- 1Medical School of Chinese PLA, Beijing, China
- 2Department of Stomatology, The First Medical Center, Chinese PLA General Hospital, Beijing, China
- 3Orthopedic Laboratory of PLA General Hospital, Beijing, China
Extracellular vesicles (EVs), nano-sized bilayer membrane structures containing lipids, proteins and nucleic acids, play key roles in intercellular communication. Compared to stem cells, EVs have lower tumorigenicity and immunogenicity, are easier to manage and cause fewer ethic problems. In recent years, EVs have emerged as a potential solution for tissue regeneration in stomatology through cell-free therapies. The present review focuses on the role of EVs in dental and maxillofacial tissue repair and regeneration, including in dental and periodontal tissue, maxilla and mandible bone, temporomandibular joint cartilage, peripheral nerve and soft tissue. We also make a brief overview on the mechanism of EVs performing functions. However, limitations and challenges in clinical application of EVs still exist and should be addressed in future researches.
1 Introduction
Damage or defects in dental and maxillofacial tissue are usually caused by various factors, such as trauma, infection, inflammation, and tumors. Severe tissue defects will bring substantial pain for patients and seriously affect their quality of life. At present, oral and maxillofacial tissue defects are often directly filled with exogenous materials or replaced with maxillofacial prostheses. However, neither structure nor function can be attained in this way. Achieving physiological repair and the true regeneration of dental and maxillofacial tissues has become a research focus.
Over the past two decades, numerous preclinical and clinical studies have demonstrated the critical role of mesenchymal stem cells (MSCs) in tissue engineering and regenerative medicine, due to their self-renewal, multidirectional differentiation and immune regulation capabilities. These characteristics are shared by different types of oral MSCs, such as dental pulp stem cells (DPSCs) (Zhao et al., 2012; Di Tinco et al., 2021), gingival mesenchymal stem cells (GMSCs) (Nakao et al., 2021) and stem cells from exfoliated deciduous teeth (SHEDs) (Miura et al., 2003). Although the therapeutic effect of MSCs for the treatment of oral diseases has been affirmed, there are certain problems caused by cell therapy. Therefore, as the main component of cell paracrine signaling, extracellular vesicles (EVs) have attracted more attention from scholars.
EVs are membrane vesicles secreted by different types of cells, including immunocytes, blood cells, and MSCs, and have been extracted from a wide range of body fluids including urine, breast milk, saliva, synovial fluid, and plasma (Yáñez-Mó et al., 2015). EVs contain proteins, lipids, nucleic acids (miRNA, mRNA, and DNA), or other bioactivators (Colombo et al., 2014), depending on the type and differentiation status of the parent cells and the stimulating factors in the microenvironment. After interacting with the target cell under certain conditions, the contents of the EVs are released into the target cell, thereby mediating intercellular communication and exerting biological functions (Maia et al., 2018).
EVs are roughly divided into three subtypes according to their size and biogenesis: exosomes, microvesicles, and apoptotic bodies (Théry et al., 2018). Exosomes originate from the endosomal system by inward budding of late endocytic compartments, and have a double-layer lipid structure with a diameter ranging from 30 to 150 nm. Microvesicles (MVs) are regenerated from direct budding of the plasma membrane, with a diameter of approximately 100–1,000 nm. Apoptotic bodies are generally produced during the process of apoptosis, with a diameter of approximately 1–5 μm (van Niel et al., 2018). The formation of apoptotic bodies results from cell decomposition, which is a complex process that varies among types of cells; thus, the cellular components of apoptotic bodies are also different (Díaz-Flores et al., 2018). Different types of EVs have different characteristics based on their distinct contents. The sorting and packaging of EVs cargo are a complex and multi-staged process rather than a random grab (Elsharkasy et al., 2020). Several mechanisms may play a role: 1) Autologous protein composition of EVs affects its biogenesis and release, such as components of the endosomal sorting complex for transport (ESCRT), Rab GTPases, annexin and flotillin (Gutiérrez-Vázquez et al., 2013; Villarroya-Beltri et al., 2014). 2) Some RNA binding proteins are involved in the sorting of miRNA in EVs, which is regulated by specific sequence motifs (Villarroya-Beltri et al., 2013; Santangelo et al., 2016). 3) EVs may contain proteins capable of binding and sorting its own mRNA, such as Arc (Pastuzyn et al., 2018). Researchers have demonstrated that EVs participate in most physiological or pathological processes in cells (You et al., 2020), including signal transduction (Codagnone et al., 2017), cell growth and development (Zheng et al., 2020), metabolism (Akbar et al., 2019), and immune responses (Szekeres-Bartho et al., 2018). The advantages of EVs also include effectively reducing the possibility of tumorigenesis, immunological rejection and ethical issues caused by cell therapy. Moreover, their effectiveness lasted for approximately 6 months under appropriate storage conditions (Yu et al., 2014). Therefore, the application of EVs is expected to prevent the adverse consequences of cell therapy in oral and maxillofacial tissue repair and promote the development of regenerative medicine.
In summary, due to the limitations of stem cell therapy, cell-free therapy has become a research focus for the physiological repair and regeneration of defective tissue. Using different EV sources to repair different tissue types is a sensible strategy to achieve better effects. The use of EVs to repair oral and maxillofacial tissue defects is reviewed in more detail below (Figure 1).
2 The use of EVs in oral and maxillofacial tissue repair and regeneration
2.1 Dental tissue
Dental tissue includes enamel, dentin, pulp and cementum. Pulp, a critical structure rich in cells, blood vessels and nerves, is protected by mineralized enamel and dentin. If caries is not treated properly and timely, bacteria will easily enter the pulp system and affect pulp tissue, leading to irreversible pulpitis or pulp necrosis that requires root canal treatment. However, after the treatment, teeth lose their nutrient supply and are prone to discoloration or even fracture, which is not conducive to maintaining oral cavity health. To achieve pulp tissue regeneration, regenerative endodontic procedures have been proposed as an alternative to conventional pulp treatment.
2.1.1 Pulp
According to some researchers, the core problem of pulp regeneration is whether there is a healthy pulp stump or residual pulp stem cells. Otherwise, the new growth of tissue is not true pulp regeneration but the replacement of pulp with new periodontal tissue (Lui et al., 2020). EVs directly promote the proliferation, migration, odontogenic differentiation and revascularization of pulp stem cells or precursor cells, either of which can speed up pulp regeneration. A study showed that EVs derived from stem cells from the apical papilla (SCAPs) promoted the migration, proliferation and differentiation of endogenous stem cells around the root apex, thereby promoting pulpo-dentinal complex regeneration (Zhuang et al., 2020). Endogenous stem cells refer to mesenchymal stem cells from other body locations; for example, human bone marrow mesenchymal stem cells (BMMSCs) were recruited to the root apex and became an important source of stem cells promoting pulp regeneration (Ivica et al., 2020). Huang et al. demonstrated that exosomes isolated from dental pulp stem cells (DPSC-Exos) increased the secretion of pulp-like tissue by naïve DPSCs and suggested that odontogenic differentiation was promoted by the transfer of microRNAs (miRNAs) in exosomes (Huang et al., 2016). In addition, DPSC-Exos also induced blood vessel regeneration in vitro by promoting the proliferation of endothelial cells and increasing the expression of angiogenic factors (Xian et al., 2018).
2.1.2 Dentin
Dentin is formed by the mineralization of the matrix secreted by odontoblasts. During caries development, a series of autocrine and paracrine signals induce teeth to produce reparative dentin, prevent caries development, and resist pulpo-dentinal complex infection (Couve et al., 2018).
Pulp stem cells reside in the pulp cavity. These cells grow rapidly and can be induced by multiple factors to form odontoblasts and secrete dentin matrix. Swanson et al. utilized the intercellular communication properties of EVs derived from DPSCs to catalyze the formation of a reactionary dentin bridge by recruiting endogenous dental stem cells and upregulating odontogenic gene expression, thereby increasing mineralization in vitro (Swanson et al., 2020). Neural crest-related Schwann cell precursors (SCPs) are an important cellular source of dental MSCs, and able to migrate to the injured site, differentiate into odontoblasts and dental pulp cells (Kaukua et al., 2014). It is found that DPSCs share a common lineage with neural crest-derived SCPs and have neural crest stem features, so they can differentiate to both Schwann cell (SCs) and odontoblast lineages (Uribe-Etxebarria et al., 2017; Luzuriaga et al., 2019). Moreover, DPSC-Exos, especially exosomes derived from lipopolysaccharide (LPS)-pretreated DPSCs, play a critical role in promoting the proliferation, migration and odontogenic differentiation of SCs (Li et al., 2021).
