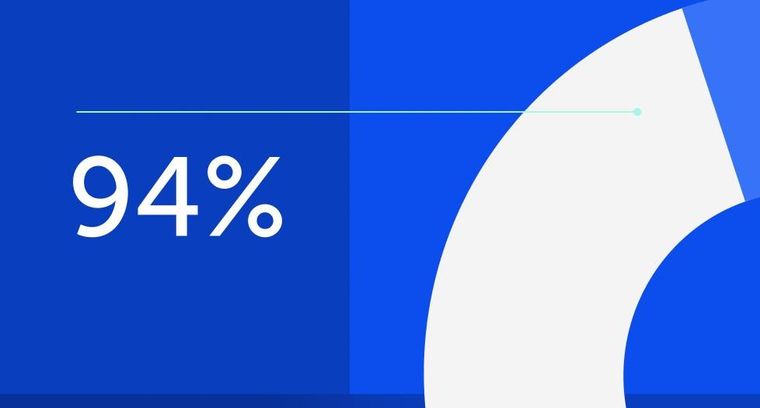
94% of researchers rate our articles as excellent or good
Learn more about the work of our research integrity team to safeguard the quality of each article we publish.
Find out more
ORIGINAL RESEARCH article
Front. Physiol., 25 November 2022
Sec. Red Blood Cell Physiology
Volume 13 - 2022 | https://doi.org/10.3389/fphys.2022.1021553
This article is part of the Research TopicImages from Red Cells, Volume IIView all 6 articles
Storage of packed red blood cells is associated with changes in erythrocytes that over time increasingly impair cellular function and potentially contribute to adverse effects associated with blood transfusion. Exposure of phosphatidylserine at the outer membrane leaflet of erythrocytes and shedding of microvesicles (MVs) during packed red blood cell storage are alterations assumed to increase the risk of prothrombotic events in recipients. Here, we used rotational thromboelastometry to study the coagulation process in blood samples with erythrocytes from stored PRBCs reconstituted with freshly prepared platelet-rich plasma. We explored the influence of following effects on the coagulation process: 1) PRBC storage duration, 2) differences between erythrocytes from stored PRBCs compared to freshly drawn erythrocytes, and 3) the contribution of added MVs. Interestingly, despite of a higher fraction of PS-positive cells, erythrocytes from PRBCs stored for 6 weeks revealed longer clotting times than samples with erythrocytes stored for 2 or 4 weeks. Further, clotting times and clot formation times were considerably increased in samples reconstituted with erythrocytes from stored PRBCs as compared to fresh erythrocytes. Moreover, MVs added to reconstituted samples elicited only comparably small and ambiguous effects on coagulation. Thus, this study provides no evidence for an amplified clotting process from prolonged storage of PRBCs but on the contrary implicates a loss of function, which may be of clinical significance in massive transfusion. Our observations add to the increasing body of evidence viewing erythrocytes as active players in the clotting process.
Over the course of routine 6-week cold storage in packed red blood cell (PRBC) units, erythrocytes progressively accumulate biochemical alterations, collectively termed “storage lesions”. These changes include irreversible lipid and protein modifications via glycation, altered energy metabolism, methemoglobin formation and increased oxidative stress. Some notable consequences are an impaired connectivity of the cytoskeleton, altered cation influx/efflux, reduced cell membrane deformability, changes in cell morphology and ultimately hemolysis (Yoshida et al., 2019). Adverse clinical effects have been associated with prolonged storage time of the PRBC units prior to transfusion. PRBC transfusion is for example associated with an increased risk of organ failure (Zallen et al., 1999), infection (Spadaro et al., 2017), hemolytic reactions (Vamvakas and Blajchman, 2010), alloimmunization (Desai et al., 2015) and thromboembolism (Dubovoy and Engoren, 2016). Such transfusion-related thromboembolic events have been suspected to be triggered by microparticles/microvesicles (MVs) that progressively accumulate in the PRCBs (Rubin et al., 2010). These storage-induced MVs are generated by an exovesiculation process of the plasma membrane, display distinct membrane protein composition, and contain high amounts of phosphatidylserine (PS) on their surface (Salzer et al., 2008). By exposing this negatively charged phospholipid, which is normally confined to the inner leaflet of the bilayer, and by their high membrane curvature, MVs provide an ideal surface for the assembly of factors of the clotting cascade. Phosphatidylserine is not only present at MVs, but also becomes increasingly exposed at the surface of PRBCs during prolonged storage (Lu et al., 2011; Dinkla et al., 2014; Mittag et al., 2015). In fact, both MVs (Salzer et al., 2008; Van Der Meijden et al., 2012; Rubin et al., 2013; Aung et al., 2017) and PRBCs (Lu et al., 2011; Dinkla et al., 2014; Mittag et al., 2015) exhibit prothrombotic characteristics as assessed by various assays in vitro. This implicates that both components of the storage unit might actually contribute to the increased in vivo risk of thromboembolism following transfusion.
