- 1McNeish Laboratory, School of Chemistry, Food and Pharmacy, Department of Pharmacology, University of Reading, London, United Kingdom
- 2Tinker Laboratory, William Harvey Research Institute, Clinical Pharmacology and Precision Medicine, Queen Mary University, London, United Kingdom
There is strong evidence that the omega-3 polyunsaturated fatty acids (n-3 PUFAs) docosahexaenoic acid (DHA) and eicosapentaenoic acid (EPA) have cardioprotective effects. n-3 PUFAs cause vasodilation in hypertensive patients, in part controlled by increased membrane conductance to potassium. As KATP channels play a major role in vascular tone regulation and are involved in hypertension, we aimed to verify whether n-3 PUFA-mediated vasodilation involved the opening of KATP channels. We used a murine model in which the KATP channel pore subunit, Kir6.1, is deleted in vascular smooth muscle. The vasomotor response of preconstricted arteries to physiologically relevant concentrations of DHA and EPA was measured using wire myography, using the channel blocker PNU-37883A. The effect of n-3 PUFAs on potassium currents in wild-type native smooth muscle cells was investigated using whole-cell patch clamping. DHA and EPA induced vasodilation in mouse aorta and mesenteric arteries; relaxations in the aorta were sensitive to KATP blockade with PNU-37883A. Endothelium removal didn’t affect relaxation to EPA and caused a small but significant inhibition of relaxation to DHA. In the knock-out model, relaxations to DHA and EPA were unaffected by channel knockdown but were still inhibited by PNU-37883A, indicating that the action of PNU-37883A on relaxation may not reflect inhibition of KATP. In native aortic smooth muscle cells DHA failed to activate KATP currents. We conclude that DHA and EPA cause vasodilation in mouse aorta and mesenteric arteries. Relaxations in blocker-treated arteries from knock-out mice demonstrate that KATP channels are not involved in the n-3 PUFA-induced relaxation.
1 Introduction
The cardioprotective effects of marine-derived omega-3 polyunsaturated fatty acids (n-3 PUFAs) first drew attention when epidemiological studies found a lower incidence of cardiovascular diseases in regions of the world with a high consumption of fish (Bang et al., 1980; Kagawa et al., 1982). Since then, numerous studies have confirmed that n-3 PUFAs have vasodilatory effects in hypertensive humans, as reviewed in (Miller et al., 2014; Colussi et al., 2017; AbuMweis et al., 2018), and in preclinical models of hypertension, as reviewed in (Mozaffarian and Wu, 2011; Bercea et al., 2021). Two of the most studied n-3 PUFAs involved in vasodilation are eicosapentaenoic acid (EPA) and docosahexaenoic acid (DHA); these are constituents of the lipid component of cell membranes, and are known to modulate the activity of ion channels, such as K+ channels (Hamilton and Kamp, 1999).
Hypertension is associated with endothelium and vascular smooth muscle cell (VSMC) dysfunction, reviewed in (Touyz et al., 2018). However, in blood vessels isolated from animals, the endothelium appears to play only a limited role in the short-term effects of DHA and EPA on vascular tension. For example, in rat aorta and mesenteric arteries, DHA and EPA-induced relaxation is only slightly reduced after endothelium removal (Engler and Engler, 2000; Engler et al., 2000; Sato et al., 2013; Sato et al., 2014; Limbu et al., 2018), and EPA-induced relaxation is reduced but not abolished in sheep pulmonary artery (Singh et al., 2010). Contraction in VSMCs is largely controlled through Ca2+ entry. This is opposed by K+ efflux leading to hyperpolarization, closure of Ca2+ channels, and vasodilation (Nelson and Quayle, 1995). Multiple VSMC mechanisms are proposed to explain the effect of n-3 PUFAs, many of which include the hyperpolarizing effects of activating K+ channels. Limited results were obtained with respect to L-type voltage-gated Ca2+ channel involvement in n-3 PUFA-mediated vasodilation (Engler and Engler, 2000; Engler et al., 2000; Singh et al., 2010), whereas K+ channels have been found to play a greater role.
K+ channels involved in the regulation of vascular tone have been found to be activated by n-3 PUFAs, reviewed in (Elinder and Liin, 2017), leading to vasodilation. Multiple studies have investigated the ion channel targets of n-3 PUFAs, and found only a small contribution from BKCa (Ye et al., 2002; Lai et al., 2009; Wang et al., 2011; Hoshi et al., 2013; Sato et al., 2014; Nagaraj et al., 2016) and no contribution from Kv1.2 or 1.3 (Sato et al., 2014), SKCa (Limbu et al., 2018) as well as other types of KCa (Engler and Engler, 2000; Engler et al., 2000; Villalpando et al., 2015), and IK (Sato et al., 2014) channels (Liin et al., 2015; Larsson et al., 2018).
Vascular KATP channels are involved in responses to vasodilators, which seem to stimulate these channels (Nelson et al., 1990; Kubo et al., 1997; Quayle et al., 1997; Quinn et al., 2004). Nitric oxide leads to hyperpolarization in rabbit mesenteric artery VSMCs by activating KATP channels (Murphy and Brayden, 1995), and prostanoids also activate KATP channels (Hide et al., 1995; Ney and Feelisch, 1995; Eguchi et al., 2007). Pharmacological agents used to study vasodilation have also been used to study these effects, for example channel openers like cromakalim, and blockers such as PNU-37883A (Humphrey et al., 1996). Clinically, openers of vascular KATP channels such as pinacidil can be used in the treatment of hypertension (Friedel and Brogden, 1990). Despite this, very few studies have investigated a direct role of KATP channels in n-3 PUFA-mediated vasodilation, but some have identified a contribution. For example, in rat aorta DHA and EPA (or their metabolites) induced vasodilation in an endothelium-independent manner, which is reduced by treatment with KATP channel blockers (Engler and Engler, 2000; Sato et al., 2013; Sato et al., 2014).
Vascular KATP channels are composed of a Ki6.1 pore subunit, and a SUR2B accessory subunit (Yamada et al., 1997). Global genetic deletion of either subunit leads to hypertension and coronary artery vasospasm, resulting in absence of hyperpolarising currents and death (Chutkow et al., 2002; Miki et al., 2002). Finally, knocking out KATP channel subunits in either the endothelium or VSMC has been suggested to have detrimental effects on coronary artery circulation (Kakkar et al., 2006; Malester et al., 2007; Aziz et al., 2014; Aziz et al., 2017).
In addition to the cell membrane, the inner membrane of mitochondria also contains KATP channels, which mediates ATP-sensitive K+ currents (Paggio et al., 2019). In most part, plasma membrane KATP and mitochondrial KATP are regulated by the same ligands (Paucek et al., 1992); moreover, pinacidil, a vasodilator, activates both types of channel to a similar extent (Liu et al., 2001). Mitochondrial KATP exhibits many properties similar to those of the plasma membrane KATP channel, for example the subunit structure appears to be qualitatively similar (Garlid et al., 1996; Bajgar et al., 2001). Loss of the mitochondrial Kir pore subunit suppressed activation by diazoxide, a KATP channel opener (Foster et al., 2012), and suppressed the cardioprotective effects of diazoxide (Paggio et al., 2019).
This study aimed to investigate whether concentrations of DHA and EPA relevant to those found in human plasma following meal enriched with these fatty acids (Newens et al., 2011) can activate vascular KATP channels leading to vasodilation. The actions of n-3 PUFAs have been found to be dependent on the type of artery (Shimokawa and Godo, 2016; Limbu et al., 2018), so we investigated both the aorta (elastic artery) and the mesenteric (resistance artery). Secondly, we studied whether n-3 PUFAs act through the pore subunit. For the latter, we used a mouse knock-out (KO) of VSMC Kir6.1; this KO leads to hypertensive animals and VSMC that do not respond well to vasodilators (Aziz et al., 2014).
