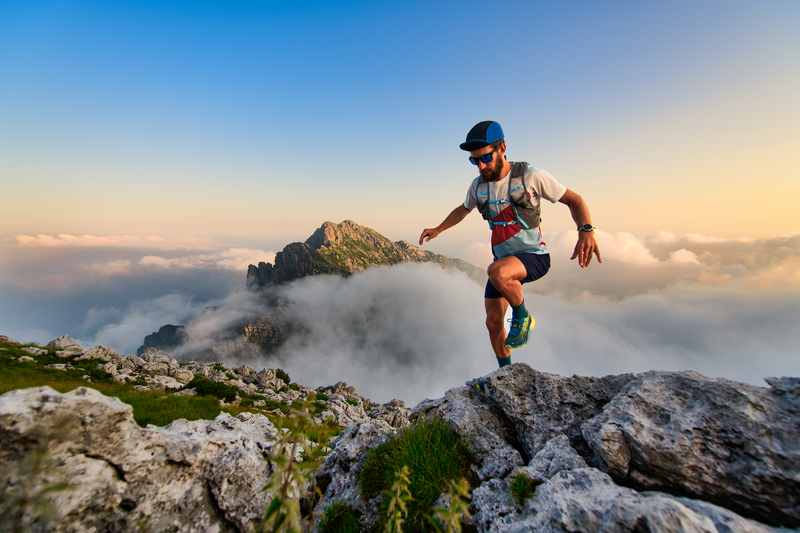
95% of researchers rate our articles as excellent or good
Learn more about the work of our research integrity team to safeguard the quality of each article we publish.
Find out more
ORIGINAL RESEARCH article
Front. Physiol. , 04 January 2023
Sec. Developmental Physiology
Volume 13 - 2022 | https://doi.org/10.3389/fphys.2022.1031950
This article is part of the Research Topic Adverse outcomes of preeclampsia: From mother to baby, pregnancy to postpartum View all 10 articles
Introduction: Preeclampsia (PE) is a gestational hypertensive disease with unclear pathogenesis. This study aimed to identify the genes that play an important role in determining the pathogenesis of PE using bioinformatics analysis and fundamental researches.
Materials and methods: Datasets from the Gene Expression Omnibus (GEO) database were used to screen for differentially expressed genes (DEGs). The NCBI, SangerBox, and other databases were used to analyze the functions of the DEGs. Targetscan7, miRWalk, ENCORI, DIANA TOOLS, CircBank databases, and the Cytoscape tool were used to construct the lncRNA/circRNA-miRNA- LEP network. SRAMP, RPISeq, RBPsuite, and catRPAID were used to analyze the RNA modifications of LEP. Immune cell infiltration was analyzed using the dataset GSE75010. Placental tissues from normal pregnant women and PE patients were collected, screened for gene expression using reverse transcription quantitative polymerase chain reaction (RT-qPCR) and western blotting. The results were further verified in HTR-8/SVneo cell line hypoxia model and PE mouse model.
Results: Our analyses revealed that LEP was significantly upregulated in eight datasets. Kyoto Encyclopedia of Genes and Genomes (KEGG) and Gene Ontology (GO) analyses indicated that LEP was involved in the JAK/STAT signaling pathway, angiogenesis, and placental development. Immune cell infiltration analysis showed that M1 and M2 macrophages differed between normal pregnancies and those in PE patients. A competing endogenous RNA (ceRNA) network was constructed, and proteins interacting with LEP were identified. RNA modification sites of LEP were also identified. Finally, the overexpression of LEP in PE was confirmed in clinical samples, HTR-8/SVneo cell line and PE mouse model.
Conclusion: Our results indicate that LEP overexpression is associated with PE and may be a potential diagnostic marker and therapeutic target.
Preeclampsia (PE) is a hypertensive disease associated with pregnancy and is characterized by new-onset hypertension after 20 weeks of gestation, with or without proteinuria, which can affect multiple organs (listed, 2020). It is characterized by placental dysplasia and endothelial dysfunction (Rana et al., 2019; Han and Dong, 2021). PE is one of the leading causes of maternal, fetal, and neonatal deaths, affecting 2%–8% of pregnancies (Ives et al., 2020). PE can be divided into two subtypes: early-onset (<34 weeks) and late-onset (>34 weeks). Abnormal placental development is more strongly associated with early-onset PE, whereas late-onset PE is usually secondary to maternal microvascular disease or is associated with heredity (Dahlia Raymond and Peterson, 2011). Unfortunately, the pathogenesis of PE remains unclear, and there is no gold standard for treatment, apart from the delivery of the placenta. It is crucial to elucidate the pathogenesis of PE and identify sensitive biomarkers for predicting this disease.
Leptin (encoded by the LEP gene) is a polypeptide hormone secreted primarily by adipose tissue, and the placenta is also the body’s leptin producing tissue. In addition to increased maternal fat mass, placental leptin production is one of the key sources of increased maternal circulating leptin levels. Placental leptin regulates placental functions via autocrine or paracrine signaling, and is considered an essential signaling molecule in the reproductive system. It regulates gonadotropin production, blastocyst formation, implantation, normal placental formation, and communication between the fetus and the placenta. In addition, leptin regulates proliferation, protein synthesis, invasion, and apoptosis of placental cells, and plays a crucial role in the early stages of pregnancy (Perez-Perez et al., 2018). Nonn et al. (2021) reported elevated angiotensin IV (Ang IV) levels in the maternal circulation during pregnancy. Ang IV-induced reduction in basal mitochondrial respiration in trophoblastic cells may alter placental metabolism by increasing leptin levels. This study also suggested that the mechanisms underpinning hypertensive disease in pregnancy may be related to changes in leptin and cellular metabolism (Nonn et al., 2021). Cai et al. found that miR-519d targets LEP and downregulates its expression, promoting the proliferation and migration/invasion of HTR-8/SVneo cells, which may impede the development of PE (Cai et al., 2021). Huang et al. showed that miR-18b-3p was decreased and LEP was increased in placental tissue of PE rats. LEP was the direct target gene of miR-18b-3p. And human umbilical cord mesenchymal stem cells (hucMSCs) upregulated miR-18b-3p and targeted leptin, thereby reducing the levels of inflammatory factors in the placental tissues of PE rats (Huang et al., 2021). In light of these data suggesting that leptin is involved in the development of PE, this study aimed to further analyze LEP expression and explore additional therapeutic targets for PE.
In this study, we identified differentially expressed genes (DEGs) by comparing gene expression profiles in placental tissues from women who experienced normal pregnancy with tissues from PE patients. We then conducted Kyoto Encyclopedia of Genes and Genomes (KEGG) and Gene Ontology (GO) analyses, immune cell infiltration, and protein-protein interaction (PPI) network analyses. We also constructed a competing endogenous RNA (ceRNA) network based on the screened microRNAs (miRNAs), long non-coding RNAs (lncRNAs), and circular RNAs (circRNAs), and predicted the N6-methyladenosine (m6A) modification sites of LEP and related m6A-binding proteins. Clinical samples were collected, and HTR-8/SVneo cell line hypoxia model and PE mouse model were established, and then screened for gene expression using reverse transcription quantitative polymerase chain reaction (RT–qPCR) and western blotting. The identification and analysis of the LEP gene will clarify the role of LEP in the pathophysiology of PE and its potential association with PE, and further understand the pathogenesis of PE. This study aimed to provide more theoretical basis for clarifying that LEP may be a potential diagnostic marker and therapeutic target for PE.