2.1.3 Cementum
Cementum is a thin layer on the root surface that serves as an antiresorptive barrier and anchorage for a functional periodontal ligament (Feller et al., 2016), which is paramount for periodontal regeneration. Extensive literature about stem cells promoting cementum repair has been published. For example, periodontal ligaments mesenchymal stem cells (PDLSCs) are the most favorable stem cell population utilized in periodontal regeneration, due to their high expression of cementoblastic/osteoblastic markers, including alkaline phosphatase, MEPE, bone sialoprotein, osteocalcin, and TGFβ receptor type I, which are responsible for the formation of the cementum-periodontal ligament complex (Seo et al., 2004). Dental Follicle Stem Cells (DFSCs) were also demonstrated to form PDL-like structures or calcified nodules with cementum-like structures, which suggested their potential for periodontal differentiation (Han et al., 2010). A recently published paper showed that the effects of macrophages on cementoblast mineralization were mediated by exosomes. Compared with M0 (unpolarized macrophages), M1-polarized macrophages attenuated cementoblast mineralization, while M2-polarized macrophages enhanced cementoblast mineralization (Zhao et al., 2022). However, there are few articles about EVs promoting cementum regeneration.
2.2 Periodontal tissue
Periodontitis is considered as a global epidemic. Long-standing pathological factors may destroy the supporting periodontal tissue and result in tooth loss. Conventional periodontal treatment can successfully reduce the number of pathogens in periodontal defects. However, no effective methods for regenerating and reconstructing lost tissues have been developed. Since the inflammatory microenvironment caused by periodontitis is the main cause for the abnormal function and regenerative defects of local stem cells, the progression of periodontitis and disease severity is closely related to the host’s immune response. Therefore, many researchers have begun to promote periodontal tissue regeneration by reducing inflammation and mediating immunoregulation.
2.2.1 Alveolar bone
Many studies have shown that EVs increase the bone regenerative capacity of stem cells in periodontitis tissues and alleviate the inhibitory effects of local stem cells caused by inflammation. Research has shown that human periodontal ligament cells (hPDLCs) cultured in conditioned medium of BMMSCs increased expression of bone formation-related genes and proteins (Shyh-Chang and Ng, 2017), which indicates that BMMSC-EVs promoted odontogenic differentiation in hPDLCs. BMMSC-EVs have therapeutic effects on rats with periodontitis. This effect may partly be mediated by BMMSC-EVs-mediated activation of the OPG-RANKL-RANK signaling pathway that regulates the function of osteoclasts and promotes TGF-β1 expression and macrophage M2 polarization, thus inhibiting periodontitis and alveolar bone absorption (Liu et al., 2021). In addition, with powerful anti-inflammatory and immunomodulatory effects, DPSC-Exos effectively promoted alveolar bone reconstruction and periodontal epithelial healing in a mouse model through the exosome-mediated transmission of miR-1246 (Shen et al., 2020). It is also known that the accumulation of intracellular ROS, a mediator of mitogen-activated protein kinase (MAPK) family members, affects MAPK/JNK signaling pathways, which can be inhibited by antioxidants to reduce ROS accumulation (Yang et al., 2018). A recent study showed that in an inflammatory microenvironment, ROS levels were significantly reduced in PDLSCs after being treated with LPS-preconditioned DFSC-EVs, which possibly exhibited their antioxidant effects through the ROS/MAPK signaling pathway, thereby treating periodontitis (Huang et al., 2022).
2.2.2 Periodontal ligament
The density and directional arrangement of fibers in the periodontal ligament (PDL) are closely related to the growth of the cementum, the distribution of masticatory force and the remodeling of alveolar bone. Adipose stem cells (ADSCs) were demonstrated to promote the formation of a new functional PDL by producing more oblique and horizontal fibers with an ideal arrangement and direction (Monsarrat et al., 2014). EVs from adipose stem cells (ADSC-EVs) were injected into the ligature-induced rat periodontal pocket, and then well-organized periodontal tissue was observed perpendicular to the cementum and alveolar bone. Thus, ADSC-EVs enhanced the reconstruction of collagen fibers during PDL wound healing. Moreover, the therapeutic effect of ADSC-EVs was superior to that of ADSCs alone; the former induced a significantly larger newly formed periodontal tissue area (Mohammed et al., 2018), which may be relevant to the different metabolic processes associated with EVs and stem cells in the body. Another study demonstrated that MSC-EVs activated CD73-mediated adenosine receptor activation of the prosurvival AKT and ERK signaling pathways to promote the proliferation and migration of PDLCs, enhance cell viability, improve periodontal ligament function and promote tissue regeneration in a rat periodontal ligament defect model (Chew et al., 2019).
2.3 Temporomandibular joint cartilage
The temporomandibular joint comprises the mandibular condyle, articular facet, articular disc, articular capsule and articular ligaments. The cartilage on the joint surface has a strong protective effect on the bone. Due to the limited self-healing capacity of joint cartilage, temporomandibular joint osteoarthritis (TMJOA) often occurs after excessive wear (Zhao and Xie, 2021). The main treatment strategy is to inhibit the inflammatory response, prevent the gradual destruction of cartilage and subchondral bone, induce cartilage regeneration, and restore function (Wang et al., 2015).
The key to repairing cartilage defects is regulating the inflammatory response to prevent cartilage damage, promote the proliferation and migration of cartilage cells, and increase cartilage matrix synthesis. MiR-100, the product of EVs derived from SHEDs, inhibited the expression of proinflammatory factors (such as IL-6 and IL-8) and matrix metalloproteinases (such as MMP1 and MMP-9), thereby reducing inflammation in the temporomandibular joint and preventing further cartilage damage (Luo et al., 2019). Researchers established a rabbit TMJOA model and found that human umbilical cord blood mesenchymal stem cells (UCB-MSCs) demonstrated robust potential for cartilage protection and regeneration. MSC-EVs also promoted the recovery of TMJOA by regulating inflammation (Kim et al., 2019). In addition, MSC-EVs were proved to enhance the repair of critical-sized osteochondral defects in an adult immunocompetent rat model, and the new hyaline cartilage and underlying subchondral bone closely resembled normal tissue (Zhang et al., 2016). Researchers have suggested that this outcome could be attributed to exosomal CD73-mediated adenosine activation of AKT, ERK, and AMPK signaling, which increases chondrocyte activity and induces cell proliferation and tissue regeneration through kinase phosphorylation (Zhang et al., 2018).
2.4 Maxillofacial soft tissue
In recent years, soft tissue replacement has been increasingly demanded for treating congenital malformation, traumatic repair and postoperative rehabilitation of maxillofacial tumors. Researchers are seeking a stem cell-based therapy or cell-free approach to producing engineered adipose tissue to develop an ideal soft tissue substitute. Exosome-like vesicles derived from adipose tissue induced adipogenesis in ADSCs and promoted the proliferation, migration, and angiogenic potential of aortic endothelial cells (Dai et al., 2017). MiR-450a-5p carried by EVs derived from adipose tissue triggered adipogenic signals in ADSCs by inhibiting the expression of WISP2 and promoting the differentiation of adipose cells (Zhang et al., 2017). In recent years, EVs have also been widely studied in the context of skin damage repair, but there have been few reports on the repair of maxillofacial skin. The main mechanism by which skin defects are repaired has been verified in different phases. For example, ADSC-EVs exerted anti-inflammatory effects by eliciting macrophage switching from an M1 to an M2 phenotype during the inflammatory phase (Lo Sicco et al., 2017), while EVs derived from UCB-MSCs stimulated wound healing by reducing scar formation in the remodeling stage (Zhang et al., 2021). In the proliferative phase, critical steps involve promoting the proliferation and migration of human dermal fibroblasts and human keratinocytes, as well as inducing angiogenic activity in endothelial cells (Zeng and Liu, 2021). Exosomes from human endometrial MSCs promoted the expression of angiogenic markers and the proliferation and migration of human umbilical vein endothelial cells (Ha et al., 2020).