2 Methods
2.1 Animals
All experiments were performed according to ARRIVE2.0 guidelines (Percie du Sert et al., 2020). Mice were maintained in 12 h:12 h light: dark cycle, a room temperature of 21°C and humidity of 50 ± 15%, with ad libitum access to food and water. Only male mice were used, as some studies have found that the blood pressure-reducing effects of DHA are stronger in men than women (Singhal et al., 2013). Moreover, there is evidence that acute supplementation of n-3 PUFAs does not affect outcomes related to post-prandial vasodilator responses in healthy female volunteers (Doppler flow and flow-mediated dilatation) but enhances them in males (Newens et al., 2011). 46 C57 BL6 mice were used housed at University of Reading, 25 C57 BL6 mice were used housed at William Harvey Institute Queen Mary University London, and 25 sm22cre + Kir6.1 (flx/flx) KO C57 BL6 mice were used housed at William Harvey Institute Queen Mary University London. The sm22cre + Kir6.1 (flx/flx) KO were obtained as discussed in (Aziz et al., 2014). Briefly, these are smooth muscle cell specific and obtained from crossing smooth muscle 22alpha promoter driven cre-transgenic mice (sm22cre) with Kir6.1 homozygous floxed (Kir6.1 (flx/flx)) mice, followed by a cross of the offspring. The KO animals were genotyped as previously described. All experiments were conducted in accordance with the Guide for the Care and Use of Laboratory Animals published by the British Home Office regulations (covered by project license PE9055EAD) and by the US National Institutes of Health (NIH Publication No. 85-23, revised 1996).
2.2 Materials
All salts for Krebs and electrophysiology solutions were obtained from Fisher Scientific. U46619 (Tocris BioTechne 1932; PubChem CID 5311493) and PNU-37883A (Tocris BioTechne 2095; PubChem CID 64392) were dissolved in 100% DMSO (PubChem CID 679), with further dilutions performed in DMSO; levcromakalim (Tocris 1378, PubChem CID 93504) was dissolved in distilled deionised water; DHA (Sigma D2534, PubChem CID 445580) and EPA (Sigma E2011, PubChem CID 446284) were dissolved in 100% ethanol, with further dilutions performed in distilled deionised water. The final concentration of ethanol was 0.3%. Phenylephrine (Tocris 2838, PubChem CID 6041) and acetylcholine (Sigma A6625, PubChem CID 6060) were dissolved in distilled deionised water. All stocks were prepared at 10 mM. MgATP (A9187, PubChem CID 5957) and NaADP (A2754, PubChem CID 6022) were purchased from Sigma.
2.3 Wire myography
8–10 week old male C57 BL6 mice were killed according to schedule one of the Animals (Scientific Procedures) Act 1986.
The animal was first anaesthetized using isoflurane, followed by immediate decapitation. The aorta and mesenteric vascular beds were extracted and placed on ice-cold Krebs buffer (118 mM NaCl, 3.6 mm KCl, 1.2 mm MgSO4.7H2O, 1.2 mm KH2PO4, 2.5 mm CaCl2, 11 mm glucose, 24 mm NaHCO3).
The aorta and third order mesenteric artery (2 mm in length) were cleaned of fat and connective tissue and mounted on a wire myograph (Danish Myotechnology, 620M) connected to a force transducer (PowerLab ML846, ADInstruments) and the Labchart 7 software suite. The organ bath was filled with Krebs heated at 37°C and bubbled with carbogen (95% O2 and 5% CO2). The tissue was subjected to zero tension followed by equilibration for 20 min. The tissue was then stretched to a standardized tension of 5–6 mn (aorta) and 1-2 mn (mesenteric artery) according to the DMT normalization module in Labchart 7.
The presence of a functional endothelium was tested by first constricting the artery with 1–3 µm phenylephrine, followed by relaxation with 1 µm acetylcholine. A relaxation of over 50% was considered to represent a viable endothelium. In experiments investigating the role of the endothelium, the artery was tested as before, mechanically denuded by rubbing a 40 µm silver wire around the inner wall, and retested. A relaxation of less than 10% was considered to indicate a lack of functional endothelium.
After resting, the arteries were constricted with 10–30 nM U46619. The concentration response curves to DHA and EPA were then performed (for concentrations 100 nm–30 µm) in the presence or absence of drug treatment with 3 µm of the blocker PNU-37883A, which is purported to work through the Kir6.1 pore subunit of vascular KATP (Cui et al., 2003). For the aorta, a single U46619 concentration response curve was performed following 20 min treatment, with a time control aortic ring from the same aorta used as a control. For the mesenteric, two concentration response curves were performed in the same tissue, before and after treatment.
2.4 Isolation of aortic smooth muscle cells and patch-clamp electrophysiology
The aorta was collected as indicated above and placed in physiological solution (125 mm NaCl, 4.8 mm KCl, 1.2 mm KH2PO4, 1.1 mm EDTA, 1.7 mm MgCl2, 1 mm EGTA, 10 mm HEPES, and 11 mm glucose, pH 7.4). The artery was cleaned of fat and connective tissue, cut into four segments, and slit open. To isolate smooth muscle cells, the tissue was digested in low-Ca2+ physiological solution (136 mm NaCl, 5.6 mm KCl, 4.17 mm NaHCO3, 0.44 mm NaH2PO4, 0.42 mm Na2HPO4, 10.47 mm MgCl2, 0.1 mm CaCl2, 10 mm HEPES, pH 7.4), and then in dissociation solution (125 mm NaCl, 5 mM KCl, 0.1 mm CaCl2, 1 mm MgCl2, 10 mm NaHCO3, 0.5 mm KH2PO4, 0.5 mm NaH2PO4, 10 mm glucose, 10 mm HEPES, pH 7.2), as follows. The tissue was placed in low-Ca2+ physiological solution containing 2 mg/ml collagenase type 2 (Sigma 17101015) for 10 min at room temperature followed by 20 min at 37°C. The tissue was then washed in physiological solution and gently triturated. The tissue was then incubated in dissociation solution containing 0.7 mg/ml papain (Sigma P3125), 0.25 mg/ml BSA, and 0.5 mm DTT for 15 min at 37°C with shaking, and then in dissociation solution containing 0.5 mg/ml collagenase and 0.25 mg/ml BSA for 15 min at 37°C. The tissue was then washed in dissociation solution and triturated in dissociation solution. The cells were kept at 4°C until used. All recordings were made on the same days as the cell isolation.
K+ currents were recorded with a voltage clamp at −75 mV, in whole-cell configuration. These currents were evoked with a 30 m, 115 mV voltage step.
Whole cell currents were acquired with a Multiclamp 700B amplifier and digitised using a Digidata 1550B (Molecular Devices, United States), sampled at 100 kHz and lowpass filtered at 10 kHz. All data were visualized and stored onto a PC using the pClamp11 (Molecular Devices, United States) software routine.
The internal solution contained 107 mM KCl, 1.2 mM MgCl2, 1 mM CaCl2, 10 mm EGTA, 5 mm HEPES, 0.1 mm MgATP (Sigma A9187) and 1 mm NaADP (Sigma A2754), pH 7.2. The external solution contained 110 mm NaCl, 5 mm KCl, 1.2 mm MgCl2, 1.8 mm CaCl2, 10 mm glucose, and 10 mm HEPES, pH 7.2.
2.5 Data analysis and statistical procedures
Data analysis was performed in GraphPad Prism 7. Data is presented as mean ± SEM. We did not perform blinding or randomization.