All datasets [GSE10588 (Saei et al., 2021), GSE74341 (Kang et al., 2021), GSE66273 (Qi et al., 2021), GSE54618 (Saei et al., 2021), GSE4707 (Saei et al., 2021), GSE44711 (Kang et al., 2021), GSE35574 (Saei et al., 2021), and GSE24129 (Ma et al., 2021)] containing normal pregnancy and PE placental tissue sequencing data were downloaded from the GEO database (https://www.ncbi.nlm.nih.gov/). We then performed bioinformatic analyses on these data.
To identify DEGs, we sorted the DEGs from all eight datasets (GSE10588, GSE74341, GSE66273, GSE54618, GSE4707, GSE44711, GSE35574, and GSE24129) in ascending order of logFC values. DEGs were selected based on p < 0.05. Differential expression referred to significantly altered (upregulated or downregulated) gene expression at a genomic level. An interactive Venn diagram of the upregulated genes in each dataset was prepared using Evenn (http://www.ehbio.com/test/venn/#/). Simultaneously, omicstudio (https://www.omicstudio.cn/index) was used to plot the volcano maps. Additionally, shinyGEO (https://gdancik.Shinyapps.io/shinyGEO/) was used to quantify LEP expression in each dataset.
To analyze LEP function in PE, NCBI (https://www.ncbi.nlm.nih.gov/) and SangerBox (http://www.sangerbox.com/) were used to perform single-gene KEGG pathway analysis and GO analysis. The Gene Ontology Biological Process (GO_BP), Gene Ontology Molecular Function (GO_MF), and Gene Ontology Cellular Component (GO_CC) terms for LEP were explored.
The GSE75010 (Leavey et al., 2016) dataset was selected to analyze immune cell abundance in samples from 157 patients with either normal pregnancy or that with PE. For the analysis, 80 PE patients were divided into LEP–high and LEP–low expression groups, and gene set enrichment analysis (GSEA) and single sample GSEA (ssGSEA) were performed. CIBERSORT algorithm was used to determine the ratios of the immune cells. The R package “clusterProfiler” was used for GSEA analysis, and ssGSEA analysis was performed using the R package “GSVA".
Upstream binding miRNAs of LEP were predicted using several target gene prediction programs, including miRWalk (http://mirwalk.umm.uni-heidelberg.de/), miRDB (http://mirdb.org/), miRabel (http://bioinfo.univ-rouen.fr/), and TargetScan7 (http://www.targetscan.org/). Only the 24 predicted miRNAs that appeared in all four programs were included in the subsequent analyses. These 24 miRNAs were also scored using TargetScan by entering the human gene symbol “LEP”, followed by each of the 24 miRNA names one at a time, and recording the scores that were obtained. LncRNAs targeting the screened miRNAs were predicted and analyzed using ENCORI (https://starbase.sysu.edu.cn/) and DIANA TOOLS (http://snf-515788.vm.okeanos.grnet.gr/). CircBank (http://www.circbank.cn/searchMiRNA.html) was used to predict the circRNAs. Cytoscape software was used to visualize the lncRNA/circRNA-miRNA-LEP regulatory network.
To construct a PPI network, we performed a search on the STRING website (https://string-db.org/) using “LEP” in the “protein name” module and “Homo sapiens” in the organism module. We set the following key parameters: meaning of network edges (“evidence”), the minimum required interaction score [“medium confidence (0.400)”], and the maximum number of interactors to show (“no more than 20 interactors” in the 1st shell). LEP-binding proteins were also analyzed using GeneMANIA (https://genemania.org/) to determine the interaction between LEP-related proteins. The SangerBox portal was used to perform KEGG and GO analyses of LEP-related genes.
SRAMP (http://www.cuilab.cn/sramp/) was used to predict LEP m6A modification sites and their positions in the RNA secondary structure. The LEP FASTA mRNA sequence without introns was used for this analysis, with the parameters “Analyze RNA secondary structure” and tissue “Generic (default)". The query sequence was shown as RNA. ENCORI (https://starbase.sysu.edu.cn/) and RBPsuite (http://www.csbio.sjtu.edu.cn/bioinf/RBPsuite/) were used to identify proteins that can interact with LEP. RPISeq (http://pridb.gdcb.iastate.edu/RPISeq/) was used to predict the probability of proteins binding to LEP. The protein and RNA sequences were inserted in plain text format, and RF classifier and SVM classifier prediction scores were obtained. RBPsuite (http://www.csbio.sjtu.edu.cn/bioinf/RBPsuite/) and catRPAID (http://service.tartaglialab.com/page/catrapid_group) were used to identify the RNA regions of LEP most likely to be bound by IGF2BP3.
All samples were collected from women who underwent cesarean section at the Department of Obstetrics and Gynecology of the Tianjin Medical University General Hospital between August 2021 and July 2022. This study was approved by the Medical Ethics Committee of the Tianjin Medical University General Hospital and the approval number is IRB2020-KY-008. Samples were collected with verbal informed consent from all patients. The pregnant women were divided into two groups, namely normal pregnancy and PE. The normal pregnancy group comprised pregnant women with no history of hypertension or other clinicopathological changes. Inclusion in the PE group was based on the following criteria: 1) diagnosis of systolic blood pressure of 140 mmHg or higher, or diastolic blood pressure of 90 mmHg or higher, on 2 occasions at least 4 h apart after 20 weeks of gestation; and urine protein levels of 300 mg or more every 24 h, or protein/creatinine ratio 0.3 mg/dL or higher, or test paper reading ++; or 2) in the absence of proteinuria, new-onset hypertension associated with any of the following changes: thrombocytopenia, renal insufficiency, liver function impairment, pulmonary edema, or new-onset headache unresponsive to medication that cannot be explained by other diagnoses or visual symptoms (listed, 2020). Exclusion criteria included multiple pregnancies, hypercoagulable state, gestational diabetes, chronic hypertension, autoimmune diseases, kidney and liver disease, and the use of aspirin or anticoagulants during pregnancy. Placental tissue was extracted immediately after cesarean section and placental villus tissue was dissected at 4°C. After cleaning with cold PBS, samples were quickly stored in liquid nitrogen.
The HTR-8/SVneo cell line was obtained from BeNa Culture Collection (BNCC, Beijing, China). The HTR-8/SVneo cell line was cultured with RPMI-1640 medium (Gibco BRL, Grand Island, NY, United State) supplemented with 10% fetal bovine serum (Gibco, Australia), and antibiotics (100 U/ml penicillin and 100 μg/ml streptomycin). Cells were incubated at 37°C in 5% CO2. For hypoxia, HTR-8/SVneo cell line was cultured in a 3-gas incubator with 1% oxygen, 5% carbon dioxide, serum-free for 24 h to simulate the process of PE.
All mouse experiments were conducted in accordance with protocols approved by the Tianjin medical university animal care and use committee and followed guidelines for animal welfare. Eight-week-old C57BL/6J female and male mice were purchased from Beijing Hufukang Biotechnology Co., LTD. Mating was performed at a 2:1 ratio of male to female. We validated the overexpression of LEP in PE using a PE mouse model constructed by Han et al. The PE mouse model was established by injecting placenta-derived extracellular vesicles (pcEVs), which was obtained from normal pregnant mice, into pregnant C57BL/6J mice to increase circulating pcEV levels, and induced preeclampsia-like changes such as hypertension and proteinuria. In addition, two other models were used to complement this PE mouse model in this study. C57BL/6 J non-pregnant mice developed hypertension and proteinuria after injection of pcEVs to increase circulating pcEV levels. Enhanced clearance of circulating pcEVs of PE pregnant mouse model prevented clinical phenotypes of PE induced by pcEVs (Han et al., 2020). Therefore, we refer to this PE model to validate the overexpression of LEP in PE. In our study, on day 17–18 of gestation, pregnant mice were injected with PBS buffer through the tail vein as the control group or pcEVs as the PE group. Each pregnant mouse was injected with 100 μL. The intervention concentration (1 × 10∧7 pcEVs/mouse) was referenced to the concentration used by (Han et al., 2020). Blood pressure was measured 30 min after injection through the tail vein of the mice. Then, the mice were sacrificed under anesthesia, the placenta and fetus were dissected, and the collected placenta and fetus were weighed.
