam K. G., Franzini-Armstrong C., Allen P. D. (2002). Multiple regions of RyR1 mediate functional and structural interactions with alpha(1S)-dihydropyridine receptors in skeletal muscle. Biophys. J. 83 (6), 3230–3244. doi:10.1016/S0006-3495(02)75325-3
Protasi F., Pietrangelo L., Boncompagni S. (2021a). Calcium entry units (CEUs): Perspectives in skeletal muscle function and disease. J. Muscle Res. Cell. Motil. 42 (2), 233–249. doi:10.1007/s10974-020-09586-3
Protasi F., Pietrangelo L., Boncompagni S. (2021b). Improper remodeling of organelles deputed to Ca(2+) handling and aerobic ATP production underlies muscle dysfunction in ageing. Int. J. Mol. Sci. 22 (12), 6195. doi:10.3390/ijms22126195
Protasi F., Sun X. H., Franzini-Armstrong C. (1996). Formation and maturation of the calcium release apparatus in developing and adult avian myocardium. Dev. Biol. 173 (1), 265–278. doi:10.1006/dbio.1996.0022
Protasi F., Takekura H., Wang Y., Chen S. R., Meissner G., Allen P. D., et al. (2000). RYR1 and RYR3 have different roles in the assembly of calcium release units of skeletal muscle. Biophys. J. 79 (5), 2494–2508. doi:10.1016/S0006-3495(00)76491-5
Qiu R., Lewis R. S. (2019). Structural features of STIM and Orai underlying store-operated calcium entry. Curr. Opin. Cell. Biol. 57, 90–98. doi:10.1016/j.ceb.2018.12.012
Rios E., Ma J. J., Gonzalez A. (1991). The mechanical hypothesis of excitation-contraction (EC) coupling in skeletal muscle. J. Muscle Res. Cell. Motil. 12 (2), 127–135. doi:10.1007/BF01774031
Roos J., DiGregorio P. J., Yeromin A. V., Ohlsen K., Lioudyno M., Zhang S., et al. (2005). STIM1, an essential and conserved component of store-operated Ca2+ channel function. J. Cell. Biol. 169 (3), 435–445. doi:10.1083/jcb.200502019
Rossi A. E., Boncompagni S., Dirksen R. T. (2009). Sarcoplasmic reticulum-mitochondrial symbiosis: Bidirectional signaling in skeletal muscle. Exerc. Sport Sci. Rev. 37 (1), 29–35. doi:10.1097/JES.0b013e3181911fa4
Rossi A. E., Boncompagni S., Wei L., Protasi F., Dirksen R. T. (2011). Differential impact of mitochondrial positioning on mitochondrial Ca(2+) uptake and Ca(2+) spark suppression in skeletal muscle. Am. J. Physiol. Cell. Physiol. 301 (5), C1128–C1139. doi:10.1152/ajpcell.00194.2011
Royer L., Sztretye M., Manno C., Pouvreau S., Zhou J., Knollmann B. C., et al. (2010). Paradoxical buffering of calcium by calsequestrin demonstrated for the calcium store of skeletal muscle. J. Gen. Physiol. 136 (3), 325–338. doi:10.1085/jgp.201010454
Rudolf R., Mongillo M., Magalhaes P. J., Pozzan T. (2004). In vivo monitoring of Ca(2+) uptake into mitochondria of mouse skeletal muscle during contraction. J. Cell. Biol. 166 (4), 527–536. doi:10.1083/jcb.200403102
Ryan J. F., Tedeschi L. G. (1997). Sudden unexplained death in a patient with a family history of malignant hyperthermia. J. Clin. Anesth. 9 (1), 66–68. doi:10.1016/S0952-8180(96)00207-3
Sacchetto R., Volpe P., Damiani E., Margreth A. (1993). Postnatal development of rabbit fast-twitch skeletal muscle: Accumulation, isoform transition and fibre distribution of calsequestrin. J. Muscle Res. Cell. Motil. 14 (6), 646–653. doi:10.1007/BF00141561
Saito A., Seiler S., Chu A., Fleischer S. (1984). Preparation and morphology of sarcoplasmic reticulum terminal cisternae from rabbit skeletal muscle. J. Cell. Biol. 99 (3), 875–885. doi:10.1083/jcb.99.3.875
Sandow A., Taylor S. R., Preiser H. (1965). Role of the action potential in excitation-contraction coupling. Fed. Proc. 24 (5), 1116–1123.
