- Department of Cell Biology and Imaging, Institute of Zoology and Biomedical Research, Faculty of Biology, Jagiellonian University, Krakow, Poland
Light is one of most important factors synchronizing organisms to day/night cycles in the environment. In Drosophila it is received through compound eyes, Hofbauer-Buchner eyelet, ocelli, using phospholipase C-dependent phototransduction and by deep brain photoreceptors, like Cryptochrome. Even a single light pulse during early life induces larval-time memory, which synchronizes the circadian clock and maintains daily rhythms in adult flies. In this study we investigated several processes in adult flies after maintaining their embryos, larvae and pupae in constant darkness (DD) until eclosion. We found that the lack of external light during development affects sleep time, by reduction of night sleep, and in effect shift to the daytime. However, disruption of internal CRY- dependent photoreception annuls this effect. We also observed changes in the expression of genes encoding neurotransmitters and their receptors between flies kept in different light regime. In addition, the lack of light during development results in decreasing size of mushroom bodies, involved in sleep regulation. Taking together, our results show that presence of light during early life plays a key role in brain development and affects adult behavior.
Introduction
Light plays a key role in the synchronization of animal behavior to cyclical changes in environmental conditions, it acts as an arousal signal for diurnal animals and promotes sleep in nocturnal ones (Redlin, 2001). Drosophila is a very useful model for sleep/activity research since it exhibits a clear daily activity pattern. Under LD12:12 (12 h of light and 12 h of darkness) conditions it shows two peaks of activity, morning and evening, while in the middle of the day and night it has resting time called siesta and sleep, respectively. This rhythmic behavior is regulated by brain sleep centers, glial cells, as well as by different clusters of clock cells (Grima et al., 2004; Stoleru et al., 2004; Picot et al., 2007; Cusumano et al., 2009; Zhang et al., 2009; Yao and Shafer, 2014; Chatterjee et al., 2018; Guo et al., 2018; Schlichting et al., 2019). Sleep in flies is defined as at least 5 min of total inactivity with elevated sensory threshold and specific body posture (Shaw, 2000). Daytime (siesta) and nighttime sleep, which are the deepest around noon, and at midnight, respectively constitute total sleep. Siesta is usually less deep and has shorter single sleep episodes as well as lower arousal threshold comparing to nighttime sleep (Hendricks et al., 2000; Huber et al., 2004).
Sleep is regulated by several neurotransmitters: promoting sleep as serotonin and gamma-aminobutyric acid (GABA) (Yuan et al., 2005, 2006; Chung et al., 2009; Haynes et al., 2015; Qian et al., 2017) and wake-promoting as dopamine, octopamine, and histamine (Friggi-Grelin et al., 2003; Hamasaka and Nässel, 2006; Pantazis et al., 2008; Mao and Davis, 2009; Crocker et al., 2010; Liu et al., 2012; Ueno et al., 2012; Oh et al., 2013; Sitaraman et al., 2015; Alejevski et al., 2019) and those playing a dual role depending on target cells (acetylcholine, glutamate) (Buchner et al., 1986; Yasuyama and Salvaterra, 1999; Sinakevitch-Pean et al., 2001; McCarthy et al., 2011; Raghu and Borst, 2011; Takemura et al., 2011; Liu and Wilson, 2013; Mauss et al., 2014, 2015; Tomita et al., 2015; Robinson et al., 2016). In addition, there are neurotransmitters regulating clock network activity: Pigment-dispersing factor (PDF) and short Neuropeptide F (sNFP), which play a role in the integration of clock and sleep (Shang et al., 2013; Kunst et al., 2014).
Dopamine is a monoamine synthesized from tyrosine or phenylalanine mainly in a set of specific neurons in the presence of tyrosine hydroxylase encoded in Drosophila by the ple gene. Once produced and released into synaptic cleft it can bind to specific receptors on postsynaptic cells in the following structures involved in sleep regulation: Dop1R1 in neurons of mushroom bodies, ellipsoid body and fan-shaped body, Dop1R2 in neurons of mushroom bodies and DD2R in three pairs of dorsal neurons in the ventral nerve cord and sleep promoting protocerebral bridge interneurons (Friggi-Grelin et al., 2003; Mao and Davis, 2009; Liu et al., 2012; Ueno et al., 2012; Sitaraman et al., 2015; Tomita et al., 2021).
The another neurotransmitter involved in light transmission and sleep regulation is histamine. It is released mostly by the retina photoreceptors and synthesized by histidine decarboxylase (Hdc). One of its receptor HisCl1 is expressed in photoreceptors and clock neurons, and the other one—Ort receptor—in the lamina and medulla interneurons (Hamasaka and Nässel, 2006; Pantazis et al., 2008; Oh et al., 2013; Alejevski et al., 2019).
Octopamine is synthesized by anterior paired lateral neurons (APL) using tyramine beta hydroxylase (Tbh) and its signal is transmitted to mushroom bodies through Oamb receptor (Crocker et al., 2010).
All these neurotransmitters control activity of specific neurons located in structures called sleep centers. The most important ones are mushroom bodies (MB) and central complex in the central and dorsal brain (Joiner et al., 2006; Pitman et al., 2006; Donlea et al., 2011; Liu et al., 2012; Guo et al., 2016). The mushroom body is formed by Kenyon cells (Kc), and can be divided into morphologically distinct parts: calyx, which contains synapses between Kc and projection neurons, and five output lobes (α, α′, β, β’, γ), composed of synapses between Kc, mushroom body output neurons and dopaminergic neurons (Aso et al., 2014). Output signals from multiple sensory pathways are received by calyx and then transferred to the lobes. MB integrate and process also visual signals coming from medulla, lobula and posterior lateral protocerebrum (Morante and Desplan, 2008; Vogt et al., 2016; Li et al., 2020).
Light input is received by the visual system: retinal photoreceptors, Hofbauer-Buchner eyelet and ocelli through the rhodopsin-dependent phototransduction pathway (Busto et al., 1999; Hassan et al., 2000). All photoreceptors use histamine to communicate with downstream neurons (Hamasaka and Nässel, 2006), but some of them use also acetylcholine as a neurotransmitter, for example eyelets which contact directly with clock cells (Pollack and Hofbauer, 1991; Yasuyama and Meinertzhagen, 1999; Helfrich-Förster et al., 2002; Malpel et al., 2002; Damulewicz et al., 2020). In addition, light is detected by deep brain photoreceptors, Cryptochrome (CRY) and Quasimodo (QSM), which are mostly involved in clock synchronization (VanVickle-Chavez and Van Gelder, 2007; Chen et al., 2011). Moreover, rhodopsin 7, which is involved in non-visual light signaling, regulates morning activity and time of siesta (Kistenpfennig et al., 2017).
In larvae light activates CRY located in clock neurons (four LNvs and DN1s) and photoreceptors of the Bolwig organ (BO), which contains rhodopsin 5 or rhodopsin 6 (Malpel et al., 2002; Mazzoni et al., 2005) and communicates directly with the larval pacemaker to synchronize this oscillator to changes in environmental light conditions (Keene et al., 2011; Klarsfeld et al., 2011). Although larvae do not show circadian behavior, their clock is entrained by light which can affect the clock in adult flies. It has been shown that a single light pulse during embryonal or postembryonic development can synchronize activity of adult flies kept in constant darkness (Sehgal et al., 1992; Zhao et al., 2019).
The role of light in the sleep regulation is already well described, however, little is known about the link between light exposure during development and sleep amount in adult life. In this study, using Drosophila as a model, we showed that lack of light in early life affects behavior of adult flies.
Material and methods
Flies
The following strains of Drosophila melanogaster were used: wild type CantonS, norpA7 mutant (BDSC, no. 5685), cry01 mutant (Dolezelova et al., 2007), OK107-Gal4 (kindly donated by P. Menegazzi, Wurzburg University), UAS-mCD8::GFP (BDSC, stock no. 5137).
Flies were maintained on a standard cornmeal medium at constant temperature 25°C. The control group was kept under LD12:12 (12 h of light and 12 h of darkness) regime during development and adulthood (group described as LD/LD), while experimental flies were kept in constant darkness (DD) during development (embryonal, larval and pupal stage) (group described here as DD/DD). Because sleep assay was measured on the second day of LD12:12 conditions, we added the additional group called DD/LD, in which flies exposed to DD during development were transferred for 2 days to LD12:12.
Behavioral assays
Locomotor activity was recorded using Drosophila Activity Monitoring System (DAMS, Trikinetics, Waltham) for 5 days in LD12:12 and 6 days in DD. Activity was counted every 1 min and analyzed in Excel using “Befly!” software (Department of Genetics, Leicester University). Lomb—Scargle normalized periodogram was used to determine rhythmic flies; individuals with period below 10 (confidence level 0.05) were regarded as arrhythmic. Flies, which did not survive until the end of experiments were removed from analyses. Every experiment was repeated three times, at least 60 flies in total were used.
Sleep analysis was performed on the second day of LD12:12, and sleep was recorded as at least 5 min of a fly immobility.
Immunohistochemistry
Males from the strain OK107 > GFP were collected at ZT1 (1 hour after lights-on in LD 12:12) or CT1 (in DD), their heads were fixed in 4% paraformaldehyde and brains were isolated. GFP fluorescence of this strain is strong enough to skip additional staining steps.