The detailed steps for obtaining pcEVs are as follows, placentas from normal pregnant C57BL/6J mice between 17, 18 days were washed with ice-cold sterile PBS, cut into small pieces, and frozen in liquid nitrogen. Placentas were gently added to 1 ml PBS and homogenized at 4°C. The placenta homogenates were centrifuged at 1,500 × g for 20 min at 4°C to remove intact cells. The supernatant was centrifuged at 13,000 × g at 4°C for 2 min to remove large cell debris, and then centrifuged at 4°C at 100,000 × g for 60 min (twice) to collect pcEVs and resuspend in PBS (Han et al., 2020).
Total RNA was extracted using the Trizol reagent (Thermo Fisher Scientific, Inc. United State), and cDNA was obtained by reverse transcription using the TransScript® First-Strand cDNA Synthesis SuperMix (Transgen Biotech Corporation, China). The cDNA was amplified using the Hieff UNICON universal Blue qPCR SYBR Green Master Mix kit (YEASEN Corporation, China). The reaction volume was 20 μL, including 10 μL of Universal Blue qPCR SYBR Green Master Mix, 7.6 μL of nucleic acid-free water, 0.2 μL of each primer, and 2 μL of cDNA product. The PCR cycling conditions were as follows: 95°C for 2 min for 1 cycle, 95°C for 10 s, 60°C for 30 s for 40 cycles, followed by the melting curve stage. GAPDH was used as an internal reference and relative mRNA expression was calculated using the 2−ΔΔCT method. The following primer sequences were used for amplification:
GAPDH
Forward: 5′- AAGGTGAAGGTCGGAGTCAAC-3′,
Reverse: 5′- GGGGTCATTGATGGCAACAAT -3′,
LEP
Forward: 5′- TGCCTTCCAGAAACGTGATCC -3′,
Reverse: 5′- CTCTGTGGAGTAGCCTGAAGC -3’.
LINC00473
Forward: 5′- TCATTTCCCTACCTGCTCCT-3′,
Reverse: 5′- CAGTGTCTGCACATCGCTAAT-3’.
Total protein was extracted from each 50 mg placenta sample using 300 μL of protein lysis buffer, which was composed of RIPA buffer (Solarbio, China), PMSF (Solarbio, China), protease inhibitor (MedChemExpress, China), and DNA enzyme inhibitor (Solarbio, China). The total protein concentration was determined using a bicinchoninic acid (BCA) assay (Solarbio, China). Protein samples (30 μg) were electrophoresed on a 15% sodium dodecyl sulfate polyacrylamide gel at 80 V for 30 min and 120 V for 60 min. After electrophoresis, the protein samples were transferred to polyvinylidene fluoride (PVDF) membrane at 80 V, for 100 min, and the blot was washed thrice with TBST for 5 min. After blocking non-specific binding with 5% milk for 2 h at room temperature, the membranes were washed thrice with TBST for 5 min. The membranes were incubated in mouse anti-leptin (Sino Biological, China), rabbit anti-leptin (ABclonal, China) or rabbit anti-GAPDH (Cell Signaling Technology Inc. United State) and mouse anti-β actin (ZSGB-BIO, China) primary antibodies at 4 °C with low agitation overnight. The next day, the primary antibody was recovered and the membrane was washed thrice with TBST for 5 min. The blots were incubated with horseradish peroxidase (HRP)-labeled goat anti-mouse/rabbit IgG (ZSGB-BIO, China) at room temperature for 1 h, then washed thrice with TBST for 10 min. Protein expression was visualized using the chemiluminescence with GAPDH used as a loading control. The relative expression of leptin was calculated as the ratio of the optical density values of leptin to GAPDH. ImageJ was used to measure the gray values.
Statistical and image analyses were performed using GraphPad Prism 8.0 software (GraphPad Software, San Diego, CA, United State). Data are expressed as mean ± standard error of the mean (SEM). Comparisons between two groups were performed using an independent sample t-test. The criterion for statistical significance was set at p < 0.05.
We selected eight datasets of PE data (GSE10588, GSE74341, GSE66273, GSE54618, GSE4707, GSE44711, GSE35574, and GSE24129) from the GEO database, based on the literature. Our analyses revealed that LEP was significantly overexpressed in all eight databases (Figure 1A). LEP expression was higher in the PE group compared with the normal pregnancy group. In datasets GSE74341 and GSE4707, PE was divided into early-onset and late-onset PE, and dataset GSE74341 showed that LEP expression was higher in early-onset PE than in late-onset PE (Figure 1C). And LEP is labeled in the volcano maps of each dataset (p < 0.05) (Figure 1B). Together, these results show that LEP is significantly upregulated in PE.
FIGURE 1. Identification of differentially expressed genes. (A) Venn diagram showing one upregulated gene, LEP. (B) Volcano plot of gene expression profile data in GSE10588, GSE74341, GSE66273, GSE54618, GSE4707, GSE44711, GSE35574, and GSE24129 datasets. (C) The quantity of LEP expression in the GSE10588, GSE74341, GSE66273, GSE54618, GSE4707, GSE44711, GSE35574, and GSE24129 datasets.
Next, we focused on the relationship between LEP and PE development. Single-gene KEGG and GO analyses of LEP were performed to evaluate its biological functions (Tables 1,2). Single-gene KEGG pathway analysis showed that LEP is associated with the Janus kinase/signal transducer and activator of transcription (JAK/STAT) and adipocytokine signaling pathways, HIF-1-alpha transcription factor network, and developmental biology. The GO_BP annotation showed that LEP is involved in angiogenesis, placental development, response to estradiol, regulation of blood pressure, female pregnancy, positive regulation of tyrosine phosphorylation of STAT protein, positive regulation of receptor signaling pathway via JAK/STAT, positive regulation of MAPK cascade, and other biological processes; while GO_CC annotation revealed links to extracellular region, extracellular space, and cytoplasm. The GO_MF annotations were mainly related to peptide receptor binding and hormone activity.
157 samples from GSE75010 were selected to study the infiltration of immune cells into normal pregnancy and PE placental tissues. Among the 22 immune cell types assessed, the number of naive B Cells, resting NK cells, activated NK cells, M1 macrophages, M2 macrophages, and eosinophils was significantly different between the two groups (Figures 2A,B). Next, 80 PE patients were divided into LEP high and low expression groups for GSEA. The results revealed that angiogenesis, placental development, and response to estradiol were enriched (Figure 2C). Concurrent ssGSEA revealed differences in activated dendritic cells, central memory CD8 T Cells, macrophages, memory B Cells, and T follicular helper cells between the two groups (Figure 2D).