For the analysis of myography data, and n of a minimum of five biological repeats were used, where n corresponds to the number of animals. Power calculations were conducted using the on-line tool http://www.stat.ubc.ca/∼rollin/stats/ssize/n2.html based on our preliminary myograph data. This study used the parameters of a common standard deviation, a normal distribution, a 0.05 type 1 error rate and a power of 80%. The vasodilatory response was calculated as a percentage of the reduction from the stable plateau of U46619 and the concentration response curve was plotted. To test for significance, two-way ANOVA multiple comparisons was used, followed by Bonferroni’s post-test. A p-value of <0.05 was considered statistically significant.
For the analysis of electrophysiological data, and n of eight biological repeats were used, where n is the number of animals from which cells were isolated or where cell lines were used form a separate passage. The current densities were calculated as a fraction of the baseline. We tested for normal distribution using the Shapiro-Wilk normality test, as it did not follow a normal distribution this data was presented as median and min to max whiskers. To test for significance, a Friedman test was performed. A p-value of less than 0.05 was considered statistically significant.
3 Results
3.1 The effect of n-3 PUFAs on mouse aorta and mesenteric arteries
3.1.1 The role of KATP channels in n-3 PUFA-dependent relaxation in mouse aorta and mesenteric
We studied the effects of DHA and EPA in mouse aorta and mesenteric arteries by conducting a concentration response curve to acute treatment following pre-constriction with U46619, a thromboxane A2 mimetic. Vehicle control experiments showed ethanol had no effect on tone (see representative experiment in Supplementary Figure S1). We did not use glibenclamide as a selective inhibitor in our studies as we (data not shown) and others (Cocks et al., 1990) have observed that it is an antagonist of thromboxane A2 receptors and leads to relaxation of U46619-induced tone. Comparable maximal tone was elicited in both treated and untreated vessels (Supplementary Figure S2). We found that in the aorta relaxation was significantly reduced for both EPA and DHA (Figures 1A–D), but that in the mesenteric artery there was no effect on n-3 PUFA-mediated relaxations (Figures 1E–H). Therefore, we continued further experiments only in the aorta.
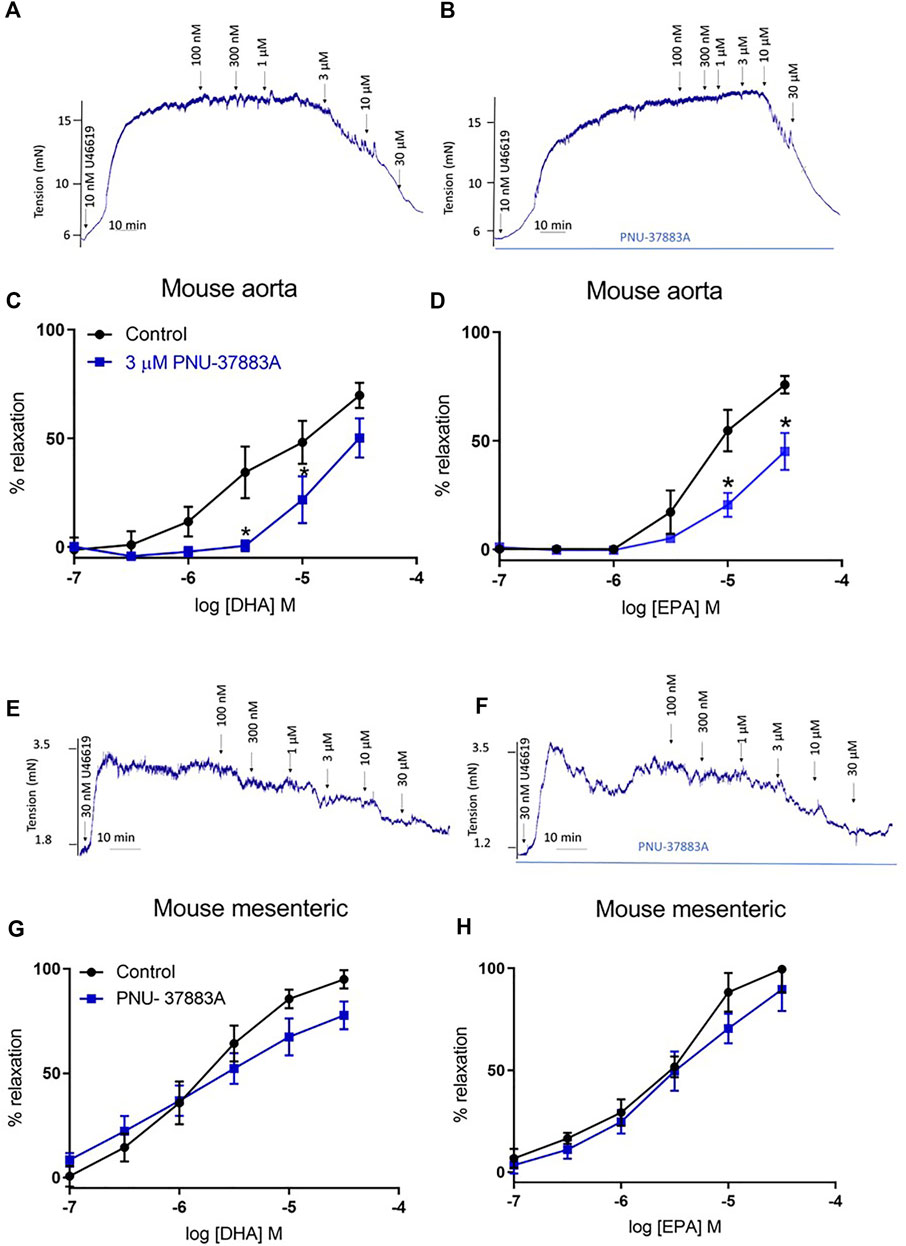
FIGURE 1. Concentration response curves showing the effects of inhibition of KATP channels on n-3 PUFA-induced relaxation in mouse aorta and mesenteric preconstricted with U46619. (A) Representative organ bath traces showing the effect of DHA (100 nm–30 μm) on mouse aortic tone. (B) Representative organ bath traces showing the effect of PNU-37883A treatment on DHA (100 nm–30 μm)-induced changes in mouse aortic tone. (C) DHA- and (D) EPA-induced relaxation in mouse aorta following treatment with the KATP inhibitor PNU-37883A (3 µm). (E) Representative organ bath traces showing the effect of DHA (100 nm–30 μm) on mouse mesenteric tone. (F) Representative organ bath traces showing the effect of PNU-37883A treatment on DHA (100 nm–30 μm)-induced changes in mouse mesenteric tone. (G) DHA- and (H) EPA-induced relaxation in mouse mesenteric following treatment with the KATP inhibitor PNU-37883A (3 µm). n = 5, data represented as mean ± SEM. *p < 0.05, significant difference from control curve assessed by 2-way ANOVA followed by Bonferroni multiple comparison test.
3.1.2 The role of the endothelium in n-3 PUFA-dependent relaxation in mouse aorta
We assessed the role of endothelium on n-3 PUFA-mediated dilations in the mouse aorta. We found that endothelium removal did not affect the relaxation to EPA, and partly reduced the relaxation to DHA at a low concentration (3 µm) (Figure 2).