2.5 Peripheral nerves
Peripheral nerve injuries typically lead to motion and sensory dysfunction in different parts of the body. For example, the trigeminal and facial nerves are easily affected by maxillofacial tumors or severe trauma, causing damage to facial sensation and even leading to facial paralysis. Nerve regeneration after injury is a complex process involving the differentiation of SCs, the recruitment of macrophages, the growth of blood vessels and the regeneration of axons (Hercher et al., 2022). Interestingly, dental MSCs, which derived from the neural crest during embryonic development, show stronger nerve regenerative and neuroprotective effects than MSCs derived from other sources (Ibarretxe et al., 2012; Mayo et al., 2014). Furthermore, a study compared three types of dental MSCs (follicle, papilla, and pulp) from a single donor and found that MSCs derived from pulp showed higher neurogenic potential than those from the follicle and papilla (Ullah et al., 2016). Application of conditioned media (CM) or concentrated EVs secreted by dental stem cells have also been found to facilitate nerve repair and regeneration. With a model of polyneuropathy in diabetic rats, DPSC-conditioned media (DPSC-CM) were found to promote neurite outgrowth of dorsal root ganglion neurons and increase the viability and myelin-related protein expression of SCs (Omi et al., 2017). The gene expression of transient receptor potential vanilloid channel-1 (TRPV1), a pain receptor thought to be related to the regeneration of peripheral nerves, can be upregulated after nerve injury, and a TRPV1 antagonist can inhibit the injury process and promote axon regeneration (Ren et al., 2015). However, researchers found that DPSC-CM upregulated neuron-related markers and the gene expression of TRPV1, and promoted the survival and regeneration of isolated primary trigeminal ganglion neuronal cells (TGNCs), thereby repairing damaged trigeminal nerves (Sultan et al., 2020). GMSC-EVs effectively promoted the proliferation and migration of SCs, upregulated the expression of genes associated with the SC repair phenotype, and activated this phenotype to promote the regeneration of peripheral nerves (Mao et al., 2019). In addition, the nerve repairing effect of EVs from other source of cells has also been reported, such as SCs (Ching et al., 2018) or macrophages (Qing et al., 2018) derived EVs. Mechanical stimulation affected intercellular communication between neurons and SCs by changing the miRNA composition of SC-EVs. EVs produced by mechanical stimulation of SCs transferred miR-23b-3p from SCs to neurons, downregulated the expression of neuropilin 1, and promote the repair and regeneration of damaged peripheral nerves to some extent (Xia et al., 2020).
2.6 Tongue
Morbidity associated with oral cancers is high worldwide, and the tongue is the most vulnerable tissue. Currently, the standard method for treating oral cancer is surgical resection of the malignant tumor and involved tissue and reconstruction of the defective tissue with a free flap. Subsequently, postoperative chemotherapy is usually performed. However, using tissue flaps to rebuild the tongue has difficulty in functional restoration, especially taste bud regeneration in the reconstruction area. Thus, this treatment seriously affects patients’ prognosis and quality of life (Mannelli et al., 2018). Therefore, regenerating the lingual papilla and taste buds is the key to successfully rebuilding the tongue. By establishing a critical-sized tongue defect model in rats and combining the transplantation of small intestinal submucosa–extracellular matrix (SIS-ECM) with GMSC-EVs, researchers found that local implantation of exosome/SIS-ECM significantly increased the expression of CK14 and CK8 compared to the SIS-ECM control group, thus enhancing the regeneration of epithelial progenitor cells and facilitating taste bud and lingual papilla restoration (Zhang et al., 2019). Although studies of EV repair of the tongue are rare and have certain limitations, the results suggest that EVs are promising for clinical tongue reconstruction and taste bud regeneration after tongue carcinoma resection (The effects of EVs in dental and maxillofacial tissue repair and regeneration are listed in Table 1).
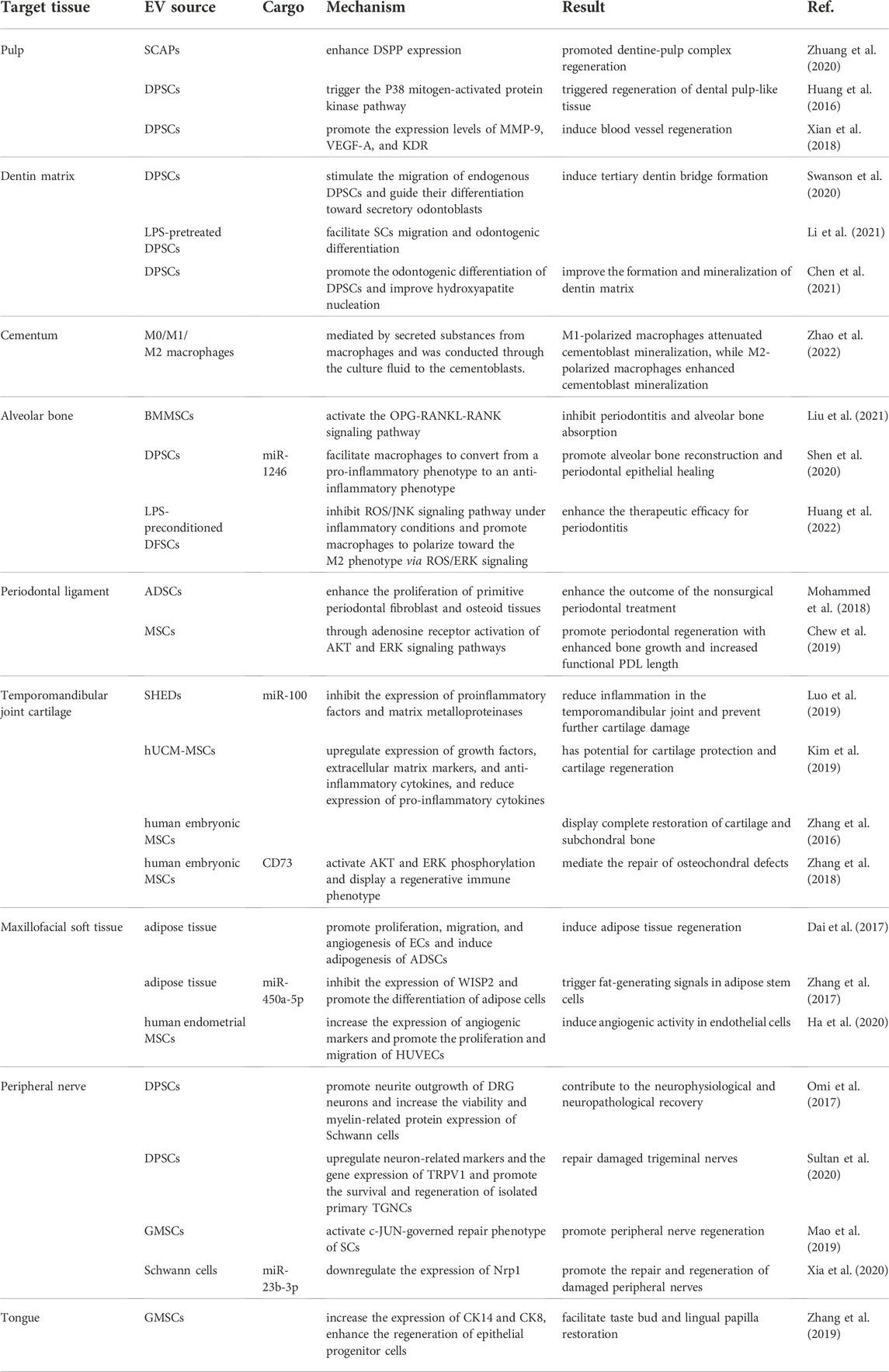
TABLE 1. Outlines of the effect of EVs in dental and maxillofacial tissue repair and regeneration approaches.
3 Mechanism by which EVs promote dental and maxillofacial tissue repair and regeneration
Cell-to-cell communication involving exosome-borne cargo, such as miRNA, has emerged as a critical mechanism for tissue repair. Moreover, EVs can be taken up by various cells such as tissue cells, immune cells, and vascular endothelial cells, thus affecting the tissue repair process in multiple ways. This multifactor synergistic effect may be the key to the strong ability of MSC-EVs to promote tissue regeneration.
3.1 Enhancing proliferation, migration, and differentiation of tissue cells
MSCs regulate the biological properties of local tissue cell by releasing EVs, thereby maintaining tissue homeostasis and regeneration. Regarding wound healing, EVs enhanced the proliferation and migration of keratinocytes, fibroblasts, and endothelial cells (Tutuianu et al., 2021). This effect is often accompanied by upregulation of the PI3K-AKT-mTOR-HIF-1α signaling pathway and blocked by PI3K-AKT inhibitor both in vitro and in vivo (Liu et al., 2021). Another study showed that exosomes mediated the crosstalk between keratinocytes and macrophages in cutaneous wound healing as impairment in miRNA packaging of keratinocyte exosomes inhibited inflammation resolution and compromised functional wound closure (Zhou et al., 2020).
