- 1Department of Biological Sciences, Faculty of Pharmaceutical Sciences, Teikyo University, Tokyo, Japan
- 2Department of Molecular Biology, Faculty of Pharmaceutical Sciences, Teikyo University, Tokyo, Japan
Serotonin (5-hydroxytryptamine, 5-HT) is a phylogenetically conserved modulator of numerous aspects of neural functions. Serotonergic neurons in the dorsal and median raphe nucleus provide ascending innervation to the entire forebrain and midbrain. Another important neural modulatory system exists in the midbrain, the dopaminergic system, which is associated to reward processing and motivation control. Dopaminergic neurons are distributed and clustered in the brain, classically designated as groups A8–A16. Among them, groups A8–A10 associated with reward processing and motivation control are located in the midbrain and projected to the forebrain. Recently, midbrain dopaminergic neurons were shown to be innervated by serotonergic neurons and modulated by 5-HT, with the crosstalk between serotonergic and dopaminergic systems attracting increased attention. In birds, previous studies revealed that midbrain dopaminergic neurons are located in the A8-A10 homologous clusters. However, the detailed distribution of dopaminergic neurons and the crosstalk between serotonergic and dopaminergic systems in the bird are poorly understood. To improve the understanding of the regulation of the dopaminergic by the serotonergic system, we performed in situ hybridization in the chick brainstem. We prepared RNA probes for chick orthologues of dopaminergic neuron-related genes; tyrosine hydroxylase (TH) and dopa decarboxylase (DDC), noradrenaline related genes; noradrenaline transporter (NAT) and dopamine beta-hydroxylase (DBH), and serotonin receptor genes; 5-HTR1A, 5-HTR1B, 5-HTR1D, 5-HTR1E, 5-HTR1F, 5-HTR2A, 5-HTR2B, 5-HTR2C, 5-HTR3A, 5-HTR4, 5-HTR5A, and 5-HTR7. We confirmed that the expression of tyrosine hydroxylase (TH) and NAT was well matched in all chick dopaminergic nuclei examined. This supported that the compensation of the function of dopamine transporter (DAT) by NAT is a general property of avian dopaminergic neurons. Furthermore, we showed that 5-HTR1A and 5-HTR1B were expressed in midbrain dopaminergic nuclei, suggesting the serotonergic regulation of the dopaminergic system via these receptors in chicks. Our findings will help us understand the interactions between the dopaminergic and serotonergic systems in birds at the molecular level.
Introduction
Serotonin (5-hydroxytryptamine, 5-HT) is a phylogenetically conserved modulatory neurotransmitter (Marin et al., 2020). Many studies have suggested that the function of 5-HT is prominent in a broad range of processes, including cognition, behavior, and emotion, with these associations being evolutionarily conserved in the animal kingdom (Kandel et al., 2000; Bacque-Cazenave et al., 2020). For example, 5-HTR1B in the nucleus accumbens is essential for the expression of social reward (Liu et al., 2020) and 5-HTR1A knockout mice exhibit elevated anxiety level (O’Leary et al., 2020). Dopamine (DA) is another prominent modulatory neurotransmitter conserved in vertebrates, the functions of which include motor control and motivation-related behavior such as reward and punishment learning (Wise, 2004; Puig et al., 2014). Both the 5-HT and DA systems are involved in processes of motivation-related behavior and interact with each other (Boureau and Dayan, 2011; De Deurwaerdere and Di Giovanni, 2017; Yagishita, 2020; Beyeler et al., 2021; De Deurwaerdere and Di Giovanni, 2021; Peters et al., 2021). To understand the phylogenetic continuity of the neural basis for cognition and emotion, it is necessary to deepen our understanding of the neural circuits that control cognitive and emotional behaviors in animals other than mammals. Birds are excellent model animals to elucidate the evolutionary continuity of the neural basis of cognition and emotion (Rosa Salva et al., 2015; Papini et al., 2019). In birds, the 5-HT system has been suggested to modulate fear-related behavior (Krause et al., 2017; Phi Van et al., 2018; Krause et al., 2019). In addition, the DA system is known to be involved in motor control, learning, and contribution to the acquisition and control of birdsong (Rieke, 1980, Rieke, 1981; Gunturkun, 2005; Fee and Goldberg, 2011). However, the neural circuits that are modulated by 5-HT and DA at a cellular level, and the interactions between the 5-HT and DA systems in avian brains are poorly understood.
In mammals, the dopaminergic neurons are distributed and clustered in the brain, classically designated as cell groups A8-A16 (Dahlstroem and Fuxe, 1964; Bjorklund and Dunnett, 2007). Among them, midbrain dopaminergic neurons cell groups, that is, the retrorubral area (A8), the substantia nigra pars compacta (SNc, A9), and the ventral tegmental area (VTA, A10), have special importance and have been intensively studied in regard to their association with the functions of motor control and motivation-related behavior because they project to a broad area of the forebrain, including the mesolimbic (VTA to nucleus accumbens, amydgala, and hippocampus), mesocortical (VTA to prefrontal cortex), and nigrostriatal (SNc to striatum) projections (Hillarp et al., 1966; Bjorklund and Dunnett, 2007). There is a considerable heterogeneity within and between the nuclei of dopaminergic neurons with respect to multiple aspects, such as connectivity, electrophysiological properties, and molecular and behavioral functions (Lammel et al., 2014; Anderegg et al., 2015; Poulin et al., 2020). In addition, the VTA contains diverse populations of dopaminergic, glutamatergic, and GABAergic neurons, as well as combinatorial neurons that release more than one neurotransmitter and a complex microcircuitry that integrates interactions among local dopaminergic, glutamatergic, and GABAergic neurons (Morales and Margolis, 2017). The midbrain dopaminergic nuclei with such characteristics have been known to receive serotonergic neuron projections from the dorsal raphe (DR) nucleus (Ogawa et al., 2014; Beier et al., 2015; Ogawa and Watabe-Uchida, 2018). The type of serotonergic receptors expressed in dopaminergic neurons and the mechanism by which they are regulated in neural circuit levels has become clear in recent years (Wang et al., 2019; Peters et al., 2021).
In birds, previous studies have revealed that the midbrain dopaminergic neurons are located in the A8–A10 homologous clusters in the midbrain (Ikeda and Goto, 1971; Dube and Parent, 1981; Guglielmone and Panzica, 1984; Moons et al., 1994; Reiner et al., 2004). Moreover, the afferent and efferent connections of the midbrain dopaminergic nuclei in birds are similar in extent to those of mammals (Csillag, 1999; Durstewitz et al., 1999; Mezey and Csillag, 2002; Balint and Csillag, 2007; Balint et al., 2011). In terms of molecular characteristics, the dopaminergic system of birds was also thought to be largely conserved with that of mammals (Yamamoto Vernier, 2011). However, in recent years, sauropsids, including birds and reptiles, were reported to have lost the dopamine transporter (DAT) gene from the genome, with the noradrenaline transporter (NAT) being proposed to compensate for the function of DAT in sauropsids (Lovell et al., 2015). Therefore, the degree of conservation of the bird dopaminergic system with that of the mammalian dopaminergic system needs to be reexamined. Furthermore, in avian brains, the interactions between the dopaminergic and serotonergic systems are still poorly understood.
In the present study, to better understand the midbrain dopamine system in birds and to improve our understanding of the interactions between the dopamine and serotonin systems in birds, we investigated the chick brainstem at the molecular level using in situ hybridization (ISH). We selected chick orthologs of dopaminergic neuron-related marker genes: tyrosine hydroxylase (TH) and dopa decarboxylase (DDC); noradrenaline neuron-related marker genes: noradrenaline transporter (NAT) and dopamine beta-hydroxylase (DBH); and serotonin receptor genes: 5-HTR1A, 5-HTR1B, 5-HTR1D, 5-HTR1E, 5-HTR1F, 5-HTR2A, 5-HTR2B, 5-HTR2C, 5-HTR3A, 5-HTR4, 5-HTR5A, and 5-HTR7. We confirmed that the expression of TH and NAT was well matched in all chick dopaminergic nuclei examined. This supported that the compensation of the function of DAT by NAT is a general property of avian dopaminergic neurons. Furthermore, we found that 5-HTR1A and 5-HTR1B were expressed in dopaminergic nuclei and in cells other than dopaminergic neurons. Understanding the nature of serotonin receptor-expressing cells in avian dopaminergic nuclei will help us understand the serotonergic regulation of the avian dopamine system and elucidate the functional correspondences between the avian and mammalian dopamine systems.
Materials and methods
Animals
Fertilized eggs of domestic chickens of the Cobb strain (Gallus gallus domesticus) were purchased from a local company (3-M, Aichi, Japan). Eggs were incubated at the Teikyo University (Kaga, Itabashi-ku, Tokyo, Japan). Animal experiments were performed as described previously by Yamaguchi et al. (2008a, 2008b). Briefly, newly hatched chicks (P0) were transferred to dark plastic enclosures in a dark warm cage at 30°C for 1 d (P1). We used 10 chick brainstems for the detection of tyrosine hydroxylase (TH), 8 for noradrenaline transporter (NAT), 7 for dopa decarboxylase (DDC), 7 for dopamine beta-hydroxylase (DBH), 5 for 5-HTR1A, 5 for 5-HTR1B, 3 for 5-HTR1D, 3 for 5-HTR1E, 3 for 5-HTR1F, 4 for 5-HTR2A, 3 for 5-HTR2B, 4 for 5-HTR2C, 4 for 5-HTR3A, 3 for 5-HTR4, 3 for 5-HTR5A, 3 for 5-HTR7, and 2 for reverse-transcription polymerase chain reaction (RT-PCR) experiments (Supplementary Table S1). Previous studies showed that embryonic development of the catecholamine system is almost completed before hatching (Guglielmone and Panzica, 1984) and distribution of dopamine neurons does not show difference between ages after hatched (Moons et al., 1994), suggesting that the distribution of dopamine neurons in the brainstem of P1 chick was almost same as that in the adult chick. The expression level of 5-HTRs may change during post-natal development, but this is the issue needs to be addressed in the future. All experimental procedures were reviewed and approved by the Committee on Animal Experiments of Teikyo University and conducted in accordance with the guidelines of the national regulations for animal welfare in Japan.