For TH staining wild type flies were used. Fixed brains were washed in PBS and 0.2% PBST (PBS with Triton X), then unspecific antigens were blocked with Normal Goat Serum (NGS) for 1 h. Samples were incubated with mouse anti-TH antibodies (1:1000, Immunostar) for 3 days at 4°C. Then, brains were washed three times in 0.2% PBST and incubated for 2 h with goat anti-mouse antibodies conjugated with Cy3 (1:500, ThermoFisher). After washing in 0.2% PBST, brains were mounted in Vectashield medium (Vector) and examined with a Zeiss Meta 510 Laser Scanning Microscope.
The size of the brain and GFP-labelled mushroom bodies lobes was measured using ImageJ software (Area measurements). To avoid changes in body size, dependent on overcrowding, the same number of parental flies was used for every group.
The TH immunofluorescence intensity was measured for specific dopaminergic cells: PPL1, PAM and PPM3 because these cluster are known to innervate sleep centers. Area of every single cell was selected manually and mean grey value was measured using ImageJ software.
Experiments were repeated 3 times and each repetition was composed of 10 brains per group.
Gene expression analysis by qPCR
For gene expression analysis we divided the experimental flies into two groups—first was continuously kept in constant darkness (DD/DD), the second one was moved to LD12:12 for 2 days (DD/LD) to provide light input and synchronize flies. All groups were collected at the same time—ZT1 (1 hour after lights-on for control and flies transferred to LD12:12) and CT1 (for flies kept in DD). At least 20 heads were cut off and the total RNA was isolated using a TriReagent (Invitrogen). 1 μg of the total RNA was used to prepare cDNA with the High Capacity cDNA Reverse Transcription Kit (Thermo Fisher Scientific) and random primers. cDNA (diluted 1:10) was used for SybrGreen qPCR (KapaBiosystems) analysis. The specific primers (the specificity was controlled with Primer BLAST and gel electrophoresis) used for the reaction are listed in Table 1. Expression level was calculated by relative standard curve. The reference gene used was rpl32. Experiments were repeated 5 times.
Statistical analysis
GraphPad Prism software was used for statistics and making graphs. Outliers were removed using Grubbs’ test (GraphPad online software). Shapiro-Wilk’s test was used to check normality in distribution. Data were analyzed using one-way ANOVA with post-hoc Tukey’s test or t-test to detect statistically significant differences between groups.
Results
Light exposure during development is not necessary to maintain rhythmicity in behavior, but it affects activity/sleep ratio. It has been shown that larvae kept in DD can be easily synchronized to light:dark regime, which suggests that their clock develops normally. Our data confirmed these results because flies which were kept in DD during development and transferred to LD12:12 after eclosion were perfectly synchronized and after moving back to DD they were able to maintain 24 h rhythmicity. We focused our research on the other aspect of behavior ‒ activity and sleep amount and pattern. We observed that flies from the experimental group (DD/LD) were more active than the control (LD/LD) (p = 0.0052), activity index was increased (p = 0.0025) and their sleep amount during the night, measured in minutes, was decreased (p = 0.0202), while siesta was not changed (p = 0.0723) (Figure 1). Moreover, control flies had longer sleep during the night than during the day, while experimental ones had similar sleep level all over the day.

FIGURE 1. Light during embryonal/larval stage regulates sleep. Wild-type flies (Canton S, CS) were grown starting from embryo in LD12:12 or DD regime. After eclosion all flies were kept in LD12:12 and 2-days old males were used for DAMS locomotor analysis. Sleep and activity level were measured during second day of experiment. (A) Sleep profile of flies (measured as number of 5-min beans per hour) maintained without light during early life (DD/LD) shows differences with control (LD/LD) during first 8 h after light-on and during whole night. (B) Sleep time measured as number of minutes per 12 h showed that DD/LD group has decreased level of sleep during the night. Control flies show higher level of sleep during the night than during siesta, while experimental insects have similar level of sleep throughout the day. (C) Total activity measured as number of minutes per 24 h was increased in flies which spend early life in darkness compare with control. (D) Activity index measured as ratio of total activity per day and number of minutes awaked. Mean ± SEM. Statistically significant differences shown as asterisks **p ≤ 0.01, ***p ≤ 0.001.
Neurotransmission is affected by the lack of light during development
Sleep is regulated by several neurotransmitters released by specific neurons and recognized by receptors on target cells. Because we observed increased activity and decreased sleep level in the experimental flies, we expected to see changes in the expression of wake-promoting factors. The most important ones are dopamine, histamine and octopamine, as well as PDF and sNPF which affect the clock network. To investigate how light during development regulates these pathways we decided to check changes in the mRNA level of both, neurotransmitters and their receptors in heads. We compared mRNA level of selected genes in adult flies from three different groups: flies which were maintained during the development and as adults in DD (group DD/DD), flies developing in DD and transferred to LD12:12 as adults, which is similar to the experiment for sleep analysis (group DD/LD) and control flies developing and kept in LD12:12 after eclosion (group LD/LD).
First, we investigated effect of light during development on dopamine signaling. Our results showed that dopamine synthesis was decreased in flies developing in constant darkness (Figure 2A, LD/LD vs. DD/LD p = 0.00159, LD/LD vs. DD/DD p = 0.0286), which suggests that light during development is necessary to maintain high level of dopamine signaling. This result was not expected, as dopamine is wake-promoting factor, and knowing that experimental flies are more active we predicted to observe higher dopamine synthesis. However, the opposite situation was observed for Dop1R1, which was expressed at higher level in the groups DD/LD and DD/DD (Figure 2B, LD/LD vs. DD/LD p = 0.1905, LD/LD vs. DD/DD p = 0.0159). The level of Dop1R2 mRNA was increased in the group DD/DD, but it was decreased after moving flies to LD12:12 (Figure 2C, LD/LD vs. DD/LD p > 0.9999, LD/LD vs. DD/DD p = 0.0159). Finally, DD2R showed the pattern of expression similar to Dop1R2, but the observed changes were not statistically significant (Figure 2D, LD/LD vs. DD/LD p = 0.0.6857, LD/LD vs. DD/DD p = 0.0635). The obtained results suggest more complex, cell-type specific regulation of dopamine signaling.

FIGURE 2. Darkness affects dopamine pathway. qPCR analysis of expression level of genes connected with dopamine signaling for flies maintained in normal conditions LD12:12 (LD/LD), maintained in DD (DD/DD) and developed in darkness and after eclosion transferred to LD12:12 (DD/LD). Expression checked for: (A) tyrosine hydroxylase (ple), (B) dopamine receptor Dop1R1, (C) Dop1R2 and (D) DD2R. N = 5. Mean ± SEM.
The another neurotransmitter involved in light transmission and sleep regulation is histamine. The expression pattern of Hdc (LD/LD vs. DD/LD p = 0.0079, LD/LD vs. DD/DD p = 0.0079), HisCl1 (LD/LD vs. DD/LD p = 0.0159, LD/LD vs. DD/DD p = 0.0286) and ort (LD/LD vs. DD/LD p = 0.01159, LD/LD vs. DD/DD p = 0.0571) in our experiment was similar, with decreased level in flies kept in DD during development (Figures 3A–C).
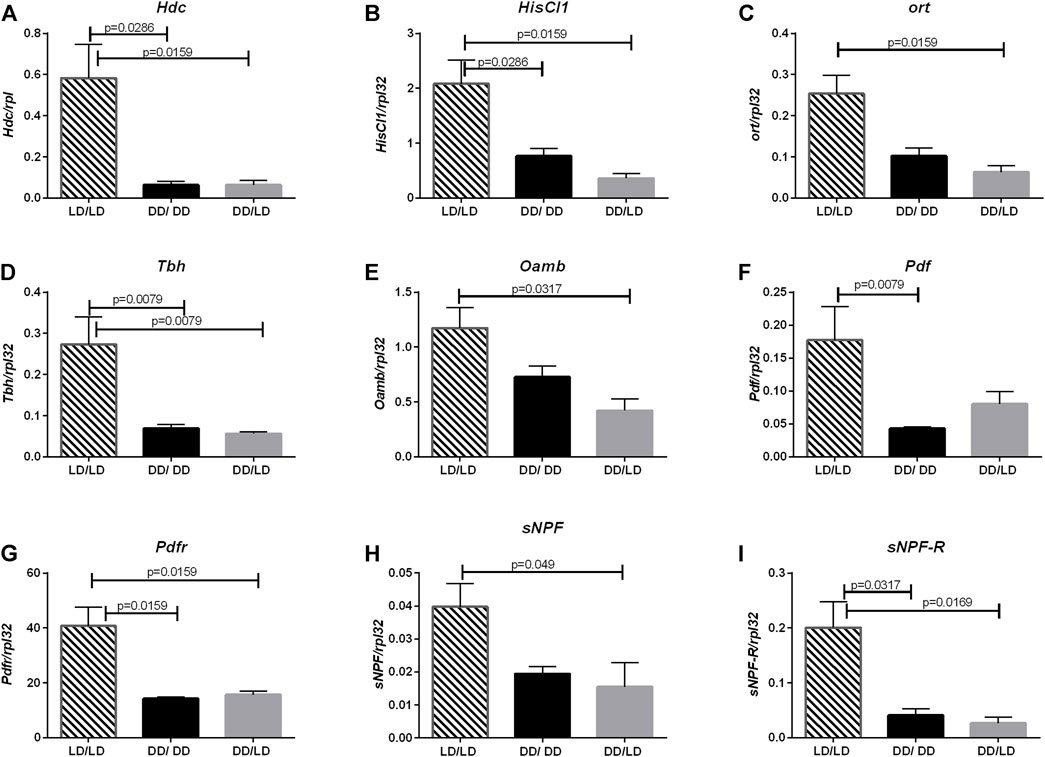
FIGURE 3. Lack of light changes neurotransmitters signaling level. qPCR analysis of expression level of genes connected with histamine, octopamine, PDF and sNPF signaling for flies maintained in normal conditions LD12:12 (LD/LD), maintained in DD and developed in darkness (DD/DD) and after eclosion transferred to LD12:12 (DD/LD). Expression checked for: (A) histidine decarboxylase (Hdc), (B) receptor HisCl1, (C) receptor Ort. (D) Tbh and (E) receptor Oamb, (F) Pdf, (G) Pdfr, (H) sNPF and (I) sNPF-R. N = 5. Mean ± SEM.