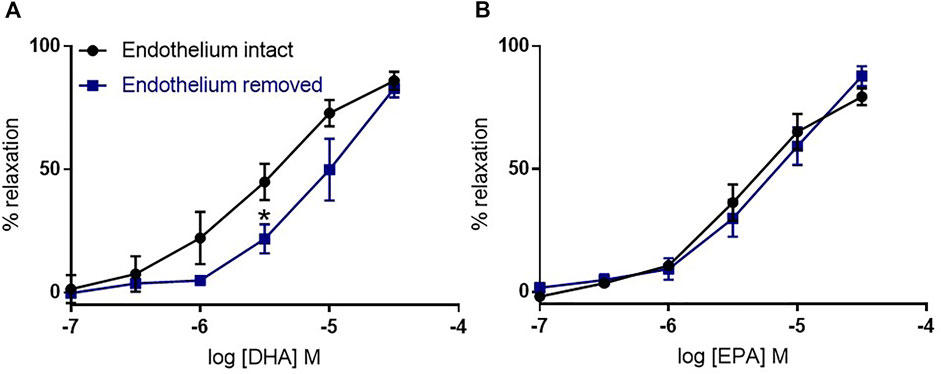
FIGURE 2. The effects of endothelium removal on DHA- and EPA-induced relaxation in mouse aortic rings preconstricted with U46619. (A) DHA- and (B) EPA-induced relaxation in denuded mouse aorta compared with intact rings from the same animal. n = 5, data represented as mean ± SEM. *p < 0.05, significant difference from control curve assessed by 2-way ANOVA followed by Bonferroni multiple comparison test.
3.2 Validation of Kir6.1 knock out model with an opener of KATP channels
We studied the role of the Kir6.1 subunit using a knock-out (KO) mouse model and compared this to wild-type littermate controls. We first confirmed that Kir6.1 KO channels do respond to KATP-selective channel openers. To study this, we performed concentration response curves to the KATP opener levcromakalim, reported to act through the SUR subunit (Moreau et al., 2000). We found that levcromakalim causes relaxation in both the KO and the WT, with significantly less relaxation in the KO at low concentrations, and treatment with PNU-37883A inhibited relaxation in both WT and KO (Figure 3).
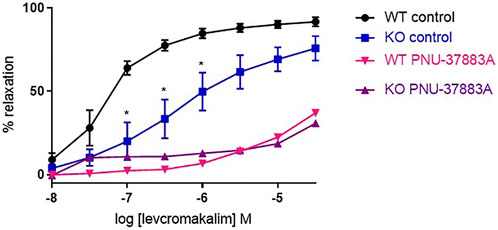
FIGURE 3. The effect of the KATP-selective channel opener levcromakalim on Kir6.1 KO mouse aortic rings preconstricted with U46619. Levcromakalim-induced relaxation in WT and KO mouse aorta following treatment with the KATP inhibitor PNU-37883A (3 µm) compared with untreated rings from the same animal. n = 5, data represented as mean ± SEM. *p < 0.05, significant difference from control curve assessed by 2-way ANOVA followed by Bonferroni multiple comparison test.
3.3 n-3 PUFA-dependent relaxation in Kir6.1 KO
We then compared WT and KO responses to DHA and EPA (Figure 4). In the VSMC Kir6.1 KO model there was no reduction of the relaxation produced by DHA or EPA; interestingly, there appeared to be a small but significant increase in the relaxation produced. Thus, VSMC Kir6.1 does not appear to be involved in relaxations produced by n-3 PUFAs.
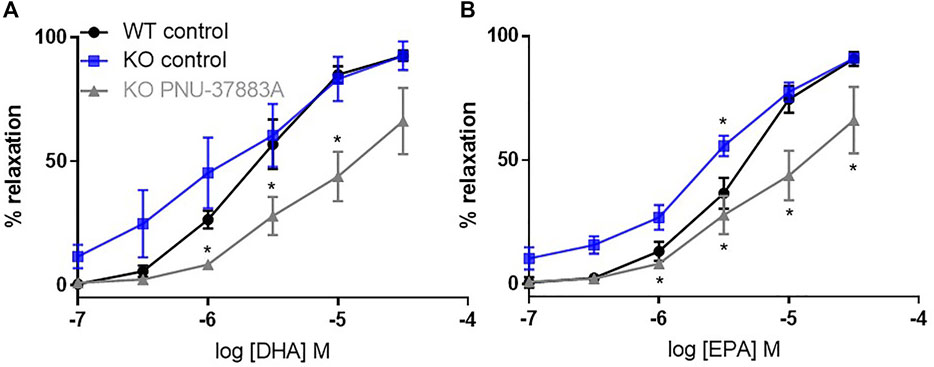
FIGURE 4. The effects of inhibition of KATP channels on n-3 PUFA-induced relaxation in Kir6.1 KO mouse aortic rings preconstricted with U46619. (A) DHA- and (B) EPA-induced relaxation in Kir6.1 KO mouse aorta following treatment with the KATP inhibitor PNU-37883A (3 µm) compared with untreated rings from the same animal. n = 5, data represented as mean ± SEM. *p < 0.05, significant difference from control curve assessed by 2-way ANOVA followed by Bonferroni multiple comparison test.
Surprisingly PNU-37883A led to a significant reduction in the percentage of relaxation to both DHA and EPA (Figure 4) despite Kir6.1 being the putative target of PNU-37883A.
3.4 The effect of DHA on K+ currents in vascular smooth muscle cells
The effect of DHA on K+ currents in primary VSMC from WT mouse aorta was measured. We found that the bath application of 30 µm DHA had no effect on K+ current densities. However, the bath application of 10 µm levcromakalim resulted in increased current densities and this increase was reverted to baseline values by the subsequent bath application of PNU-37882A (Figure 5). These observations suggest that the currents measured from the primary aorta VSMCs contained a KATP, levcromakalim-sensitive component and that DHA does not affect this component. We also found that currents in a stable cell line expressing the KATP structure Kir6.1/SUR2B were unaffected by DHA (Supplementary Figure S2), but activated by either levcromakalim or pinacidil, both of which were subsequently inhibited by PNU-37883A.
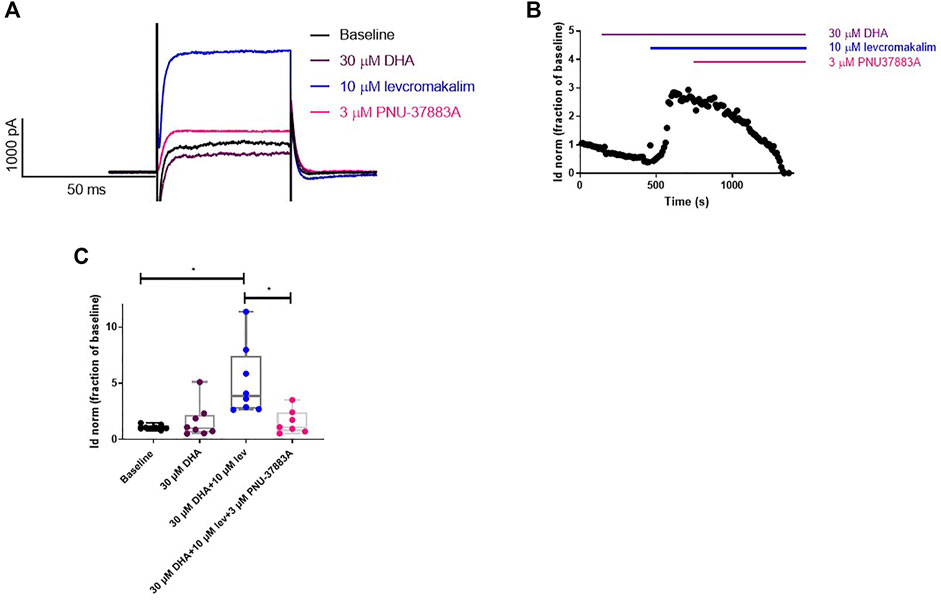
FIGURE 5. DHA does not affect K+ currents in vascular smooth muscle cells isolated from mouse aorta. (A) Representative current traces. (B) Representative whole cell current density time course trace. (C) DHA (30 µm) does not affect the K+ current density in primary mouse aorta VSMCs. The KATP-selective opener levcromakalim (10 µm) causes an increase in these currents which is reversed by the KATP blocker PNU-37883A (3 µm). n = 8, data represented as normalised current density as a fraction of the baseline, with median and min to max whiskers. *p < 0.05, significant difference from baseline curve assessed by one-way ANOVA with Friedman’s test and Dunn’s correction for multiple comparisons. Lev = levcromakalim.