For a long time, the role of erythrocytes in hemostasis has been regarded as rather passive and mainly ascribed to their high abundance in blood: 1) the rheological properties of blood flowing through the circulatory system ascertain that the bulk of erythrocytes pushes the smaller platelets towards the vessel walls and thereby assures quick sealing in case of vascular damage; 2) thrombus formation was assumed to be based on platelet activation and fibrin filament assembly to result in a locally growing meshwork that necessarily but rather accidently entraps erythrocytes passing by. However, several findings indicate that this view is too simplistic. There is evidence for direct interaction between platelets and erythrocytes via αIIbβ3–ICAM4 and reduced fibrin and thrombus formation upon blockage of this interaction (Hermand et al., 2003; Du et al., 2014). Erythrocytes have further been shown to modulate platelet function in several other ways (Rocca and FitzGerald, 1997; Weisel and Litvinov, 2019). Conversely, activated platelets can rapidly induce procoagulant properties in erythrocytes. Lysophosphatidic acid (LPA), a lipid mediator secreted by activated platelets, induces a signaling cascade in erythrocytes, which results in calcium influx (Yang et al., 2000), MV release (Chung et al., 2007) and PS exposure by activating the phospholipid scramblase TMEM16F (Ohlinger et al., 2020). These LPA-induced effects would clearly assign a coagulation-promoting role to erythrocytes likely during both thrombin generation and clot formation. Moreover, during clot contraction, the shape of entrapped erythrocytes is transformed into a polyhedron-like structure due to contractile forces exerted by platelets and the fibrin meshwork, thereby forming an impermeable barrier essential for wound healing (Cines et al., 2014). Thus, it is now assumed that erythrocytes actively contribute to coagulation, probably being involved at several steps of this complex process. However, while several pro- and anti-hemostatic effects of erythrocytes and their interactions with other cellular and plasma components of the coagulation machinery were assessed by assays in vitro (Weisel and Litvinov, 2019), it still remained largely unknown whether and if - to which extent - each of these effects is relevant for thrombus formation in vivo.
Rapid and accurate assessment of coagulation is often required in a clinical setting (Ho and Pavey, 2017). Viscoelastic tests like thromboelastography and thromboelastometry determine coagulation properties of whole blood samples, thereby measuring activities of both platelets and plasma factors (Whiting et al., 2015). Rotational thromboelastometry (ROTEM) provides insights on formation kinetics, stability, and degradation of blood clots at the bed-side. Various tests using different activators/inhibitors provide an overview over hemostasis and its response to therapy with coagulation factors and blood products. Using ROTEM, a recent murine blood banking study revealed that stored mouse erythrocytes have impaired coagulation properties compared to fresh mouse erythrocytes (Pulliam et al., 2020), contradicting the notion of them being procoagulant due to storage lesions.
The aim of this study was to elucidate the impact of storage of human PRBCs on clot formation as assessed by ROTEM assays in reconstituted blood samples. In the first part we explored the effect of storage duration by sampling of PRBCs from the same units after 2, 4 and 6 weeks, and assessing coagulation properties upon reconstitution with fresh, autologous platelet-rich plasma. In the second part, coagulation properties of AB0- and rhesus-matched PRBCs stored for prolonged periods were compared to those of freshly drawn, autologous erythrocytes. Finally, we studied the effects that MVs accumulating during prolonged storage exert on coagulation properties.
The observational volunteer study was approved by the Ethics Committee of the Medical University of Vienna (1043/2015), and was registered at clinicaltrials.gov (NCT02639780). Twelve healthy volunteers (Supplementary Table S1) were enrolled after providing written informed consent. Each volunteer donated 450 ml blood for a PRBC unit. In addition, blood (9 ml) was drawn at 2, 4, and 6 weeks after the initial blood donation to prepare control samples for viscoelastic tests and platelet-rich plasma (PRP) from the same individual.
Whole blood was drawn from a cubital vein of the study participants, and processed as described previously by the Austrian Red Cross Blood Donation Services (Prosenz et al., 2019). Briefly, blood was leukodepleted and separated into blood components. All PRBC units were stored in saline-adenine-glucose-mannitol solution according to standard blood banking conditions with uninterrupted cooling at 4°C for up to 42 days. Aliquots of PRBCs were aseptically drawn from storage bags at indicated time points. These PRBC units were used for autologous reconstitution of blood samples for ROTEM experiments in the first part of the study. The PRBC units used in the second part of this study were purchased from the Austrian Red Cross Blood Donation Services and stored as described above for up to 6 weeks.
Aliquots were aseptically sampled from PRBC units and centrifuged (5 min at 1000 g). Pelleted erythrocytes were washed 3 times in phosphate buffered saline (PBS) by centrifugation (5 min at 1000 g), and the supernatants discarded. The erythrocytes were diluted to 2 × 107 cells/mL in PBS containing 7.5 mM glucose and 1 mM EDTA (pH = 7.4). Twenty µL/mL of FITC-coupled lactadherin (Lact-FITC, 83 μg/ml; Haematologic Technologies, Inc.) were added, and samples immediately subjected to flow cytometry (488 nm laser, 30,000 events; FACSCalibur, Becton Dickinson).
Hemolysis in the supernatant of PRBC units was assessed by Drabkin’s reagent, containing 1 g/L sodiumbicarbonate, 50 mg/L potassiumcyanide, and 200 mg/L potassiumferricyanide (Sigma Aldrich). 100 µM of supernatant were mixed with 600 µM of reagent, incubated for 30 min at room temperature, the optical density at 540 nm determined in a spectrophotometer, and the amount of hemoglobin calculated against a standard curve.