Histology preparations
First, P1 chicks were anesthetized by an intraperitoneal injection (0.40 ml/individual) of a 1:1 mixture of ketamine (10 mg/ml, Ketalar-10, Sankyo Co., Tokyo, Japan) and xylazine (2 mg/ml, Sigma, St. Louis, MO, United States). Then, anesthetized chicks were transcardially perfused with 4% paraformaldehyde in 0.1 M phosphate buffered saline (pH 7.5, PFA-PBS). After perfusion, whole brains were quickly dissected up to the spinal cord level, immediately immersed in PFA-PBS for 1 day at 4°C and placed in an 18% sucrose/PFA-PBS solution for cryoprotection for 2 day at 4°C. Next, brains with sucrose substitution were embedded in Tissue-Tek OCT compound (Sakura Finetechnical, Tokyo, Japan), frozen promptly on dry ice, and stored at −80°C until sectioning. Frozen brain blocks were cut into 18 µm-thick sections using a cryostat (Leica CM3050S or Leica CM 1850, Leica Biosystems, Nußloch, Germany). We mounted serial sections on glass slide (Platinum PRO, cat# PRO-01, MATSUNAMI Glass Ind., Osaka, Japan). The level of serial coronal sections (A3.4 to A1.0) corresponded to those of the atlas by Kuenzel and Masson (1988).
cDNA cloning and RNA probe preparations
Total RNA was extracted from the chick brain using the TRIzol reagent (Invitrogen, Carlsbad, CA, United States) and reverse-transcribed using the SuperScript III kit (Invitrogen) with oligo (dT) primers, according to the manufacturer’s instructions. RT-PCR was performed using the following gene-specific primer (forward and reverse) pairs: TH: 5′-GCCTCATTGAAGATGCCAGG-3′ and 5′-AGCCAGTCCTCTCTTTCAGG-3′, respectively; NAT: 5′-GAACAAACAGATCCAGCCCG-3′ and 5′-CTTCACGCCTTTCCACAGAC-3′, respectively; DDC: 5′-TGGTGGACTACGTTGCAGAT-3′ and 5′-TTGTCAAAGGAGCAGCAAGG-3′, respectively; DBH: 5′-AGCCAAGAGACGACCTACTG-3′ and 5′-CATTGCTCCTGTTCTCCGTG-3′, respectively. PCR amplicons were subcloned into the pGEM-T easy vector (Promega, Madison, WI, United States). All sequences were confirmed using Sanger sequencing. For 5-HTR1A, 5-HTR1B, 5-HTR1D, 5-HTR1E, 5-HTR1F, 5-HTR2A, 5-HTR2B, 5-HTR2C, 5-HTR3A, 5-HTR4, 5-HTR5A, and 5-HTR7 probes, we used previously constructed plasmids (Fujita et al., 2020; Fujita et al., 2022a) (Supplementary Table S2). Plasmids containing the cDNA fragments for TH, NAT, DDC, DBH, 5-HTR1A, 5-HTR1B, 5-HTR1D, 5-HTR1E, 5-HTR1F, 5-HTR2A, 5-HTR2B, 5-HTR2C, 5-HTR3A, 5-HTR4, 5-HTR5A, and 5-HTR7 were amplified by PCR using the M13 primer pair. Amplicons containing the T7 and SP6 promoter sites were purified using a PCR purification kit (Qiagen, Valencia, CA, United States). Digoxigenin (DIG)-labeled sense and antisense RNA probes were prepared by in vitro transcription using a DIG RNA labeling kit (Roche, Basel, Switzerland). All subclones of 5-HTRs were confirmed by Sanger sequencing (Supplementary Figure S1) and were validated by denaturing RNA electrophoresis using agarose gels (Supplementary Figure S2). For double ISH analysis, TH fluorescein-labeled sense and antisense RNA probes were prepared using a fluorescein RNA labeling kit (Roche) in the same manner as above.
In situ hybridization
ISH experiments were performed as described previously by Fujita et al. (2019), with some modifications. Briefly, brain sections on slide glasses were refixed in 4% PFA-PBS, pretreated, and hybridized with DIG-labeled RNA probes at 70°C. After stringent washes, hybridized probes were detected via an immunohistochemical examination with an alkaline phosphatase-conjugated anti-DIG antibody (1:1000; Roche). For signal visualization, a chromogenic reaction with a nitro blue tetrazolium/5-bromo-4-chloro-3-indolyl phosphate (NBT/BCIP) was performed at 25°C for the following durations: TH, NAT, DDC, and DBH, 15–19 h; 5-HTR1A, 5-HTR1B, 5-HTR1D, and 5-HTR1E, 18.25–18.5 h; 5-HTR2A, 5-HTR2C, and 5-HTR3A, 18.25–18.6 h; 5-HTR1F, 18.25–20 h; 5-HTR2B and 5-HTR5A, 20 h; 5-HTR4, 18.5–18.6 h; and 5-HTR7, 18.5 h. Sense probes were used as negative controls in every experiment.
Double in situ hybridization
Double ISH experiments were performed as described previously by Fujita et al. (2022a). Briefly, during hybridization, DIG-labeled and fluorescein-labeled RNA probes were mixed and hybridized simultaneously. After the first chromogenic reaction with NBT/BCIP, sections on slide glasses were treated with 100 mM glycine (pH 2.2) for antibody detachment. After washing in PBS, fluorescein-labeled probes were detected immunohistochemically using an alkaline phosphatase-conjugated anti-fluorescein antibody (1:1000; Roche). For signal visualization, the second color chromogenic reactions were performed at 25°C using SIGMAFAST Fast Red TR/Naphthol AS-MX tablets (cat#F4523, Sigma-Aldrich, St. Louis, MO, United States). The durations for the first and second reactions were as follows: first reaction, 5-HTR1A or 5-HTR1B, 2–3 h and second reaction, TH, 18–114 h. Sense probes were used as negative controls in every experiment. We only determined clearly double labelled cells as double labelled.
Imaging and data processing
Digital photographs of sections on each slide glass were acquired semiautomatically using NanoZoomer 2.0 HT or NanoZoomer XR systems (Hamamatsu Photonics, Shizuoka, Japan). The microscopic fields of interest were cropped using the NDP. view2 software (ver. 2.7.25, https://www.hamamatsu.com/; Hamamatsu Photonics, Shizuoka, Japan). For ISH imaging, cropped images were converted to 8-bit images, and their brightness and contrast were adjusted using ImageJ (ver. 1.52a, https://imagej.nih.gov/ij/; National Institute of Health, Bethesda, MD, United States). For fluorescent imaging, digital photographs were acquired semiautomatically using the NanoZoomer XR system in the bright and fluorescent modes using a TRITC filter.
Results
Selection of chick orthologues of mammalian dopaminergic and noradrenergic neuron-related genes and 5-HTR genes
To clarify the distribution of DA neurons and the crosstalk between the dopaminergic and serotonergic system in the chick midbrain, we selected chick orthologs of the following mammalian dopaminergic neuron-related genes: TH and DDC, and noradrenergic neuron-related genes: NAT and DBH, as well as all chick 5-HTR genes (5-HTR1A, 5-HTR1B, 5-HTR1D, 5-HTR1E, 5-HTR1F, 5-HTR2A, 5-HTR2B, 5-HTR2C, 5-HTR3A, 5-HTR4, 5-HTR5A, and 5-HTR7; except 5-HTR6, for which we could not obtain a PCR amplicon). The features of dopaminergic and noradrenergic neuron-related genes are as follows: TH is a rate-limiting enzyme for dopamine biosynthesis; DDC is an enzyme necessary for the stepwise production of dopamine, but also involved in a wider range of processes, including serotonin production; NAT is a transporter for noradrenaline reuptake from the synaptic cleft to the neuron that released noradrenaline and is encoded by the solute carrier family 6 member 2 (Slc6a2) gene; and DBH is an enzyme responsible for noradrenaline production from dopamine (Bjorklund and Dunnett, 2007; Yamamoto and Vernier, 2011). We found that orthologs between chicks and humans exhibited the following sequence similarities (protein and DNA): TH, 77.2% and 74.8%, respectively; DDC, 77.4% and 73.5%, respectively; NAT, 85.7% and 76.1%, respectively; DBH, 69% and 73.1%, respectively. We also searched for sequence similarities between 5-HTR genes and genes in other animals in previous studies (Fujita et al., 2020; Fujita et al., 2022a). The information summary of these ortholog gene probes is provided in Table 1 and Supplementary Table S2. We designed probes to detect multiple transcript variants of the orthologs registered in the database. We also performed in situ hybridization (ISH) and analyzed the expression patterns of orthologs in the brainstem of chicks.
Expression of tyrosine hydroxylase, dopa decarboxylase, noradrenaline transporter, and dopamine beta-hydroxylase in the chick brainstem
We performed ISH analysis to reveal the expression patterns of the dopaminergic and noradrenergic neuron-related genes, TH, DDC, NAT, and DBH in neighboring sections around A3.4 to A 1.0 in the brainstems of naive chicks on post-hatch day 1 (P1). Accordingly, we detected cells with strong signals of TH and NAT in the VTA, SNc, GCt, A8, MR, LoC, RPgc, and DR (Figures 1A,B,F,G,K,L,P,Q, Figures 1A′,B′,F′,G′,K′,L′,P′,Q′). We also observed cells with strong signals of DDC in the VTA, SNc, between the VTA and SNc, A8, MR, LoC, and DR (Figures 1C,H,M,R, Figures 1C′,H′,M′,R′). We also found cells with strong signals of DBH in between the A8 and MR, LoC, and between the LoC and RPgc (Figures 1D,I,N, Figures 1D′,I′,N′). As noradrenergic neurons contain dopamine as a synthetic intermediate, noradrenergic neurons express TH, DDC, and DBH, whereas dopaminergic neurons express only TH and DDC but not DBH (Bjorklund and Dunnett, 2007; Yamamoto and Vernier, 2011). In mammals, DAT is considered to be the most accurate single-gene marker gene for dopaminergic neurons (Lemmel et al., 2015). However, in amniotes, including birds, DAT is deleted from the genome, with NAT being suggested to compensate the function of DAT (Lovell et al., 2015). We found that the expression patterns of TH, NAT, and DDC were similar in the SNc, VTA, and A8 in chicks, and further confirmed the absence of DBH signals.