FIGURE 2. Placental immune cell infiltration analysis. (A,B) Differences in placental immune cell infiltration between normal pregnancy and PE. (C) A cohort of 80 PE patients were divided into LEP high and low expression groups for GSEA. Angiogenesis: NES: 1.27; FDR: 0.16; p-value: 0.016. Placenta development: NES: 1.51; FDR: 0.13; p-value: 0.007. Response to estradiol: NES: 1.54; FDR: 0.11; p-value: 0.005; (D) ssGSEA of the LEP high and low expression groups, to analyze immune cell infiltration. ns (not significant), * p < 0.05, ** p < 0.01, *** p < 0.001.
We screened the miRWalk, miRDB, TargetScan, and miRabel databases and identified 24 miRNAs that potentially target LEP mRNAs (Figure 3A). These included hsa-miR-212-5p, hsa-miR-4661-3p, hsa-miR-1182, hsa-miR-3936, hsa-miR-1224-3p, hsa-miR-6890-3p, hsa-miR-147a, hsa-miR-5699-5p, hsa-miR-1304-5p, hsa-miR-4683, hsa-miR-668-3p, hsa-miR-4267, hsa-miR-6870-5p, hsa-miR-942-5p, hsa-miR-7855-5p, hsa-miR-3907, hsa-miR-619-5p, hsa-miR-7151-5p, hsa-miR-33a-3p, hsa-miR-6868-3p, hsa-miR-3173-5p, hsa-miR-6756-3p, hsa-miR-1245b-3p, and hsa-miR-5692a. Additionally, these 24 miRNAs were scored (Figure 3B). KEGG and GO analyses were performed on the selected miRNAs to explore their functions (Tables 3,4). KEGG analysis showed significant differences in the relevance of the TGF-beta, PI3K-Akt, MAPK, and JAK/STAT signaling pathways. GO analysis revealed comparisons with the stress-activated MAPK cascade, immune system process, blood coagulation, and in utero embryonic development.
FIGURE 3. Construction of a lncRNA/circRNA-miRNA-LEP regulatory network. (A) The upstream miRNAs of LEP were predicted by miRWalk, miRDB, Targetscan and miRabel databases and the intersecting miRNAs were selected (24 miRNAs). (B) The 24 miRNAs targeting LEP were scored in the Targetscan database. (C) The upstream lncRNAs targeting miRNAs were predicted using the ENCORI and DIANA TOOLS databases and the intersecting lncRNAs were selected (11 lncRNAs). (D) The upstream circRNAs targeting miRNAs were predicted using the ENCORI and CircBank databases and the intersecting circRNAs were selected (6 circRNAs) (E) The lncRNA/circRNA-miRNA-LEP regulatory network was constructed using Cytoscape. (F) RT-qPCR analysis of LINC00473 expression in PE placental tissue samples (n = 10) compared with that in normal pregnancy placental tissue samples (n = 10). * p < 0.05, ** p < 0.01.
We screened the ENCORI and DIANA TOOLS databases and identified 11 lncRNAs that might target miRNAs (Figure 3C), including hsa-miR-147a/LINC00473; hsa-miR-212-5p/LIFR-AS1, EXTL3-AS1, KCNQ1OT1, and NEAT1; hsa-miR-668-3p/SNHG12, TTN-AS1, KCNQ1OT1, NEAT1, MEG3, and FTX; hsa-miR-942-5p/AC159540.1 and LINC01011. We also screened the ENCORI and CircBank databases and identified 6 circRNAs that may target miRNAs, including hsa-miR-147a/hsa_circ_0000175; hsa-miR-212-5p/hsa_circ_0004333, hsa_circ_0075961, hsa_circ_0001699, hsa_circ_0001731, and hsa_circ_0081673 (Figure 3D). Based on the miRNAs, lncRNAs, and circRNAs screened, a ceRNA network containing 4 miRNAs, 11 lncRNAs, and 6 circRNAs was constructed (Figure 3E). In addition, we selected LINC00473 in the ceRNA network to detect its expression; LINC00473 was highly expressed in placentas from preeclamptic women compared with those from normal pregnant women. This finding is consistent with the results of the microarray analysis (Figure 3F). The clinical information of the normal pregnant women and patients with PE is listed in Table 5.
The STRING database analysis identified 20 leptin-binding proteins. As shown in Figure 4A, LEPR, GHRL, GCG, IAPP, PPARG, STAT3, SOCS3, JAK2, NPY, PTPN1, PPARGC1A, CEBPA, CCK, CRP, PRKAA2, PRL, INS, ADIPOQ, RXRA, and PRKAG1 were predicted to interact with leptin. Using GeneMANIA, we constructed a PPI network, which showed that LEPR, HK3, CD33, GHRL, SOCS3, CEBPA, PTPN1, CLU, SH2B1, FABP4, ZBTB17, PTGDS, GRN, IGFBP4, ARNT, PRKAA2, PRKAG2, JAK2, PRKAB2, and MED8 interact with leptin (Figure 4B). We then performed KEGG and GO analyses of the proteins predicted from the STRING database. KEGG analysis showed significant differences in the relevance of the PI3K-Akt and JAK/STAT signaling pathways. GO_BP classification revealed that proteins co-expressed with leptin were mainly involved in placental development, signal transduction, growth hormone signaling pathway via JAK/STAT, receptor signaling pathway via JAK/STAT, leptin-mediated signaling pathway, and female pregnancy. GO_CC annotations were mainly associated with the RNA polymerase II transcription regulator complex (Figures 4C,D). The protein KEGG analysis revealed that STAT3, PRL, SOCS3, LEPR, and JAK2 were associated with leptin in the JAK/STAT signaling pathway, suggesting that leptin may interact with these proteins to activate JAK/STAT signaling in PE. KEGG and GO analyses of leptin-binding proteins from geneMANIA are shown in Supplementary Figure S1.
FIGURE 4. PPI networks exploring leptin-protein interactions. Leptin-binding proteins were identified using (A) the STRING tool, and (B) GeneMANIA. (C,D) KEGG and GO analyses of leptin-binding proteins from the STRING tool.
Using SRAMP, a sequence-based m6A modification site predictor, we identified m6A modification sites in the LEP mRNA sequence, and displayed it on a high-confidence RNA secondary structure (Figures 5A,B). We then used ENCORI and RBPsuite to identify an m6A-modified protein that interacts with LEP, IGF2BP3. Using RPISeq, we predicted the probability of IGF2BP3 associating with LEP. Predictions with probabilities >0.5 are considered “positive,” indicating that the corresponding RNA and protein are likely to interact. For IGF2BP3, the RF classifier score was 0.8, and the SVM classifier score was 0.82 (Figure 5C). The IGF2BP3 motif was analyzed using the RBPsuite online database (Figure 5D). We used catRPAID to screen for LEP RNA regions that were most likely to be bound by IGF2BP3. The results revealed interaction profile peaks around the 500–600 nt site, indicating that IGF2BP3 may bind to LEP at this site (Figure 5E). We also used RBPsuite to explore the binding sites of IGF2BP3 on LEP, and the results indicated that IGF2BP3 may bind LEP at the 1,600–1,800 nt and 500–600 nt sites (Figure 5F).
FIGURE 5. RNA modification of LEP. (A,B) The m6A modification site and its location in the RNA secondary structure (high confidence). (C) m6A-modified proteins that interact with LEP (IGF2BP3) were predicted using ENCORI and RBPsuite. The probability that IGF2BP3 interacts with LEP were predicted using RPISeq, which indicates RF classifier and SVM classifier scores. (D,E) The IGF2BP3 motif was analyzed using RBPsuite and catRPAID to screen for regions of the LEP mRNA most likely to be bound by IGF2BP3. (F) The binding site of IGF2BP3 on LEP was explored with RBPsuite.