(Reconstituted) blood samples were stimulated with calcium and ellagic acid (INTEM) or calcium and tissue factor (EXTEM), according to the manufacturer’s instructions and immediately subjected to viscoelastic coagulation testing by ROTEM delta (Tem International GmbH). Clotting time (CT), clot formation time (CFT), clot firmness 10 min after CT (A10), maximum clot firmness (MCF), and α angle were assessed for each sample.
Freshly drawn blood samples were centrifuged for 1 min at 1000 g, supernatants (platelet-rich plasma (PRP)) collected and later used for the reconstitution experiments. Erythrocyte pellets were resuspended in PBS and washed 3 times in PBS (1 min at 3000 g). The cells from the erythrocyte pellets were used as fresh autologous control cells for the ROTEM experiments in the second part of the study.
Aliquots were aseptically removed from PRBCs 2, 4, and 6 weeks of cold storage. RBCs were pelleted by centrifugation (1 min at 3000 g) and washed 3 times in PBS before further use. In the second part of the study, the supernatant after the first centrifugation step was processed further for MV preparation (see below).
Microvesicles were prepared from stored PRBC units as previously described (Salzer et al., 2008). Briefly, the supernatant of PRBC units was centrifuged at 16,000 g for 30 min at 4°C, and the pellet suspended in PBS. After a short centrifugation step (3000 g for 1 min) to remove contaminating cellular debris, the supernatant was separated and centrifuged again at 16,000 x g for 30 min. The vesicle pellet was suspended in PBS to result in a 40-fold vesicle concentration (40 x MV) as compared to that of the supernatant in PRBC units.
Two hundred µL of washed and pelleted erythrocytes from PRBCs were combined with an equal volume of freshly prepared autologous PRP to give a reconstituted blood sample with a hematocrit of 50%. Similarly, in the second part of the study, freshly prepared, autologous, packed erythrocytes or heterologous, AB0- and rhesus-matched PRBCs were combined with an equal volume of “modified” PRP preparations resulting in a reconstituted blood sample with a hematocrit of 50%. In the modified PRPs, either 5% and 25% of the volume were replaced by PBS (PRP1 and PRP2, controls) or by a 40 x MV vesicle suspension that result in MV concentrations in the reconstituted blood sample equal or in 5 times excess to that measured in the supernatant of stored PRBC units (1 x MV and 5 x MV, respectively).
Statistical analyses were performed using SPSS 25 (IBM Statistics) and Prism 6 (GraphPad Software, Inc.). Changes of PS exposure and hemolysis over time were analyzed using one-way ANOVA with multiple comparison testing. Pairwise comparisons were performed by paired Student’s t-test.
In this study we addressed the question whether prolonged storage affects coagulation properties of erythrocytes. For this purpose, erythrocytes collected from PRBC units were combined with freshly prepared, autologous or heterologous matched PRP. The coagulation properties of these reconstituted blood samples were assayed by ROTEM. In the first part of the study, PRBC units were prepared from blood donated by healthy participants and stored for up to 42 days at 4°C. Hemolysis and the number of cells with phosphatidylserine (PS) exposed at their surface were assessed on a weekly basis. In line with previous studies (Lu et al., 2011; Dinkla et al., 2014; Mittag et al., 2015), both markers significantly increased during storage. At 6 weeks, 0.59 ± 0.16% of cells in PRBCs had hemolyzed and 1.08 ± 0.32% of cells exposed PS at the outer membrane leaflet (Figure 1A). After 2, 4 and 6 weeks, fresh blood samples were obtained from the participants to prepare PRP. Aliquots from the PRBC units were drawn, cell pellets prepared, washed and combined with autologous PRP to give reconstituted blood samples of 50% hematocrit. Coagulation properties of these samples were assessed in INTEM and EXTEM ROTEM assays. Under these conditions, CTs increased from 253 ± 19 to 346 ± 109 s in INTEM assays and from 87 ± 10 to 100 ± 17 s in EXTEM assays between weeks 2 and 6 of storage, respectively (Figure 1B). The increase in CT values was significant both between week 2 and 6 and between week 4 and 6. Further, CFTs significantly increased between week 2 and 6 in INTEM assays (Figure 1B; Table 1). These data implicate that prolonged storage either diminishes prothrombotic properties or induces antithrombotic properties in PRBCs. This was unexpected since the fraction of PS positive cells - a potential procoagulant factor - was significantly higher in PRBCs at week 6 than at week 2 (Figure 1A). To evaluate the effect of blood fractionation and recombination on coagulation parameters, control blood samples reconstituted from freshly prepared RBCs and PRP to yield 50% hematocrit were compared with unprocessed blood samples. Reconstituted samples revealed similar CTs but considerably increased CFT values (Supplementary Table S2) both in INTEM and EXTEM assays. The latter might be explained by some loss in platelet counts during PRP preparation.
FIGURE 1. Coagulation properties of PRBCs are progressively impaired during storage. PRBCs from blood donated by 12 healthy volunteers were stored for up to 6 weeks. (A) Aliquots were removed weekly and tested for the percentage of erythrocytes that had undergone hemolysis (top panel) or that exposed phosphatidylserine (PS) (bottom panel), respectively. Analyses were performed using repeated-measures-ANOVA with Dunnett’s multiple comparison. Significant changes from base values (week 0) are indicated for p < 0.05 *, p < 0.01 **, p < 0.001 *** and p < 0.0001 ****. (B) In weeks 2, 4 and 6, aliquots drawn from PRBC units were recombined with autologous platelet-rich plasma (PRP) prepared from fresh blood donations and tested by thromboelastometry applying both INTEM and EXTEM assays. Clotting times (CT) and clot formation times (CFT) are shown in Box Whisker blots with mean values indicated by crosses. Statistically significant differences were assessed by paired t-tests and indicated by stars (*p ≤ 0.05). Numerical values of the data in Figure 1B are given in Table 1.