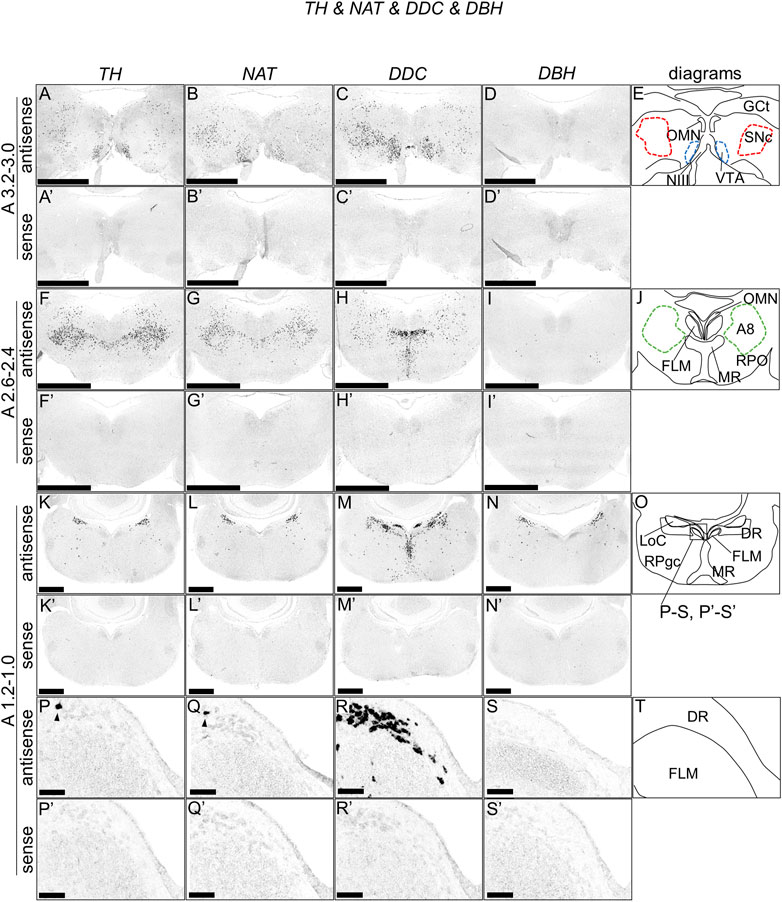
FIGURE 1. In situ hybridization of TH, NAT, DDC, and DBH in the P1 chick brainstem. Digoxigenin-labeled RNA antisense [TH, (A,F,K,P), NAT, (B,G,L,Q), DDC, (C,H,M,R), and DBH, (D,I,N,S)] and sense [TH, (A′,F′,K′,P′), NAT, (B′,G′,L′,Q′), DDC, (C′,H′,M′,R′), and DBH, (D′,I′,N′,S′)] probes were used for in situ hybridization in coronal sections of P1 chick brainstems. To evaluate the expression patterns of TH, NAT, DDC, and DBH, sections of 10 P1 chick brainstems were analyzed for TH, 8 for NAT, 7 for DDC, and 7 for DBH. Representative images of the levels of expression in neighboring sections (A3.2–3.0, A2.6–2.4, and A1.2–1.0) from 3 P1 chick brainstems. (P–S and P′–S′) Magnified views of brainstem areas in the box in (O). The levels of expression in these sections were in accordance with those mentioned in the Kuenzel and Masson’s chick atlas (Kuenzel and Masson, 1988). (E,J,O,T) Diagrams of coronal sections shown in panels (A), (F), (K), and (P). Arrowheads indicate signals. A8: A8 cell group; DR, dorsal raphe; FLM, fasciculus longitudinalis medialis; GCt, griseum centrale; LoC, locus coeruleus; MR, median raphe; NIII, nervus oculomotorius; OMN, oculomotor nucleus; P1, post-hatch day 1; RPgc, nucleus reticularis pontis caudalis; RPO, nucleus reticularis pontis oralis; VTA, ventral tegmental area. Scale bars = 2.5 mm (A–I) and (A′–I′), 1 mm (K–N) and (K′–N′), and 100 µm (P–S) and (P′–S′).
Expression of 5-HTR1A in the chick brainstem
We examined the expression pattern of 5-HTR1A in sections A3.4 to A2.4 in P1 chick brainstems (Figure 2). We detected strong signals in the optic tectum (OT) in a layered manner (Figures 2A,C,A′,C′), nucleus intercolicularis (ICo) (Figures 2A,A′), oculomotor nucleus (OMN) (Figures 2A–D, Figures A′–D′), fasciculus longitudinalis medialis (FLM), and MR (Figures 2C,D,C′,D′), in consistency with our previous study (Fujita et al., 2022a). However, we sparsely detected cells with signals in the SNc, VTA, and A8 (Figures 2C,D,C′,D′).
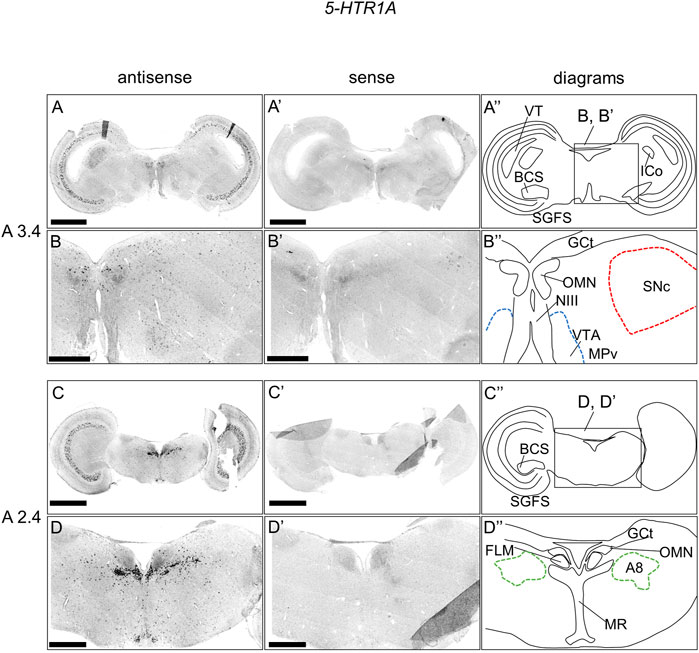
FIGURE 2. In situ hybridization of 5-HTR1A in the P1 chick brainstem. Digoxigenin-labeled RNA antisense (A–D) and sense (A′–D′) 5-HTR1A probes were used for in situ hybridization in coronal sections of P1 chick brainstems. To evaluate the expression patterns of 5-HTR1A, we analyzed sections from five chick brainstems; representative images of chick brainstem sections are shown. (B) and (B′) and (D) and (D′) Magnified views of brain areas in the box in (A′′) and (C′′). (A′′–D′′) Diagrams of coronal sections shown in the rightmost panels. The levels of expression in sections (A 3.4 and A 2.4) were in accordance with those mentioned in the Kuenzel and Masson’s chick atlas (Kuenzel and Masson, 1988). A8: A8 cell group; BCS, brachium colliculi superiors; FLM, fasciculus longitudinalis medialis; GCt, griseum centrale; ICo, nucleus intercolicularis; MR, median raphe; NIII, nervus oculomotorius; OMN, oculomotor nucleus; P1, post-hatch day 1; PPT, pedunculopontine tegmental nucleus; SGFS, stratum griseum et fibrosum superficiale; SNc, substantia nigra pars compacta; VT, ventriculus tecti mesencephalic; VTA, ventral tegmental area. Scale bars = 2.5 mm (A,C) and (A′,C′) and 1 mm (B,D) and (B′,D′).
Expression of 5-HTR1B in the chick brainstem
As with 5-HTR1A, we examined the expression pattern of 5-HTR1B in sections A3.4 to A2.4 in P1 chick brainstems (Figure 3). We detected strong signals in the OT in a layered manner and in a wide area of the brainstem, consistent with our previous findings (Figures 3A–D, Figures 3A′–D′) (Fujita et al., 2022a). In addition, we detected strong signals in the nucleus mesencephalicus proundus pars ventralis (MPv) (Figures 3A,B,A′,B′) and signals in the SNc, VTA, and A8 (Figures 3A–D, Figures 3A′–D′).
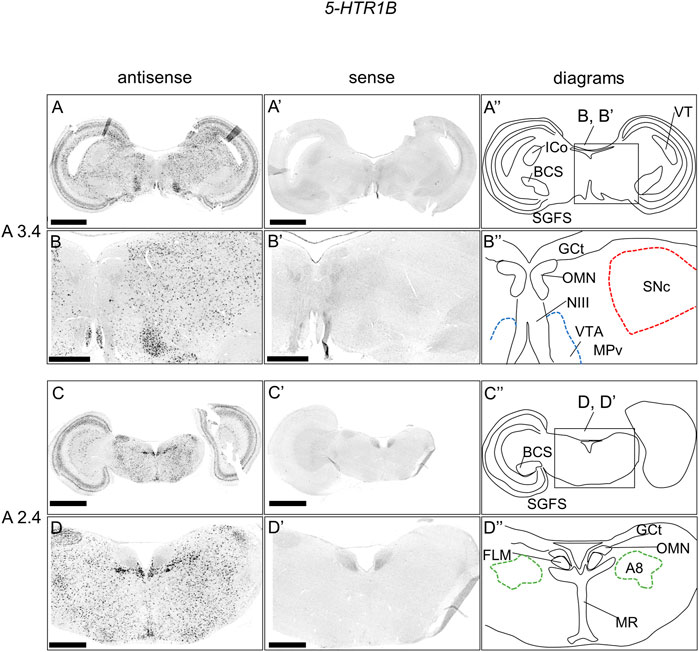
FIGURE 3. In situ hybridization of 5-HTR1B in the P1 chick brainstem. Digoxigenin-labeled RNA antisense (A–D) and sense (A′–D′) 5-HTR1B probes were used for in situ hybridization in coronal sections of P1 chick brainstems. To evaluate the expression patterns of 5-HTR1B, we analyzed sections from five chick brainstems; representative images of chick brainstem sections are shown. (B) and (B′) and (D) and (D′) Magnified views of brain areas in the box in (A′′) and (C′′). (A′′–D′′) Diagrams of coronal sections shown in the rightmost panels. The levels of expression in sections (A 3.4 and A 2.4) were in accordance with those mentioned in the Kuenzel and Masson’s chick atlas (Kuenzel and Masson, 1988). A8: A8 cell group; BCS, brachium colliculi superiors; FLM, fasciculus longitudinalis medialis; GCt, griseum centrale; ICo, nucleus intercolicularis; MR, median raphe; NIII, nervus oculomotorius; OMN, oculomotor nucleus; P1, post-hatch day 1; PPT, pedunculopontine tegmental nucleus; SGFS, stratum griseum et fibrosum superficiale; SNc, substantia nigra pars compacta; VT, ventriculus tecti mesencephalic; VTA, ventral tegmental area. Scale bars = 2.5 mm (A,C) and (A′,C′) and 1 mm (B,D) and (B′,D′).