The next pathway affected by light conditions during development was octopamine. Light during the development decreased the expression level of both, Tbh (LD/LD vs. DD/LD p = 0.0079, LD/LD vs. DD/DD p = 0.0079) and Oamb (LD/LD vs. DD/LD p = 0.0317, LD/LD vs. DD/DD p = 0.1143), however, flies developing in DD and then transferred to LD had the lowest amount of transcript (Figures 3D,E.).
Finally, we checked the expression level of two neurotransmitter genes known to be involved directly in the circadian control of sleep: sNPF and PDF and their receptors, sNPF-R and PDFR. All of them had the similar pattern—in flies developing in DD their expression level was decreased, and after transferring adults to LD, the level of mRNA did not change significantly (Figures 3F–I, for Pdf LD/LD vs. DD/LD p = 0.0952, LD/LD vs. DD/DD p = 0.0079, for Pdfr LD/LD vs. DD/LD p = 0.0159, LD/LD vs. DD/DD p = 0.0159, for sNPF LD/LD vs. DD/LD p = 0.049, LD/LD vs. DD/DD p = 0.0635, for sNPF-R LD/LD vs. DD/LD p = 0.0169, LD/LD vs. DD/DD p = 0.0317).
Photoreception affects synthesis of neurotransmitters
Flies receive light signal through different pathways. The most important is the retinal phototransduction pathway, which requires phospholipase C (PLC), encoded by the gene norpA. NorpA mutants are almost blind, however, they still have partial light sensitivity through deep brain photoreceptors. Because we did not observe significat changes in gene expression between wild type flies kept in DD/DD and DD/LD conditions, and to avoid impact of light input received by additional photoreceptors, we perform this experiment for LD/LD and DD/DD groups only. Flies with norpA mutation developing in LD12:12 conditions, showed normal activity profile and they were able to maintain rhythmicity in DD. However, similarly to CS, norpA mutants, developing in DD, were more active than control in LD12:12 (p = 0.0003), but activity index was not changed (p = 0.8724). Their pattern of sleep was changed and the total amount of sleep was decreased during the day (p = 0.0001) and night (p = 0.0039) (Figure 4).

FIGURE 4. NorpA mutants show behavioral changes when developed without light. (A) Sleep pattern shows changes of sleep between experimental (DD/LD) and control group (LD/LD). (B) Sleep level is decreased both, during the day and night. (C) Total activity is significantly increased in flies which grown up in darkness. (D) Activity index measured as ratio of total activity per day and number of minutes awaked. Mean ± SEM. Statistically significant differences marked as asterisks: **p ≤ 0.01, ***p ≤ 0.001.
Having in mind that photoreception through the retina photoreceptors can affect neurotransmission level in the brain we checked gene expression of the previously selected genes in head homogenates from norpA mutants.
In dopamine pathway we found changes only in Dop1R1 expression between norpA mutants developing in LD12:12 and DD (p = 0.0317), while tyrosine hydroxylase (p = 0.6905), Dop1R2 (p = 0.0952) and DD2R (p = 0.3095) encoding genes were expressed at the similar level in both groups (Figure 5). This result suggests that light during embryonic and postembryonic development affects Dop1R2 and ple expression in PLC-dependent manner.

FIGURE 5. Light during early life affects only one of dopamine receptors in norpA mutant flies. qPCR analysis of expression level of genes connected with dopamine signaling for flies maintained in normal conditions LD12:12 (LD/LD), maintained and developed in darkness (DD/DD). Expression checked for: (A) tyrosine hydroxylase (ple), (B) dopamine receptor Dop1R1, (C) Dop1R2 and (D) DD2R. N = 5. Mean ± SEM.
Histamine synthesis (Hdc gene expression) was decreased in norpA mutants growing without light (Figure 6A, p = 0.0079), but mRNA levels of HisCl1 (Figure 6B, p = 0.8413) and ort genes of histamine receptors (Figure 6C, p = 0.4206) were unchanged. However, other neurotransmission pathways checked previously (octopamine, PDF and sNPF) seem do not to be PLC-dependent, as Tbh (Figure 6D, p > 0.9999), Oamb (Figure 6E, p = 0.2222.), Pdf (Figure 6F, p = 0.8413), Pdfr (Figure 6G, p = 0.1508), sNPF (Figure 6H, p = 0.0556) and sNPF-R (Figure 6I, p > 0.9999) mRNAs had similar level in LD/LD and DD/DD groups in norpA mutants.
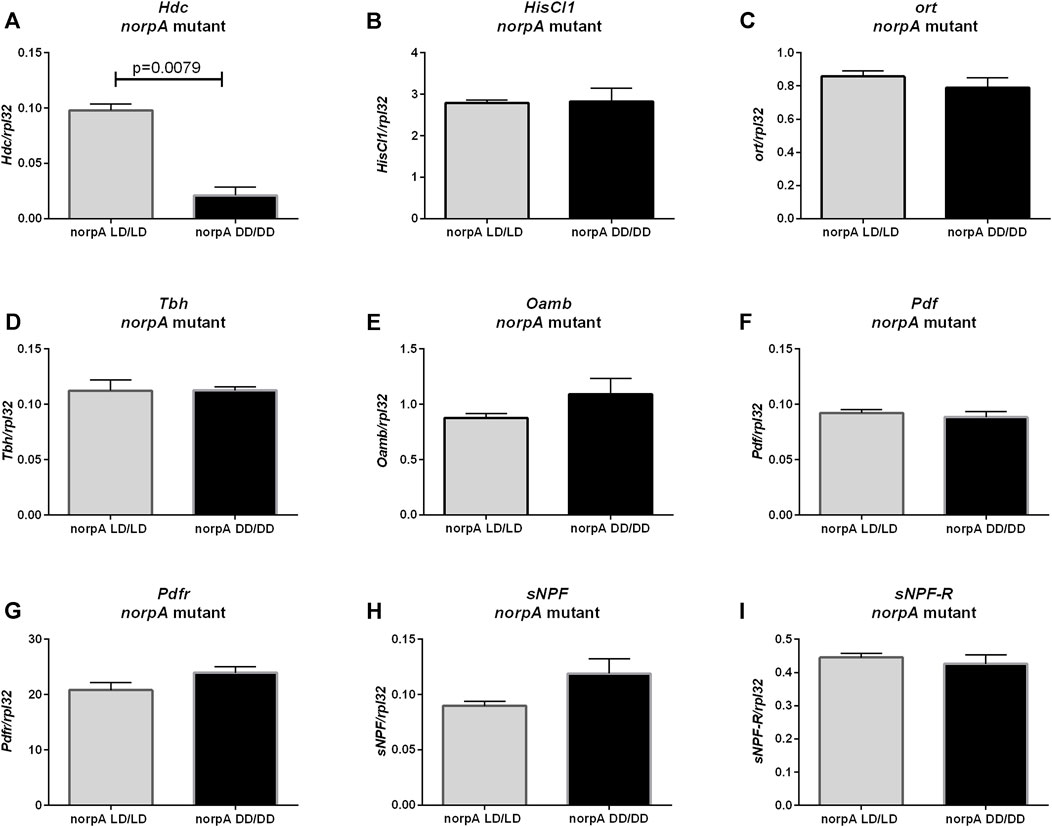
FIGURE 6. Effect of light on neurotransmitters pathway in norpA mutants. mRNA level was changed only for (A) Hdc gene. Histamine receptors: (B) HisCl1 and (C) ort were expressed on the same level. Octopamine signaling seems to be not affected: (D) Tbh, (E) Oamb. Clock signaling was not changed: (F) Pdf, (G) Pdfr, (H) sNPF, (I) sNPF-R. N = 5. Mean ± SEM.
To examine effect of light detected by deep brain photoreceptors, which can affect neurons directly, we used cry01 mutants. Surprisingly, we did observe neither differences in activity (p = 0.9137), activity index (p = 0.5335), sleep pattern nor its level (for day p = 0.874, for night p = 0.334) between flies reared in LD and DD (Figure 7). This result indicates that CRY is the most important light photoreceptor during development.

FIGURE 7. Cryptochrome is a key factor in larval light absorbance affecting behavior in adults. cry01 mutants kept in darkness during development did not show changes in (A) sleep pattern, (B) sleep level nor (C) activity level. (D) Activity index measured as ratio of total activity per day and number of minutes awaked. Mean ± SEM.