EV-mediated cellular communication between osteoblasts and osteoclasts is a newly discovered mechanism that regulates bone reconstruction. Osteoblasts release EVs containing RANKL and transfer them to the precursor cells of osteoclasts, which then differentiate into osteoclasts by stimulating the OPG/RANKL/RANK signaling pathway, which is critical in bone metabolism (Huynh et al., 2016). Exosomes secreted by SHEDs (SHED-Exos) effectively promoted human umbilical vein endothelial cells (HUVECs) blood vessel formation and osteogenic differentiation in BMMSCs. These effects can be suppressed by an AMPK inhibitor, suggesting that SHED-Exos contribute to bone regeneration by promoting neovascularization and new bone formation through the AMPK signaling pathway (Wu et al., 2019; Liu et al., 2020). In addition, increasing evidence suggests that apoptosis is a crucial link in tissue repair and regeneration, but the exact functions of apoptotic events remain largely unclear (Li et al., 2020). Apoptosis is closely related to cell proliferation (Pellettieri et al., 2010), differentiation (Hristov et al., 2004), and elimination of senescent cells (Jeon et al., 2017), and is essential for the maintenance of normal tissue homeostasis (Gaipl et al., 2005). During apoptosis, BMMSCs produce large amounts of apoptotic cell-derived extracellular vesicles. These EVs activate JNK signal transduction by increasing intracellular reactive oxygen species levels, effectively promoting the proliferation, migration, and osteogenic differentiation of BMMSCs and accelerating new bone formation (Li et al., 2022).
3.2 Preventing apoptosis of tissue cells
Despite promoting cell proliferation, migration and differentiation, EVs also facilitate the survival of recipient cells. Under pathological conditions such as hypoxia and inflammation, MSC-EVs promote tissue regeneration by maintaining tissue cell survival and preventing apoptosis. For example, EVs suppressed the inflammatory immune microenvironment, which is often enriched by proinflammatory immune cells and cytokines that reduce chondrocytes apoptosis (Yin et al., 2022). Another study revealed that hucMSC-EVs inhibited chondrocyte apoptosis by lowering the m6A level of NLRP3 mRNA with miR-1208 targeting combined with METTL3 (Zhou et al., 2022).
Neurons are a special kind of tissue cells and EVs can also promote nerve regeneration by inhibiting neuronal apoptosis. Researchers have found that BMMSC-EVs prevented hippocampal neuron apoptosis induced by oxygen and glucose deprivation by regulating the JMJD3/p53/KLF2 axis, which caused miR-93 to be transferred into hippocampal neurons (Luo et al., 2022). MSC-EVs reduced apoptosis and inflammation, and promoted angiogenesis after spinal cord injury, and miR-21-5p was one of the most highly expressed miRNAs in these EVs. Moreover, inhibition of miR-21-5p in MSC-EVs significantly reversed the beneficial effects of EVs on motor function and apoptosis, an effect that was associated with modulating FasL expression (Zhou et al., 2019).
3.3 Increasing mineralization
Mineralization is one of the most important steps in repairing and regenerating teeth, alveolar bone and the jawbone. Exogenous administration of osteogenic exosomes increased BMMSC mineralization in a dose-dependent manner, and the increase in mineralization was accompanied by upregulation of the expression of osteogenic markers including osteocalcin, bone sialoprotein and RUNX family transcription factor 2 (RUNX2), and increased protein secretion of the same gene products (Swanson et al., 2020). A study showed that mineralizing preosteoblast MC3T3-E1 exosomes had a significant promotion effect on bone marrow stromal cell osteogenic differentiation, as manifested by upregulated expression of the osteogenic marker genes RUNX2 and ALP, as well as enhanced matrix mineralization (Cui et al., 2016). In addition, during dentin formation, calcium mobilization and Ca2+ entry across the plasma membrane by odontoblasts are key physiological events. STIM1 is a Ca2+ concentration sensor located in the endoplasmic reticulum. When STIM1 expression is upregulated, Ca2+ is transferred to the extracellular matrix (ECM), and DPSCs release more EVs carrying minerals and organic matrix. These EVs are capable of promoting the odontogenic differentiation of DPSCs and improving hydroxyapatite nucleation, thus playing a key role in the differentiation of pulp stem cells and the formation and mineralization of dentin matrix (Chen et al., 2021).
3.4 Promoting angiogenesis
Blood vessels transmit mineral substances, growth factors and various progenitor cells to corresponding regions to participate in regenerative activity and help maintain homeostasis. Rapid vascular regeneration and reconstruction provide sufficient oxygen and nutrients for tissue and cells (Grosso et al., 2017). Angiogenesis is also a dynamic process based on vascular endothelial cells (ECs) and their surrounding environment. EC proliferation, migration, and tube formation are the foundations of vascularization (Lv et al., 2020). Liu et al. found that EVs derived from human induced pluripotent stem cells promoted the proliferation, migration and angiogenesis of vascular endothelial cells by activating the PI3K/Akt pathway (Liu et al., 2017). MiR-126, miR-130a, and miR-132 in EVs also play important roles in regulating these processes (Zhu et al., 2018). Huang et al. found that LPS-pretreated exosomes from DPSCs changed the expression profiles of miRNAs and activated MAPK signaling by increasing the expression of Kinase insertion domain receptor (KDR/VEGFR2), and also raised the levels of vascular endothelial growth factor (VEGF) expression, which eventually promoted the proliferation, migration and tube formation capacity of HUVECs in vitro (Huang et al., 2021). In addition, vascular damage through different mechanisms can also be inhibited or even reversed by MSC-derived exosomes. Exosomes play a critical role in repairing DNA double-strand breaks and alleviating oxidative damage. Exposure to MSC exosomes reduced apoptosis caused by radiation-induced DNA damage in vascular endothelial cells (Wen et al., 2016).
3.5 Anti-inflammatory effects and immunoregulation
EVs have strong anti-inflammatory and immunoregulatory effects. Researchers have shown that MSC-Exos combined with functionalized scaffolds induced innate and adaptive immunomodulatory responses to guide the biological communication network (Su et al., 2021). The number, size and biologically active material of EVs are altered in numerous inflammatory conditions. Moreover, EVs affected the cellular functions of neutrophils, monocytes, macrophages and their precursor hematopoietic stem and progenitor cells (Akbar et al., 2021). TNF-α stimulation increases the number of exosomes secreted from GMSCs and enhances exosomal expression of CD73, thereby inducing anti-inflammatory M2 macrophage polarization (Nakao et al., 2021). DPSC-Exos inhibit CD4+ T-cell differentiation into T helper cells and induce the transformation of these cells into regulatory T cells, thus elevating the levels of anti-inflammatory factors and playing an immunomodulatory role. In addition, scholars constructed a rat model of bisphosphonate-related osteonecrosis of the jaw and demonstrated that MSC-EVs prevented aging in stem cells, osteoblasts and fibroblasts induced by zoledronic acid and reduced the generation of inflammatory cytokines, thereby preventing bisphosphonate-related jaw necrosis (Watanabe et al., 2020). Moreover, because of their powerful anti-inflammatory properties, EVs have the ability to promote angiogenesis in an inflammatory environment. Studies have shown that inflammation may increase the number of exosomes secreted by PDLSCs. Exosomes released from inflamed PDLSCs promote angiogenesis in HUVECs by upregulating the expression of the vascular-specific marker CD31 and vascular endothelial growth factor (Zhang et al., 2020).
4 Conclusion and prospects
EVs are important factors for cellular communication and material transmission. In regenerative medicine, MSC-EVs have the potential to repair and regenerate oral tissue (Shi et al., 2020), including dental tissue, periodontal tissue, the maxilla and mandible, joint cartilage, maxillofacial soft tissue, peripheral nerves and other important structures. In addition, the source and state of donor cells play important roles in stem cell differentiation and tissue regeneration (Huang et al., 2016). For example, studies have shown that DPMSCs and BMMSCs have therapeutic effects in a rat stroke model, but the decrease in infarct volume in the DPMSC treatment group was more obvious than that in the BMMSC group (Song et al., 2017). The contents of EVs depend on the parent cell type, thus we supposed that EVs derived from these two stem cells might also have different therapeutic effects on stroke. However, aging is an unfavorable factor in mesenchymal stem cells from the perspective of cell-based treatment. The decreased function of aged stem cells is related to the attenuation of tissue regeneration. DPMSCs from young donors are more resistant to apoptosis than those from older donors and exhibit higher nonhomologous end-joining DNA repair activity (Zhai et al., 2017), which may explain why dental pulp stem cells from deciduous teeth are some of the most widely studied cells in oral tissue repair and regenerative medicine.