Expression of 5-HTR1F in the chick brainstem
We also examined the expression of 5-HTR1F in sections of P1 chick brainstems and detected signals in a part of the BCS and a weak layered pattern in the OT (Figures 4A,A′,A′′). When we observed the part of the OT in detail, we detected weak signals in the stratum griseum et fibrosum superficiale (SGFS; layers 5a, 9, and 11) and sparse signals in the stratum griseum periventriculare (SGP, layer 15) (Figures 4B,B′).
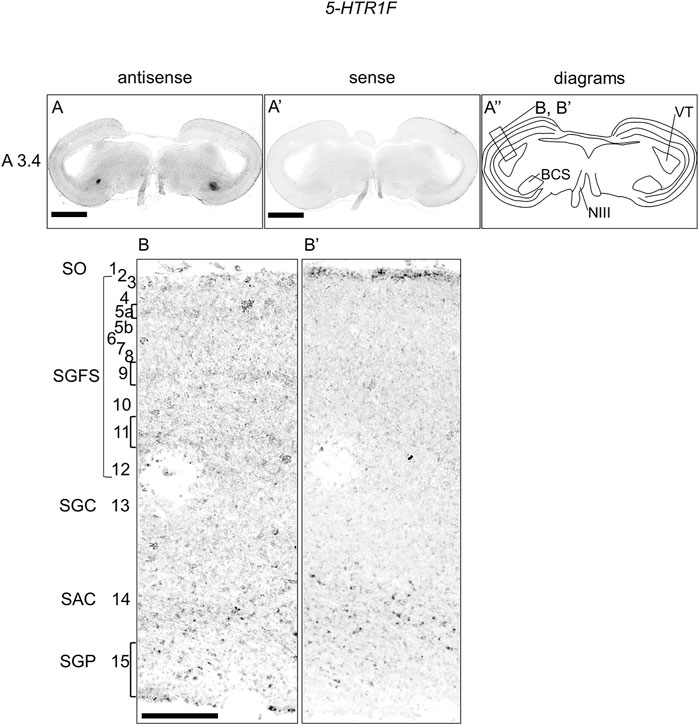
FIGURE 4. In situ hybridization of 5-HTR1F in the P1 chick brainstem. Digoxigenin-labeled RNA antisense (A,B) and sense (A′,B′) 5-HTR1F probes were used for in situ hybridization in coronal sections of P1 chick brainstems. To evaluate the expression patterns of 5-HTR1F, we analyzed sections from three chick brainstems; representative images of chick brain sections are shown. (A′′) Diagrams of coronal sections shown in the rightmost panels. The levels of expression in sections (A3.4) were in accordance with those mentioned in the Kuenzel and Masson’s chick atlas (Kuenzel and Masson, 1988). (B,B′) Magnified views of the regions of optic tectum. Numbers represent tectal layers according to Ramon y Cajal (Ramon y Cajal, 1911). The leftmost alphabetical system of nomenclature was in accordance with that mentioned in the Kuenzel and Masson’s chick atlas (Kuenzel and Masson, 1988). BCS, brachium colliculi superiors; NIII, nervus oculomotorius; P1, post-hatch day 1; SAC, stratum album centrale; SGC, stratum griseum centrale; SGFS, stratum griseum et fibrosum superficiale; SGP, stratum griseum periventriculare; SO, stratum opticum. Scale bars = 2.5 mm (A,A′) and 250 µm (B,B′).
Expression of 5-HTR2A and 5-HTR2C in the chick brainstem
We examined the expression of 5-HTR2A and 5-HTR2C in sections of P1 chick brainstems but did not detect any clear expression patterns (Figures 5A,B,A′,B′,A′′,B′′). However, when we observed the part of the OT in detail, we detected 5-HTR2A signals in the stratum album centrale (layer 14) and a part of SGC (layer 13) (Figures 5C,C′). In addition, we detected 5-HTR2C signals in the SGFS (layer11) and SAC (layer 14) (Figure 5D,D′).
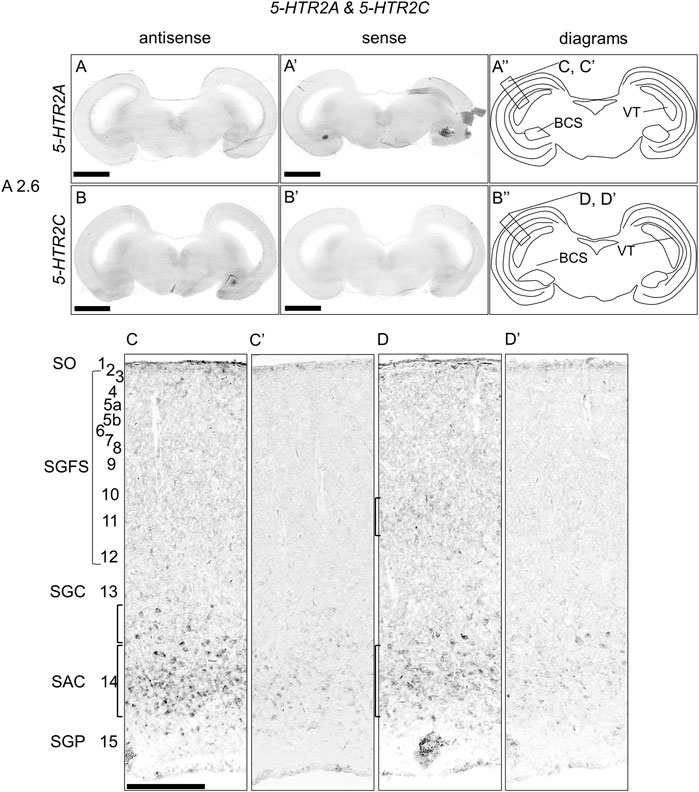
FIGURE 5. In situ hybridization of 5-HTR2A and 5-HTR2C in the P1 chick brainstem. Digoxigenin-labeled RNA antisense [5-HTR2A, (A,C), and 5-HTR2C, (B,D)] and sense [5-HTR2A, (A′,C′), and 5-HTR2C, (B′,D′)] probes were used for in situ hybridization in coronal sections of P1 chick brainstems. To evaluate the expression patterns of 5-HTR2A and 5-HTR2C, we analyzed sections from four chick brainstems for 5-HTR2A and 4 for 5-HTR2C; representative images of chick brainstem sections are shown. (A′′,B′′) Diagrams of coronal sections shown in the rightmost panels. The levels of expression in sections (A2.6) were in accordance with those mentioned in the Kuenzel and Masson’s chick atlas (Kuenzel and Masson, 1988). (C,D) and (C′,D′) Magnified views of the regions of optic tectum. Numbers represent tectal layers according to Ramon y Cajal (Ramon y Cajal, 1911). The leftmost alphabetical system of nomenclature was in accordance with that mentioned in the Kuenzel and Masson’s chick atlas (Kuenzel and Masson, 1988). BCS, brachium colliculi superiors; NIII, nervus oculomotorius; P1, post-hatch day 1; SAC, stratum album centrale; SGC, stratum griseum centrale; SGFS, stratum griseum et fibrosum superficiale; SGP, stratum griseum periventriculare; SO, stratum opticum. Scale bars = 2.5 mm (A,B) and (A′,B′) and 250 µm (C,D) and (C′,D′).
Expression of 5-HTR1D, 5-HTR1E, 5-HTR2B, 5-HTR3A, 5-HTR4, 5-HTR5A, and 5-HTR7 in the chick brainstem
We examined the expression of 5-HTR1D, 5-HTR1E, 5-HTR2B, 5-HTR3A, 5-HTR4, 5-HTR5A, and 5-HTR7 in sections around A3.4 to A2.4 in P1 chick brainstems but did not detect any signals, suggesting that either the levels of expression of 5-HTR genes were very low or cells expressing 5-HTR genes were very rare. This was supported by the results of RT-PCR using cDNA synthesized from the chick brainstems (Supplementary Figure S3).
Double ISH analysis of 5-HTR1A and 5-HTR1B with dopaminergic neuron marker genes in the chick brainstem
We found that 5-HTR1A and 5-HTR1B were expressed in the A8, SNc, and VTA. Subsequently, we examined whether 5-HTR-expressing cells were dopaminergic neurons or not. We performed a double ISH analysis in sections A3.0 and A3.2, including SNc and VTA, to test whether 5-HTR1A- or 5-HTR1B-expressing cells expressed a dopaminergic neuron marker gene, TH (Figure 6). We detected cells with alternating 5-HTR1A or TH signals, but did not detect any double positive cells for 5-HTR1A and TH in the SNc (Figures 6A,A′) and the VTA (Figures 6D,D′). Similarly, we detected cells with alternating 5-HTR1B or TH signals but no double positive cells for 5-HTR1B and TH in the SNc (Figures 6B,B′) and the VTA (Figures 6E,E′).