Schneider M. F., Chandler W. K., Scorzeto M., Giacomello M., Toniolo L., Canato M., et al. (1973). Voltage dependent charge movement of skeletal muscle: A possible step in excitation-contraction couplingMitochondrial Ca2+-handling in fast skeletal muscle fibers from wild type and calsequestrin-null mice. NaturePLoS One 2428 (539510), 244e74919–246. doi:10.1371/journal.pone.0074919
Schneider M. F. (1994). Control of calcium release in functioning skeletal muscle fibers. Annu. Rev. Physiol. 56, 463–484. doi:10.1146/annurev.ph.56.030194.002335
Scorzeto M., Giacomello M., Toniolo L., Canato M., Blaauw B., Paolini C., et al. (2013). Mitochondrial Ca2+-handling in fast skeletal muscle fibers from wild type and calsequestrin-null mice. PLoS One 8 (10), e74919. doi:10.1371/journal.pone.0074919
Scott B. T., Simmerman H. K., Collins J. H., Nadal-Ginard B., Jones L. R. (1988). Complete amino acid sequence of canine cardiac calsequestrin deduced by cDNA cloning. J. Biol. Chem. 263 (18), 8958–8964. doi:10.1016/s0021-9258(18)68401-7
Sebastien M., Giannesini B., Aubin P., Brocard J., Chivet M., Pietrangelo L., et al. (2018). Deletion of the microtubule-associated protein 6 (MAP6) results in skeletal muscle dysfunction. Skelet. Muscle 8 (1), 30. doi:10.1186/s13395-018-0176-8
Serano M., Pietrangelo L., Paolini C., Guarnier F. A., Protasi F. (2022). Oxygen consumption and basal metabolic rate as markers of susceptibility to malignant hyperthermia and heat stroke. Cells 11 (16), 2468. doi:10.3390/cells11162468)
Shen K. H., Lin C. H., Chang H. K., Chen W. C., Chen S. H. (2008). Premarin can act via estrogen receptors to rescue mice from heatstroke-induced lethality. Shock 30 (6), 668–674. doi:10.1097/SHK.0b013e31817538cb
Shin D. W., Ma J., Kim D. H. (2000). The asp-rich region at the carboxyl-terminus of calsequestrin binds to Ca(2+) and interacts with triadin. FEBS Lett. 486 (2), 178–8.
Sidman R. D., Gallagher E. J. (1995). Exertional heat stroke in a young woman: Gender differences in response to thermal stress. Acad. Emerg. Med. 2 (4), 315–319. doi:10.1111/j.1553-2712.1995.tb03229.x
Strazis K. P., Fox A. W. (1993). Malignant hyperthermia: A review of published cases. Anesth. Analg. 77 (2), 297–304. doi:10.1213/00000539-199308000-00014
Sugioka K., Shimosegawa Y., Nakano M. (1987). Estrogens as natural antioxidants of membrane phospholipid peroxidation. FEBS Lett. 210 (1), 37–39. doi:10.1016/0014-5793(87)81293-0
Sun X. H., Protasi F., Takahashi M., Takeshima H., Ferguson D. G., Franzini-Armstrong C. (1995). Molecular architecture of membranes involved in excitation-contraction coupling of cardiac muscle. J. Cell. Biol. 129 (3), 659–671. doi:10.1083/jcb.129.3.659
Szegedi C., Sarkozi S., Herzog A., Jona I., Varsanyi M. (1999). Calsequestrin: More than 'only' a luminal Ca2+ buffer inside the sarcoplasmic reticulum. Biochem. J. 337 (1), 19–22. doi:10.1042/bj3370019
Takekura H., Nishi M., Noda T., Takeshima H., Franzini-Armstrong C. (1995). Abnormal junctions between surface membrane and sarcoplasmic reticulum in skeletal muscle with a mutation targeted to the ryanodine receptor. Proc. Natl. Acad. Sci. U. S. A. 92 (8), 3381–3385. doi:10.1073/pnas.92.8.3381
The European Malignant Hyperpyrexia Group (1984). A protocol for the investigation of malignant hyperpyrexia (MH) susceptibility. Br. J. Anaesth. 56 (1117), 1267–1269.