3.5 No role for mitochondrial KATP channels in n-3 PUFA-dependent relaxation in mouse aorta
The Kir6.1 KO is expected to affect KATP in the VSMC plasma membrane, but it has not been established what the effect is on other KATP channels in VSMCs. We used the blocker 5-HD, which is proposed to act through the SUR subunit (Jaburek et al., 1998). We found that inhibition of mitochondrial KATP did not significantly affect relaxation to either DHA or EPA, confirming that mitochondrial KATP is not involved in the effect (Figure 6).
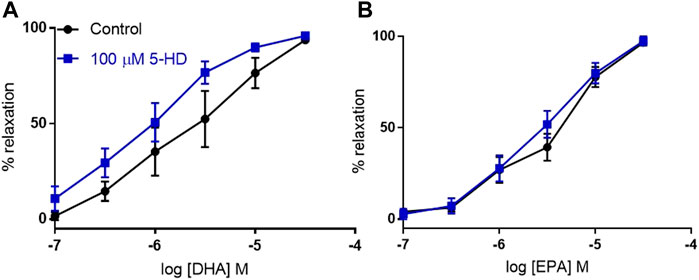
FIGURE 6. The effects of inhibition of mitochondrial KATP channels on n-3 PUFA-induced relaxation in mouse aortic rings preconstricted with U46619. (A) DHA- and (B) EPA-induced relaxation in mouse aorta following treatment with the selective mitochondrial KATP inhibitor 5-HD (100 µM) compared with untreated rings from the same animal. n = 5, data represented as mean ± SEM. *p < 0.05, significant difference from control curve assessed by two way ANOVA followed by Bonferroni multiple comparison test.
4 Discussion and conclusion
In this study we demonstrated using a sm22cre + Kir6.1 (flx/flx) Kir6.1 KO that it is highly unlikely that the subtype of KATP most common in smooth muscle cells (Kir6.1/SUR2B) underlies the n-3 PUFA-mediated relaxations observed in the aorta. This is despite relaxations produced by DHA and EPA having a major component sensitive to pharmacological blockade of KATP in WT mice. The results also call into question the selectivity of a pharmacological tool commonly used to inhibit KATP mediated responses.
KATP channels have been previously implicated in n-3 PUFA-mediated vasodilation, with KATP inhibition suppressing DHA-induced relaxation (Engler and Engler, 2000; Engler et al., 2000; Sato et al., 2013; Sato et al., 2014; Villalpando et al., 2015). Therefore, in this study we investigated whether DHA- and EPA-induced relaxations may involve these channels using pharmacological inhibitors. The actions of n-3 PUFAs show heterogeneity based on the type of artery (Shimokawa and Godo, 2016; Limbu et al., 2018), so we studied n-3 PUFA-mediated relaxation in both the aorta (elastic artery) and the mesenteric artery (resistance artery). We demonstrated that DHA and EPA caused concentration-dependent relaxations in both artery types at physiologically relevant concentrations (100 nm-30 µm) that are below the peak concentration recorded in human plasma after an n-3 PUFA rich meal (70 µm) (Newens et al., 2011). Relaxations in the aorta were largely endothelium-independent and significantly attenuated by the KATP blocker PNU-37883A but those in the mesenteric were unaffected.
Vascular KATP channels are composed of a Kir6.1 pore subunit and a SUR2B accessory subunit (Yamada et al., 1997). We investigated whether DHA and EPA activate vascular KATP channels using a mouse KO model of VSMC Kir6.1. We first confirmed Kir6.1 KO channel response to KATP-selective channel opener levcromakalim, with levcromakalim being purported to activate KATP by an action on the SUR subunit (Schwanstecher et al., 1998; Hambrock et al., 1999). As expected, levcromakalim caused relaxation in both the KO and the WT, with significant inhibition of relaxation in the KO. The vascular KATP channel blocker PNU-37883A (Humphrey et al., 1996) inhibited levcromakalim-mediated relaxation in the KO, to the same degree as in the WT. The residual relaxation to levcromakalim may have been expected as Kir6.1/SUR2B KATP channel subtypes are expressed in the endothelium (Tang et al., 2010; Aziz et al., 2017). In addition, PNU-37883A may not only act through the Kir6.1 subunit; it might be inhibiting another channel such as a voltage-gated Ca2+ channels (Tomoda et al., 2005). We would have used another KATP blocker to confirm this, but our studies (data not shown) and others (Cocks et al., 1990) have shown that glibenclamide leads to relaxation of U46619-induced tone.
Having confirmed a role for VSMC KATP with the structure Kir6.1/SUR2B in vascular relaxation to levcromakalim, we compared WT and KO response to DHA and EPA and found no inhibition of the relaxation in the KO. Despite this, PNU-37883A still blocked this largely endothelium-independent relaxation, further indicating the inhibition of n-3 PUFA-mediated relaxation produced by PNU-37883A is highly unlikely to be due to an effect on KATP channels with subunit composition of Kir6.1/SUR2B. We considered whether the blocker might be inhibiting n-3 PUFA-mediated relaxation through the vascular subtype Kir6.2/SUR2B (Cui et al., 2003; Kovalev et al., 2004; Teramoto, 2006). However, this is unlikely as although this subtype mRNA is present in the KO, the channel is not functional (Aziz et al., 2014). A possible target is the endothelial Kir6.1/SUR2B subtype (Aziz et al., 2017), but this is unlikely because the relaxation to n-3 PUFAs that we observed is largely endothelium-independent.
The KO is expected to affect the pore subunit of plasma membrane vascular KATP, but it has not been established what the effect is on other KATP channels in VSMC, such as mitochondrial KATP. Mitochondrial KATP channels mediate ATP-sensitive K+ currents (Paggio et al., 2019), and are largely regulated by the same ligands as plasma membrane KATP channels (Paucek et al., 1992); the vasodilator pinacidil activates both types of channel to a similar extent (Liu et al., 2001). Moreover, mitochondrial KATP channels are activated by diazoxide, a KATP channel opener (Foster et al., 2012) which has cardioprotective effects (Paggio et al., 2019). In the present study, pharmacological blockade of mitochondrial KATP with 5-HD (Jaburek et al., 1998) did not significantly affect relaxation to either DHA or EPA, suggesting that mitochondrial KATP is not involved in this relaxation. This finding also eliminates the possibility that PNU-37883A might be acting on mitochondrial KATP channels.
As the myography data from the KO indicated n-3 PUFAs do not affect KATP currents and this was confirmed using whole cell patch clamp. DHA had no effect on the density of total K+ currents in native VSMC or stable cell lines expressing Kir6.1/SUR2B. This agrees with a previous study where a DHA-induced increase in outward K+ current in rat coronary VSMCs was not reduced by KATP channel inhibition with glyburide (Wang et al., 2011). These data confirm that the relaxation produced by n-3 PUFAs does not involve activation of vascular KATP (Kir 6.1/SUR2B) or any other KATP subtype.