Moreover, we did not observe changes in ple (Figure 8A, p = 0.4), Dop1R1 (Figure 8B, p > 0.9999) nor DD2R expression (Figure 8D, p = 0.0571), but Dop1R2 mRNA level was increased in flies reared in DD, similarly to wild-type flies (Figure 8C, p = 0.0266). Histamine (Hdc, Figure 9A, p = 0.309, HisCl1, Figure 9B, p = 0.4, Ort, Figure 9C, p = 0.2857), octopamine (Tbh, Figure 9D, p = 0.8413, Oamb, Figure 9E, p = 0.1143), PDF (Pdf, Figure 9F, p = 0.3095, Pdfr, Figure 9G, p = 0.5476) and sNPF (sNPF, Figure 9H, p = 0.0571, sNPF-R, Figure 9I, p = 0.5476) signaling components were also not changed.

FIGURE 8. Light during early life affects only one of dopamine receptors in cry01 mutant flies. qPCR analysis of expression level of genes connected with dopamine signaling for flies maintained in normal conditions LD12:12 (LD/LD), maintained in DD and developed in darkness (DD/DD). Expression checked for: (A) tyrosine hydroxylase (ple), (B) dopamine receptor Dop1R1, (C) Dop1R2 and (D) DD2R. N = 5. Mean ± SEM.
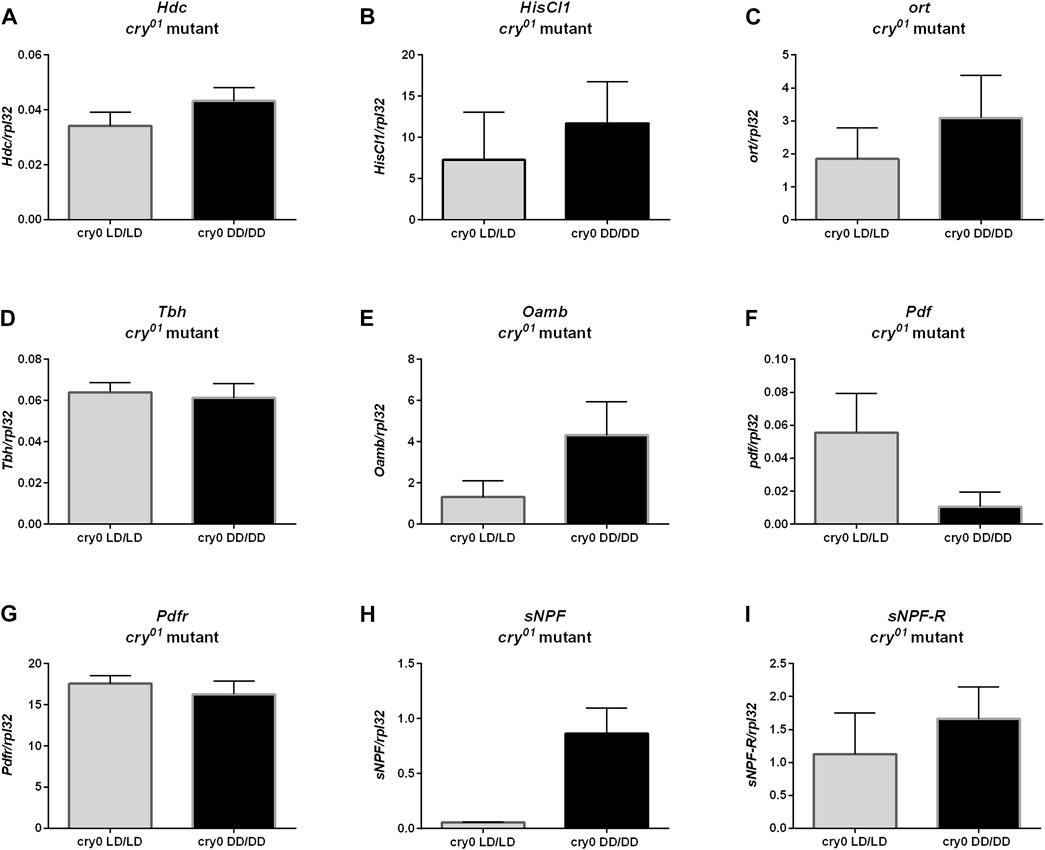
FIGURE 9. cry01 mutants does not show changes in neurotransmitters signaling. mRNA of genes coding (A) Hdc, (B) HisCl1, (C) ort, (D) Tbh, (E) Oamb (F) Pdf, (G) Pdfr, (H) sNPF and (I) sNPF-R did not differ between groups maintained in LD12:12 and darkness during early life. N = 5. Mean ± SEM.
Light affects development of mushroom bodies and dopaminergic input to mushroom bodies
Sleep is controlled by many different cell types, but the most important ones are sleep centers located in the mushroom bodies (MB). Because we observed the strong effect of light during development on sleep level in adult flies, we wondered whether it can be correlated with MB size changes. We used transgenic cross OK-107-Gal4 with UAS-mCD8::GFP to visualize MB. We reared flies in LD or DD, respectively and collected brains of 2-days old males. We measured brain size as well as mushroom bodies of every single individual and found that flies kept in DD had smaller MB (p < 0.0001), while the brain size was not changed (p = 0.4483) (Figures 10A–C).
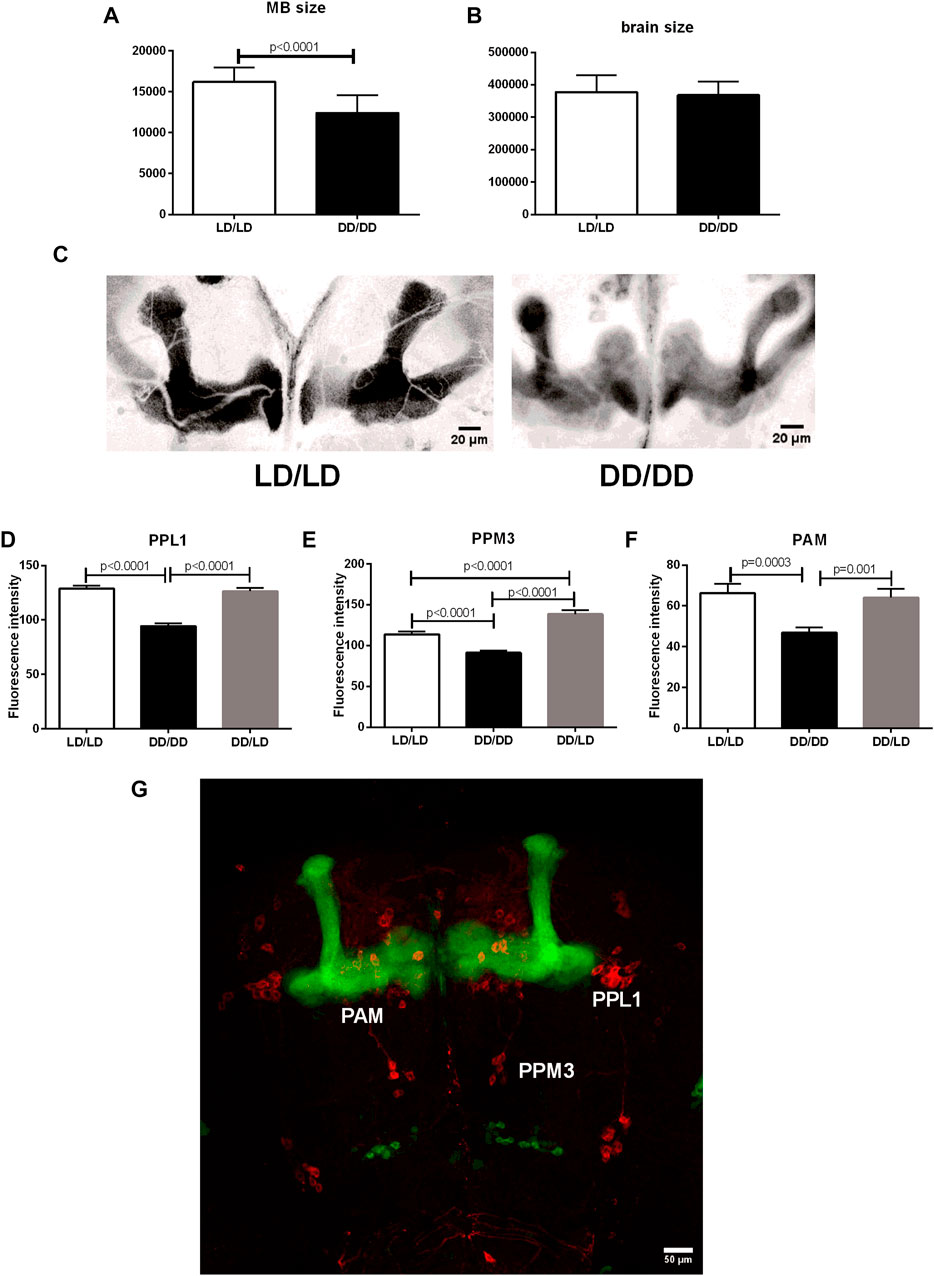
FIGURE 10. Mushroom bodies development and its dopaminergic input depends on light during early life. (A) The size of MBs is significantly decreased in flies from DD/DD group, while (B) the whole brain size was not changed. (C) Representative confocal images of MB from flies developed in LD and DD conditions. Images showed here were modified: selected area was cropped, 8-bit image was color inverted and contrast was enhanced. (D–G): Tyrosine hydroxylase immunofluorescence signal measured in specific dopaminergic cell clusters innervating sleep centers showed decreased level in DD/DD group. DD/LD group showed TH level similar to the control. Total number of brains used for experiments: 30 per group. Mean ± SEM. (G): confocal image of the brain - OK107 > GFP strain has visible mushroom bodies (green), anti-TH immunostaining (red) marks dopaminergic clusters.