However, there are also many limitations and challenges in the clinical application of EVs for regenerative treatments. First, an effective method of isolating and purifying EVs from their parent cells or liquid has not been developed. At present, EVs are primarily extracted by ultracentrifugation, immunoadsorption, precipitation or microfluidic separation (Koritzinsky et al., 2017), all of which are cumbersome and error-prone. Therefore, applying these vesicles clinically is difficult due to the low extraction levels. Second, there is still a lack of horizontal comparisons of EVs in tissue repair and regeneration. For example, what is the reparative effect of EVs from different MSCs or what are the differences in composition and function between EV and CM from the same cell remain to be determined in widespread clinical studies. Third, a simple and controllable approach for specifically transporting EVs to injured sites is required for clinical application of EVs.
The combination of EVs and biomaterials has also become a popular research focus. For example, Diomede et al. combined a three-dimensional printed scaffold of PLA and human GMSCs-EVs to promote bone healing in rat skull defects (Diomede et al., 2018). Nooshabadi et al. fabricated a chitosan-glycerol hydrogel loaded with exosomes isolated from human endometrial stem cells, which was used for wound treatment in a mouse model (Nooshabadi et al., 2020). Ideal biomaterials always have superior performance and enhance the effects of extracellular vesicles to a certain extent, thus, finding new materials to combine with EVs for tissue damage repair must be a hot area of research in the future.
Author contributions
ZL and SW collected and summarized the references and wrote the manuscript. NH and SY searched and analyzed the manuscript. QS and JX made substantial contributions to the conception, design, and critical revision of the manuscript. All authors read and approved the final manuscript.
Funding
This work was supported by the National Natural Science Foundation of China (No. 81901034 and 51972339), the Beijing Natural Science Foundation (No. 7212091) and Military Medical Science Foundation (No. 21QNPY104).
Conflict of interest
The authors declare that the research was conducted in the absence of any commercial or financial relationships that could be construed as a potential conflict of interest.
Publisher’s note
All claims expressed in this article are solely those of the authors and do not necessarily represent those of their affiliated organizations, or those of the publisher, the editors and the reviewers. Any product that may be evaluated in this article, or claim that may be made by its manufacturer, is not guaranteed or endorsed by the publisher.
References
Akbar N., Paget D., Choudhury R. P. (2021). Extracellular vesicles in innate immune cell programming. Biomedicines 9 (7), 713. doi:10.3390/biomedicines9070713
Akbar N., Azzimato V., Choudhury R. P., Aouadi M. (2019). Extracellular vesicles in metabolic disease. Diabetologia 62, 2179–2187. doi:10.1007/s00125-019-05014-5
Chen Y., Koshy R., Guirado E., George A. (2021). STIM1 a calcium sensor promotes the assembly of an ECM that contains Extracellular vesicles and factors that modulate mineralization. Acta Biomater. 120, 224–239. doi:10.1016/j.actbio.2020.10.011
Chew J., Chuah S. J., Teo K., Zhang S., Lai R. C., Fu J. H., et al. (2019). Mesenchymal stem cell exosomes enhance periodontal ligament cell functions and promote periodontal regeneration. Acta Biomater. 89, 252–264. doi:10.1016/j.actbio.2019.03.021
Ching R. C., Wiberg M., Kingham P. J. (2018). Schwann cell-like differentiated adipose stem cells promote neurite outgrowth via secreted exosomes and RNA transfer. Stem Cell Res. Ther. 9, 266. doi:10.1186/s13287-018-1017-8
Codagnone M., Recchiuti A., Lanuti P., Pierdomenico A. M., Cianci E., Patruno S., et al. (2017). Lipoxin A(4) stimulates endothelial miR-126-5p expression and its transfer via microvesicles. FASEB J. official Publ. Fed. Am. Soc. Exp. Biol. 31, 1856–1866. doi:10.1096/fj.201600952R
Colombo M., Raposo G., Théry C. (2014). Biogenesis, secretion, and intercellular interactions of exosomes and other extracellular vesicles. Annu. Rev. Cell Dev. Biol. 30, 255–289. doi:10.1146/annurev-cellbio-101512-122326
Couve E., Lovera M., Suzuki K., Schmachtenberg O. (2018). Schwann cell phenotype changes in aging human dental pulp. J. Dent. Res. 97 (3), 347–355. doi:10.1177/0022034517733967
Cui Y., Luan J., Li H., Zhou X., Han J. (2016). Exosomes derived from mineralizing osteoblasts promote ST2 cell osteogenic differentiation by alteration of microRNA expression. FEBS Lett. 590, 185–192. doi:10.1002/1873-3468.12024
Dai M., Yu M., Zhang Y., Tian W. (2017). Exosome-like vesicles derived from adipose tissue provide biochemical cues for adipose tissue regeneration. Tissue Eng. Part A 23 (21), 1221–1230. doi:10.1089/ten.TEA.2017.0045
Di Tinco R., Bertani G., Pisciotta A., Bertoni L., Pignatti E., Maccaferri M., et al. (2021). Role of PD-L1 in licensing immunoregulatory function of dental pulp mesenchymal stem cells. Stem Cell Res. Ther. 12, 598. doi:10.1186/s13287-021-02664-4
Díaz-Flores L., Gutiérrez R., Alvarez-Argüelles H., Díaz-Flores L., González R., Martín-Vasallo P., et al. (2018). Extracellular multivesicular bodies in tissues affected by inflammation/repair and tumors. Ultrastruct. Pathol. 42, 448–457. doi:10.1080/01913123.2018.1534915
Diomede F., Gugliandolo A., Cardelli P., Merciaro I., Ettorre V., Traini T., et al. (2018). Three-dimensional printed PLA scaffold and human gingival stem cell-derived extracellular vesicles: A new tool for bone defect repair. Stem Cell Res. Ther. 9 (1), 104. doi:10.1186/s13287-018-0850-0
Elsharkasy O. M., Nordin J. Z., Hagey D. W., de Jong O. G., Schiffelers R. M., Andaloussi S. E., et al. (2020). Extracellular vesicles as drug delivery systems: Why and how. Adv. Drug Deliv. Rev. 159, 332–343. doi:10.1016/j.addr.2020.04.004
Feller L., Khammissa R. A., Thomadakis G., Fourie J., Lemmer J. (2016). Apical external root resorption and repair in orthodontic tooth movement: Biological events. Biomed. Res. Int. 2016, 4864195. doi:10.1155/2016/4864195
Gaipl U. S., Voll R. E., Sheriff A., Franz S., Kalden J. R., Herrmann M. (2005). Impaired clearance of dying cells in systemic lupus erythematosus. Autoimmun. Rev. 4, 189–194. doi:10.1016/j.autrev.2004.10.007
Grosso A., Burger M. G., Lunger A., Schaefer D. J., Banfi A., Di Maggio N. (2017). It takes two to tango: Coupling of angiogenesis and osteogenesis for bone regeneration. Front. Bioeng. Biotechnol. 5, 68. doi:10.3389/fbioe.2017.00068
Gutiérrez-Vázquez C., Villarroya-Beltri C., Mittelbrunn M., Sánchez-Madrid F. (2013). Transfer of extracellular vesicles during immune cell-cell interactions. Immunol. Rev. 251, 125–142. doi:10.1111/imr.12013
Ha D. H., Kim H. K., Lee J., Kwon H. H., Park G. H., Yang S. H., et al. (2020). Mesenchymal stem/stromal cell-derived exosomes for immunomodulatory therapeutics and skin regeneration. Cells 9 (5), E1157. doi:10.3390/cells9051157