One limitation of this study is that we did not investigate the contribution of other K+ channels. Of interest, several other types of potassium channel have been proposed to be involved in omega-3-mediated vasodilation, for example BKCa and Kv7.4/5 (Bercea et al., 2021). Notably, (Frampton et al., 2022), have recently demonstrated in Xenopus oocytes that n-3 PUFAs target the Kv7.4 and Kv7.5 subtypes, which are also highly expressed in vascular tissue. Therefore, future research should be focused on these channel subtypes in the vasculature.
In conclusion, this study found that DHA and EPA act to regulate vasodilation and hence are likely to reduce blood pressure, and these relaxations have a component apparently sensitive to the blockade of KATP in the aorta. However, knocking out the vascular smooth muscle cell pore subunit Kir6.1 had no effect on these vasodilatory responses, yet the putative Kir6.1 selective blocker PNU-37883A still led to a significant reduction in the percentage of relaxation to both DHA and EPA in the KO. Hence, the knock-out model data also casts light on the selectivity of PNU-37883A for VSMC KATP channels with the composition Kir6.1/SUR2B. We confirmed that n-3 PUFAs do not affect vascular KATP currents using whole-cell patch-clamping in native cells from WT mouse aorta, where DHA had no effect on the density of total K+ currents. This study provides evidence of heterogeneity between mouse aorta and mesenteric in the mechanisms of n-3 PUFA-mediated vasodilation and shows that plasma membrane and mitochondrial KATP do not mediate DHA or EPA-induced vasodilation.
Data availability statement
The raw data supporting the conclusions of this article will be made available by the authors, without undue reservation.
Ethics statement
The animal study was reviewed and approved by the University of Reading ethics committee.
Author contributions
CB: conceptualization, methodology, data curation, formal analysis, investigation, visualization, writing—original draft, writing—review and editing. RL: data curation, formal analysis, investigation, visualization. KB: data curation, formal analysis, investigation. K-EN: supervision, writing—review and editing. QA: supervision, writing—review and editing. AT: conceptualization, supervision, visualization, writing-review and editing. FT: conceptualization, supervision, visualization, writing-review and editing. GC: conceptualization, supervision, visualization, writing-review and editing. AM: conceptualization, formal analysis, funding acquisition, methodology, project administration, resources, supervision, validation, visualization, writing—original draft, writing—review and editing.
Funding
This research was funded by the PG/19/57/34568 from the British Heart Foundation and 1R01HL146514-01A1 from the National Institutes of Health (United States).
Conflict of interest
The authors declare that the research was conducted in the absence of any commercial or financial relationships that could be construed as a potential conflict of interest.
Publisher’s note
All claims expressed in this article are solely those of the authors and do not necessarily represent those of their affiliated organizations, or those of the publisher, the editors and the reviewers. Any product that may be evaluated in this article, or claim that may be made by its manufacturer, is not guaranteed or endorsed by the publisher.
Supplementary material
The Supplementary Material for this article can be found online at: https://www.frontiersin.org/articles/10.3389/fphys.2022.1033216/full#supplementary-material
References
Abumweis S., Jew S., Tayyem R., Agraib L. (2018). Eicosapentaenoic acid and docosahexaenoic acid containing supplements modulate risk factors for cardiovascular disease: A meta-analysis of randomised placebo-control human clinical trials. J. Hum. Nutr. Diet. 31, 67–84. doi:10.1111/jhn.12493
Aziz Q., Li Y., Anderson N., Ojake L., Tsisanova E., Tinker A. (2017). Molecular and functional characterization of the endothelial ATP-sensitive potassium channel. J. Biol. Chem. 292, 17587–17597. doi:10.1074/jbc.M117.810325
Aziz Q., Thomas A. M., Gomes J., Ang R., Sones W. R., Li Y., et al. (2014). The ATP-sensitive potassium channel subunit, Kir6.1, in vascular smooth muscle plays a major role in blood pressure control. Hypertension 64, 523–529. doi:10.1161/HYPERTENSIONAHA.114.03116
Bajgar R., Seetharaman S., Kowaltowski A. J., Garlid K. D., Paucek P. (2001). Identification and properties of a novel intracellular (mitochondrial) ATP-sensitive potassium channel in brain. J. Biol. Chem. 276, 33369–33374. doi:10.1074/jbc.M103320200
Bang H. O., Dyerberg J., Sinclair H. M. (1980). The composition of the Eskimo food in north Western Greenland. Am. J. Clin. Nutr. 33, 2657–2661. doi:10.1093/ajcn/33.12.2657
Bercea C. I., Cottrell G. S., Tamagnini F., Mcneish A. J. (2021). Omega-3 polyunsaturated fatty acids and hypertension: A review of vasodilatory mechanisms of docosahexaenoic acid and eicosapentaenoic acid. Br. J. Pharmacol. 178, 860–877. doi:10.1111/bph.15336
Chutkow W. A., Pu J., Wheeler M. T., Wada T., Makielski J. C., Burant C. F., et al. (2002). Episodic coronary artery vasospasm and hypertension develop in the absence of Sur2 K(ATP) channels. J. Clin. Invest. 110, 203–208. doi:10.1172/JCI15672
Cocks T. M., King S. J., Angus J. A. (1990). Glibenclamide is a competitive antagonist of the thromboxane A2 receptor in dog coronary artery in vitro. Br. J. Pharmacol. 100, 375–378. doi:10.1111/j.1476-5381.1990.tb15812.x
Colussi G., Catena C., Novello M., Bertin N., Sechi L. A. (2017). Impact of omega-3 polyunsaturated fatty acids on vascular function and blood pressure: Relevance for cardiovascular outcomes. Nutr. Metab. Cardiovasc. Dis. 27, 191–200. doi:10.1016/j.numecd.2016.07.011
Cui Y., Tinker A., Clapp L. H. (2003). Different molecular sites of action for the KATP channel inhibitors, PNU-99963 and PNU-37883A. Br. J. Pharmacol. 139, 122–128. doi:10.1038/sj.bjp.0705228
Eguchi S., Kawano T., Yinhuatanaka K., Yasui S., Mawatari K., Takahashi A., et al. (2007). Effects of prostaglandin E1 on vascular ATP-sensitive potassium channels. J. Cardiovasc. Pharmacol. 50, 686–691. doi:10.1097/FJC.0b013e3181583d9b
Elinder F., Liin S. I. (2017). Actions and mechanisms of polyunsaturated fatty acids on voltage-gated ion channels. Front. Physiol. 8, 43. doi:10.3389/fphys.2017.00043
Engler M. B., Engler M. M., Browne A., Sun Y. P., Sievers R. (2000). Mechanisms of vasorelaxation induced by eicosapentaenoic acid (20:5n-3) in WKY rat aorta. Br. J. Pharmacol. 131, 1793–1799. doi:10.1038/sj.bjp.0703754
Engler M. B., Engler M. M. (2000). Docosahexaenoic acid--induced vasorelaxation in hypertensive rats: Mechanisms of action. Biol. Res. Nurs. 2, 85–95. doi:10.1177/109980040000200202
Foster D. B., Ho A. S., Rucker J., Garlid A. O., Chen L., Sidor A., et al. (2012). Mitochondrial ROMK channel is a molecular component of mitoK(ATP). Circ. Res. 111, 446–454. doi:10.1161/CIRCRESAHA.112.266445
Frampton D. J. A., Choudhury K., Nikesjo J., Delemotte L., Liin S. I. (2022). Subtype-specific responses of hKv7.4 and hKv7.5 channels to polyunsaturated fatty acids reveal an unconventional modulatory site and mechanism. Elife 11, e77672. doi:10.7554/eLife.77672
Friedel H. A., Brogden R. N. (1990). Pinacidil. A review of its pharmacodynamic and pharmacokinetic properties, and therapeutic potential in the treatment of hypertension. Drugs 39, 929–967. doi:10.2165/00003495-199039060-00008
Garlid K. D., Paucek P., Yarov-Yarovoy V., Sun X., Schindler P. A. (1996). The mitochondrial KATP channel as a receptor for potassium channel openers. J. Biol. Chem. 271, 8796–8799. doi:10.1074/jbc.271.15.8796
Hambrock A., Loffler-Walz C., Kloor D., Delabar U., Horio Y., Kurachi Y., et al. (1999). ATP-sensitive K+ channel modulator binding to sulfonylurea receptors SUR2A and SUR2B: Opposite effects of MgADP. Mol. Pharmacol. 55, 832–840.