Additionally, we checked tyrosine hydroxylase immunofluorescence in specific cell clusters innervating MB. We found that flies kept in DD during development have lower expression of TH in PPL1, PPM3 and PAM neurons, however, transferring flies to the light:dark conditions restored normal level of the enzyme, but in the case of PPM3 the amount of TH was higher than in the control group (Figures 10D–G).
Discussion
Sleep is one of most important functions of organism. During resting time many processes occur, like memory consolidation, wound healing, etc. Sleep is regulated by internal signals coming from the circadian clock and sleep centers, but it is also responsive to external signals, mostly light inputs. Sleep regulation is very complex and requires multicellular communication and homeostasis. Light is a well-known arousal factor, however, mid-day siesta is promoted during the most intense light exposure.
Mechanisms affecting sleep have already been investigated carefully focusing on different aspects, however, little is known about the role of light during development in the regulation of sleep. Larvae have the circadian clock, which can be synchronized by environmental light. Adults eclosing from pupae, which during postembryonic development were reared in constant light or constant darkness, can be easily synchronized by light and they are able to keep normal period of locomotor activity in DD (Sheeba et al., 2002; Zhao et al., 2019). Moreover, single 12 h light pulse in the first instar larva is sufficient to keep the daily rhythmicity in adult life. It has been shown that this entrainment can be observed in an individual fly, however, the whole population is not synchronized, meaning that each fly has different phase of the rhythm. This indicates that the endogenous clock starts to tick independently of light and goes continuously until adulthood (Sehgal et al., 1992; Zhao et al., 2019). The larval time-memory, results from cyclic per and tim expression in the pacemaker in the brain (Kaneko et al., 1997). However, in peripheral oscillators PER is expressed during embryogenesis and postembryonic development, and its level increases during development, but there are no daily cycling of PER before adulthood (Zhao et al., 2019). A light pulse given to larvae causes phase shift of the rhythm in adults and there are differences in the magnitude and direction of shifts between wild type and pers mutants (Kaneko et al., 2000). The mechanism of this process depends on changes in the larval molecular clock induced by light received by both photoreceptive pathways: CRY- and rhodopsin-dependent (Kaneko et al., 2000). Having in mind these larval time-memory aspects, it was not surprising that flies used in our experiments showed normal pattern of activity in adult life and they were able to keep rhythmicity in constant darkness with the period length similar to control. However, total level of activity was increased in insects reared in DD, and their activity index was higher, which means that they were more active during waking time. They also slept less, especially during the night when compared to flies developing in normal LD12:12 conditions. Interestingly, sleep was decreased during first 8 h of the day and during the night starting 3 h after lights-off, while during the rest of the time, the number of sleep bins was similar to the control group. Flies were already synchronized to LD12:12 regime, as morning and evening peaks of activity, amplitude of the rhythm and time of sleep were the same in both groups. Similar changes, higher activity and decreased sleep time, were observed in norpA mutants, however, activity index was not changed and sleep was affected in both during the day and night. This indicates that light perception through photoreceptors is only partially responsible for the observed changes in this behavior. On the other hand, flies lacking functional CRY did not show any changes in activity and sleep profile and level, which indicates that CRY is a key factor affecting observed changes.
The obtained results suggest that our experimental flies had the normally working circadian clock, but some pathways regulating wakefulness were enhanced. Our first goal was to check dopamine, as it is well-known wake-promoting factor (Andretic et al., 2005). Surprisingly, wild-type flies reared in DD showed reduced expression level of ple, encoding tyrosine hydroxylase (TH)—key enzyme in dopamine synthesis, and in effect also dopamine level in the brain most probably was decreased. Since the inhibition of dopamine-releasing neurons causes hyperactivity in response to mechanical startle (Friggi-Grelin et al., 2003), it is possible that also our experimental flies having lower dopamine level could be more active after light changes, as we observed most severe changes in behavior in hours after lights-on and lights-off. Moreover, our flies were probably stressed by transferring them from DD to LD12:12, so they could be also more sensitive to light, and all of this can reduce dopamine level (Neckameyer and Weinstein, 2005). On the other hand, the group DD/DD had lower ple expression level, even without any light input. To investigate this problem in details, we focused on dopaminergic neurons innervating the central complex (PPM3) and mushroom bodies (PPL1 and PAM), the structures hosting a sleep center (Mao and Davis, 2009). Immunofluorescence intensity measurements showed that the lack of light during development decreased TH level in these clusters, however transferring flies to LD rescued this effect. It means that light can directly enhance dopamine synthesis in specific cells, which can affect sleep control. Dysregulation of the balance in dopamine production between different clusters may enhance stress and in effect promote wakefulness.
Although our experimental flies showed decreased dopamine synthesis, at the same time they had increased Dop1R1 and Dop1R2 expression. This support our findings that dopamine signaling is changed in specific target neurons. DopR mutants showed increased sleep level (Lebestky et al., 2009), which supports our data, that shorter sleep time is correlated with increased DopR expression. However, DopR regulates Repetitive Startle-induced Hyperactivity (ReSH) and sleep in the opposite way, acting on specific cells (Lebestky et al., 2009). Wake-promoting effect of dopamine, but not ReSH, occurs mostly in clock neurons (Parisky et al., 2008; Shang et al., 2008; Lebestky et al., 2009). The main pacemaker cells, l-LNvs, are wake-promoting neurons during the day (Parisky et al., 2008; Shang et al., 2008), however, they do not play this role in constant darkness (Shang et al., 2008), as their firing rate is enhanced by the acute light exposure (Sheeba et al., 2008a; 2008b). This group of cells is sensitive to dopamine, which increases cAMP level and causes depolarization of cell membrane, but it does not affect firing rates of the l-LNvs (Shang et al., 2011; Fernandez-Chiappe et al., 2020). Moreover, decreasing of Dop1R2 expression in s-LNvs decreases nighttime sleep (Fernandez-Chiappe et al., 2020). Dopamine signaling to l-LNvs is suppressed by light, and in effect, sleep during the night is more sensitive to dopamine than during the day, and this effect is even stronger in constant darkness (Shang et al., 2011). Our data suggest that Dop1R2 expression can be inhibited by light directly, as flies developing and maintained continuously in DD showed higher level of Dop1R2 mRNA than flies reared in DD and transferred to LD (DD/LD group) or the control flies developing in LD12:12 (LD/LD group). On the other hand, ple and Dop1R1 expression seems to be continuously inhibited by the lack of light during development, while DD2R expression level is not affected by light conditions. The results obtained for cry01 and norpA mutants are very interesting, since they show that gene expression of the dopamine pathway in the group DD/LD strictly depends on photoreception—Dop1R1 is CRY-dependent, while Dop1R2 is PLC—dependent, and ple is both CRY and PLC dependent. The fact, that dopamine acts through Cryptochrome, and both of them are required for nocturnal increased activity was shown also by other authors (Kumar et al., 2012). However, only Dop1R1 seems to be related to the disruption of night-time sleep in flies from DD/DD group, as its enhanced expression follows behavioral changes in wild-type and norpA mutants, while cry01 kept in the same light regimes does not change behavior nor Dop1R1 level.
In case of histamine, the main neurotransmitter used by retina photoreceptors, it has been shown that it is involved in the regulation of sleep/wake cycles through HisCl1 located on clock neurons (Oh et al., 2013; Alejevski et al., 2019). Flies maintained in DD during development had lower expression of Hdc gene, which encodes a key enzyme in histamine synthesis. Moreover, gene expression of both known receptors HisCl1 and Ort was decreased. Histamine has been identified as a wake-promoting agent however our experimental flies showed decreased histamine and increased both activity time and activity during waking. Light effect on histamine synthesis seems to be precisely regulated by Cryptochrome, but not by PLC, while expression of HisCl1 and ort receptor genes are regulated by both PLC and CRY.
Another neurotransmission pathway involved in sleep and affected by darkness during the postembryonic development was octopamine. Here, again, in flies reared in DD we observed lower expression of Tbh (a key enzyme involved in octopamine synthesis) and octopamine receptor gene Oamb, which indicates that this signaling pathway was decreased in adults. It was previously shown that octopamine regulates activity/sleep cycle in larvae, since the activation of Tdc-expressing neurons decreases sleep time, while Tbh and Oamb mutations or silencing increases sleep time (Szuperak et al., 2018). Octopamine is also a wake-promoting agent in adult flies, which forces l-LNv cells to release PDF during the day (Oh et al., 2014). However, it has been shown that sleep regulation using this neurotransmitter does not involve mushroom bodies, although they express octopamine receptors (Crocker and Sehgal, 2008). Octopamine signaling inhibition in DD applied during development, seems to depend on both CRY and PLC, because it was not changed in cry01 and norpA mutants. However, our results suggest that the observed changes in mRNA levels of cry01 and norpA do not drive sleep inhibition during the night.