Tijskens P., Jones L. R., Franzini-Armstrong C. (2003). Junctin and calsequestrin overexpression in cardiac muscle: The role of junctin and the synthetic and delivery pathways for the two proteins. J. Mol. Cell. Cardiol. 35 (8), 961S0022282803001810–974. doi:10.1016/s0022-2828(03)00181-0
Tobin J. R., Jason D. R., Challa V. R., Nelson T. E., Sambuughin N. (2001). Malignant hyperthermia and apparent heat stroke. JAMA 286 (2), 168jlt0711–1694. doi:10.1001/jama.286.2.168
Tomasi M., Canato M., Paolini C., Dainese M., Reggiani C., Volpe P., et al. (2012). Calsequestrin (CASQ1) rescues function and structure of calcium release units in skeletal muscles of CASQ1-null mice. Am. J. Physiol. Cell. Physiol. 302 (3), C575–C586. doi:10.1152/ajpcell.00119.2011
Valle G., Vergani B., Sacchetto R., Reggiani C., De Rosa E., Maccatrozzo L., et al. (2016). Characterization of fast-twitch and slow-twitch skeletal muscles of calsequestrin 2 (CASQ2)-knock out mice: Unexpected adaptive changes of fast-twitch muscles only. J. Muscle Res. Cell. Motil. 37 (6), 225–233. doi:10.1007/s10974-016-9463-3
Venditti P., Di Meo S. (1997). Effect of training on antioxidant capacity, tissue damage, and endurance of adult male rats. Int. J. Sports Med. 18 (7), 497–502. doi:10.1055/s-2007-972671
Vig M., Peinelt C., Beck A., Koomoa D. L., Rabah D., Koblan-Huberson M., et al. (2006). CRACM1 is a plasma membrane protein essential for store-operated Ca2+ entry. Science 312 (5777), 1220–1223. doi:10.1126/science.1127883
Wagner E. J., Ronnekleiv O. K., Bosch M. A., Kelly M. J. (2001). Estrogen biphasically modifies hypothalamic GABAergic function concomitantly with negative and positive control of luteinizing hormone release. J. Neurosci. 21 (6), 2085–2093. doi:10.1523/jneurosci.21-06-02085.2001
Wang L., Zhang L., Li S., Zheng Y., Yan X., Chen M., et al. (2015). Retrograde regulation of STIM1-Orai1 interaction and store-operated Ca2+ entry by calsequestrin. Sci. Rep. 5, srep11349, 11349. doi:10.1038/srep11349
Wang S., Trumble W. R., Liao H., Wesson C. R., Dunker A. K., Kang C. H. (1998). Crystal structure of calsequestrin from rabbit skeletal muscle sarcoplasmic reticulum. Nat. Struct. Biol. 5 (6), 476–483. doi:10.1038/nsb0698-476
Wang W., Fang H., Groom L., Cheng A., Zhang W., Liu J., et al. (2008). Superoxide flashes in single mitochondria. Cell. 134 (2), 279–290. doi:10.1016/j.cell.2008.06.017
Wappler F., Fiege M., Steinfath M., Agarwal K., Scholz J., Singh S., et al. (2001). Evidence for susceptibility to malignant hyperthermia in patients with exercise-induced rhabdomyolysis. Anesthesiology 94 (1), 95–100. doi:10.1097/00000542-200101000-00019
Weber A. (1966). Energized calcium transport and relaxing factors. Curr. Top. Bioenergetics 1, 203–254. doi:10.1016/b978-1-4831-9969-6.50012-3
Wedel D. J., Quinlan J. G., Iaizzo P. A. (1995). Clinical effects of intravenously administered dantrolene. Mayo Clin. Proc. 70 (3), 241–246. doi:10.4065/70.3.241
Wei L., Gallant E. M., Dulhunty A. F., Beard N. A. (2009). Junctin and triadin each activate skeletal ryanodine receptors but junctin alone mediates functional interactions with calsequestrin. Int. J. Biochem. Cell. Biol. 41 (11), 2214–2224. doi:10.1016/j.biocel.2009.04.017
Wei L., Salahura G., Boncompagni S., Kasischke K. A., Protasi F., Sheu S. S., et al. (2011). Mitochondrial superoxide flashes: Metabolic biomarkers of skeletal muscle activity and disease. FASEB J. 25 (9), 3068–3078. doi:10.1096/fj.11-187252
Wei-Lapierre L., Carrell E. M., Boncompagni S., Protasi F., Dirksen R. T. (2013). Orai1-dependent calcium entry promotes skeletal muscle growth and limits fatigue. Nat. Commun. 4, 2805ncomms3805. doi:10.1038/ncomms3805
Wilson A. C., Meethal S. V., Bowen R. L., Atwood C. S. (2007). Leuprolide acetate: A drug of diverse clinical applications. Expert Opin. Investig. Drugs 16 (11), 1851–1863. doi:10.1517/13543784.16.11.1851