To further explore the expression of LEP in PE, we collected placental tissue samples and examined the expression of LEP in these specimens using RT-qPCR. Our results, shown in Figure 6A, reveal that LEP expression in PE placental tissue samples (n = 22) was significantly higher than its expression in normal pregnancy placental tissue samples (n = 21), which is consistent with the bioassay results. To confirm that LEP is upregulated at the protein level, western blotting was used to determine protein expression in placental tissue samples from normal pregnant women (n = 20) and PE patients (n = 23). The clinical information of the normal pregnant women and patients with PE is listed in Table 6. As shown in Figure 6B, leptin protein expression was significantly higher in the placental tissues of patients with PE than in normal pregnant women. In addition, the overexpression of LEP in PE was validated in the HTR-8/SVneo cell line and in a PE mouse model. In the hypoxia model of HTR-8/SVneo cell line, we found that LEP was upregulated at mRNA level under hypoxic conditions by RT-qRCR (Figure 6C). In the mouse model of PE, we found that the blood pressure (systolic and diastolic) in the PE group was significantly higher than that in the PBS group (Figure 6D), and the placental and fetal weights were significantly lower in the PE group than those in the PBS group (Figure 6E). It was also observed in appearance that the PE group was significantly smaller than the PBS group (Figure 6F). Importantly, our results indicate that LEP is overexpressed in the placentas of preeclamptic pregnant mice by western blot (Figure 6G).
FIGURE 6. Validation of LEP/leptin expression in clinical samples, the HTR-8/SVneo cell line, and a PE mouse model. (A) RT-qPCR analysis of LEP expression in PE placental tissue samples (n = 22) compared with normal pregnancy placental tissue samples (n = 21). (B) Western blot analysis of leptin expression in PE placental tissue samples (n = 23) compared with normal pregnancy placental tissue samples (n = 20). (C) RT-qPCR analysis of LEP expression under normal oxygen concentration and hypoxia, control: normal oxygen concentration for 24 h, hypoxia: hypoxia condition for 24 h.(D) Blood pressure in PBS group (n = 5) compared with that in the pcEV group (n = 8). (E) Fetus weight and placental weight in PBS group (n = 47) compared with that in the pcEV group (n = 25). 5 litters in each group. (F) The appearance of fetus and placenta in PBS group compared with that in the pcEV group. (G) Western blot analysis of leptin expression in PBS group (n = 5) compared with that in the pcEV group (n = 8). * p < 0.05, ** p < 0.01, *** p < 0.001, **** p < 0.0001.
In this study, a screen of datasets in the GEO database revealed that LEP is significantly upregulated in PE. Using TargetScan, miWalk, miRDB, miRabel, ENCORI, CircBank, and other websites to identify LEP-targeting miRNAs, lncRNAs, and circRNAs, we constructed a lncRNA/circRNA-miRNA-LEP regulatory network that may regulate the abnormal expression of LEP in PE. We also performed single-gene KEGG, GO, and immune cell infiltration analyses, and by constructing a PPI network, we explored proteins interacting with LEP and their associated pathways and biological functions. We predicted m6A modification sites of LEP and related m6A-binding proteins, and importantly, we verified the overexpression of LEP mRNA and protein levels in clinical samples, the HTR-8/SVneo cell line and a PE mouse model using RT-qPCR and western blotting.
Leptin expression is higher in women experiencing normal pregnancies than in non-pregnant women, suggesting that leptin supports implantation and placental growth. Serum leptin levels increase weeks or months before the onset of PE, which may indicate that leptin itself is involved in the early-onset of PE. And studies have shown that serum leptin levels can be used as a biomarker to distinguish between early- and late-onset PE (Taylor et al., 2015; Hao et al., 2020; Liu et al., 2020). Leptin plays several roles in the regulation of pregnancy-related functions, while metabolic disorders and dynamic imbalances related to leptin during pregnancy play a decisive role in the occurrence and development of PE (de Knegt et al., 2021). Furthermore, using rat models, Ibrahim et al. proved that leptin can increase blood pressure and affect endothelial function, exhibiting pro-inflammatory properties (Ibrahim et al., 2013). In this study, we found that LEP was highly expressed in patients with PE, and we speculate that LEP may be a potential biomarker for the prevention, diagnosis, and treatment of PE. In the subsequent study, we can use siRNA or plasmid to knock down or over-express LEP in HTR-8/SVneo cell line, and experimentally verify whether it will change the migration and invasion ability of HTR-8/SVneo cells. At present, impaired trophoblast cell migration and invasion ability is considered to be one of the important mechanisms in the development of PE (Jiang et al., 2020). Thus, the role of LEP in the occurrence and development of preeclampsia was explored by targeting LEP.
The JAK/STAT pathway is a major intracellular signal transduction pathway. It is a critical downstream regulator of cytokines, hormones, and growth factors. Four members of the JAK family (JAK1, JAK2, JAK3, and TYK2) and seven members of the STAT family (STAT1-4, STAT5A/B, and STAT6) have been identified in mammals. Depending on the cytokine or growth factor that stimulates signaling, different combinations of JAKs and STATs are activated with a high degree of specificity (Dodington et al., 2018). At present, JAK/STAT signaling pathway has been studied in PE. Qu et al. found that hypoxia-inducible factor (HIF)-3α regulates the growth of EVT by up-regulating Fms-like tyrosine kinase receptor (Flt) 1/JAK/STAT signaling during hypoxia, which affects the progression of PE (Qu et al., 2021). However, there are few studies on the involvement of LEP in JAK/STAT signaling in PE. Previous studies have shown that leptin regulates placental amino acid transport by activating JAK/STAT signaling (JAK2 or STAT3) (Alijotas-Reig et al., 2017). STAT3 may play an important role in mediating trophoblast invasion (Zhang et al., 2018). Therefore, LEP may be involved in the development of PE through the JAK/STAT signaling pathway. In this study, the LEP single gene, miRNA, and PPI network-related gene KEGG analyses all showed that LEP might be associated with JAK/STAT signaling in PE. Therefore, we speculate that LEP may play an important role in the genesis and development of PE via this signaling pathway, which is a hypothesis worthy of further investigation.
Previous studies report that JAK/STAT signaling pathway has a certain relationship with immune infiltration. Wang et al. demonstrated that levamisole (LMS) inhibited T-cell activation and downregulated related molecules by inhibiting the activation of JAK/STAT signaling pathway (Wang et al., 2022). Zhou et al. found that the expansion of renal CD8+TRM cells may mediate and maintain renal inflammation and injury in lupus nephritis (LN), and the maintenance of renal CD8+TRM cell effector function depends on JAK/STAT signaling in LN kidneys (Zhou et al., 2020). All these studies have shown that JAK/STAT signaling pathway can regulate the occurrence and development of diseases by acting on immune cells through different signaling molecules. Therefore, we performed an immune cell infiltration assay in the hope that further studies can be conducted. An abnormal response of the maternal immune system to the placenta may be the first pathogenic step in PE, followed by a systemic inflammatory response involving the endothelium (Rambaldi et al., 2019). It is well known that not only does the number of macrophages change in patients with PE, but they also have a different state of polarization compared to patients with normal pregnancy. The total number of macrophages in the placenta of PE patients increased, while the number of M1 and M2 macrophages increased and decreased, respectively (Yao et al., 2019). Ji et al. showed that chemerin, by activating the CMKLR1/Akt/CEBPα axis, promotes the polarization of macrophages to an M1 subtype and inhibits the migration, invasion, and angiogenesis of trophoblast cells, thus participating in the initiation and development of PE (Ji et al., 2021). This finding is consistent with our results from the database screen. Our analysis of immune cell infiltration showed an increased percentage of M1 and a decreased percentage of M2 macrophages in PE patients compared to normal pregnant women. There were also differences in macrophages between the high and low LEP expression groups, suggesting that the level of LEP expression may be related to the change of macrophage infiltration in placenta. In our analysis of GSE75010, the expression of some other immune cells also changed. Our results suggest that the differences in immune cell infiltration in PE placentas may be related to differences in LEP expression.