TABLE 1. Storage time-dependent change in coagulation characteristics in blood samples reconstituted with PRBCs.
In the second part of the study, we modified the reconstitution scheme by comparing fresh, autologous erythrocytes and heterologous erythrocytes harvested from ABO- and rhesus-matched PRBCs after 5 or 6 weeks of storage. Moreover, to assess the effect of MVs that accumulate in PRBC units on coagulation properties, MVs were prepared from the supernatant of PRBCS, and were added to reconstituted samples. In these samples, fractions of PRP were replaced by corresponding amounts of MV suspensions to achieve final MV concentrations equivalent (1 x MV) or in 5 times excess (5 x MV) to that measured in the supernatant of stored PRBC units. Control samples had the respective fractions of PRP replaced by PBS (PRP1 and PRP2).
As compared to fresh, autologous RBCs, stored ABO-matched heterologous RBCs collected from PRBCs and reconstituted with PRP1 (Figure 2; Table 2) or PRP2 (Table 2) revealed increased CT and CFT values both in INTEM and EXTEM assays. Apart from CFT in EXTEM assays, the effects were considerable (20%–41% increase in CT and CFT values, respectively) and significant. Similarly, a paired comparison of erythrocytes from stored PRBCs versus erythrocytes from fresh blood reconstituted with MV-supplemented PRP (see below) showed significantly increased CTs and CFTs, this time also in CFT EXTEM (Table 2). Together, these data indicate that erythrocytes from stored PRBCs loose some inherent coagulation-promoting property upon storage for 5–6 weeks as compared to fresh autologous erythrocytes.
FIGURE 2. Coagulability is reduced in samples reconstituted with erythrocytes from stored PRBCs as compared to fresh erythrocytes. Freshly prepared PRP (PRP1 as described in the text) from healthy donors was combined with fresh autologous erythrocytes or with erythrocytes from AB0- and rhesus-matched PRBC units that had been stored for 5–6 weeks. Reconstituted blood samples were tested by thromboelastometry applying both INTEM and EXTEM assays. Clotting times (CT) and clot formation times (CFT) are shown in Box Whisker blots with the mean values indicated by crosses. Statistically significant differences were assessed by paired t-tests and indicated by stars (**p ≤ 0.01; ***p ≤ 0.001). Numerical values are given in Table 2 (note that this data set corresponds to blood samples reconstituted with PRP1).
TABLE 2. Coagulation parameters in blood samples reconstituted with erythrocytes from stored PRBCs compared to fresh erythrocytes.
Lastly, to assess the contribution of PRBC-derived MVs on coagulation, blood samples reconstituted with PRP1 and PRP2 were compared with those reconstituted with 1 x MV and 5 x MV, respectively. In samples with fresh erythrocytes, the presence of MVs did not significantly alter CT values in INTEM or EXTEM assays (Figure 3; Table 3A). A significantly decreased CT value (reduced by 13% in vesicle-containing samples) was only seen in erythrocytes from stored PRBCs combined with 5 x MV (Table 3B). In contrast, INTEM CFT values were significantly increased in 1 x MV and 5 x MV samples, both when combined with fresh erythrocytes and stored erythrocytes from PRBC units. Samples with fresh erythrocytes revealed CFT times prolonged by 12% and 18% in the presence of equivalent doses or excess doses of MVs, respectively (Table 3A,B). Stored erythrocytes from PRBCs combined with MVs had the respective CFT times prolonged by 12% and 8% (Table 3A,B). These findings reveal non-uniform effects of MVs on the coagulation process and, unexpectedly, a bias towards an anti-hemostatic impact of MVs in the reconstituted samples.
FIGURE 3. Microvesicles from the supernatant of PRBC units elicit only minor effects on coagulability of reconstituted blood samples. Microvesicles (MVs) isolated from the supernatant of PRBC units. Platelet-rich plasma (PRP) was prepared from freshly drawn blood samples and fractions (5% or 25%) of the PRP were replaced by MV suspensions to give final MV concentrations equivalent (1 x MV) and in 5 times excess (5 x MV) to that in the PRBC unit, (A, B) respectively. Control samples had the respective fractions of PRP replaced by PBS (PRP1 and PRP2). Blood samples reconstituted from these PRP preparations and fresh autologous erythrocytes were tested by thromboelastometry applying both INTEM and EXTEM assays. Clotting times (CT) and clot formation times (CFT) are shown in Box Whisker blots with the mean values indicated by crosses. Statistically significant differences were assessed by paired t-tests and indicated by stars (*p ≤ 0.05; **p ≤ 0.01). Numerical values are given in Table 3A,B (note that these data sets correspond to blood samples reconstituted with fresh RBCs).