Hamilton J. A., Kamp F. (1999). How are free fatty acids transported in membranes? Is it by proteins or by free diffusion through the lipids? Diabetes 48, 2255–2269. doi:10.2337/diabetes.48.12.2255
Hide E. J., Ney P., Piper J., Thiemermann C., Vane J. R. (1995). Reduction by prostaglandin E1 or prostaglandin E0 of myocardial infarct size in the rabbit by activation of ATP-sensitive potassium channels. Br. J. Pharmacol. 116, 2435–2440. doi:10.1111/j.1476-5381.1995.tb15092.x
Hoshi T., Wissuwa B., Tian Y., Tajima N., Xu R., Bauer M., et al. (2013). Omega-3 fatty acids lower blood pressure by directly activating large-conductance Ca²⁺-dependent K⁺ channels. Proc. Natl. Acad. Sci. U. S. A. 110, 4816–4821. doi:10.1073/pnas.1221997110
Humphrey S. J., Smith M. P., Cimini M. G., Buchanan L. V., Gibson J. K., Khan S. A., et al. (1996). Cardiovascular effects of the K-ATP channel blocker U-37883A and structurally related morpholinoguanidines. Methods Find. Exp. Clin. Pharmacol. 18, 247–260.
Jaburek M., Yarov-Yarovoy V., Paucek P., Garlid K. D. (1998). State-dependent inhibition of the mitochondrial KATP channel by glyburide and 5-hydroxydecanoate. J. Biol. Chem. 273, 13578–13582. doi:10.1016/s0021-9258(19)57796-1
Kagawa Y., Nishizawa M., Suzuki M., Miyatake T., Hamamoto T., Goto K., et al. (1982). Eicosapolyenoic acids of serum lipids of Japanese islanders with low incidence of cardiovascular diseases. J. Nutr. Sci. Vitaminol. 28, 441–453. doi:10.3177/jnsv.28.441
Kakkar R., Ye B., Stoller D. A., Smelley M., Shi N. Q., Galles K., et al. (2006). Spontaneous coronary vasospasm in KATP mutant mice arises from a smooth muscle-extrinsic process. Circ. Res. 98, 682–689. doi:10.1161/01.RES.0000207498.40005.e7
Kovalev H., Quayle J. M., Kamishima T., Lodwick D. (2004). Molecular analysis of the subtype-selective inhibition of cloned KATP channels by PNU-37883A. Br. J. Pharmacol. 141, 867–873. doi:10.1038/sj.bjp.0705670
Kubo M., Quayle J. M., Standen N. B. (1997). Angiotensin II inhibition of ATP-sensitive K+ currents in rat arterial smooth muscle cells through protein kinase C. J. Physiol. 503 (3), 489–496. doi:10.1111/j.1469-7793.1997.489bg.x
Lai L. H., Wang R. X., Jiang W. P., Yang X. J., Song J. P., Li X. R., et al. (2009). Effects of docosahexaenoic acid on large-conductance Ca2+-activated K+ channels and voltage-dependent K+ channels in rat coronary artery smooth muscle cells. Acta Pharmacol. Sin. 30, 314–320. doi:10.1038/aps.2009.7
Larsson J. E., Larsson H. P., Liin S. I. (2018). KCNE1 tunes the sensitivity of KV7.1 to polyunsaturated fatty acids by moving turret residues close to the binding site. Elife 7, e37257. doi:10.7554/eLife.37257
Liin S. I., Silvera Ejneby M., Barro-Soria R., Skarsfeldt M. A., Larsson J. E., Starck Harlin F., et al. (2015). Polyunsaturated fatty acid analogs act antiarrhythmically on the cardiac IKs channel. Proc. Natl. Acad. Sci. U. S. A. 112, 5714–5719. doi:10.1073/pnas.1503488112
Limbu R., Cottrell G. S., Mcneish A. J. (2018). Characterisation of the vasodilation effects of DHA and EPA, n-3 PUFAs (fish oils), in rat aorta and mesenteric resistance arteries. PLoS One 13, e0192484. doi:10.1371/journal.pone.0192484
Liu Y., Ren G., O'Rourke B., Marban E., Seharaseyon J. (2001). Pharmacological comparison of native mitochondrial K(ATP) channels with molecularly defined surface K(ATP) channels. Mol. Pharmacol. 59, 225–230. doi:10.1124/mol.59.2.225
Malester B., Tong X., Ghiu I., Kontogeorgis A., Gutstein D. E., Xu J., et al. (2007). Transgenic expression of a dominant negative K(ATP) channel subunit in the mouse endothelium: Effects on coronary flow and endothelin-1 secretion. FASEB J. 21, 2162–2172. doi:10.1096/fj.06-7821com
Miki T., Suzuki M., Shibasaki T., Uemura H., Sato T., Yamaguchi K., et al. (2002). Mouse model of Prinzmetal angina by disruption of the inward rectifier Kir6.1. Nat. Med. 8, 466–472. doi:10.1038/nm0502-466
Miller P. E., van Elswyk M., Alexander D. D. (2014). Long-chain omega-3 fatty acids eicosapentaenoic acid and docosahexaenoic acid and blood pressure: A meta-analysis of randomized controlled trials. Am. J. Hypertens. 27, 885–896. doi:10.1093/ajh/hpu024
Moreau C., Jacquet H., Prost A. L., D'Hahan N., Vivaudou M. (2000). The molecular basis of the specificity of action of K(ATP) channel openers. EMBO J. 19, 6644–6651. doi:10.1093/emboj/19.24.6644
Mozaffarian D., Wu J. H. (2011). Omega-3 fatty acids and cardiovascular disease: Effects on risk factors, molecular pathways, and clinical events. J. Am. Coll. Cardiol. 58, 2047–2067. doi:10.1016/j.jacc.2011.06.063
Murphy M. E., Brayden J. E. (1995). Nitric oxide hyperpolarizes rabbit mesenteric arteries via ATP-sensitive potassium channels. J. Physiol. 486 (1), 47–58. doi:10.1113/jphysiol.1995.sp020789
Nagaraj C., Tang B., Nagy B. M., Papp R., Jain P. P., Marsh L. M., et al. (2016). Docosahexaenoic acid causes rapid pulmonary arterial relaxation via KCa channel-mediated hyperpolarisation in pulmonary hypertension. Eur. Respir. J. 48, 1127–1136. doi:10.1183/13993003.01814-2015
Nelson M. T., Huang Y., Brayden J. E., Hescheler J., Standen N. B. (1990). Arterial dilations in response to calcitonin gene-related peptide involve activation of K+ channels. Nature 344, 770–773. doi:10.1038/344770a0
Nelson M. T., Quayle J. M. (1995). Physiological roles and properties of potassium channels in arterial smooth muscle. Am. J. Physiol. 268, C799–C822. doi:10.1152/ajpcell.1995.268.4.C799
Newens K. J., Thompson A. K., Jackson K. G., Wright J., Williams C. M. (2011). DHA-rich fish oil reverses the detrimental effects of saturated fatty acids on postprandial vascular reactivity. Am. J. Clin. Nutr. 94, 742–748. doi:10.3945/ajcn.110.009233
Ney P., Feelisch M. (1995). Vasodilator effects of PGE1 in the coronary and systemic circulation of the rat are mediated by ATP-sensitive potassium (K+) channels. Agents actions. Suppl. 45, 71–76. doi:10.1007/978-3-0348-7346-8_11