Neurotransmitters involved in the regulation of the clock mechanism are very interesting in the context of sleep and light. The main clock neurotransmitter is PDF and its function in sleep regulation is very complex. It inhibits dopaminergic arousal neurons PPM3 or activates DN1p, which in turn inhibits tubular bulbar cells (Tubu). PDF released from l-LNVs is also responsible for nocturnal activity (Sheeba et al., 2008a) and light-dependent arousal (Shang et al., 2008). It is possible that the lack of light during development causes defects in the l-LNv excitability, which in turn affects cell physiology, neurotransmitter production, and in effect sleep time. This is supported by the fact, that light-induced firing-rate of l-LNvs depends on CRY, and is not observed in cry mutants (Emery et al., 2000; Sheeba et al., 2008b; Fogle et al., 2011). Decreased Pdf and Pdfr expression observed in our experimental flies may cause weaker inhibition of PPM3 and, in effect, higher total activity.
The second neurotransmitter present in the circadian clock neurons, called sNPF is broadly expressed in the brain, including sleep centers (Nässel et al., 2008; Johard et al., 2009) and its role in the sleep regulation is well documented (Shang et al., 2013). sNPF expressed in s-LNvs shows circadian changes in abundance and promotes normal night-time sleep (Kula-Eversole et al., 2010; Shang et al., 2013), which matches with our results. We observed that expression of PDF and sNPF, as well as their receptors is regulated by light during development in PLC and CRY-dependent manner. However, these changes do not affect behavior.
Comparing wild-type flies with mutants used in the study we can conclude that the exposure to DD during development affects sleep during the night, reduces its time and increases activity time, with lower locomotor activity during wake. This indicates that experimental flies spent more time being active, but their activity level was similar to that in the control. Observed changes are regulated mostly by increasing DopR1 expression. Changes in the expression of other gene studied seems do not to be connected with the observed behavioral phenotype because we did not observe them in norpA mutants which show nocturnal sleep deficits similar to wild-type flies. However, norpA mutants showed increased total activity time with no changes of activity index, which indicates that they were more and longer active than blind flies reared in LD.
The last part of our work showed that lack of light during the development affects the size of mushroom bodies. Flies kept in DD had similar body size, their brain size was also not changed, but the area of MB was significantly decreased. It was previously shown that larvae in DD have reduced number of ganglion mother cells (GMCs) derived from mushroom bodies neuroblasts, similarly to per01 and cry01 mutants kept in LD12:12 (Dapergola et al., 2021). In addition, NBs size was smaller than in control, and their transcriptional activity was decreased. In effect, the size of larval brain was decreased too. Surprisingly, the size of adult brain was normal (Dapergola et al., 2021), which was also confirmed in our study. As it was shown that development of mushroom bodies is affected by light conditions, we decided to check what happens with this sleep-controlling structure in the adult brain. Our results showed that the MB development was constantly affected, as in DD they did not grow to the normal size. It supports an idea that light is necessary for proliferation of specific cell types in the brain. However, since we did not measure Kenyon cells number, it is possible that size changes are the consequence of decreased number of Kc cells or their processes and arborizations, which build MB lobes. Because the total size of the brain was not changed, other brain areas should be enlarged, however we did not have methods to check this interesting issue. The question which part of the brain or which cell types can compensate this difference in size reminds open for further investigations. What is interesting, sleep deprivation also affects NB number (Szuperak et al., 2018). It has been shown that 3 h of mechanical sleep deprivation in the second instar larvae inhibits neuroblasts proliferation in about 15%, and this effect can be recovered after sleep rebound (Szuperak et al., 2018). One of the explanations of the smaller size of MB in flies developing in DD might be that, lack of light decreases their sleep time during larval stage, which in turn affects NBs proliferation rate. This indicates how important is light, clock and sleep in early stages of brain development.
Data availability statement
The original contributions presented in the study are included in the article/supplementary materials, further inquiries can be directed to the corresponding author.
Author contributions
MD designed the study, performed the experiments, analyzed data and wrote the manuscript. AT performed the experiments, EP contributed to the writing of the manuscript. All authors contributed to the article and approved the submitted version.
Funding
This work was funded by grant from the Polish National Science Centre (Narodowe Centrum Nauki, NCN) no. UMO-2017/27/B/NZ3/00859 to MD.
Conflict of interest
The authors declare that the research was conducted in the absence of any commercial or financial relationships that could be construed as a potential conflict of interest.
Publisher’s note
All claims expressed in this article are solely those of the authors and do not necessarily represent those of their affiliated organizations, or those of the publisher, the editors and the reviewers. Any product that may be evaluated in this article, or claim that may be made by its manufacturer, is not guaranteed or endorsed by the publisher.
References
Alejevski F., Saint-Charles A., Michard-Vanhée C., Martin B., Galant S., Vasiliauskas D., et al. (2019). The HisCl1 histamine receptor acts in photoreceptors to synchronize Drosophila behavioral rhythms with light-dark cycles. Nat. Commun. 10, 252. doi:10.1038/s41467-018-08116-7
Andretic R., Van Swinderen B., Greenspan R. J. (2005). Dopaminergic modulation of arousal in Drosophila. Curr. Biol. 15, 1165–1175. doi:10.1016/j.cub.2005.05.025
Aso Y., Hattori D., Yu Y., Johnston R. M., Nirmala A., Ngo T., et al. (2014). The neuronal architecture of the mushroom body provides a logic for associative learning. eLife 3, e04577. doi:10.7554/eLife.04577
Buchner E., Buchner S., Crawford G., Mason W. T., Salvaterra P. M., Sattelle D. B. (1986). Choline acetyltransferase-like immunoreactivity in the brain of Drosophila melanogaster. Cell Tissue Res. 246. doi:10.1007/BF00218999
Busto M., Iyengar B., Campos A. R. (1999). Genetic dissection of behavior: Modulation of locomotion by light in the Drosophila melanogaster larva requires genetically distinct visual system functions. J. Neurosci. 19, 3337–3344. doi:10.1523/jneurosci.19-09-03337.1999
Chatterjee A., Lamaze A., De J., Mena W., Chélot E., Martin B., et al. (2018). Reconfiguration of a multi-oscillator network by light in the Drosophila circadian clock. Curr. Biol. 28, 2007–2017. e4. doi:10.1016/j.cub.2018.04.064
Chen K. F., Peschel N., Zavodska R., Sehadova H., Stanewsky R. (2011). QUASIMODO, a novel GPI-anchored Zona Pellucida protein involved in light input to the drosophila circadian clock. Curr. Biol. 21, 719–729. doi:10.1016/j.cub.2011.03.049
Chung B. Y., Kilman V. L., Keath J. R., Pitman J. L., Allada R. (2009). The GABAA receptor RDL acts in peptidergic PDF neurons to promote sleep in Drosophila. Curr. Biol. 19, 386–390. doi:10.1016/j.cub.2009.01.040
Crocker A., Sehgal A. (2008). Octopamine regulates sleep in Drosophila through protein kinase A-dependent mechanisms. J. Neurosci. 28, 9377–9385. doi:10.1523/JNEUROSCI.3072-08a.2008
Crocker A., Shahidullah M., Levitan I. B., Sehgal A. (2010). Identification of a neural circuit that underlies the effects of octopamine on sleep:wake behavior. Neuron 65, 670–681. doi:10.1016/j.neuron.2010.01.032
Cusumano P., Klarsfeld A., Chélot E., Picot M., Richier B., Rouyer F. (2009). PDF-modulated visual inputs and cryptochrome define diurnal behavior in Drosophila. Nat. Neurosci. 12, 1431–1437. doi:10.1038/nn.2429
Damulewicz M., Ispizua J. I., Ceriani M. F., Pyza E. M. (2020). Communication among photoreceptors and the central clock affects sleep profile. Front. Physiol. 11, 993–1017. doi:10.3389/fphys.2020.00993
Dapergola E., Menegazzi P., Raabe T., Hovhanyan A. (2021). Light stimuli and circadian clock affect neural development in Drosophila melanogaster. Front. Cell Dev. Biol. 9, 595754–595814. doi:10.3389/fcell.2021.595754