Using rotational thromboelastometry (ROTEM) as a tool to more closely mimic in vivo-like conditions, the prime finding of our study is that storage-associated changes in erythrocytes from stored PRBCs alter inherent coagulation properties. This finding was validated 1) in the time course experiment comparing PRBCs stored for longer versus shorter periods upon reconstitution with autologous PRP (Figure 1B; Table 1) and 2) in the comparison of fresh autologous RBCs with heterologous, AB0- and rhesus-matched erythrocytes from PRBCs stored for prolonged periods (Figure 2; Table 2). Clotting times were significantly prolonged upon storage for 6 weeks (as compared to 2 weeks) despite the higher proportion of PS-positive cells in these PRBCs (Figure 1A). Surface-exposed PS is generally considered as a procoagulant factor promoting assembly of components of the coagulation cascade, thereby accelerating thrombin generation. Thus, our findings implicate that the PS-dependent procoagulant contribution is more than outweighed by an anticoagulant property of PRBCs attained upon prolonged storage. This anticoagulant property of erythrocytes from stored PRBCs became even more evident when these were compared to fresh erythrocytes by ROTEM, revealing significant effects on CTs and CFTs (Figure 2 and Table 2). The latter suggests that some process involving the interaction with fibrin fibers and/or platelets likely deteriorates during prolonged storage of PRBCs. Our data are in line with recent findings from a murine blood banking study describing a loss in coagulability of aged PRBCs (Pulliam et al., 2020), thereby implicitly corroborating the emerging view that RBCs play an active role in physiological hemostasis (Weisel and Litvinov, 2019). At present, we can only speculate about molecular mechanisms mediating the functional involvement of erythrocytes in coagulation, but the procoagulant response of erythrocytes upon exposure to platelet-derived lysophosphatidic acid (LPA) can be considered as a potential factor (discussed further below).
The second result of our study was the observation that MVs shed by erythrocytes during prolonged storage have a rather small impact on coagulation when present in the reconstituted samples. The only statistically significant prothrombotic effect was observed when MVs were added to PRBC samples in 5 times excess resulting in a shortening of the clotting time by 13% in INTEM assays (Table 3B). While this particular finding is in line with the prevailing view on MVs (PS-rich vesicular surfaces accelerating thrombin generation) it vanishes when MVs were added to samples in amounts that reflect the vesicle/erythrocyte ratios in the PRBC unit. In contrast, the presence of vesicles (both in equivalent and excess amounts) significantly prolonged CFT in INTEM assays (Figure 3; Table 3). This finding suggests that vesicles disturb the process of clot formation probably by interfering with the interactions between erythrocytes and platelets and/or the crosslinking of fibrin filaments. A recent study by Fischer et al. reported a reduction in clotting times in INTEM assays by 18% when vesicles from the supernatant of PRBC units were added to blood samples (Fischer et al., 2017). They also observed an - albeit statistically not significant–increase in CFT values. Although roughly in line with our findings, it is difficult to directly compare results of both studies due to differences in vesicle preparation and blood sample composition.
There is currently no consensus as to the effect of transfusion on hemostasis. Several studies report an association between PRBC transfusion and increased venous thromboembolic risk, suggesting a procoagulant effect (Goel et al., 2018; Rothstein et al., 2019). However, in non-variceal gastrointestinal hemorrhage, blood transfusion within 24 h of presentation was associated with increased mortality (Taha et al., 2011) and specifically with an increased risk of re-bleeding (Restellini et al., 2013), suggesting a possible anti-hemostatic effect. However, this effect could also be attributed to increased portal vein pressures following transfusion. Conceivably, pro- and anti-hemostatic effects of PRBC transfusions involve different mechanisms with potentially detrimental effects depending on the pathological background of the recipient. One can envisage hypothetical scenarios where blood transfusions may be associated with either increased bleeding or an enhanced thromboembolic risk. Physiological clotting requires the concerted interplay of plasmatic with cellular factors resulting in a rapid, avalanche-like, yet controlled process of local clot formation. Assuming a central role of erythrocytes in this process, a high responsiveness of erythrocytes to local triggers like platelet-secreted LPA may be crucial. The fast presentation of PS at the surface of erythrocytes upon exposure to LPA and the efficient release of MVs could be essential to initiate and confine clot formation at/to the site of vascular injury. PRBC storage lesions could affect the responsiveness of erythrocytes by reducing the efficacy of their intracellular signaling for rapid PS exposure and the membrane flexibility for vesicle shedding. This, however, is no contradiction to the fact that a fraction of erythrocytes in aged PRBCs already expose PS at their surface. Distributing such erythrocytes and MVs systemically throughout the circulatory system may sequester some clotting factors and - by lowering their availability - may hamper rather than promote local physiological clot formation. This could possibly explain transfusion-associated re-bleeding in non-variceal gastrointestinal hemorrhage. On the contrary, MVs from aged PRBCs were shown to induce pulmonary microthrombus formation in a mouse model (Kim et al., 2020). Part of the prothrombotic activity was mediated by endothelial cells activated by vesicle exposure. Similarly, MVs from PRBC units can elicit effects on neutrophils, platelets and monocytes (Jank and Salzer, 2011; Kent et al., 2014; Fischer et al., 2017). Thus, one may assume that, upon transfusion, MVs become absorbed by endothelial and blood cells, and their thrombogenic effect is mainly exerted indirectly via activation of these cells rather than by direct participation in coagulation. Moreover, procoagulant MVs are not only produced during storage, but also after transfusion within the recipient. This is corroborated by results from a recent study using a model of recipient-mimicking conditions. After in vitro reconstitution of stored PRBCs with recipient blood, PS-positive MVs progressively increased over time. Clearly, these transfusion-induced MVs were not experimentally accessible in our study (Tzounakas et al., 2022).