The ceRNA networks constructed using bioinformatic tools are useful for exploring the role of mRNAs in disease. Recent studies have shown that lncRNAs and circRNAs can positively or negatively regulate miRNAs to influence the expression of downstream mRNAs and play an important role in the development of PE (Chen, 2016; Song et al., 2017). Zhang et al. constructed a lncRNA-related ceRNA network that regulates the expression of key genes in early-onset PE, including 21 lncRNAs, 3 mRNAs, and 69 miRNAs (Zhang et al., 2020). In their study, Yu et al. revealed that SNHG16 expression is downregulated in PE placentas. SNHG16 regulates trophoblast cell migration and invasion via the miR-218-5p/LASP1 axis (Yu et al., 2021). Data from Ou et al. confirmed that hsa_circ_0111277 is upregulated in PE placenta, and that circ_0111,277 acted as a sponge for hsa-miR-494-3p in trophoblast cells by regulating the HTRA1/notch-1 signaling pathway, which inhibited the migration and invasion of these cells (Ou et al., 2020). However, there are still relatively few studies that have focused on the lncRNA/circRNA-miRNA-mRNA regulatory networks in PE. Therefore, we investigated lncRNA/circRNA-miRNA-LEP networks that may regulate LEP expression in PE, which is a likely target for developing new therapeutic strategies for PE. However, although our study describes a lncRNA/circRNA-miRNA-LEP regulatory network in PE, there is still a lack of studies on the pathological process of PE regulated by the lncRNA/circRNA-miRNA-LEP regulatory network, which may be a new challenge.
The m6A RNA modification is the most common internal modification in eukaryotic genes and plays a unique role in regulating mRNA metabolism, including mRNA splicing, output, localization, translation, and stability. M6A-modified mRNA also plays a vital role in many biological processes such as embryonic development, cell proliferation, and tumor formation (Zhou et al., 2021). Recently, studies on the m6A modification of RNA have become more extensive. Hou et al. found that LINC00460/DHX9/IGF2BP2 complex may regulate the expression of high mobility group AT-hook 1 (HMGA1) by recognizing the m6A modification site of HMGA1, thereby enhancing its mRNA stability and promoting the metastasis of colorectal cancer (Hou et al., 2021). Zhang et al. found that IGF2BP1 recognized and stabilized the mRNA of PEG10 in an m6A-dependent manner, enhancing the expression of PEG10, thereby accelerating the cell cycle and promoting EC progression (Zhang et al., 2021). Gu et al. found that increased METTL3 expression and m6A RNA methylation were associated with increased HNRNPC1/C2 expression in placental trophoblasts in PE, suggesting that abnormal m6A modification may be one of the causes of trophoblast cell dysfunction in PE (Gu et al., 2021). Guo et al. found that ALKBH5 which is an m6A demethylase was significantly upregulated, and PPARG expression downregulated in PE placentas. ALKBH5 interference reduced m6A levels on PPARG, increased the stability of PPARG, and promoted PPARG translation. Moreover, ALKBH5 interference significantly promoted the proliferation, migration, and epithelial-to-mesenchymal transition of HTR-8/SVneo cells, as well as the inhibition of apoptosis and oxidative stress (Guo et al., 2022). Wang et al. found that HSPA1A may be involved in the pathophysiology of PE, and showed that m6A modification significantly upregulated the expression of HSPA1A and its protein, suggesting that m6A plays a key role in gene expression regulation and is involved in the pathophysiological processes underpinning PE (Wang et al., 2020). Furthermore, studies have indicated that m6A may play an important role in blood pressure regulation (Mo et al., 2019). Based on numerous studies of m6A in PE and other diseases, we sought to explore whether m6A affects the translational stability of LEP in PE. Currently, few studies which have focused on the RNA modification of LEP in PE, and our study found that there is an m6A modification site on LEP, as well as an m6A binding protein, IGF2BP3, that may interact with LEP. RNA modification often relies on consensus motifs to form secondary structures that bind to RNA-modifying proteins known as writers, readers, and erasers. Therefore, the IGF2BP3 motif was analyzed. In addition, we found that IGF2BP3 is likely to bind LEP at the 500–600 nt site. Interestingly, the effect of m6A modification on targeted mRNAs depends primarily on the different m6A binding proteins. A study by Wang et al. revealed that IGF2BP3 affects the stability of TMBIM6 by participating in the m6A modification of TMBIM6 (Wang et al., 2021). Therefore, our study suggests that IGF2BP3 may bind LEP mRNAs to influence the development of PE. We hypothesize that RNA modification of LEP might stabilize the transcript and promote the expression of leptin.
Notably, the expression of LEP/leptin, and LINC00473 in clinical PE tissues were detected by both RT-qPCR and western blot analyses. We confirmed that the expression of LEP/leptin, LINC00473 in PE was significantly higher than that in normal pregnancy. Together, these data confirmed that overexpression LEP/leptin may indeed play a role in PE. In addition, the high expression of LEP in PE was verified in both the hypoxic HTR-8/SVneo cell line and the PE mouse model. By investigating the expression of LEP in PE in vitro and in vivo, we further found that LEP may play a role in the pathogenesis of PE. The study by Han et al. (2020) successfully generated a mouse model of PE. Injection of pcEVs into pregnant C57BL/6J mice to increase circulating pcEV levels leads to preeclampsia-like changes such as hypertension, proteinuria, and other pathological changes such as vascular injury and constriction. Our results also showed that pregnant C57BL/6J female mice with increased circulating pcEVs by pcEVs injection developed hypertension and fetal growth restriction. These provided a basis for us to validate the high expression of LEP in PE using this PE mouse model. However, this study does have some limitations. Although our investigations involved related pathway analyses, we didn’t conduct in-depth research or further study on the predicted miRNAs, lncRNAs, and circRNAs. Further in vitro and in vivo experiments are needed to explore whether the upstream predicted lncRNAs or circRNAs binds to miRNAs and whether miRNAs binds to LEP, and then to investigate the regulatory effect of upstream non-coding RNAs (ncRNAs) on LEP in the case of knockdown or overexpression, and its effect on the occurrence and development of PE. It would be worthwhile exploring these regulatory RNAs in future studies to better understand the etiology and pathological mechanisms of PE. In addition, validation of LEP as a possible biomarker for PE by using clinical samples collected in the third trimester with a clear diagnosis of normal pregnancy or PE at the time of collection has certain limitations. This requires us to conduct prospective studies, such as examining the levels of LEP in peripheral blood during pregnancy, to predict PE.
In this study, database screening identified the LEP gene to be upregulated in PE and bioinformatics tools were used to predict the corresponding miRNAs, lncRNAs, and circRNAs, and construct a lncRNA/circRNA-miRNA-LEP regulatory network. We then investigated the function of LEP by KEGG, GO, and immune cell infiltration analyses, in addition to predicting m6A modification sites and corresponding binding proteins. Finally, we verified the high expression of LEP in clinical samples, the hypoxic HTR-8/SVneo cell line and the PE mouse model at an mRNA and protein level. These data lay the foundation for further research on the role of leptin in the pathogenesis of PE, which could lead to a better theoretical basis for predicting, preventing, and treating PE in clinical settings.