Dolezelova E., Dolezel D., Hall J. C. (2007). Rhythm defects caused by newly engineered null mutations in Drosophila’s cryptochrome gene. Genetics 177, 329–345. doi:10.1534/genetics.107.076513
Donlea J. M., Thimgan M. S., Suzuki Y., Gottschalk L., Shaw P. J. (2011). Inducing sleep by remote control facilitates memory consolidation in Drosophila. Science 332, 1571–1576. doi:10.1126/science.1202249
Emery P., Stanewsky R., Hall J. C., Rosbash M. (2000). A unique circadian-rhythm photoreceptor. Nature 404, 456–457. doi:10.1038/35006558
Fernandez-Chiappe F., Hermann-Luibl C., Peteranderl A., Reinhard N., Senthilan P. R., Hieke M., et al. (2020). Dopamine signaling in wake-promoting clock neurons is not required for the normal regulation of sleep in drosophila. J. Neurosci. 40, 9617–9633. doi:10.1523/JNEUROSCI.1488-20.2020
Fogle K. J., Parson K. G., Dahm N. A., Holmes T. C., Sheeba V., Parisky K. M., et al. (2011). CRYPTOCHROME is a blue-light sensor that regulates neuronal firing rate. Science 331, 1409–1413. doi:10.1126/science.1199702
Friggi-Grelin F., Coulom H., Meller M., Gomez D., Hirsh J., Birman S. (2003). Targeted gene expression in Drosophila dopaminergic cells using regulatory sequences from tyrosine hydroxylase. J. Neurobiol. 54, 618–627. doi:10.1002/neu.10185
Grima B., Chélot E., Xia R., Rouyer F. (2004). Morning and evening peaks of activity rely on different clock neurons of the Drosophila brain. Nature 431, 869–873. doi:10.1038/nature02935
Guo F., Holla M., Díaz M. M., Rosbash M. (2018). A circadian output circuit controls sleep-wake arousal in Drosophila. Neuron 100, 624–635. doi:10.1016/j.neuron.2018.09.002
Guo F., Yu J., Jung H. J., Abruzzi K. C., Luo W., Griffith L. C., et al. (2016). Circadian neuron feedback controls the Drosophila sleep-activity profile. Nature 536, 292–297. doi:10.1038/nature19097
Hamasaka Y., Nässel D. R. (2006). Mapping of serotonin, dopamine, and histamine in relation to different clock neurons in the brain of Drosophila. J. Comp. Neurol. 494, 314–330. doi:10.1002/cne.20807
Hassan J., Busto M., Iyengar B., Campos A. R. (2000). Behavioral characterization and genetic analysis of the Drosophila melanogaster larval response to light as revealed by a novel individual assay. Behav. Genet. 30, 59–69. doi:10.1023/A:1002090627601
Haynes P. R., Christmann B. L., Griffith L. C. (2015). A single pair of neurons links sleep to memory consolidation in Drosophila melanogaster. Elife 4. doi:10.7554/eLife.03868
Helfrich-Förster C., Edwards T., Yasuyama K., Wisotzki B., Schneuwly S., Stanewsky R., et al. (2002). The extraretinal eyelet of Drosophila: Development, ultrastructure, and putative circadian function. J. Neurosci. 22, 9255–9266. doi:10.1523/jneurosci.22-21-09255.2002
Hendricks J. C., Finn S. M., Panckeri K. A., Chavkin J., Williams J. A., Sehgal A., et al. (2000). Rest in Drosophila is a sleep-like state. Neuron 25, 129–138. doi:10.1016/S0896-6273(00)80877-6
Huber R., Hill S. L., Holladay C., Biesiadecki M., Tononi G., Cirelli C. (2004). Sleep homeostasis in Drosophila melanogaster. Sleep 27, 628–639. doi:10.1093/sleep/27.4.628
Johard H. A. D., Yoishii T., Dircksen H., Cusumano P., Rouyer F., Helfrich-Förster C., et al. (2009). Peptidergic clock neurons in Drosophila: Ion transport peptide and short neuropeptide F in subsets of dorsal and ventral lateral neurons. J. Comp. Neurol. 516, 59–73. doi:10.1002/cne.22099
Joiner W. J., Crocker A., White B. H., Sehgal A. (2006). Sleep in Drosophila is regulated by adult mushroom bodies. Nature 441, 757–760. doi:10.1038/nature04811
Kaneko M., Hamblen M. J., Hall J. C. (2000). Involvement of the period gene in developmental time-memory: Effect of the per(Short) mutation on phase shifts induced by light pulses delivered to Drosophila larvae. J. Biol. Rhythms 15, 13–30. doi:10.1177/074873040001500103
Kaneko M., Helfrich-Förster C., Hall J. C. (1997). Spatial and temporal expression of the period and timeless genes in the developing nervous system of drosophila: Newly identified pacemaker candidates and novel features of clock gene product cycling. J. Neurosci. 17, 6745–6760. doi:10.1523/jneurosci.17-17-06745.1997
Keene A. C., Mazzoni E. O., Zhen J., Younger M. A., Yamaguchi S., Blau J., et al. (2011). Distinct visual pathways mediate drosophila larval light avoidance and circadian clock entrainment. J. Neurosci. 31, 6527–6534. doi:10.1523/JNEUROSCI.6165-10.2011
Kistenpfennig C., Grebler R., Ogueta M., Hermann-Luibl C., Schlichting M., Stanewsky R., et al. (2017). A new rhodopsin influences light-dependent daily activity patterns of fruit flies. J. Biol. Rhythms 32, 406–422. doi:10.1177/0748730417721826
Klarsfeld A., Picot M., Vias C., Chélot E., Rouyer F. (2011). Identifying specific light inputs for each subgroup of brain clock neurons in Drosophila larvae. J. Neurosci. 31, 17406–17415. doi:10.1523/JNEUROSCI.5159-10.2011
Kula-Eversole E., Nagoshi E., Shang Y., Rodriguez J., Allada R., Rosbash M. (2010). Surprising gene expression patterns within and between PDF-containing circadian neurons in Drosophila. Proc. Natl. Acad. Sci. U. S. A. 107, 13497–13502. doi:10.1073/pnas.1002081107
Kumar S., Chen D., Sehgal A. (2012). Dopamine acts through Cryptochrome to promote acute arousal in Drosophila. Genes Dev. 26, 1224–1234. doi:10.1101/gad.186338.111
Kunst M., Hughes M. E., Raccuglia D., Felix M., Li M., Barnett G., et al. (2014). Calcitonin gene-related peptide neurons mediate sleep-specific circadian output in Drosophila. Curr. Biol. 24, 2652–2664. doi:10.1016/j.cub.2014.09.077
Lebestky T., Chang J. S. C., Dankert H., Zelnik L., Kim Y. C., Han K. A., et al. (2009). Two different forms of arousal in Drosophila are oppositely regulated by the dopamine D1 receptor ortholog DopR via distinct neural circuits. Neuron 64, 522–536. doi:10.1016/j.neuron.2009.09.031
Li J., Mahoney B. D., Jacob M. S., Caron S. J. C. (2020). Visual input into the Drosophila melanogaster mushroom body. Cell Rep. 32, 108138. doi:10.1016/j.celrep.2020.108138
Liu Q., Liu S., Kodama L., Driscoll M. R., Wu M. N. (2012). Two dopaminergic neurons signal to the dorsal fan-shaped body to promote wakefulness in Drosophila. Curr. Biol. 22, 2114–2123. doi:10.1016/j.cub.2012.09.008
Liu W. W., Wilson R. I. (2013). Glutamate is an inhibitory neurotransmitter in the Drosophila olfactory system. Proc. Natl. Acad. Sci. U. S. A. 110, 10294–10299. doi:10.1073/pnas.1220560110
Malpel S., Klarsfeld A., Rouyer F. (2002). Larval optic nerve and adult extra-retinal photoreceptors sequentially associate with clock neurons during Drosophila brain development. Development 129, 1443–1453. doi:10.1242/dev.129.6.1443
Mao Z., Davis R. L. (2009). Eight different types of dopaminergic neurons innervate the Drosophila mushroom body neuropil: Anatomical and physiological heterogeneity. Front. Neural Circuits 3, 5. doi:10.3389/neuro.04.005.2009
Mauss A. S., Meier M., Serbe E., Borst A. (2014). Optogenetic and pharmacologic dissection of feedforward inhibition in Drosophila motion vision. J. Neurosci. 34, 2254–2263. doi:10.1523/JNEUROSCI.3938-13.2014
Mauss A. S., Pankova K., Arenz A., Nern A., Rubin G. M., Borst A. (2015). Neural circuit to integrate opposing motions in the visual field. Cell 162, 351–362. doi:10.1016/j.cell.2015.06.035
Mazzoni E. O., Desplan C., Blau J. (2005). Circadian pacemaker neurons transmit and modulate visual information to control a rapid behavioral response. Neuron 45, 293–300. doi:10.1016/j.neuron.2004.12.038
McCarthy E. V., Wu Y., deCarvalho T., Brandt C., Cao G., Nitabach M. N. (2011). Synchronized bilateral synaptic inputs to Drosophila melanogaster neuropeptidergic rest/arousal neurons. J. Neurosci. 31, 8181–8193. doi:10.1523/JNEUROSCI.2017-10.2011
Morante J., Desplan C. (2008). The color-vision circuit in the medulla of Drosophila. Curr. Biol. 18, 553–565. doi:10.1016/j.cub.2008.02.075
Nässel D. R., Enell L. E., Santos J. G., Wegener C., Johard H. A. D. (2008). A large population of diverse neurons in the Drosophila central nervous system expresses short neuropeptide F, suggesting multiple distributed peptide functions. BMC Neurosci. 9, 90. doi:10.1186/1471-2202-9-90
Neckameyer W. S., Weinstein J. S. (2005). Stress affects dopaminergic signaling pathways in Drosophila melanogaster. Stress 8, 117–131. doi:10.1080/10253890500147381
Oh Y., Jang D., Sonn J. Y., Choe J. (2013). Histamine-HisCl1 receptor Axis regulates wake-promoting signals in Drosophila melanogaster. PLoS One 8, e68269. doi:10.1371/journal.pone.0068269