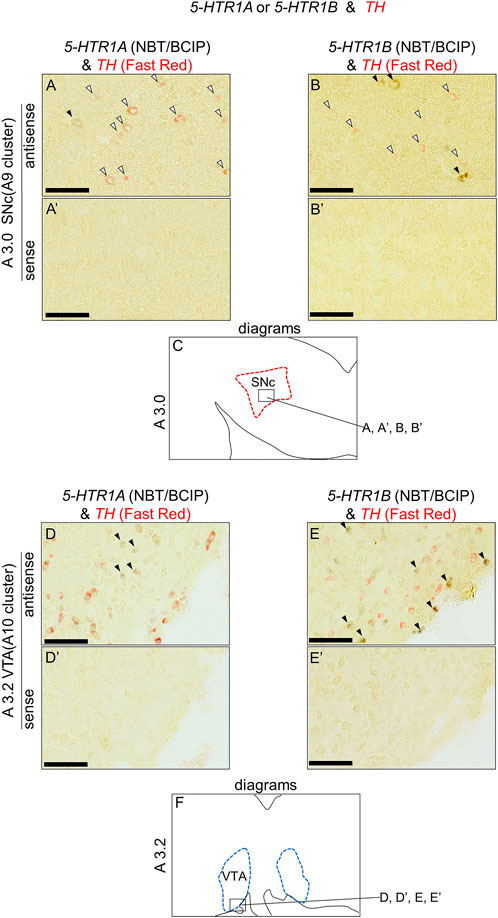
FIGURE 6. Double in situ hybridization of 5-HTR1A or 5-HTR1B and TH in the SNc and VTA of the P1 chick brainstem. Digoxigenin-labeled (5-HTR1A and 5-HTR1B) and fluorescein-labeled TH RNA antisense (A,B,D,E) and sense (A′,B′,D′,E′) probes were used for double in situ hybridization in coronal sections of P1 chick brainstems. (A,B) and (A′,B′) Magnified views of dopaminergic nuclei in the box in (C). Black arrowheads indicate 5-HTR1A or 5-HTR1B signals, whereas white arrowheads indicate TH signals. (C) Diagrams of coronal sections shown in the A3.0 sections (Supplementary Figure S4). (D,E) and (D′,E′) Magnified views of dopaminergic nuclei in the box in (F). Black arrowheads indicate 5-HTR1A or 5-HTR1B signals, whereas white arrowheads indicate TH signals. (F) Diagrams of coronal sections shown in the A3.2 sections (Supplementary Figure S4). SNc, substantia nigra pars compacta; VTA, ventral tegmental area. Scale bars = 100 µm.
Discussion
Distribution and molecular property of dopaminergic neurons in the midbrain of chicks
In the present study, we described the distribution of midbrain dopaminergic neurons in the brainstem of chicks, which were characterized by the expression of the chick orthologs of mammalian dopaminergic neuron-related genes, TH and DDC, and the lack of expression of the chick ortholog of mammalian noradrenergic neuron-related gene, DBH (Figure 1). We clearly showed that midbrain dopaminergic neurons were distributed in the A8, A9 (SNc), and A10 (VTA), in agreement with previous studies (Ikeda and Goto, 1971; Dube and Parent, 1981; Guglielmone and Panzica, 1984; Moons et al., 1994). Generally, ISH analysis detects only the cell bodies of neurons, making it suitable for the purpose of mapping the distributions of dopaminergic neuron cells and evaluating heterogeneity.
In addition, we showed that dopaminergic neurons existed in the DR (Figures 1P–S, Figures 1P′–S′). In mammals, the DR dopaminergic neurons exist as a specialized midbrain dopaminergic subsystem that encodes incentive salience and controls the expression of incentive memory, with focal projections mainly to the central amygdala and the bed nucleus of stria terminalis (Hasue and Shammah-Lagnado, 2002; Poulin et al., 2014; Lin et al., 2020; Lin et al., 2021). To our knowledge, not much is known about DR dopaminergic neurons in birds. In the future, through the use of neuronal tracers, it will be interesting to determine whether the functions and projection targets of DR dopaminergic neurons are conserved between birds and mammals.
In mammals, the numbers and distribution of dopaminergic neurons in the midbrain dopaminergic nuclei have been extensively quantified (Swanson, 1982; German and Manaye, 1993; Nair-Roberts et al., 2008). For example, Swanson (1982) estimated that two-thirds of cells were dopaminergic in the rat VTA. Furthermore, considerable heterogeneities have been detected within the nuclei, which contain glutamatergic and GABAergic neurons in addition to dopaminergic neurons (Nair-Roberts et al., 2008; Lammel et al., 2014). However, currently, there is no data on what type of cells exist in the dopaminergic nuclei of birds and in what proportions. Yet, our data indicated that a TH-positive/DDC-positive/DBH-negative cell population exists in many of these dopaminergic nuclei (VTA, SNc, and A8). Our results suggested that the proportion of dopaminergic neurons in the dopaminergic nuclei of birds is not inconsistent with those of mammals. However, a more detailed estimation of avian dopaminergic nuclei will be necessary in the future.
In a previous study, Lovell et al. (2015) showed that the DAT gene was deleted in the genomes of sauropsids (birds and reptiles) and that NAT was expressed in the SNc and VTA of songbirds, suggesting that NAT compensates for the function of DAT in sauropsids (Lovell et al., 2015). We found that all nuclei containing dopaminergic neurons in the chick midbrain also expressed NAT, suggesting that the expression pattern of NAT was a general molecular property of the midbrain dopaminergic neurons in the chick. Hence, our results supported the theory of NAT compensating the function of DAT.
Serotonergic regulation via 5-HTR1A and 5-HTR1B in chick midbrain dopaminergic nuclei
We found that 5-HTR1A was expressed in the A8, SNc, and VTA in the chick midbrain (Figure 2), suggesting that chick dopaminergic nuclei are modulated by serotonin through 5-HTR1A. We also found that 5-HTR1A-expressing cells in the dopaminergic nuclei did not overlap with TH-expressing neurons in the chick midbrain (Figure 7). In mammals, 5-Htr1a was not strongly detected in dopaminergic nuclei (Pompeiano et al., 1992; Burnet et al., 1995; Pasqualetti et al., 1996; Vilaró et al., 2020). In addition, using neuronal cell type markers revealed that 5-Htr1a-expressing cells did not overlap with Th-expressing neurons but with glutamatergic or GABAergic neurons in the mouse rostral brainstem (Bonnavion et al., 2010). Accordingly, 5-HTR1A-expressing cells in the chick dopaminergic nuclei might be glutamatergic or GABAergic neurons. Our findings regarding the expression of 5-HTR1A in dopaminergic nuclei will facilitate the elucidation of the mechanism by which 5-HTR1A-mediated regulation of dopaminergic nuclei affects behavior in chicks and the understanding of the interactions between dopaminergic and serotonergic systems in birds.
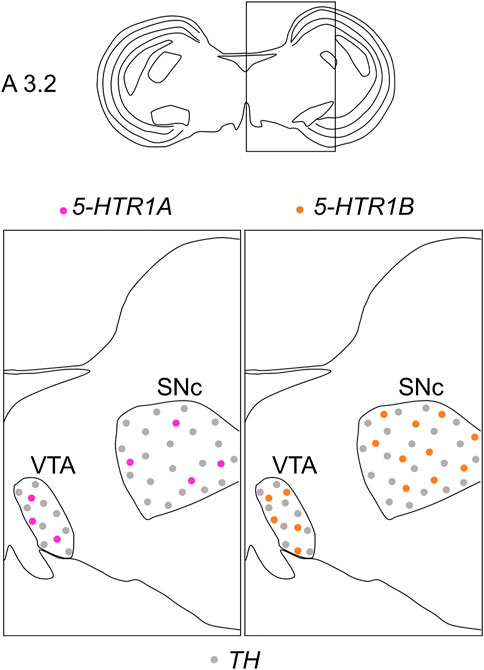
FIGURE 7. Schematic summary of the expression patterns of 5-HTR1A or 5-HTR1B and TH in dopaminergic nuclei in the P1 chick midbrain. Representative expression patterns in sections around A 3.2 are exhibited in colored areas (magenta, 5-HTR1A; orange, 5-HTR1B; grey, TH). The alternating dotted pattern indicates that the cells were sparsely distributed with alternating 5-HTR1A (or 5-HTR1B) and TH signals but no double positive signal. The levels of expression in sections were in accordance with those mentioned in the Kuenzel and Masson’s chick atlas (Kuenzel and Masson, 1988). P1, post-hatch day 1; SNc, substantia nigra pars compacta; VTA, ventral tegmental area.
We found that 5-HTR1B was clearly expressed in the A8, SNc, and VTA in the chick midbrain (Figure 3), further suggesting that chick dopaminergic nuclei are modulated by serotonin through 5-HTR1B. We also found that 5-HTR1B-expressing cells in the dopaminergic nuclei did not overlap with TH-expressing neurons in the chick midbrain (Figure 7), suggesting that 5-HTR1B-expressing cells in the dopaminergic nuclei were glutamatergic or GABAergic neurons. It is possible that 5-HTR1B-expressing glutamatergic- or GABAergic neurons, which constitute a local microcircuit in dopaminergic nuclei, might regulate dopaminergic neurons in the chick midbrain. In mammals, high density signals of 5-HTR1B were detected in the broad brain areas, including the substantia nigra and VTA using radioligands (Bonaventure et al., 1997; Varnas et al., 2001). On the contrary, ISH analysis revealed the lack of signals of 5-HTR1B specific RNA probes in the substantia nigra and VTA, suggesting that the high densities of 5-Htr1b protein found in these regions of mismatch were located on axon terminals of extrinsic origin, the presynaptic receptors, or presynaptic autoreceptors of serotonergic innervations (Bruinvels et al., 1994; Varnas et al., 2005). These findings suggested that dopaminergic neurons in the midbrain dopaminergic nuclei are regulated by serotonin via 5-HTR1B, and these properties might be evolutionarily conserved between birds and mammals. Differences in the expression of 5-HTR1B between bird and mammalian dopaminergic nuclei have suggested that the serotonin-regulated local microcircuitries in the dopaminergic nuclei between bird and mammals are different.