The datasets presented in this study can be found in online repositories. The names of the repository/repositories and accession number(s) can be found in the article/Supplementary Material.
The studies involving human participants were reviewed and approved by the Medical Ethics Committee of the Tianjin Medical University General Hospital. Written informed consent for participation was not required for this study in accordance with the national legislation and the institutional requirements.
YW analyzed the data, conducted experiments, recruited patients, collected samples, and drafted the manuscript. XB and XG collected the samples and analyzed the data. XLG, YC, HL, and WF analyzed the data. CH developed the hypothesis, designed the study, and drafted the manuscript.
This work was supported by Grant 82071674 and 82101772 from the Natural Science Foundation of China; and Grant 20JCYBJC00440 from the Natural Science Foundation of Tianjin Municipal Science and Technology. The work was funded by Tianjin Key Medical Discipline (Specialty) Construction Project (TJYXZDXK-031A).
The authors declare that the research was conducted in the absence of any commercial or financial relationships that could be construed as a potential conflict of interest.
All claims expressed in this article are solely those of the authors and do not necessarily represent those of their affiliated organizations, or those of the publisher, the editors and the reviewers. Any product that may be evaluated in this article, or claim that may be made by its manufacturer, is not guaranteed or endorsed by the publisher.
The Supplementary Material for this article can be found online at: https://www.frontiersin.org/articles/10.3389/fphys.2022.1031950/full#supplementary-material
Alijotas-Reig J., Esteve-Valverde E., Ferrer-Oliveras R., Llurba E., Gris J. M. (2017). Tumor necrosis factor-alpha and pregnancy: Focus on biologics. An updated and comprehensive review. Clin. Rev. Allergy Immunol. 53 (1), 40–53. doi:10.1007/s12016-016-8596-x
Cai H., Li D., Wu J., Shi C. (2021). miR-519d downregulates LEP expression to inhibit preeclampsia development. Open Med. (Wars) 16 (1), 1215–1227. doi:10.1515/med-2021-0244
Chen L. L. (2016). Linking long noncoding RNA localization and function. Trends Biochem. Sci. 41 (9), 761–772. doi:10.1016/j.tibs.2016.07.003
Dahlia Raymond B. M. B. S., Peterson M. D. (2011). A critical review of early-onset and late-onset preeclampsia. Obstet. Gynecol. Surv. 66 (8), 497–506. doi:10.1097/OGX.0b013e3182331028
de Knegt V. E., Hedley P. L., Kanters J. K., Thagaard I. N., Krebs L., Christiansen M., et al. (2021). The role of leptin in fetal growth during pre-eclampsia. Int. J. Mol. Sci. 22 (9), 4569. doi:10.3390/ijms22094569
Dodington D. W., Desai H. R., Woo M. (2018). JAK/STAT - emerging players in metabolism. Trends Endocrinol. Metab. 29 (1), 55–65. doi:10.1016/j.tem.2017.11.001
Gu Y., Chu X., Morgan J. A., Lewis D. F., Wang Y. (2021). Upregulation of METTL3 expression and m6A RNA methylation in placental trophoblasts in preeclampsia. Placenta 103, 43–49. doi:10.1016/j.placenta.2020.10.016
Guo Y., Song W., Yang Y. (2022). Inhibition of ALKBH5-mediated m(6) A modification of PPARG mRNA alleviates H/R-induced oxidative stress and apoptosis in placenta trophoblast. Environ. Toxicol. 37 (4), 910–924. doi:10.1002/tox.23454
Han C., Dong J. F. (2021). Saving placental thrombomodulin. Blood 137 (7), 873–874. doi:10.1182/blood.2020008659
Han C., Wang C., Chen Y., Wang J., Xu X., Hilton T., et al. (2020). Placenta-derived extracellular vesicles induce preeclampsia in mouse models. Haematologica 105 (6), 1686–1694. doi:10.3324/haematol.2019.226209
Hao S., You J., Chen L., Zhao H., Huang Y., Zheng L., et al. (2020). Changes in pregnancy-related serum biomarkers early in gestation are associated with later development of preeclampsia. PLoS One 15 (3), e0230000. doi:10.1371/journal.pone.0230000
Hou P., Meng S., Li M., Lin T., Chu S., Li Z., et al. (2021). LINC00460/DHX9/IGF2BP2 complex promotes colorectal cancer proliferation and metastasis by mediating HMGA1 mRNA stability depending on m6A modification. J. Exp. Clin. Cancer Res. 40 (1), 52. doi:10.1186/s13046-021-01857-2
Huang Q., Gong M., Tan T., Lin Y., Bao Y., Fan C. (2021). Retraction note: Human umbilical cord mesenchymal stem cells-derived exosomal MicroRNA-18b-3p inhibits the occurrence of preeclampsia by targeting LEP. Nanoscale Res. Lett. 16 (1), 119. doi:10.1186/s11671-022-03765-6
Ibrahim H. S., Omar E., Froemming G. R., Singh H. J. (2013). Leptin increases blood pressure and markers of endothelial activation during pregnancy in rats. Biomed. Res. Int. 2013, 298401. doi:10.1155/2013/298401
Ives C. W., Sinkey R., Rajapreyar I., Tita A. T. N., Oparil S. (2020). Preeclampsia-pathophysiology and clinical presentations: JACC state-of-the-art review. J. Am. Coll. Cardiol. 76 (14), 1690–1702. doi:10.1016/j.jacc.2020.08.014
Ji Z. S., Jiang H., Xie Y., Wei Q. P., Yin X. F., Ye J. H., et al. (2021). Chemerin promotes the pathogenesis of preeclampsia by activating CMKLR1/p-Akt/CEBPɑ axis and inducing M1 macrophage polarization. Cell Biol. Toxicol. 1, 611–628. doi:10.1007/s10565-021-09636-7
Jiang S., Chen Q., Liu H., Gao Y., Yang X., Ren Z., et al. (2020). Preeclampsia-associated lncRNA INHBA-AS1 regulates the proliferation, invasion, and migration of placental trophoblast cells. Mol. Ther. Nucleic Acids 22, 684–695. doi:10.1016/j.omtn.2020.09.033
Kang Q., Li W., Xiao J., Yu N., Fan L., Sha M., et al. (2021). Identification of potential crucial genes associated with early-onset preeclampsia via bioinformatic analysis. Pregnancy Hypertens. 24, 27–36. doi:10.1016/j.preghy.2021.02.007
Leavey K., Benton S. J., Grynspan D., Kingdom J. C., Bainbridge S. A., Cox B. J. (2016). Unsupervised placental gene expression profiling identifies clinically relevant subclasses of human preeclampsia. Hypertension 68 (1), 137–147. doi:10.1161/HYPERTENSIONAHA.116.07293
listed Na (2020). Gestational hypertension and preeclampsia: ACOG practice bulletin, number 222. Obstetrics Gynecol. 135 (6), e237–e60.