Two limitations of this ROTEM-based study should shortly be addressed: 1) even though ROTEM analyses entail a broad assessment of coagulation properties, hemostasis in vivo is also influenced by vasculature, blood cells and hemodynamic factors. These interactions are experimentally inaccessible by ROTEM, thereby posing a general limit to these analyses in simulating coagulation processes in vivo. 2) The experimental approach of this study required the fractionation and preparation of blood components (fresh PRP, fresh erythrocytes, erythrocytes from stored PRBCs and MVs) and the reconstitution of these components into “artificial” blood samples. Comparing ROTEM values of fresh unprocessed blood with that of samples reconstituted from fresh erythrocytes and PRP revealed similar clotting times, yet increased clot formation times (Supplementary Table S2) both in INTEM and EXTEM assays, indicating a modulation of coagulation properties in reconstituted samples. However, a pairwise comparison with respective direct controls allowed to dissect the relative influences of RBC storage and MVs on the coagulation process. ROTEM analyses of experimentally reconstituted blood samples could and should further be exploited to address the effects of other blood cells, platelet counts and plasma factors on coagulation and may thereby be established as a valuable complementary approach in hemostasis research.
Taken together, our results and these considerations suggest that transfusion of PRBC units stored for prolonged periods might affect two rather independent phenomena: a thrombogenic effect due to activation of cells in the circulatory system by co-transfused MVs and an anti-hemostatic effect due to changes in erythrocytes from stored PRBCs that impair their physiological contribution to coagulation. This can be either viewed as loss of a physiologic erythrocyte function in coagulation or as a storage-induced gain in a coagulation-retarding property. Future studies will have to delineate molecular mechanisms that enroll erythrocytes in physiologic hemostasis and the alteration of this function in erythrocytes from stored PRBC units. This functional alteration, however, should be added to the list of changes in PRBCs that are commonly addressed as “storage lesions”.
The original contributions presented in the study are included in the article/Supplementary Material, further inquiries can be directed to the corresponding author.
The studies involving human participants were reviewed and approved by Ethics Committee of the Medical University of Vienna (1043/2015). The patients/participants provided their written informed consent to participate in this study.
TÖ, DB, EM, and US designed the study. TÖ, MF, MW, and EM acquired the data. TÖ, EM, MF, MW, JB, CJ, DB, and US analyzed and contributed to the interpretation of the data. US drafted the manuscript. EM and DB revised the manuscript critically. All authors approved the final version.
The authors declare that the research was conducted in the absence of any commercial or financial relationships that could be construed as a potential conflict of interest.
All claims expressed in this article are solely those of the authors and do not necessarily represent those of their affiliated organizations, or those of the publisher, the editors and the reviewers. Any product that may be evaluated in this article, or claim that may be made by its manufacturer, is not guaranteed or endorsed by the publisher.
The Supplementary Material for this article can be found online at: https://www.frontiersin.org/articles/10.3389/fphys.2022.1021553/full#supplementary-material
SUPPLEMENTARY TABLE S1 | Characteristics of blood donors.
SUPPLEMENTARY TABLE S2 | Comparison of coagulation properties between whole blood and reconstituted blood samples. Freshly drawn blood samples were either left unprocessed (whole blood sample) or separated in platelet-rich plasma (PRP) and erythrocytes, and further recombined in a 1:1 volume ratio (reconstituted blood sample). These blood samples were assessed by thromboelastometry applying both INTEM and EXTEM assays. Clotting time (CT) and clot formation time (CFT) are given in seconds, A10 and MCF in millimeters and α angle in degree°. The results are given in mean values ± standard deviations. Significant differences between the samples are indicated in bold with p-values ≤ 0.05.
Aung H. H., Tung J. P., Dean M. M., Flower R. L., Pecheniuk N. M. (2017). Procoagulant role of microparticles in routine storage of packed red blood cells: Potential risk for prothrombotic post-transfusion complications. Pathology 49 (1), 62–69. Epub 2016/12/03. doi:10.1016/j.pathol.2016.10.001
Chung S. M., Bae O. N., Lim K. M., Noh J. Y., Lee M. Y., Jung Y. S., et al. (2007). Lysophosphatidic acid induces thrombogenic activity through phosphatidylserine exposure and procoagulant microvesicle generation in human erythrocytes. Arterioscler. Thromb. Vasc. Biol. 27 (2), 414–421. Epub 2006/11/18. doi:10.1161/01.ATV.0000252898.48084.6a