Han C., Yang Z., Zhou W., Jin F., Song Y., Wang Y., et al. (2010). Periapical follicle stem cell: A promising candidate for cementum/periodontal ligament regeneration and bio-root engineering. Stem Cells Dev. 19, 1405–1415. doi:10.1089/scd.2009.0277
Hercher D., Nguyen M. Q., Dworak H. (2022). Extracellular vesicles and their role in peripheral nerve regeneration. Exp. Neurol. 350, 113968. doi:10.1016/j.expneurol.2021.113968
Hristov M., Erl W., Linder S., Weber P. C. (2004). Apoptotic bodies from endothelial cells enhance the number and initiate the differentiation of human endothelial progenitor cells in vitro. Blood 104, 2761–2766. doi:10.1182/blood-2003-10-3614
Huang C. C., Narayanan R., Alapati S., Ravindran S. (2016). Exosomes as biomimetic tools for stem cell differentiation: Applications in dental pulp tissue regeneration. Biomaterials 111, 103–115. doi:10.1016/j.biomaterials.2016.09.029
Huang X., Qiu W., Pan Y., Li J., Chen Z., Zhang K., et al. (2021). Exosomes from LPS-stimulated hDPSCs activated the angiogenic potential of HUVECs in vitro. Stem Cells Int. 2021, 6685307. doi:10.1155/2021/6685307
Huang Y., Liu Q., Liu L., Huo F., Guo S., Tian W. (2022). Lipopolysaccharide-preconditioned dental follicle stem cells derived small extracellular vesicles treating periodontitis via reactive oxygen species/mitogen-activated protein kinase signaling-mediated antioxidant effect. Int. J. Nanomedicine 17, 799–819. doi:10.2147/IJN.S350869
Huynh N., VonMoss L., Smith D., Rahman I., Felemban M. F., Zuo J., et al. (2016). Characterization of regulatory extracellular vesicles from osteoclasts. J. Dent. Res. 95 (6), 673–679. doi:10.1177/0022034516633189
Ibarretxe G., Crende O., Aurrekoetxea M., García-Murga V., Etxaniz J., Unda F. (2012). Neural crest stem cells from dental tissues: A new hope for dental and neural regeneration. Stem Cells Int. 2012, 103503. doi:10.1155/2012/103503
Ivica A., Ghayor C., Zehnder M., Valdec S., Weber F. E. (2020). Pulp-derived exosomes in a fibrin-based regenerative root filling material. J. Clin. Med. 9 (2), E491. doi:10.3390/jcm9020491
Jeon O. H., Kim C., Laberge R. M., Demaria M., Rathod S., Vasserot A. P., et al. (2017). Local clearance of senescent cells attenuates the development of post-traumatic osteoarthritis and creates a pro-regenerative environment. Nat. Med. 23, 775–781. doi:10.1038/nm.4324
Kaukua N., Shahidi M. K., Konstantinidou C., Dyachuk V., Kaucka M., Furlan A., et al. (2014). Glial origin of mesenchymal stem cells in a tooth model system. Nature 513, 551–554. doi:10.1038/nature13536
Kim H., Yang G., Park J., Choi J., Kang E., Lee B. K. (2019). Therapeutic effect of mesenchymal stem cells derived from human umbilical cord in rabbit temporomandibular joint model of osteoarthritis. Sci. Rep. 9 (1), 13854. doi:10.1038/s41598-019-50435-2
Koritzinsky E. H., Street J. M., Star R. A., Yuen P. S. (2017). Quantification of exosomes. J. Cell. Physiol. 232 (7), 1587–1590. doi:10.1002/jcp.25387
Li J., Ju Y., Liu S., Fu Y., Zhao S. (2021). Exosomes derived from lipopolysaccharide-preconditioned human dental pulp stem cells regulate Schwann cell migration and differentiation. Connect. Tissue Res. 62 (3), 277–286. doi:10.1080/03008207.2019.1694010
Li M., Xing X., Huang H., Liang C., Gao X., Tang Q., et al. (2022). BMSC-derived ApoEVs promote craniofacial bone repair via ROS/JNK signaling. J. Dent. Res. 101, 714–723. doi:10.1177/00220345211068338
Li M., Liao L., Tian W. (2020). Extracellular vesicles derived from apoptotic cells: An essential link between death and regeneration. Front. Cell Dev. Biol. 8, 573511. doi:10.3389/fcell.2020.573511
Liu L., Guo S., Shi W., Liu Q., Huo F., Wu Y., et al. (2021a). Bone marrow mesenchymal stem cell-derived small extracellular vesicles promote periodontal regeneration. Tissue Eng. Part A 27 (13), 962–976. doi:10.1089/ten.TEA.2020.0141
Liu T., Hu W., Zou X., Xu J., He S., Chang L., et al. (2020). Human periodontal ligament stem cell-derived exosomes promote bone regeneration by altering MicroRNA profiles. Stem Cells Int. 2020, 8852307. doi:10.1155/2020/8852307
Liu W., Yuan Y., Liu D. (2021b). Extracellular vesicles from adipose-derived stem cells promote diabetic wound healing via the PI3K-AKT-mTOR-HIF-1α signaling pathway. Tissue Eng. Regen. Med. 18, 1035–1044. doi:10.1007/s13770-021-00383-8
Liu X., Li Q., Niu X., Hu B., Chen S., Song W., et al. (2017). Exosomes secreted from human-induced pluripotent stem cell-derived mesenchymal stem cells prevent osteonecrosis of the femoral head by promoting angiogenesis. Int. J. Biol. Sci. 13 (2), 232–244. doi:10.7150/ijbs.16951
Lo Sicco C., Reverberi D., Balbi C., Ulivi V., Principi E., Pascucci L., et al. (2017). Mesenchymal stem cell-derived extracellular vesicles as mediators of anti-inflammatory effects: Endorsement of macrophage polarization. Stem Cells Transl. Med. 6, 1018–1028. doi:10.1002/sctm.16-0363
Lui J. N., Lim W. Y., Ricucci D. (2020). An immunofluorescence study to analyze wound healing outcomes of regenerative endodontics in an immature premolar with chronic apical abscess. J. Endod. 46 (5), 627–640. doi:10.1016/j.joen.2020.01.015
Luo H., Huang F., Huang Z., Huang H., Liu C., Feng Y., et al. (2022). microRNA-93 packaged in extracellular vesicles from mesenchymal stem cells reduce neonatal hypoxic-ischemic brain injury. Brain Res. 2022, 148042. doi:10.1016/j.brainres.2022.148042
Luo P., Jiang C., Ji P., Wang M., Xu J. (2019). Exosomes of stem cells from human exfoliated deciduous teeth as an anti-inflammatory agent in temporomandibular joint chondrocytes via miR-100-5p/mTOR. Stem Cell Res. Ther. 10 (1), 216. doi:10.1186/s13287-019-1341-7
Luzuriaga J., Pineda J. R., Irastorza I., Uribe-Etxebarria V., García-Gallastegui P., Encinas J. M., et al. (2019). BDNF and NT3 reprogram human ectomesenchymal dental pulp stem cells to neurogenic and gliogenic neural crest progenitors cultured in serum-free medium. Cell. Physiol. biochem. 52, 1361–1380. doi:10.33594/000000096
Lv L., Sheng C., Zhou Y. (2020). Extracellular vesicles as a novel therapeutic tool for cell-free regenerative medicine in oral rehabilitation. J. Oral Rehabil. 47 (1), 29–54. doi:10.1111/joor.12885
Maia J., Caja S., Strano Moraes M. C., Couto N., Costa-Silva B. (2018). Exosome-based cell-cell communication in the tumor microenvironment. Front. Cell Dev. Biol. 6, 18. doi:10.3389/fcell.2018.00018
Mannelli G., Arcuri F., Agostini T., Innocenti M., Raffaini M., Spinelli G. (2018). Classification of tongue cancer resection and treatment algorithm. J. Surg. Oncol. 117 (5), 1092–1099. doi:10.1002/jso.24991
Mao Q., Nguyen P. D., Shanti R. M., Shi S., Shakoori P., Zhang Q., et al. (2019). Gingiva-derived mesenchymal stem cell-extracellular vesicles activate Schwann cell repair phenotype and promote nerve regeneration. Tissue Eng. Part A 25 (12), 887–900. doi:10.1089/ten.TEA.2018.0176
Mayo V., Sawatari Y., Huang C. Y., Garcia-Godoy F. (2014). Neural crest-derived dental stem cells--where we are and where we are going. J. Dent. 42 (9), 1043–1051. doi:10.1016/j.jdent.2014.04.007
Miura M., Gronthos S., Zhao M., Lu B., Fisher L. W., Robey P. G., et al. (2003). Shed: Stem cells from human exfoliated deciduous teeth. Proc. Natl. Acad. Sci. U. S. A. 100, 5807–5812. doi:10.1073/pnas.0937635100