Liu J., Song G., Meng T., Zhao G. (2020). Identification of differentially expressed genes and signaling pathways in placenta tissue of early-onset and late-onset pre-eclamptic pregnancies by integrated bioinformatics analysis. Med. Sci. Monit. 26, e921997. doi:10.12659/MSM.921997
Ma B., Zhao H., Gong L., Xiao X., Zhou Q., Lu H., et al. (2021). Differentially expressed circular RNAs and the competing endogenous RNA network associated with preeclampsia. Placenta 103, 232–241. doi:10.1016/j.placenta.2020.10.010
Mo X. B., Lei S. F., Zhang Y. H., Zhang H. (2019). Examination of the associations between m(6)A-associated single-nucleotide polymorphisms and blood pressure. Hypertens. Res. 42 (10), 1582–1589. doi:10.1038/s41440-019-0277-8
Nonn O., Fischer C., Geisberger S., El-Heliebi A., Kroneis T., Forstner D., et al. (2021). Maternal angiotensin increases placental leptin in early gestation via an alternative renin-angiotensin system pathway: Suggesting a link to preeclampsia. Hypertension 77 (5), 1723–1736. doi:10.1161/HYPERTENSIONAHA.120.16425
Ou Y., Zhu L., Wei X., Bai S., Chen M., Chen H., et al. (2020). Circular RNA circ_0111277 attenuates human trophoblast cell invasion and migration by regulating miR-494/HTRA1/Notch-1 signal pathway in pre-eclampsia. Cell Death Dis. 11 (6), 479. doi:10.1038/s41419-020-2679-6
Perez-Perez A., Toro A., Vilarino-Garcia T., Maymo J., Guadix P., Duenas J. L., et al. (2018). Leptin action in normal and pathological pregnancies. J. Cell Mol. Med. 22 (2), 716–727. doi:10.1111/jcmm.13369
Qi H., Yao C., Xing J., Qin Y. (2021). Hypoxia-induced GPR4 suppresses trophoblast cell migration and proliferation through the MAPK signaling pathway. Reprod. Toxicol. 99, 1–8. doi:10.1016/j.reprotox.2020.11.001
Qu H., Yu Q., Jia B., Zhou W., Zhang Y., Mu L. (2021). HIF‑3α affects preeclampsia development by regulating EVT growth via activation of the Flt‑1/JAK/STAT signaling pathway in hypoxia. Mol. Med. Rep. 23 (1), 68. doi:10.3892/mmr.2020.11701
Rambaldi M. P., Weiner E., Mecacci F., Bar J., Petraglia F. (2019). Immunomodulation and preeclampsia. Best. Pract. Res. Clin. Obstet. Gynaecol. 60, 87–96. doi:10.1016/j.bpobgyn.2019.06.005
Rana S., Lemoine E., Granger J. P., Karumanchi S. A. (2019). Preeclampsia: Pathophysiology, challenges, and perspectives. Circ. Res. 124 (7), 1094–1112. doi:10.1161/CIRCRESAHA.118.313276
Saei H., Govahi A., Abiri A., Eghbali M., Abiri M. (2021). Comprehensive transcriptome mining identified the gene expression signature and differentially regulated pathways of the late-onset preeclampsia. Pregnancy Hypertens. 25, 91–102. doi:10.1016/j.preghy.2021.05.007
Song X., Luo X., Gao Q., Wang Y., Gao Q., Long W. (2017). Dysregulation of LncRNAs in placenta and pathogenesis of preeclampsia. Curr. Drug Targets 18 (10), 1165–1170. doi:10.2174/1389450118666170404160000
Taylor B. D., Ness R. B., Olsen J., Hougaard D. M., Skogstrand K., Roberts J. M., et al. (2015). Serum leptin measured in early pregnancy is higher in women with preeclampsia compared with normotensive pregnant women. Hypertension 65 (3), 594–599. doi:10.1161/HYPERTENSIONAHA.114.03979
Wang J., Gao F., Zhao X., Cai Y., Jin H. (2020). Integrated analysis of the transcriptome-wide m6A methylome in preeclampsia and healthy control placentas. PeerJ 8, e9880. doi:10.7717/peerj.9880
Wang J., Liu J., Wang M., Zhao F., Ge M., Liu L., et al. (2022). Levamisole suppresses CD4(+) T-cell proliferation and antigen-presenting cell activation in aplastic anemia by regulating the JAK/STAT and TLR signaling pathways. Front. Immunol. 13, 907808. doi:10.3389/fimmu.2022.907808
Wang X., Tian L., Li Y., Wang J., Yan B., Yang L., et al. (2021). RBM15 facilitates laryngeal squamous cell carcinoma progression by regulating TMBIM6 stability through IGF2BP3 dependent. J. Exp. Clin. Cancer Res. 40 (1), 80. doi:10.1186/s13046-021-01871-4
Yao Y., Xu X. H., Jin L. (2019). Macrophage polarization in physiological and pathological pregnancy. Front. Immunol. 10, 792. doi:10.3389/fimmu.2019.00792
Yu Z., Zhang Y., Zheng H., Gao Q., Wang H. (2021). LncRNA SNHG16 regulates trophoblast functions by the miR-218-5p/LASP1 axis. J. Mol. Histol. 52 (5), 1021–1033. doi:10.1007/s10735-021-09985-x
Zhang L., Wan Y., Zhang Z., Jiang Y., Gu Z., Ma X., et al. (2021). IGF2BP1 overexpression stabilizes PEG10 mRNA in an m6A-dependent manner and promotes endometrial cancer progression. Theranostics 11 (3), 1100–1114. doi:10.7150/thno.49345
Zhang Z., Wang P., Zhang L., Huang C., Gao J., Li Y., et al. (2020). Identification of key genes and long noncoding RNA-associated competing endogenous RNA (ceRNA) networks in early-onset preeclampsia. Biomed. Res. Int. 2020, 1673486. doi:10.1155/2020/1673486
Zhang Z., Wang X., Wang J., Zhang L. (2018). The decreased expression of Stat3 and p-Stat3 in preeclampsia-like rat placenta. J. Mol. Histol. 49 (2), 175–183. doi:10.1007/s10735-018-9757-4
Zhou B., Liu C., Xu L., Yuan Y., Zhao J., Zhao W., et al. (2021). N(6) -methyladenosine reader protein YT521-B homology domain-containing 2 suppresses liver steatosis by regulation of mRNA stability of lipogenic genes. Hepatology 73 (1), 91–103. doi:10.1002/hep.31220
Keywords: leptin, preeclampsia, immune infiltration, bioinformatics, competing endogenous RNA, N 6-methyladenosine
Citation: Wang Y, Bai X, Guo X, Gao X, Chen Y, Li H, Fan W and Han C (2023) Bioinformatics analysis combined with clinical sample screening reveals that leptin may be a biomarker of preeclampsia. Front. Physiol. 13:1031950. doi: 10.3389/fphys.2022.1031950
Received: 30 August 2022; Accepted: 12 December 2022;
Published: 04 January 2023.
Edited by:
Lana McClements, University of Technology Sydney, AustraliaReviewed by:
Yafeng Li, The Fifth Hospital of Shanxi Medical University, ChinaCopyright © 2023 Wang, Bai, Guo, Gao, Chen, Li, Fan and Han. This is an open-access article distributed under the terms of the Creative Commons Attribution License (CC BY). The use, distribution or reproduction in other forums is permitted, provided the original author(s) and the copyright owner(s) are credited and that the original publication in this journal is cited, in accordance with accepted academic practice. No use, distribution or reproduction is permitted which does not comply with these terms.
*Correspondence: Cha Han, dGpoYW5jaGFAdG11LmVkdS5jbg==
†These authors share first authorship
Disclaimer: All claims expressed in this article are solely those of the authors and do not necessarily represent those of their affiliated organizations, or those of the publisher, the editors and the reviewers. Any product that may be evaluated in this article or claim that may be made by its manufacturer is not guaranteed or endorsed by the publisher.
Research integrity at Frontiers
Learn more about the work of our research integrity team to safeguard the quality of each article we publish.