Cines D. B., Lebedeva T., Nagaswami C., Hayes V., Massefski W., Litvinov R. I., et al. (2014). Clot contraction: Compression of erythrocytes into tightly packed polyhedra and redistribution of platelets and fibrin. Blood 123 (10), 1596–1603. Epub 2013/12/18. doi:10.1182/blood-2013-08-523860
Desai P. C., Deal A. M., Pfaff E. R., Qaqish B., Hebden L. M., Park Y. A., et al. (2015). Alloimmunization is associated with older age of transfused red blood cells in sickle cell disease. Am. J. Hematol. 90 (8), 691–695. Epub 2015/05/13. doi:10.1002/ajh.24051
Dinkla S., Peppelman M., Van Der Raadt J., Atsma F., Novotny V. M., Van Kraaij M. G., et al. (2014). Phosphatidylserine exposure on stored red blood cells as a parameter for donor-dependent variation in product quality. Blood Transfus. 12 (2), 204–209. Epub 2013/10/15. doi:10.2450/2013.0106-13
Du V. X., Huskens D., Maas C., Al Dieri R., de Groot P. G., de Laat B. (2014). New insights into the role of erythrocytes in thrombus formation. Semin. Thromb. Hemost. 40 (1), 72–80. Epub 2013/12/21. doi:10.1055/s-0033-1363470
Dubovoy T., Engoren M. (2016). Thrombotic risks in red blood cell transfusions. Semin. Thromb. Hemost. 42 (2), 102–111. Epub 2016/02/04. doi:10.1055/s-0035-1569069
Fischer D., Bussow J., Meybohm P., Weber C. F., Zacharowski K., Urbschat A., et al. (2017). Microparticles from stored red blood cells enhance procoagulant and proinflammatory activity. Transfusion 57 (11), 2701–2711. Epub 2017/08/03. doi:10.1111/trf.14268
Goel R., Patel E. U., Cushing M. M., Frank S. M., Ness P. M., Takemoto C. M., et al. (2018). Association of perioperative red blood cell transfusions with venous thromboembolism in a north American registry. JAMA Surg. 153 (9), 826–833. Epub 2018/06/14. doi:10.1001/jamasurg.2018.1565
Hermand P., Gane P., Huet M., Jallu V., Kaplan C., Sonneborn H. H., et al. (2003). Red cell ICAM-4 is a novel ligand for platelet-activated alpha IIbbeta 3 integrin. J. Biol. Chem. 278 (7), 4892–4898. Epub 2002/12/13. doi:10.1074/jbc.M211282200
Ho K. M., Pavey W. (2017). Applying the cell-based coagulation model in the management of critical bleeding. Anaesth. Intensive Care 45 (2), 166–176. Epub 2017/03/08. doi:10.1177/0310057X1704500206
Jank H., Salzer U. (2011). Vesicles generated during storage of red blood cells enhance the generation of radical oxygen species in activated neutrophils. ScientificWorldJournal. 11, 173–185. Epub 2011/01/25. doi:10.1100/tsw.2011.25
Kent M. W., Kelher M. R., West F. B., Silliman C. C. (2014). The pro-inflammatory potential of microparticles in red blood cell units. Transfus. Med. 24 (3), 176–181. Epub 2014/05/03. doi:10.1111/tme.12123
Kim Y., Goodman M. D., Jung A. D., Abplanalp W. A., Schuster R. M., Caldwell C. C., et al. (2020). Microparticles from aged packed red blood cell units stimulate pulmonary microthrombus formation via P-selectin. Thromb. Res. 185, 160–166. Epub 2019/12/11. doi:10.1016/j.thromres.2019.11.028
Lu C., Shi J., Yu H., Hou J., Zhou J. (2011). Procoagulant activity of long-term stored red blood cells due to phosphatidylserine exposure. Transfus. Med. 21 (3), 150–157. Epub 2011/01/05. doi:10.1111/j.1365-3148.2010.01063.x
Mittag D., Sran A., Chan K. S., Boland M. P., Bandala-Sanchez E., Huet O., et al. (2015). Stored red blood cell susceptibility to in vitro transfusion-associated stress conditions is higher after longer storage and increased by storage in saline-adenine-glucose-mannitol compared to AS-1. Transfusion 55 (9), 2197–2206. Epub 2015/05/15. doi:10.1111/trf.13138
Ohlinger T., Mullner E. W., Fritz M., Sauer T., Werning M., Baron D. M., et al. (2020). Lysophosphatidic acid-induced pro-thrombotic phosphatidylserine exposure and ionophore-induced microvesiculation is mediated by the scramblase TMEM16F in erythrocytes. Blood Cells Mol. Dis. 83, 102426. Epub 2020/03/31. doi:10.1016/j.bcmd.2020.102426
Prosenz J., Ohlinger T., Mullner E. W., Marculescu R., Gerner C., Salzer U., et al. (2019). Glycated hemoglobin concentrations of red blood cells minimally increase during storage under standard blood banking conditions. Transfusion 59 (2), 454–457. Epub 2019/02/07. doi:10.1111/trf.14956
Pulliam K. E., Joseph B., Morris M. C., Veile R. A., Schuster R. M., Makley A. T., et al. (2020). Innate coagulability changes with age in stored packed red blood cells. Thromb. Res. 195, 35–42. Epub 2020/07/12. doi:10.1016/j.thromres.2020.06.047
Restellini S., Kherad O., Jairath V., Martel M., Barkun A. N. (2013). Red blood cell transfusion is associated with increased rebleeding in patients with nonvariceal upper gastrointestinal bleeding. Aliment. Pharmacol. Ther. 37 (3), 316–322. Epub 2012/12/05. doi:10.1111/apt.12170
Rocca B., FitzGerald G. A. (1997). Simply read: Erythrocytes modulate platelet function. Should we rethink the way we give aspirin? Circulation 95 (1), 11–13. Epub 1997/01/07. doi:10.1161/01.cir.95.1.11