In this study, other 5-HTR subfamily genes except 5-HTR1A and 5-HTR1B were not detected in the dopaminergic nuclei in chick midbrains. These results suggested that the levels of expression of these 5-HTR genes were very low or cells expressing 5-HTR genes were very rare. The serotonergic regulation to the reward processing via 5-HTR2A, 5-HTR2C, and 5-HTR3A in mammals has attracted increased attention (Liu et al., 2020; Peters et al., 2021). Moreover, using a combination of immunolabeling and ISH with radioisotope-labeled probe autoradiography revealed that 5-HTR3A was expressed on Th-positive neurons in the VTA in mice (Wang et al., 2019). Generally, autoradiography using radioisotope-labeled probe can achieve higher sensitivity than the conventional chemical coloring ISH method. In addition, recent single-cell RNA sequencing studies of dopaminergic nuclei showed that the levels of expression of 5-Htr genes were very low (Saunders et al., 2018; Huang et al., 2019; Tiklova et al., 2019; Poulin et al., 2020). This was consistent with our findings that the levels of expression of 5-Htr genes were too low to detect by our ISH system in this study. In the future, using more sensitive detection systems will clarify whether 5-HTR2A, 5-HTR2C, and 5-HTR3A are expressed and regulated in the avian dopaminergic system.
Expression patterns of 5-HTR1F, 5-HTR2A and 5-HTR2C in the OT layers
The optic tectum (OT) of birds has been extensively developed, exhibiting a high degree of lamination. The OT is a major component of the tectofugal pathway, which includes the retina, OT, nucleus rotundus, and entopallium, which is thought to be the most important visual pathway in birds (Wylie et al., 2009). The OT has 15 perceptible layers and a strict separation between visual input and output. In general, inputs from the retina terminate in superficial layers, whereas the main output from the OT to the nucleus rotundus comes from projection neurons in deeper layers, especially layer 13 (SGC) (Ramon y Cajal, 1911; Luksch, 2003). In a previous study, we showed that the expression patterns of 5-HTR1A and 5-HTR1B in the OT layers were mutually exclusive (Fujita et al., 2022a). In this study, to deepen our understanding of serotonergic regulation in the OT, we carefully and comprehensively performed ISH analysis and evaluated the expression of 5-HTR subtypes in the chick OT. We found that 5-HTR1F-expressing cells were distributed in layers 5a, 9, 11, and 15 (Figures 4B,B′), 5-HTR2A-expressing cells were distributed in layer 14 and a part of layer 13 (Figures 5C,C′), and 5-HTR2C-expressing cells were distributed in layers 11 and 14 (Figures 5D,D′). In addition to 5-HTR1A and 5-HTR1B (Fujita et al., 2022a), 5-HTR1F, 5-HTR2A, and 5-HTR2C might play important roles in the modulation of the function of neurons, including visual processing in the tectofugal pathway. For example, we previously showed that 5-HTR1F was preferentially expressed in the interstitial part of the hyperpallium (IHA) in the chick telencephalon (Fujita et al., 2022b). The IHA is part of the homologous region to the mammalian neocortex (Fernandez et al., 1998; Puelles et al., 2000) with projection terminals of sensory information from the thalamus, and is thus considered equivalent to layer IV of the mammalian neocortex (Bagnoli and Burkhalter, 1983; Miceli and Repérant, 1985; Miceli et al., 1990; Atoji et al., 2018; Atoji and Wild, 2019). Here, we found that 5-HTR1F was expressed in layers 5a and 9 of the chick OT (Figures 4B,B′). Recently, a detailed morphological and physiological study of neurons in layer 9 of chicken OT demonstrated the nature of input received in retinal projection via glutamatergic synapses (Kloos et al., 2019). Taken together, it might be possible that 5-HTR1F-expressing neurons in the IHA and layers 5a and 9 in the OT share a common function in processing sensory input under serotonergic modulation. In addition, a previous study that used immunohistochemistry reported that 5-HTR2A was intensely expressed throughout layer 13 in the chicken OT (Metzger et al., 2006). Our data clearly showed that 5-HTR2A-expressing cells were distributed in a part of the deep side of layer 13 and throughout layer 14 (Figures 5C,C′), in consistency with a previous study regarding the output deep layer (Metzger et al., 2006). This discordance between our data and those of the previous study is not clear, but the comparison of the layer of expression of 5-HTR2A with that of 5-HTR1A, which has been shown to be more strongly and sharply expressed in layer 13, could clarify the exact expression layer.
Conclusion
We have comprehensively described the distribution of dopaminergic neurons in the A8, SNc, and VTA of the chick midbrain and found that 5-HTR1A and 5-HTR1B were expressed in dopaminergic nuclei. 5-HTR1A and 5-HTR1B, which are mainly associated with the dopaminergic system, might be involved in the regulation of motor control and motivation-related behavior. In addition, we found that 5-HTR1F, 5-HTR2A, and 5-HTR2C were expressed in the OT in layer-selective manners. Our findings can facilitate the improvement of our understanding of the interactions between dopaminergic and serotonergic systems in birds and the serotonergic regulation of OT neural circuits.
Data availability statement
The original contributions presented in the study are included in the article/Supplementary Material, further inquiries can be directed to the corresponding author.
Ethics statement
The animal study was reviewed and approved by the Committee on Animal Experiments of Teikyo University.
Author contributions
TF and SY designed the study and performed the research; TF, NA, CM, KH, and SY analyzed the data; TF, NA, CM, SS, FK-N., KH, and SY wrote the paper. All authors have reviewed and accepted the final version of the manuscript.
Funding
This work was supported by a Teikyo University Research Encouragement Grant (TF), a Fund for the Promotion of Joint International Research [Fostering Joint International Research (B)] (TF, KH 19KK0211), Grants-in-Aid for Scientific Research from the Japan Society for the Promotion of Science (SY, 24590096, 15K07945, and 18K06667; NA, 24790089 and 20K06915; CM, 20K16472 and KH, 26440182, 17K07492, and 20K06747), the Uehara Memorial Foundation (SY), the Sagawa Foundation for Promotion of Cancer Research (SY), and a Grant-in-Aid for Scientific Research on Innovative Areas “Memory dynamism” (26115522), “Adaptive circuit shift” (15H01449) and “Evolinguistics” (20H05012) from the Ministry of Education, Culture, Sports, Science and Technology (KH), the Naito Foundation (KH), and the Japan Foundation for Applied Enzymology (KH).
Acknowledgments
We thank E. Aso, S. Nakaie, R. Nakajima, and S. Saito (Teikyo University, Faculty of Pharmaceutical Sciences) for technical assistance. We would like to thank Editage (www.editage.com) for English language editing.
Conflict of interest
The authors declare that the research was conducted in the absence of any commercial or financial relationships that could be construed as a potential conflict of interest.
Publisher’s note
All claims expressed in this article are solely those of the authors and do not necessarily represent those of their affiliated organizations, or those of the publisher, the editors and the reviewers. Any product that may be evaluated in this article, or claim that may be made by its manufacturer, is not guaranteed or endorsed by the publisher.
Supplementary material
The Supplementary Material for this article can be found online at: https://www.frontiersin.org/articles/10.3389/fphys.2022.1030621/full#supplementary-material
References
Anderegg A., Poulin J. F., Awatramani R. (2015). Molecular heterogeneity of midbrain dopaminergic neurons - moving toward single cell resolution. FEBS Lett. 589, 3714–3726. doi:10.1016/j.febslet.2015.10.022
Atoji Y., Sarkar S., Wild J. M. (2018). Differential projections of the densocellular and intermediate parts of the hyperpallium in the pigeon (Columba livia). J. Comp. Neurol. 526, 146–165. doi:10.1002/cne.24328
Atoji Y., Wild J. M. (2019). Projections of the densocellular part of the hyperpallium in the rostral Wulst of pigeons (Columba livia). Brain Res. 1711, 130–139. doi:10.1016/j.brainres.2019.01.001
Bacque-Cazenave J., Bharatiya R., Barriere G., Delbecque J. P., Bouguiyoud N., Di Giovanni G., et al. (2020). Serotonin in animal cognition and behavior. Int. J. Mol. Sci. 21, E1649. doi:10.3390/ijms21051649
Bagnoli P., Burkhalter A. (1983). Organization of the afferent projections to the Wulst in the pigeon. J. Comp. Neurol. 214, 103–113. doi:10.1002/cne.902140111
Balint E., Csillag A. (2007). Nucleus accumbens subregions: Hodological and immunohistochemical study in the domestic chick (Gallus domesticus). Cell Tissue Res. 327, 221–230. doi:10.1007/s00441-006-0295-0
Balint E., Mezey S., Csillag A. (2011). Efferent connections of nucleus accumbens subdivisions of the domestic chicken (Gallus domesticus): An anterograde pathway tracing study. J. Comp. Neurol. 519, 2922–2953. doi:10.1002/cne.22672
Beier K. T., Steinberg E. E., Deloach K. E., Xie S., Miyamichi K., Schwarz L., et al. (2015). Circuit architecture of VTA dopamine neurons revealed by systematic input-output mapping. Cell 162, 622–634. doi:10.1016/j.cell.2015.07.015
Beyeler A., Ju A., Chagraoui A., Cuvelle L., Teixeira M., Di Giovanni G., et al. (2021). Multiple facets of serotonergic modulation. Prog. Brain Res. 261, 3–39. doi:10.1016/bs.pbr.2021.02.002
Bjorklund A., Dunnett S. B. (2007). Dopamine neuron systems in the brain: An update. Trends Neurosci. 30, 194–202. doi:10.1016/j.tins.2007.03.006
Bonaventure P., Schotte A., Cras P., Leysen J. E. (1997). Autoradiographic mapping of 5-HT1B- and 5-HT1D receptors in human brain using [3H]alniditan, a new radioligand. Recept. Channels 5, 225–230.