Wium E., Dulhunty A. F., Beard N. A. (2012). A skeletal muscle ryanodine receptor interaction domain in triadin. PLoS One 7 (8), e43817. doi:10.1371/journal.pone.0043817
Wu S., Ibarra M. C., Malicdan M. C., Murayama K., Ichihara Y., Kikuchi H., et al. (2006). Central core disease is due to RYR1 mutations in more than 90% of patients. Brain 129 (6), 1470awl077–1480. doi:10.1093/brain/awl077
Wyndham C. (1977). Heat stroke and hyperthermia in marathon run-ners. Ann. N. Y. Acad. Sci. 301, 128–138. doi:10.1111/j.1749-6632.1977.tb38192.x
Yang T., Riehl J., Esteve E., Matthaei K. I., Goth S., Allen P. D., et al. (2006). Pharmacologic and functional characterization of malignant hyperthermia in the R163C RyR1 knock-in mouse. Anesthesiology 105 (6), 1164–1175. doi:10.1097/00000542-200612000-00016
Yarotskyy V., Protasi F., Dirksen R. T. (2013). Accelerated activation of SOCE current in myotubes from two mouse models of anesthetic- and heat-induced sudden death. PLoS One 8 (10), e77633. doi:10.1371/journal.pone.0077633
Yeromin A. V., Zhang S. L., Jiang W., Yu Y., Safrina O., Cahalan M. D. (2006). Molecular identification of the CRAC channel by altered ion selectivity in a mutant of Orai. Nature 443 (7108), 226–229. doi:10.1038/nature05108
Zarain-Herzberg A., Fliegel L., MacLennan D. H. (1988). Structure of the rabbit fast-twitch skeletal muscle calsequestrin gene. J. Biol. Chem. 263 (10), 4807–4812. doi:10.1016/s0021-9258(18)68857-x
Zhang L., Wang L., Li S., Xue J., Luo D. (2016). Calsequestrin-1 regulates store-operated Ca2+ entry by inhibiting STIM1 aggregation. Cell. Physiol. biochem. 38 (6), 21832183–21832193. doi:10.1159/000445574
Zhang S. L., Yu Y., Roos J., Kozak J. A., Deerinck T. J., Ellisman M. H., et al. (2005n0414). STIM1 is a Ca2+ sensor that activates CRAC channels and migrates from the Ca2+ store to the plasma membrane. Nature 437 (7060), 902–905. doi:10.1038/nature04147
Zou H., Henzel W. J., Liu X., Lutschg A., Wang X. (1997). Apaf-1, a human protein homologous to C. elegans CED-4, participates in cytochrome c-dependent activation of caspase-3. Cell. 90 (3), 405–413. doi:10.1016/s0092-8674(00)80501-2
Zvaritch E., Kraeva N., Bombardier E., McCloy R. A., Depreux F., Holmyard D., et al. (2009). Ca2+ dysregulation in Ryr1(I4895T/wt) mice causes congenital myopathy with progressive formation of minicores, cores, and nemaline rods. Proc. Natl. Acad. Sci. U. S. A. 106 (51), 21813–21818. doi:10.1073/pnas.0912126106
Keywords: Ca2+ entry unit (CEU), calsequestrin (Casq), excitation-contraction (EC) coupling, store-operated Ca2+ entry (SOCE), malignant hyperthermia susceptibility (MHS), ryanodine receptor (RyR)
Citation: Protasi F, Girolami B, Serano M, Pietrangelo L and Paolini C (2022) Ablation of Calsequestrin-1, Ca2+ unbalance, and susceptibility to heat stroke. Front. Physiol. 13:1033300. doi: 10.3389/fphys.2022.1033300
Received: 31 August 2022; Accepted: 20 September 2022;
Published: 12 October 2022.
Edited by:
Enrique Jaimovich, University of Chile, ChileReviewed by:
Angela Fay Dulhunty, Australian National University, AustraliaNagomi Kurebayashi, Juntendo University, Japan
Copyright © 2022 Protasi, Girolami, Serano, Pietrangelo and Paolini. This is an open-access article distributed under the terms of the Creative Commons Attribution License (CC BY). The use, distribution or reproduction in other forums is permitted, provided the original author(s) and the copyright owner(s) are credited and that the original publication in this journal is cited, in accordance with accepted academic practice. No use, distribution or reproduction is permitted which does not comply with these terms.
*Correspondence: Feliciano Protasi, ZmVsaWNpYW5vLnByb3Rhc2lAdW5pY2guaXQ=
†These authors have contributed equally to this work and share senior authorship