Rothstein D. H., Cairo S. B., Schaefer B. A., Lautz T. B. (2019). Association of perioperative red blood cell transfusion with postoperative venous thromboembolism in pediatric patients: A propensity score matched analysis. Pediatr. Blood Cancer 66 (10), e27919. Epub 2019/07/13. doi:10.1002/pbc.27919
Rubin O., Crettaz D., Tissot J. D., Lion N. (2010). Microparticles in stored red blood cells: Submicron clotting bombs? Blood Transfus. 8 (3), s31–s38. Suppl Epub 2010/07/08. doi:10.2450/2010.006S
Rubin O., Delobel J., Prudent M., Lion N., Kohl K., Tucker E. I., et al. (2013). Red blood cell-derived microparticles isolated from blood units initiate and propagate thrombin generation. Transfusion 53 (8), 1744–1754. Epub 2012/12/12. doi:10.1111/trf.12008
Salzer U., Zhu R., Luten M., Isobe H., Pastushenko V., Perkmann T., et al. (2008). Vesicles generated during storage of red cells are rich in the lipid raft marker stomatin. Transfusion 48 (3), 451–462. Epub 2007/12/11. doi:10.1111/j.1537-2995.2007.01549.x
Spadaro S., Taccone F. S., Fogagnolo A., Fontana V., Ragazzi R., Verri M., et al. (2017). The effects of storage of red blood cells on the development of postoperative infections after noncardiac surgery. Transfusion 57 (11), 2727–2737. Epub 2017/08/07. doi:10.1111/trf.14249
Taha A. S., McCloskey C., Craigen T., Angerson W. J., Shah A. A., Morran C. G. (2011). Mortality following blood transfusion for non-variceal upper gastrointestinal bleeding. Frontline Gastroenterol. 2 (4), 218–225. Epub 2011/10/01. doi:10.1136/fg.2011.004572
Tzounakas V. L., Anastasiadi A. T., Karadimas D. G., Velentzas A. D., Anastasopoulou V. I., Papageorgiou E. G., et al. (2022). Early and late-phase 24 h responses of stored red blood cells to recipient-mimicking conditions. Front. Physiol. 13, 907497. Epub 2022/06/21. doi:10.3389/fphys.2022.907497
Vamvakas E. C., Blajchman M. A. (2010). Blood still kills: Six strategies to further reduce allogeneic blood transfusion-related mortality. Transfus. Med. Rev. 24 (2), 77–124. Epub 2010/03/23. doi:10.1016/j.tmrv.2009.11.001
Van Der Meijden P. E., Van Schilfgaarde M., Van Oerle R., Renne T., ten Cate H., Spronk H. M. (2012). Platelet- and erythrocyte-derived microparticles trigger thrombin generation via factor XIIa. J. Thromb. Haemost. 10 (7), 1355–1362. Epub 2012/04/28. doi:10.1111/j.1538-7836.2012.04758.x
Weisel J. W., Litvinov R. I. (2019). Red blood cells: The forgotten player in hemostasis and thrombosis. J. Thromb. Haemost. 17 (2), 271–282. Epub 2019/01/09. doi:10.1111/jth.14360
Whiting P., Al M., Westwood M., Ramos I. C., Ryder S., Armstrong N., et al. (2015). Viscoelastic point-of-care testing to assist with the diagnosis, management and monitoring of haemostasis: A systematic review and cost-effectiveness analysis. Health Technol. Assess. 19 (58), 1–228. v-vi. Epub 2015/07/29. doi:10.3310/hta19580
Yang L., Andrews D. A., Low P. S. (2000). Lysophosphatidic acid opens a Ca(++) channel in human erythrocytes. Blood 95 (7), 2420–2425. Epub 2000/03/25. doi:10.1182/blood.v95.7.2420
Yoshida T., Prudent M., D'Alessandro A. (2019). Red blood cell storage lesion: Causes and potential clinical consequences. Blood Transfus. 17 (1), 27–52. Epub 2019/01/18. doi:10.2450/2019.0217-18
Keywords: packed red blood cells, clotting, transfusion, microvesicles, thromboelastometry, erythrocytes, clotting time, clot formation time
Citation: Öhlinger T, Müllner EW, Fritz M, Werning M, Baron-Stefaniak J, Jungbauer C, Baron DM and Salzer U (2022) Storage of packed red blood cells impairs an inherent coagulation property of erythrocytes. Front. Physiol. 13:1021553. doi: 10.3389/fphys.2022.1021553
Received: 17 August 2022; Accepted: 08 November 2022;
Published: 25 November 2022.
Edited by:
Giampaolo Minetti, University of Pavia, ItalyReviewed by:
Vassilis L. Tzounakas, National and Kapodistrian University of Athens, GreeceCopyright © 2022 Öhlinger, Müllner, Fritz, Werning, Baron-Stefaniak, Jungbauer, Baron and Salzer. This is an open-access article distributed under the terms of the Creative Commons Attribution License (CC BY). The use, distribution or reproduction in other forums is permitted, provided the original author(s) and the copyright owner(s) are credited and that the original publication in this journal is cited, in accordance with accepted academic practice. No use, distribution or reproduction is permitted which does not comply with these terms.
*Correspondence: Ulrich Salzer, dWxyaWNoLnNhbHplckBtZWR1bml3aWVuLmFjLmF0
†These authors have contributed equally to this work
Disclaimer: All claims expressed in this article are solely those of the authors and do not necessarily represent those of their affiliated organizations, or those of the publisher, the editors and the reviewers. Any product that may be evaluated in this article or claim that may be made by its manufacturer is not guaranteed or endorsed by the publisher.
Research integrity at Frontiers
Learn more about the work of our research integrity team to safeguard the quality of each article we publish.