Bonnavion P., Bernard J. F., Hamon M., Adrien J., Fabre V. (2010). Heterogeneous distribution of the serotonin 5-ht1a receptor mRNA in chemically identified neurons of the mouse rostral brainstem: Implications for the role of serotonin in the regulation of wakefulness and REM sleep. J. Comp. Neurol. 518, 2744–2770. doi:10.1002/cne.22331
Boureau Y. L., Dayan P. (2011). Opponency revisited: Competition and cooperation between dopamine and serotonin. Neuropsychopharmacology 36, 74–97. doi:10.1038/npp.2010.151
Bruinvels A. T., Landwehrmeyer B., Gustafson E. L., Durkin M. M., Mengod G., Branchek T. A., et al. (1994). Localization of 5-Ht1b, 5-Ht1d-Alpha, 5-Ht1e and 5-Ht1f, Receptor Messenger-Rna in Rodent and Primate Brain. Neuropharmacology 33, 367–386. doi:10.1016/0028-3908(94)90067-1
Burnet P. W. J., Eastwood S. L., Lacey K., Harrison P. J. (1995). The distribution of 5-HT1A and 5-HT2A receptor mRNA in human brain. Brain Res. 676, 157–168. doi:10.1016/0006-8993(95)00104-x
Csillag A. (1999). Striato-telencephalic and striato-tegmental circuits: Relevance to learning in domestic chicks. Behav. Brain Res. 98, 227–236. doi:10.1016/s0166-4328(98)00088-6
Dahlstroem A., Fuxe K. (1964). Evidence for the existence of monoamine-containing neurons in the central nervous system. I. Demonstration of monoamines in the cell bodies of brain stem neurons. Acta Physiol. Scand. Suppl. 232, 231–255.
De Deurwaerdere P., Di Giovanni G. (2021). 5-HT interaction with other neurotransmitters: An overview. Prog. Brain Res. 259, 1–5. doi:10.1016/bs.pbr.2021.01.001
De Deurwaerdere P., Di Giovanni G. (2017). Serotonergic modulation of the activity of mesencephalic dopaminergic systems: Therapeutic implications. Prog. Neurobiol. 151, 175–236. doi:10.1016/j.pneurobio.2016.03.004
Dube L., Parent A. (1981). The monoamine-containing neurons in avian brain: I. A study of the brain stem of the chicken (Gallus domesticus) by means of fluorescence and acetylcholinesterase histochemistry. J. Comp. Neurol. 196, 695–708. doi:10.1002/cne.901960413
Durstewitz D., Kroner S., Gunturkun O. (1999). The dopaminergic innervation of the avian telencephalon. Prog. Neurobiol. 59, 161–195. doi:10.1016/s0301-0082(98)00100-2
Fee M. S., Goldberg J. H. (2011). A hypothesis for basal ganglia-dependent reinforcement learning in the songbird. Neuroscience 198, 152–170. doi:10.1016/j.neuroscience.2011.09.069
Fernandez A. S., Pieau C., Reperant J., Boncinelli E., Wassef M. (1998). Expression of the emx-1 and dlx-1 homeobox genes define three molecularly distinct domains in the telencephalon of mouse, chick, turtle and frog embryos: Implications for the evolution of telencephalic subdivisions in amniotes. Development 125, 2099–2111. doi:10.1242/dev.125.11.2099
Fujita T., Aoki N., Fujita E., Matsushima T., Homma K. J., Yamaguchi S. (2019). The chick pallium displays divergent expression patterns of chick orthologues of mammalian neocortical deep layer-specific genes. Sci. Rep. 9, 20400. doi:10.1038/s41598-019-56960-4
Fujita T., Aoki N., Mori C., Fujita E., Matsushima T., Homma K. J., et al. (2022a). Chick hippocampal formation displays subdivision- and layer-selective expression patterns of serotonin receptor subfamily genes. Front. Physiol. 13, 882633. doi:10.3389/fphys.2022.882633
Fujita T., Aoki N., Mori C., Fujita E., Matsushima T., Homma K. J., et al. (2022b). Serotonergic neurons in the chick brainstem express various serotonin receptor subfamily genes. Front. Physiol. 2548, 815997. doi:10.3389/fphys.2021.815997
Fujita T., Aoki N., Mori C., Fujita E., Matsushima T., Homma K. J., et al. (2020). The dorsal arcopallium of chicks displays the expression of orthologs of mammalian fear related serotonin receptor subfamily genes. Sci. Rep. 10, 21183. doi:10.1038/s41598-020-78247-9
German D. C., Manaye K. F. (1993). Midbrain dopaminergic neurons (nuclei A8, A9, and A10): Three-dimensional reconstruction in the rat. J. Comp. Neurol. 331, 297–309. doi:10.1002/cne.903310302
Guglielmone R., Panzica G. C. (1984). Typology, distribution and development of the catecholamine-containing neurons in the chicken brain. Cell Tissue Res. 237, 67–79. doi:10.1007/BF00229201
Gunturkun O. (2005). Avian and mammalian "prefrontal cortices": Limited degrees of freedom in the evolution of the neural mechanisms of goal-state maintenance. Brain Res. Bull. 66, 311–316. doi:10.1016/j.brainresbull.2005.02.004
Hasue R. H., Shammah-Lagnado S. J. (2002). Origin of the dopaminergic innervation of the central extended amygdala and accumbens shell: A combined retrograde tracing and immunohistochemical study in the rat. J. Comp. Neurol. 454, 15–33. doi:10.1002/cne.10420
Hillarp N. A., Fuxe K., Dahlstrom A. (1966). Demonstration and mapping of central neurons containing dopamine, noradrenaline, and 5-hydroxytryptamine and their reactions to psychopharmaca. Pharmacol. Rev. 18, 727–741.
Huang K. W., Ochandarena N. E., Philson A. C., Hyun M., Birnbaum J. E., Cicconet M., et al. (2019). Molecular and anatomical organization of the dorsal raphe nucleus. Elife 8, e46464. doi:10.7554/eLife.46464
Ikeda H., Goto J. (1971). Distribution of monoamine-containing cells in the central nervous system of the chicken. Jpn. J. Pharmacol. 21, 763–784. doi:10.1254/jjp.21.763
Kandel E. R., Schwartz J. H., Jessell T. M., Siegelbaum S., Hudspeth A. J., Mack S. (2000). Principles of neural science. New York: McGraw-hill.
Kloos M., Weigel S., Luksch H. (2019). Anatomy and Physiology of neurons in layer 9 of the chicken optic tectum. Front. Neural Circuits 13, 63. doi:10.3389/fncir.2019.00063
Krause E. T., Kjaer J. B., Dudde A., Schrader L., Phi-Van L. (2019). Fear but not social behaviour is affected by a polymorphism in the 5’-flanking region of the serotonin transporter (5-HTT) gene in adult hens. Behav. Brain Res. 361, 50–53. doi:10.1016/j.bbr.2018.12.029
Krause E. T., Kjaer J. B., Luders C., Van L. P. (2017). A polymorphism in the 5'-flanking region of the serotonin transporter (5-HTT) gene affects fear-related behaviors of adult domestic chickens. Behav. Brain Res. 330, 92–96. doi:10.1016/j.bbr.2017.04.051
Kuenzel W. J., Masson M. (1988). A stereotaxic atlas of the brain of the chick (Gallus domesticus). Baltimore, MD: Johns Hopkins University Press.
Lammel S., Lim B. K., Malenka R. C. (2014). Reward and aversion in a heterogeneous midbrain dopamine system. Neuropharmacology 76, 351–359. doi:10.1016/j.neuropharm.2013.03.019
Lammel S., Steinberg E. E., Foldy C., Wall N. R., Beier K., Luo L. Q., et al. (2015). Diversity of transgenic mouse models for selective targeting of midbrain dopamine neurons. Neuron 85, 429–438. doi:10.1016/j.neuron.2014.12.036
Lin R., Liang J. W., Luo M. M. (2021). The raphe dopamine system: Roles in salience encoding, memory expression, and addiction. Trends Neurosci. 44, 366–377. doi:10.1016/j.tins.2021.01.002
Lin R., Liang J. W., Wang R. Y., Yan T., Zhou Y. T., Liu Y., et al. (2020). The raphe dopamine system controls the expression of incentive memory. Neuron 106, 498–514. doi:10.1016/j.neuron.2020.02.009
Liu Z. X., Lin R., Luo M. M. (2020). Reward contributions to serotonergic functions. Annu. Rev. Neurosci. 43, 141–162. doi:10.1146/annurev-neuro-093019-112252
Lovell P. V., Kasimi B., Carleton J., Velho T. A., Mello C. V. (2015). Living without DAT: Loss and compensation of the dopamine transporter gene in sauropsids (birds and reptiles). Sci. Rep. 5, 14093. doi:10.1038/srep14093
Luksch H. (2003). Cytoarchitecture of the avian optic tectum: Neuronal substrate for cellular computation. Rev. Neurosci. 14, 85–106. doi:10.1515/revneuro.2003.14.1-2.85
Marin P., Becamel C., Chaumont-Dubel S., Vandermoere F., Bockaert J., Claeysen S. (2020). Classification and signaling characteristics of 5-HT receptors: Toward the concept of 5-HT receptosomes. Handb. Behav. Neurosci. 31, 91–120. doi:10.1016/b978-0-444-64125-0.00005-0
Metzger M., Britto L. R. G., Toledo C. a. B. (2006). Monoaminergic markers in the optic tectum of the domestic chick. Neuroscience 141, 1747–1760. doi:10.1016/j.neuroscience.2006.05.020
Mezey S., Csillag A. (2002). Selective striatal connections of midbrain dopaminergic nuclei in the chick (Gallus domesticus). Cell Tissue Res. 308, 35–46. doi:10.1007/s00441-002-0514-2
Miceli D., Marchand L., Repérant J., Rio J.-P. (1990). Projections of the dorsolateral anterior complex and adjacent thalamic nuclei upon the visual Wulst in the pigeon. Brain Res. 518, 317–323. doi:10.1016/0006-8993(90)90990-s
Miceli D., Repérant J. (1985). Telencephalic afferent projections from the diencephalon and brainstem in the pigeon. A retrograde multiple-label fluorescent study. Exp. Biol. 44, 71–99.