Mohammed E., Khalil E., Sabry D. (2018). Effect of adipose-derived stem cells and their exo as adjunctive therapy to nonsurgical periodontal treatment: A histologic and histomorphometric study in rats. Biomolecules 8 (4), E167. doi:10.3390/biom8040167
Monsarrat P., Vergnes J. N., Nabet C., Sixou M., Snead M. L., Planat-Benard V., et al. (2014). Concise review: Mesenchymal stromal cells used for periodontal regeneration: A systematic review. Stem Cells Transl. Med. 3 (6), 768–774. doi:10.5966/sctm.2013-0183
Nakao Y., Fukuda T., Zhang Q., Sanui T., Shinjo T., Kou X., et al. (2021). Exosomes from TNF-α-treated human gingiva-derived MSCs enhance M2 macrophage polarization and inhibit periodontal bone loss. Acta Biomater. 122, 306–324. doi:10.1016/j.actbio.2020.12.046
Nooshabadi V. T., Khanmohamadi M., Valipour E., Mahdipour S., Salati A., Malekshahi Z. V., et al. (2020). Impact of exosome-loaded chitosan hydrogel in wound repair and layered dermal reconstitution in mice animal model. J. Biomed. Mat. Res. A 108 (11), 2138–2149. doi:10.1002/jbm.a.36959
Omi M., Hata M., Nakamura N., Miyabe M., Ozawa S., Nukada H., et al. (2017). Transplantation of dental pulp stem cells improves long-term diabetic polyneuropathy together with improvement of nerve morphometrical evaluation. Stem Cell Res. Ther. 8 (1), 279. doi:10.1186/s13287-017-0729-5
Pastuzyn E. D., Day C. E., Kearns R. B., Kyrke-Smith M., Taibi A. V., McCormick J., et al. (2018). The neuronal gene arc encodes a repurposed retrotransposon gag protein that mediates intercellular RNA transfer. Cell 172, 275–288. doi:10.1016/j.cell.2017.12.024
Pellettieri J., Fitzgerald P., Watanabe S., Mancuso J., Green D. R., Sánchez Alvarado A. (2010). Cell death and tissue remodeling in planarian regeneration. Dev. Biol. 338, 76–85. doi:10.1016/j.ydbio.2009.09.015
Qing L., Chen H., Tang J., Jia X. (2018). Exosomes and their MicroRNA cargo: New players in peripheral nerve regeneration. Neurorehabil. Neural Repair 32, 765–776. doi:10.1177/1545968318798955
Ren F., Zhang H., Qi C., Gao M. L., Wang H., Li X. Q. (2015). Blockade of transient receptor potential cation channel subfamily V member 1 promotes regeneration after sciatic nerve injury. Neural Regen. Res. 10, 1324–1331. doi:10.4103/1673-5374.162770
Santangelo L., Giurato G., Cicchini C., Montaldo C., Mancone C., Tarallo R., et al. (2016). The RNA-binding protein SYNCRIP is a component of the hepatocyte exosomal machinery controlling MicroRNA sorting. Cell Rep. 17, 799–808. doi:10.1016/j.celrep.2016.09.031
Seo B. M., Miura M., Gronthos S., Bartold P. M., Batouli S., Brahim J., et al. (2004). Investigation of multipotent postnatal stem cells from human periodontal ligament. Lancet (London, Engl. 364, 149–155. doi:10.1016/S0140-6736(04)16627-0
Shen Z., Kuang S., Zhang Y., Yang M., Qin W., Shi X., et al. (2020). Chitosan hydrogel incorporated with dental pulp stem cell-derived exosomes alleviates periodontitis in mice via a macrophage-dependent mechanism. Bioact. Mat. 5 (4), 1113–1126. doi:10.1016/j.bioactmat.2020.07.002
Shi Q., Huo N., Wang X., Yang S., Wang J., Zhang T. (2020). Exosomes from oral tissue stem cells: Biological effects and applications. Cell Biosci. 10, 108. doi:10.1186/s13578-020-00471-7
Shyh-Chang N., Ng H. H. (2017). The metabolic programming of stem cells. Genes Dev. 31 (4), 336–346. doi:10.1101/gad.293167.116
Song M., Lee J. H., Bae J., Bu Y., Kim E. C. (2017). Human dental pulp stem cells are more effective than human bone marrow-derived mesenchymal stem cells in cerebral ischemic injury. Cell Transpl. 26 (6), 1001–1016. doi:10.3727/096368916X694391
Su N., Hao Y., Wang F., Hou W., Chen H., Luo Y. (2021). Mesenchymal stromal exosome-functionalized scaffolds induce innate and adaptive immunomodulatory responses toward tissue repair. Sci. Adv. 7 (20), 7207. doi:10.1126/sciadv.abf7207
Sultan N., Amin L. E., Zaher A. R., Grawish M. E., Scheven B. A. (2020). Neurotrophic effects of dental pulp stem cells on trigeminal neuronal cells. Sci. Rep. 10 (1), 19694. doi:10.1038/s41598-020-76684-0
Swanson W. B., Gong T., Zhang Z., Eberle M., Niemann D., Dong R., et al. (2020b). Controlled release of odontogenic exosomes from a biodegradable vehicle mediates dentinogenesis as a novel biomimetic pulp capping therapy. J. Control. Release 324, 679–694. doi:10.1016/j.jconrel.2020.06.006
Swanson W. B., Zhang Z., Xiu K., Gong T., Eberle M., Wang Z., et al. (2020a). Scaffolds with controlled release of pro-mineralization exosomes to promote craniofacial bone healing without cell transplantation. Acta Biomater. 118, 215–232. doi:10.1016/j.actbio.2020.09.052
Szekeres-Bartho J., Šućurović S., Mulac-Jeričević B. (2018). The role of extracellular vesicles and PIBF in embryo-maternal immune-interactions. Front. Immunol. 9, 2890. doi:10.3389/fimmu.2018.02890
Théry C., Witwer K. W., Aikawa E., Alcaraz M. J., Anderson J. D., Andriantsitohaina R., et al. (2018). Minimal information for studies of extracellular vesicles 2018 (MISEV2018): A position statement of the international society for extracellular vesicles and update of the MISEV2014 guidelines. J. Extracell. Vesicles 7, 1535750. doi:10.1080/20013078.2018.1535750
Tutuianu R., Rosca A. M., Iacomi D. M., Simionescu M., Titorencu I. (2021). Human mesenchymal stromal cell-derived exosomes promote in vitro wound healing by modulating the biological properties of skin keratinocytes and fibroblasts and stimulating angiogenesis. Int. J. Mol. Sci. 22 (12), 6239. doi:10.3390/ijms22126239
Ullah I., Subbarao R. B., Kim E. J., Bharti D., Jang S. J., Park J. S., et al. (2016). In vitro comparative analysis of human dental stem cells from a single donor and its neuronal differentiation potential evaluated by electrophysiology. Life Sci. 154, 39–51. doi:10.1016/j.lfs.2016.04.026
Uribe-Etxebarria V., Luzuriaga J., García-Gallastegui P., Agliano A., Unda F., Ibarretxe G. (2017). Notch/Wnt cross-signalling regulates stemness of dental pulp stem cells through expression of neural crest and core pluripotency factors. Eur. Cell. Mat. 34, 249–270. doi:10.22203/eCM.v034a16
Van Niel G. D., Angelo G., Raposo G. (2018). Shedding light on the cell biology of extracellular vesicles. Nat. Rev. Mol. Cell Biol. 19 (4), 213–228. doi:10.1038/nrm.2017.125
Villarroya-Beltri C., Baixauli F., Gutiérrez-Vázquez C., Sánchez-Madrid F., Mittelbrunn M. (2014). Sorting it out: Regulation of exosome loading. Semin. Cancer Biol. 28, 3–13. doi:10.1016/j.semcancer.2014.04.009
Villarroya-Beltri C., Gutiérrez-Vázquez C., Sánchez-Cabo F., Pérez-Hernández D., Vázquez J., Martin-Cofreces N., et al. (2013). Sumoylated hnRNPA2B1 controls the sorting of miRNAs into exosomes through binding to specific motifs. Nat. Commun. 4, 2980. doi:10.1038/ncomms3980
Wang X. D., Zhang J. N., Gan Y. H., Zhou Y. H. (2015). Current understanding of pathogenesis and treatment of TMJ osteoarthritis. J. Dent. Res. 94 (5), 666–673. doi:10.1177/0022034515574770
Watanabe J., Sakai K., Urata Y., Toyama N., Nakamichi E., Hibi H. (2020). Extracellular vesicles of stem cells to prevent BRONJ. J. Dent. Res. 99 (5), 552–560. doi:10.1177/0022034520906793