Paggio A., Checchetto V., Campo A., Menabo R., di Marco G., di Lisa F., et al. (2019). Identification of an ATP-sensitive potassium channel in mitochondria. Nature 572, 609–613. doi:10.1038/s41586-019-1498-3
Paucek P., Mironova G., Mahdi F., Beavis A. D., Woldegiorgis G., Garlid K. D. (1992). Reconstitution and partial purification of the glibenclamide-sensitive, ATP-dependent K+ channel from rat liver and beef heart mitochondria. J. Biol. Chem. 267, 26062–26069. doi:10.1016/s0021-9258(18)35717-x
Percie du Sert N., Hurst V., Ahluwalia A., Alam S., Avey M. T., Baker M., et al. (2020). The ARRIVE guidelines 2.0: Updated guidelines for reporting animal research. PLoS Biol. 18, e3000410. doi:10.1371/journal.pbio.3000410
Quayle J. M., Nelson M. T., Standen N. B. (1997). ATP-sensitive and inwardly rectifying potassium channels in smooth muscle. Physiol. Rev. 77, 1165–1232. doi:10.1152/physrev.1997.77.4.1165
Quinn K. V., Giblin J. P., Tinker A. (2004). Multisite phosphorylation mechanism for protein kinase A activation of the smooth muscle ATP-sensitive K+ channel. Circ. Res. 94, 1359–1366. doi:10.1161/01.RES.0000128513.34817.c4
Sato K., Chino D., Kobayashi T., Obara K., Miyauchi S., Tanaka Y. (2013). Selective and potent inhibitory effect of docosahexaenoic acid (DHA) on U46619-induced contraction in rat aorta. J. Smooth Muscle Res. 49, 63–77. doi:10.1540/jsmr.49.63
Sato K., Chino D., Nishioka N., Kanai K., Aoki M., Obara K., et al. (2014). Pharmacological evidence showing significant roles for potassium channels and CYP epoxygenase metabolites in the relaxant effects of docosahexaenoic acid on the rat aorta contracted with U46619. Biol. Pharm. Bull. 37, 394–403. doi:10.1248/bpb.b13-00746
Schwanstecher M., Sieverding C., Dorschner H., Gross I., Aguilar-Bryan L., Schwanstecher C., et al. (1998). Potassium channel openers require ATP to bind to and act through sulfonylurea receptors. EMBO J. 17, 5529–5535. doi:10.1093/emboj/17.19.5529
Shimokawa H., Godo S. (2016). Diverse functions of endothelial NO synthases system: NO and EDH. J. Cardiovasc. Pharmacol. 67, 361–366. doi:10.1097/FJC.0000000000000348
Singh T. U., Kathirvel K., Choudhury S., Garg S. K., Mishra S. K. (2010). Eicosapentaenoic acid-induced endothelium-dependent and -independent relaxation of sheep pulmonary artery. Eur. J. Pharmacol. 636, 108–113. doi:10.1016/j.ejphar.2010.02.041
Singhal A., Lanigan J., Storry C., Low S., Birbara T., Lucas A., et al. (2013). Docosahexaenoic acid supplementation, vascular function and risk factors for cardiovascular disease: A randomized controlled trial in young adults. J. Am. Heart Assoc. 2, e000283. doi:10.1161/JAHA.113.000283
Tang Y., Long C. L., Wang R. H., Cui W., Wang H. (2010). Activation of SUR2B/Kir6.1 subtype of adenosine triphosphate-sensitive potassium channel improves pressure overload-induced cardiac remodeling via protecting endothelial function. J. Cardiovasc. Pharmacol. 56, 345–353. doi:10.1097/FJC.0b013e3181e6c7b8
Teramoto N. (2006). Pharmacological profile of U-37883A, a channel blocker of smooth muscle-type ATP-sensitive K channels. Cardiovasc. Drug Rev. 24, 25–32. doi:10.1111/j.1527-3466.2006.00025.x
Tomoda T., Yunoki T., Naito S., Ito Y., Teramoto N. (2005). Multiple actions of U-37883A, an ATP-sensitive K+ channel blocker, on membrane currents in pig urethra. Eur. J. Pharmacol. 524, 1–10. doi:10.1016/j.ejphar.2005.08.048
Touyz R. M., Alves-Lopes R., Rios F. J., Camargo L. L., Anagnostopoulou A., Arner A., et al. (2018). Vascular smooth muscle contraction in hypertension. Cardiovasc. Res. 114, 529–539. doi:10.1093/cvr/cvy023
Villalpando D. M., Navarro R., Del Campo L., Largo C., Munoz D., Tabernero M., et al. (2015). Effect of dietary docosahexaenoic acid supplementation on the participation of vasodilator factors in aorta from orchidectomized rats. PLoS One 10, e0142039. doi:10.1371/journal.pone.0142039
Wang R. X., Chai Q., Lu T., Lee H. C. (2011). Activation of vascular BK channels by docosahexaenoic acid is dependent on cytochrome P450 epoxygenase activity. Cardiovasc. Res. 90, 344–352. doi:10.1093/cvr/cvq411
Yamada M., Isomoto S., Matsumoto S., Kondo C., Shindo T., Horio Y., et al. (1997). Sulphonylurea receptor 2B and Kir6.1 form a sulphonylurea-sensitive but ATP-insensitive K+ channel. J. Physiol. 499 (3), 715–720. doi:10.1113/jphysiol.1997.sp021963
Ye D., Zhang D., Oltman C., Dellsperger K., Lee H. C., Vanrollins M. (2002). Cytochrome p-450 epoxygenase metabolites of docosahexaenoate potently dilate coronary arterioles by activating large-conductance calcium-activated potassium channels. J. Pharmacol. Exp. Ther. 303, 768–776. doi:10.1124/jpet.303.2.768
Keywords: omega-3 fatty-acids, KATP, DHA, EPA, PNU-37883A, potassium channels
Citation: Bercea C, Limbu R, Behnam K, Ng K-E, Aziz Q, Tinker A, Tamagnini F, Cottrell GS and McNeish AJ (2022) Omega-3 polyunsaturated fatty acid-induced vasodilation in mouse aorta and mesenteric arteries is not mediated by ATP-sensitive potassium channels. Front. Physiol. 13:1033216. doi: 10.3389/fphys.2022.1033216
Received: 31 August 2022; Accepted: 30 November 2022;
Published: 15 December 2022.
Edited by:
Luciana Venturini Rossoni, University of São Paulo, BrazilReviewed by:
Fraser D. Russell, University of the Sunshine Coast, AustraliaIbra S. Fancher, University of Delaware, United States
Copyright © 2022 Bercea, Limbu, Behnam, Ng, Aziz, Tinker, Tamagnini, Cottrell and McNeish. This is an open-access article distributed under the terms of the Creative Commons Attribution License (CC BY). The use, distribution or reproduction in other forums is permitted, provided the original author(s) and the copyright owner(s) are credited and that the original publication in this journal is cited, in accordance with accepted academic practice. No use, distribution or reproduction is permitted which does not comply with these terms.
*Correspondence: Cristiana Bercea, c.bercea@reading.ac.uk