Moons L., Vangils J., Ghijsels E., Vandesande F. (1994). Immunocytochemical localization of L-dopa and dopamine in the brain of the chicken (Gallus-Domesticus). J. Comp. Neurol. 346, 97–118. doi:10.1002/cne.903460107
Morales M., Margolis E. B. (2017). Ventral tegmental area: Cellular heterogeneity, connectivity and behaviour. Nat. Rev. Neurosci. 18, 73–85. doi:10.1038/nrn.2016.165
Nair-Roberts R. G., Chatelain-Badie S. D., Benson E., White-Cooper H., Bolam J. P., Ungless M. A. (2008). Stereological estimates of dopaminergic, gabaergic and glutamatergic neurons in the ventral tegmental area, substantia nigra and retrorubral field in the rat. Neuroscience 152, 1024–1031. doi:10.1016/j.neuroscience.2008.01.046
O'leary O. F., Codagnone M. G., Cryan J. F. (2020). Revisiting the behavioral genetics of serotonin: Relevance to anxiety and depression. Handb. Behav. Neurosci. 31, 665–709. doi:10.1016/b978-0-444-64125-0.00038-4
Ogawa S. K., Cohen J. Y., Hwang D., Uchida N., Watabe-Uchida M. (2014). Organization of monosynaptic inputs to the serotonin and dopamine neuromodulatory systems. Cell Rep. 8, 1105–1118. doi:10.1016/j.celrep.2014.06.042
Ogawa S. K., Watabe-Uchida M. (2018). Organization of dopamine and serotonin system: Anatomical and functional mapping of monosynaptic inputs using rabies virus. Pharmacol. Biochem. Behav. 174, 9–22. doi:10.1016/j.pbb.2017.05.001
Papini M. R., Penagos-Corzo J. C., Perez-Acosta A. M. (2019). Avian emotions: Comparative perspectives on fear and frustration. Front. Psychol. 9, 2707. doi:10.3389/fpsyg.2018.02707
Pasqualetti M., Nardi I., Ladinsky H., Marazziti D., Cassano G. B. (1996). Comparative anatomical distribution of serotonin 1A, 1D alpha and 2A receptor mRNAs in human brain postmortem. Brain Res. Mol. Brain Res. 39, 223–233. doi:10.1016/0169-328x(96)00026-5
Peters K. Z., Cheer J. F., Tonini R. (2021). Modulating the neuromodulators: Dopamine, serotonin, and the endocannabinoid system. Trends Neurosci. 44, 464–477. doi:10.1016/j.tins.2021.02.001
Phi Van V. D., Krause E. T., Phi-Van L. (2018). Modulation of fear and arousal behavior by serotonin transporter (5-HTT) genotypes in newly hatched chickens. Front. Behav. Neurosci. 12, 284. doi:10.3389/fnbeh.2018.00284
Pompeiano M., Palacios J. M., Mengod G. (1992). Distribution and cellular localization of mRNA coding for 5-ht1a receptor in the rat brain: Correlation with receptor binding. J. Neurosci. 12, 440–453. doi:10.1523/jneurosci.12-02-00440.1992
Poulin J. F., Gaertner Z., Moreno-Ramos O. A., Awatramani R. (2020). Classification of midbrain dopamine neurons using single-cell gene expression profiling approaches. Trends Neurosci. 43, 155–169. doi:10.1016/j.tins.2020.01.004
Poulin J. F., Zou J., Drouin-Ouellet J., Kim K. Y. A., Cicchetti F., Awatramani R. B. (2014). Defining midbrain dopaminergic neuron diversity by single-cell gene expression profiling. Cell Rep. 9, 930–943. doi:10.1016/j.celrep.2014.10.008
Puelles L., Kuwana E., Puelles E., Bulfone A., Shimamura K., Keleher J., et al. (2000). Pallial and subpallial derivatives in the embryonic chick and mouse telencephalon, traced by the expression of the genes Dlx-2, Emx-1, Nkx-2.1, Pax-6, and Tbr-1. J. Comp. Neurol. 424, 409–438. doi:10.1002/1096-9861(20000828)424:3<409::aid-cne3>3.0.co;2-7
Puig M. V., Rose J., Schmidt R., Freund N. (2014). Dopamine modulation of learning and memory in the prefrontal cortex: Insights from studies in primates, rodents, and birds. Front. Neural Circuits 8, 93. doi:10.3389/fncir.2014.00093
Ramón y Cajal S. (1911). “Le lobe optique des vertébrés inférieurs, toit optique des oiseaux,” in Histologie du systéme nerveux de l'homme e des vertébrés (Paris: Ramón y CajalMaloine), 196–212.
Reiner A., Perkel D. J., Bruce L. L., Butler A. B., Csillag A., Kuenzel W., et al. (2004). Revised nomenclature for avian telencephalon and some related brainstem nuclei. J. Comp. Neurol. 473, 377–414. doi:10.1002/cne.20118
Rieke G. K. (1980). Kainic acid lesions of pigeon paleostriatum: A model for study of movement disorders. Physiol. Behav. 24, 683–687. doi:10.1016/0031-9384(80)90397-2
Rieke G. K. (1981). Movement disorders and lesions of pigeon brain stem analogues of basal ganglia. Physiol. Behav. 26, 379–384. doi:10.1016/0031-9384(81)90162-1
Rosa Salva O., Mayer U., Vallortigara G. (2015). Roots of a social brain: Developmental models of emerging animacy-detection mechanisms. Neurosci. Biobehav. Rev. 50, 150–168. doi:10.1016/j.neubiorev.2014.12.015
Saunders A., Macosko E. Z., Wysoker A., Goldman M., Krienen F. M., De Rivera H., et al. (2018). Molecular diversity and specializations among the cells of the adult mouse brain. Cell 174, 1015–1030. doi:10.1016/j.cell.2018.07.028
Swanson L. W. (1982). The projections of the ventral tegmental area and adjacent regions: A combined fluorescent retrograde tracer and immunofluorescence study in the rat. Brain Res. Bull. 9, 321–353. doi:10.1016/0361-9230(82)90145-9
Tiklova K., Bjorklund A. K., Lahti L., Fiorenzano A., Nolbrant S., Gillberg L., et al. (2019). Single-cell RNA sequencing reveals midbrain dopamine neuron diversity emerging during mouse brain development. Nat. Commun. 10, 581. doi:10.1038/s41467-019-08453-1
Varnas K., Hall H., Bonaventure P., Sedvall G. (2001). Autoradiographic mapping of 5-HT(1B) and 5-HT(1D) receptors in the post mortem human brain using [(3)H]GR 125743. Brain Res. 915, 47–57. doi:10.1016/s0006-8993(01)02823-2
Varnas K., Hurd Y. L., Hall H. (2005). Regional expression of 5-HT1B receptor mRNA in the human brain. Synapse 56, 21–28. doi:10.1002/syn.20128
Vilaró M., Cortés R., Mengod G., Hoyer D. (2020). Distribution of 5-HT receptors in the central nervous system: An update. Handb. Behav. Neurosci. 31, 121–146. doi:10.1016/b978-0-444-64125-0.00006-2
Wang H. L., Zhang S. L., Qi J., Wang H. K., Cachope R., Mejias-Aponte C. A., et al. (2019). Dorsal raphe dual serotonin-glutamate neurons drive reward by establishing excitatory synapses on VTA mesoaccumbens dopamine neurons. Cell Rep. 26, 1128–1142. doi:10.1016/j.celrep.2019.01.014
Wise R. A. (2004). Dopamine, learning and motivation. Nat. Rev. Neurosci. 5, 483–494. doi:10.1038/nrn1406
Wylie D. R. W., Gutierrez-Ibanez C., Pakan J. M. P., Iwaniuk A. N. (2009). The optic tectum of birds: Mapping our way to understanding visual processing. Can. J. Exp. Psychology-Revue Can. De Psychol. Exp. 63, 328–338. doi:10.1037/a0016826
Yagishita S. (2020). Transient and sustained effects of dopamine and serotonin signaling in motivation-related behavior. Psychiatry Clin. Neurosci. 74, 91–98. doi:10.1111/pcn.12942
Yamaguchi S., Fujii-Taira I., Katagiri S., Izawa E., Fujimoto Y., Takeuchi H., et al. (2008a). Gene expression profile in cerebrum in the filial imprinting of domestic chicks (Gallus gallus domesticus). Brain Res. Bull. 76, 275–281. doi:10.1016/j.brainresbull.2008.02.002
Keywords: dopamine, serotonergic receptor, chick (Gallus gallus), A8, substantia nigra pars compacta, ventral tegmental area, optic tectum
Citation: Fujita T, Aoki N, Mori C, Serizawa S, Kihara-Negishi F, Homma KJ and Yamaguchi S (2022) Dopaminergic nuclei in the chick midbrain express serotonin receptor subfamily genes. Front. Physiol. 13:1030621. doi: 10.3389/fphys.2022.1030621
Received: 29 August 2022; Accepted: 28 October 2022;
Published: 08 November 2022.
Edited by:
Gergely Zachar, Semmelweis University, HungaryReviewed by:
Hongxing Zhang, Xuzhou Medical University, ChinaLubica Kubikova, Slovak Academy of Sciences (SAS), Slovakia
Arpad Dobolyi, Eötvös Loránd University, Hungary
Copyright © 2022 Fujita, Aoki, Mori, Serizawa, Kihara-Negishi, Homma and Yamaguchi. This is an open-access article distributed under the terms of the Creative Commons Attribution License (CC BY). The use, distribution or reproduction in other forums is permitted, provided the original author(s) and the copyright owner(s) are credited and that the original publication in this journal is cited, in accordance with accepted academic practice. No use, distribution or reproduction is permitted which does not comply with these terms.
*Correspondence: Shinji Yamaguchi, shinji-y@pharm.teikyo-u.ac.jp