Wen S., Dooner M., Cheng Y., Papa E., Del TattoM. , PereiraM. , et al. (2016). Mesenchymal stromal cell-derived extracellular vesicles rescue radiation damage to murine marrow hematopoietic cells. Leukemia 30 (11), 2221–2231. doi:10.1038/leu.2016.107
Wu J., Chen L., Wang R., Song Z., Shen Z., Zhao Y., et al. (2019). Exosomes secreted by stem cells from human exfoliated deciduous teeth promote alveolar bone defect repair through the regulation of angiogenesis and osteogenesis. ACS Biomater. Sci. Eng. 5 (7), 3561–3571. doi:10.1021/acsbiomaterials.9b00607
Xia B., Gao J., Li S., Huang L., Zhu L., Ma T., et al. (2020). Mechanical stimulation of Schwann cells promote peripheral nerve regeneration via extracellular vesicle-mediated transfer of microRNA 23b-3p. Theranostics 10 (20), 8974–8995. doi:10.7150/thno.44912
Xian X., Gong Q., Li C., Guo B., Jiang H. (2018). Exosomes with highly angiogenic potential for possible use in pulp regeneration. J. Endod. 44 (5), 751–758. doi:10.1016/j.joen.2017.12.024
Yáñez-Mó M., Siljander P. R., Andreu Z., Zavec A. B., Borràs F. E., Buzas E. I., et al. (2015). Biological properties of extracellular vesicles and their physiological functions. J. Extracell. Vesicles 4, 27066. doi:10.3402/jev.v4.27066
Yang G., Chang C. C., Yang Y., Yuan L., Xu L., Ho C. T., et al. (2018). Resveratrol alleviates rheumatoid arthritis via reducing ROS and inflammation, inhibiting MAPK signaling pathways, and suppressing angiogenesis. J. Agric. Food Chem. 66, 12953–12960. doi:10.1021/acs.jafc.8b05047
Yin B., Ni J., Witherel C. E., Yang M., Burdick J. A., Wen C., et al. (2022). Harnessing tissue-derived extracellular vesicles for osteoarthritis theranostics. Theranostics 12, 207–231. doi:10.7150/thno.62708
You Y., Borgmann K., Edara V. V., Stacy S., Ghorpade A., Ikezu T. (2020). Activated human astrocyte-derived extracellular vesicles modulate neuronal uptake, differentiation and firing. J. Extracell. Vesicles 9 (1), 1706801. doi:10.1080/20013078.2019.1706801
Yu B., Zhang X., Li X. (2014). Exosomes derived from mesenchymal stem cells. Int. J. Mol. Sci. 15 (3), 4142–4157. doi:10.3390/ijms15034142
Zeng Q. L., Liu D. W. (2021). Mesenchymal stem cell-derived exosomes: An emerging therapeutic strategy for normal and chronic wound healing. World J. Clin. Cases 9 (22), 6218–6233. doi:10.12998/wjcc.v9.i22.6218
Zhai Y., Wei R., Liu J., Wang H., Cai W., Zhao M., et al. (2017). Drug-induced premature senescence model in human dental follicle stem cells. Oncotarget 8 (5), 7276–7293. doi:10.18632/oncotarget.14085
Zhang S., Chu W. C., Lai R. C., Lim S. K., Hui J. H., Toh W. S. (2016). Exosomes derived from human embryonic mesenchymal stem cells promote osteochondral regeneration. Osteoarthr. Cartil. 24 (12), 2135–2140. doi:10.1016/j.joca.2016.06.022
Zhang S., Chuah S. J., Lai R. C., Hui J., Lim S. K., Toh W. S. (2018). MSC exosomes mediate cartilage repair by enhancing proliferation, attenuating apoptosis and modulating immune reactivity. Biomaterials 156, 16–27. doi:10.1016/j.biomaterials.2017.11.028
Zhang Y., Shi S., Xu Q., Zhang Q., Shanti R. M., Le A. D. (2019). SIS-ECM laden with GMSC-derived exosomes promote taste bud regeneration. J. Dent. Res. 98 (2), 225–233. doi:10.1177/0022034518804531
Zhang Y., Yu M., Dai M., Chen C., Tang Q., Jing W., et al. (2017). miR-450a-5p within rat adipose tissue exosome-like vesicles promotes adipogenic differentiation by targeting WISP2. J. Cell Sci. 130 (6), 1158–1168. doi:10.1242/jcs.197764
Zhang Y., Pan Y., Liu Y., Li X., Tang L., Duan M., et al. (2021). Exosomes derived from human umbilical cord blood mesenchymal stem cells stimulate regenerative wound healing via transforming growth factor-β receptor inhibition. Stem Cell Res. Ther. 12, 434. doi:10.1186/s13287-021-02517-0
Zhang Z., Shuai Y., Zhou F., Yin J., Hu J., Guo S., et al. (2020). PDLSCs regulate angiogenesis of periodontal ligaments via VEGF transferred by exosomes in periodontitis. Int. J. Med. Sci. 17 (5), 558–567. doi:10.7150/ijms.40918
Zhao Y., Xie L. (2021). An update on mesenchymal stem cell-centered therapies in temporomandibular joint osteoarthritis. Stem Cells Int. 2021, 6619527. doi:10.1155/2021/6619527
Zhao Y., Huang Y., Liu H., Tan K., Wang R., Jia L., et al. (2022). Macrophages with different polarization phenotypes influence cementoblast mineralization through exosomes. Stem Cells Int. 2022, 4185972. doi:10.1155/2022/4185972
Zhao Y., Wang L., Jin Y., Shi S. (2012). Fas ligand regulates the immunomodulatory properties of dental pulp stem cells. J. Dent. Res. 91, 948–954. doi:10.1177/0022034512458690
Zheng Y., Li J. X., Chen C. J., Lin Z. Y., Liu J. X., Lin F. J. (2020). Extracellular vesicle-derived circ_SLC19A1 promotes prostate cancer cell growth and invasion through the miR-497/septin 2 pathway. Cell Biol. Int. 44, 1037–1045. doi:10.1002/cbin.11303
Zhou H., Shen X., Yan C., Xiong W., Ma Z., Tan Z., et al. (2022). Extracellular vesicles derived from human umbilical cord mesenchymal stem cells alleviate osteoarthritis of the knee in mice model by interacting with METTL3 to reduce m6A of NLRP3 in macrophage. Stem Cell Res. Ther. 13, 322. doi:10.1186/s13287-022-03005-9
Zhou X., Brown B. A., Siegel A. P., El Masry M. S., Zeng X., Song W., et al. (2020). Exosome-mediated crosstalk between keratinocytes and macrophages in cutaneous wound healing. ACS Nano 14 (10), 12732–12748. doi:10.1021/acsnano.0c03064
Zhou X., Chu X., Yuan H., Qiu J., Zhao C., Xin D., et al. (2019). Mesenchymal stem cell derived EVs mediate neuroprotection after spinal cord injury in rats via the microRNA-21-5p/FasL gene axis. Biomed. Pharmacother. = Biomedecine Pharmacother. 115, 108818. doi:10.1016/j.biopha.2019.108818
Zhu L. L., Huang X., Yu W., Chen H., Chen Y., Dai Y. T. (2018). Transplantation of adipose tissue-derived stem cell-derived exosomes ameliorates erectile function in diabetic rats. Andrologia 50 (2), 12871. doi:10.1111/and.12871
Keywords: extracellular vesicles, regenerative medicine, dental and maxillofacial tissue repair, therapeutic effects, clinical application
Citation: Liu Z, Wang S, Huo N, Yang S, Shi Q and Xu J (2022) Extracellular vesicles: A potential future strategy for dental and maxillofacial tissue repair and regeneration. Front. Physiol. 13:1012241. doi: 10.3389/fphys.2022.1012241
Received: 05 August 2022; Accepted: 09 November 2022;
Published: 21 November 2022.
Edited by:
Gaskon Ibarretxe, University of the Basque Country, SpainReviewed by:
Rosanna Di Tinco, University of Modena and Reggio Emilia, ItalyKevin Tompkins, Chulalongkorn University, Thailand
Copyright © 2022 Liu, Wang, Huo, Yang, Shi and Xu. This is an open-access article distributed under the terms of the Creative Commons Attribution License (CC BY). The use, distribution or reproduction in other forums is permitted, provided the original author(s) and the copyright owner(s) are credited and that the original publication in this journal is cited, in accordance with accepted academic practice. No use, distribution or reproduction is permitted which does not comply with these terms.
*Correspondence: Juan Xu, bmV3eGpAaG90bWFpbC5jb20=; Quan Shi, c2hpcXVhbjMzMzNAc2luYS5jbg==
†These authors have contributed equally to this work