Oh Y., Yoon S. E., Zhang Q., Chae H. S., Daubnerová I., Shafer O. T., et al. (2014). A homeostatic sleep-stabilizing pathway in Drosophila composed of the sex peptide receptor and its ligand, the myoinhibitory peptide. PLoS Biol. 12, e1001974. doi:10.1371/journal.pbio.1001974
Pantazis A., Segaran A., Liu C. H., Nikolaev A., Rister J., Thum A. S., et al. (2008). Distinct roles for two histamine receptors (hclA and hclB) at the Drosophila photoreceptor synapse. J. Neurosci. 28, 7250–7259. doi:10.1523/JNEUROSCI.1654-08.2008
Parisky K. M., Agosto J., Pulver S. R., Shang Y., Kuklin E., Hodge J. J. L., et al. (2008). PDF cells are a GABA-responsive wake-promoting component of the Drosophila sleep circuit. Neuron 60, 672–682. doi:10.1016/j.neuron.2008.10.042
Picot M., Cusumano P., Klarsfeld A., Ueda R., Rouyer F. (2007). Light activates output from evening neurons and inhibits output from morning neurons in the Drosophila circadian clock. PLoS Biol. 5, e315–e2521. doi:10.1371/journal.pbio.0050315
Pitman J. L., McGill J. J., Keegan K. P., Allada R. (2006). A dynamic role for the mushroom bodies in promoting sleep in Drosophila. Nature 441, 753–756. doi:10.1038/nature04739
Pollack I., Hofbauer A. (1991). Histamine-like immunoreactivity in the visual system and brain of Drosophila melanogaster. Cell Tissue Res. 266, 391–398. doi:10.1007/BF00318195
Qian Y., Cao Y., Deng B., Yang G., Li J., Xu R., et al. (2017). Sleep homeostasis regulated by 5HT2b receptor in a small subset of neurons in the dorsal fan-shaped body of drosophila. Elife 6, e26519. doi:10.7554/eLife.26519
Raghu S. V., Borst A. (2011). Candidate glutamatergic neurons in the visual system of drosophila. PLoS One 6, 194722–e19511. doi:10.1371/journal.pone.0019472
Redlin U. (2001). Neural basis and biological function of masking by light in mammals: Suppression of melatonin and locomotor activity. Chronobiol. Int. 18, 737–758. doi:10.1081/CBI-100107511
Robinson J. E., Paluch J., Dickman D. K., Joiner W. J. (2016). ADAR-mediated RNA editing suppresses sleep by acting as a brake on glutamatergic synaptic plasticity. Nat. Commun. 7, 10512. doi:10.1038/ncomms10512
Schlichting M., Weidner P., Diaz M., Menegazzi P., Dalla Benetta E., Helfrich-Förster C., et al. (2019). Light-Mediated circuit switching in the Drosophila neuronal clock network. Curr. Biol. 29, 3266–3276. doi:10.1016/j.cub.2019.08.033
Sehgal A., Price J., Young M. W. (1992). Ontogeny of a biological clock in Drosophila melanogaster. Proc. Natl. Acad. Sci. U. S. A. 89, 1423–1427. doi:10.1073/pnas.89.4.1423
Shang Y., Donelson N. C., Vecsey C. G., Guo F., Rosbash M., Griffith L. C. (2013). Short neuropeptide F is a sleep-promoting inhibitory modulator. Neuron 80, 171–183. doi:10.1016/j.neuron.2013.07.029
Shang Y., Griffith L. C., Rosbash M. (2008). Light-arousal and circadian photoreception circuits intersect at the large PDF cells of the Drosophila brain. Proc. Natl. Acad. Sci. U. S. A. 105, 19587–19594. doi:10.1073/pnas.0809577105
Shang Y., Haynes P., Pírez N., Harrington K. I., Guo F., Pollack J., et al. (2011). Imaging analysis of clock neurons reveals light buffers the wake-promoting effect of dopamine. Nat. Neurosci. 14, 889–895. doi:10.1038/nn.2860
Shaw P. J., Cirelli C., Greenspan R. J., Tononi G. (2000). Correlates of sleep and waking in Drosophila melanogaster. Science 287, 1834–1837. doi:10.1126/science.287.5459.1834
Sheeba V., Chandrashekaran M. K., Joshi A., Sharma V. K. (2002). Developmental plasticity of the locomotor activity rhythm of Drosophila melanogaster. J. Insect Physiol. 48, 25–32. doi:10.1016/S0022-1910(01)00139-1
Sheeba V., Fogle K. J., Kaneko M., Rashid S., Chou Y. T., Sharma V. K., et al. (2008a). Large ventral lateral neurons modulate arousal and sleep in Drosophila. Curr. Biol. 18, 1537–1545. doi:10.1016/j.cub.2008.08.033
Sheeba V., Gu H., Sharma V. K., O’Dowd D. K., Holmes T. C. (2008b). Circadian- and light-dependent regulation of resting membrane potential and spontaneous action potential firing of Drosophila circadian pacemaker neurons. J. Neurophysiol. 99, 976–988. doi:10.1152/jn.00930.2007
Sinakevitch-Pean I., Geffard M., Plotnikova S. I. (2001). Localization of glutamate in the nervous system of the fly Drosophila melanogaster: An immunocytochemical study. J. Evol. Biochem. Physiol. 37, 83–88. doi:10.1023/A:1017574120553
Sitaraman D., Aso Y., Rubin G. M., Nitabach M. N. (2015). Control of sleep by dopaminergic inputs to the drosophila mushroom body. Front. Neural Circuits 9, 73. doi:10.3389/fncir.2015.00073
Stoleru D., Peng Y., Agosto J., Rosbash M. (2004). Coupled oscillators control morning and evening locomotor behaviour of Drosophila. Nature 431, 862–868. doi:10.1038/nature02926
Szuperak M., Churgin M. A., Borja A. J., Raizen D. M., Fang-Yen C., Kayser M. S. (2018). A sleep state in Drosophila larvae required for neural stem cell proliferation. Elife 7, 332200–e33319. doi:10.7554/eLife.33220
Takemura S. Y., Karuppudurai T., Ting C. Y., Lu Z., Lee C. H., Meinertzhagen I. A. (2011). Cholinergic circuits integrate neighboring visual signals in a drosophila motion detection pathway. Curr. Biol. 21, 2077–2084. doi:10.1016/j.cub.2011.10.053
Tomita J., Ban G., Kato Y. S., Kume K. (2021). Protocerebral bridge neurons that regulate sleep in Drosophila melanogaster. Front. Neurosci. 15, 647117–647215. doi:10.3389/fnins.2021.647117
Tomita J., Ueno T., Mitsuyoshi M., Kume S., Kume K. (2015). The NMDA Receptor promotes sleep in the fruit fly, drosophila melanogaster. PLoS One 10, e0128101. doi:10.1371/journal.pone.0128101
Ueno T., Tomita J., Tanimoto H., Endo K., Ito K., Kume S., et al. (2012). Identification of a dopamine pathway that regulates sleep and arousal in Drosophila. Nat. Neurosci. 15, 1516–1523. doi:10.1038/nn.3238
VanVickle-Chavez S. J., Van Gelder R. N. (2007). Action spectrum of Drosophila cryptochrome. J. Biol. Chem. 282, 10561–10566. doi:10.1074/jbc.M609314200
Vogt K., Aso Y., Hige T., Knapek S., Ichinose T., Friedrich A. B., et al. (2016). Direct neural pathways convey distinct visual information to Drosophila mushroom bodies. eLife 5, 1–13. doi:10.7554/eLife.14009
Yao Z., Shafer O. T. (2014). The Drosophila circadian clock is a variably coupled network of multiple peptidergic units. Science 343, 1516–1520. doi:10.1126/science.1251285
Yasuyama K., Meinertzhagen I. A. (1999). Extraretinal photoreceptors at the compound eye’s posterior margin in Drosophila melanogaster. J. Comp. Neurol. 412, 193–202. doi:10.1002/(sici)1096-9861(19990920)412:2<193::aid-cne1>3.0.co;2-0
Yasuyama K., Salvaterra P. M. (1999). Localization of choline acetyltransferase-expressing neurons in Drosophila nervous system. Microsc. Res. Tech. 42, 65–79. doi:10.1002/(SICI)1097-0029(19990415)45:2<65::AID-JEMT2>3.0.CO;2-0
Yuan Q., Joiner W. J., Sehgal A. (2006). A sleep-promoting role for the Drosophila serotonin receptor 1A. Curr. Biol. 16, 1051–1062. doi:10.1016/j.cub.2006.04.032
Yuan Q., Lin F., Zheng X., Sehgal A. (2005). Serotonin modulates circadian entrainment in Drosophila. Neuron 47, 115–127. doi:10.1016/j.neuron.2005.05.027
Zhang L., Lear B. C., Seluzicki A., Allada R. (2009). The CRYPTOCHROME photoreceptor gates PDF neuropeptide signaling to set circadian network hierarchy in Drosophila. Curr. Biol. 19, 2050–2055. doi:10.1016/j.cub.2009.10.058
Keywords: clock, sleep, dopamine pathway, histamine, octopamine, cryptochrome, mushroom bodies
Citation: Damulewicz M, Tyszka A and Pyza E (2022) Light exposure during development affects physiology of adults in Drosophila melanogaster. Front. Physiol. 13:1008154. doi: 10.3389/fphys.2022.1008154
Received: 31 July 2022; Accepted: 14 November 2022;
Published: 25 November 2022.
Edited by:
Sylvia Anton, Institut National de recherche pour l’agriculture, l’alimentation et l’environnement (INRAE), FranceReviewed by:
Abhishek Chatterjee, UMR7618 Institut d'écologie et des sciences de l’environnement de Paris (IEES), FranceAnna Hovhanyan, Julius Maximilian University of Würzburg, Germany
Copyright © 2022 Damulewicz, Tyszka and Pyza. This is an open-access article distributed under the terms of the Creative Commons Attribution License (CC BY). The use, distribution or reproduction in other forums is permitted, provided the original author(s) and the copyright owner(s) are credited and that the original publication in this journal is cited, in accordance with accepted academic practice. No use, distribution or reproduction is permitted which does not comply with these terms.
*Correspondence: Milena Damulewicz, bWlsZW5hLmRhbXVsZXdpY3pAdWouZWR1LnBs
†These authors have contributed equally to this work