- 1Fishery College, Guangdong Ocean University, Zhanjiang, China
- 2Pearl Breeding and Processing Engineering Technology Research Centre of Guangdong Province, Zhanjiang, China
- 3Guangdong Science and Innovation Center for Pearl Culture, Zhanjiang, China
- 4Guangdong Provincial Engineering Laboratory for Mariculture Organism Breeding, Zhanjiang, China
The polyamine putrescine (Put) is a ubiquitous small cationic amine. It plays an essential role in controlling the innate immune response. However, little is known about its function in mollusks. In this study, the Put content was observed to increase in the serum of pearl oyster Pinctada fucata martensii after 6 and 24 h of lipopolysaccharide (LPS) stimulation. Activities of superoxide dismutase (SOD), catalase (CAT), and glutathione peroxidase (GSH-Px) increased, and nitric oxide synthase was downregulated in the Put group (i.e., combined treatment with Put and LPS) compared with that in the LPS group (i.e., combined treatment with phosphate-buffered saline and LPS). Furthermore, activities of alkaline phosphatase and acid phosphatase were inhibited after 6 h of LPS stimulation. The expression levels of the nuclear factor kappa B, IκB kinase, Janus kinase, and signal transducer and activator of transcription proteins genes were all significantly suppressed at 12 and 24 h in the Put group. Pseudomonas aeruginosa and Bacillus subtilis grew better after being incubated with the serum from the Put group than that from the LPS group. Additionally, the Put treatment remarkably inhibited the autophagy of hemocytes mediated by the AMP-activated protein kinase-mammalian target of rapamycin-Beclin-1 pathway. This study demonstrated that Put can effectively inhibit the inflammatory response induced by LPS in pearl oysters. These results provide useful information for further exploration of the immunoregulatory functions of polyamines in bivalves and contribute to the development of immunosuppressive agents.
Introduction
Many marine bivalves are economically important. However, diseases caused by bacteria or viruses present in the surrounding water environment negatively affect the development of the marine bivalve aquaculture industry (Mitta et al., 2000; Seo et al., 2010). Aside from physical and biological barriers against invaders, cellular and humoral immunity constitutes the main part of the innate immune response of bivalves (Antunes et al., 2014; Song et al., 2015). Hemocytes are an important part of the innate immune response of bivalves. Hemocytes act through phagocytosis (Hanington et al., 2010) and release cytotoxic molecules, such as nitric oxide and hydrogen, to participate in immune response (Canesi et al., 2002). Hemocytes located in areas in close contact with external microorganisms act as sentinels for any impending infection and migrate to any infection site (Allam and Espinosa, 2016). In addition, the oxidative stress caused by a bacterial infection can trigger autophagy, which is associated with cytoprotection (Yuan et al., 2009). The autophagy response of hemocytes plays a key role in the process of resisting potential pathogens in bivalves, and it determines the expression of autophagy-related genes and signaling pathways (Moreau et al., 2015; Balbi et al., 2018; Liu et al., 2020). Although hemocytes are known to have an effect on the immune system, the efficiency of defense comes from the activation of hemocytes and the components dissolved in serum, such as lectins, antioxidant enzymes, reactive oxygen species, and lysosomal enzymes (Mitta et al., 2000; Canesi et al., 2002). At the first glance, similar to that of other invertebrates, the structure of the existing immune system of bivalves is relatively simple and contains only the innate immune system, lacking an adaptive immune system (Pipe and Coles, 1995). However, accumulating evidence shows that many molecules in bivalves, including acetylcholine (Liu Z. et al., 2016; Cao et al., 2021), biogenic amines (e.g., 5-HT, epinephrine/norepinephrine, and dopamine) (Tiscar and Mosca, 2004; Dong et al., 2017), and amino acid substances (e.g., glutamic acid and γ-aminobutyric acid) (Tiscar and Mosca, 2004; Li et al., 2016), function in regulating their immune response to pathogenic bacteria, viruses, or mechanical damage.
Polyamines, such as putrescine (Put), spermidine (Spd), and spermine, are positively charged biogenic amines that are ubiquitous in all organisms, expect for certain archaeans (Chen and Martynowicz, 1984). The level of polyamines in organisms is strictly regulated. Environmental changes, tumors, oxidative stress, pathogen infection, or injury will cause changes in the polyamine levels (Wickström, 1991; Cipolla et al., 1993; Kournoutou et al., 2014). Polyamines are not only involved in cell growth, gene regulation, differentiation, and development but also have recently been found to alter the inflammatory response in vitro and immunity (Yuan et al., 2001; Pirinen et al., 2007; Choi and Park, 2012). They act as negative immunomodulators of natural killer cell activity (Quemener et al., 1994), lymphocytes (Byrd et al., 1977), and neutrophil locomotion (Ferrante et al., 1986). Polyamines are also important antioxidants (Guérin et al., 2001; Shoji et al., 2005; Toro-Funes et al., 2013; Liu G. et al., 2016), free radical scavengers (Ha et al., 1998), and anti-inflammatory agents (Byrd et al., 1977; Løvaas and Carlin, 1991; Li et al., 2020). In general, polyamines exert their antioxidant function by increasing the activity of antioxidant molecules of an organism, such as superoxide dismutase (SOD), catalase (CAT), and glutathione peroxidase (GSH-Px), and inhibiting the generation of free radicals (Durmu and Kadioğlu, 2005; David et al., 2008; Reyes-Becerril et al., 2011; Fang et al., 2016; Yerra et al., 2016). The immune-related genes in gilthead seabream leucocytes are actively regulated by polyamines, especially Put (Reyes-Becerril et al., 2011). Recent studies have found that many signaling pathways are involved in the regulation of the immune response of polyamines, including the nuclear factor kappa B (NF-κB), phosphoinositide 3-kinase/protein kinase B, forkhead box O-3, Janus kinase (JAK)-signal transducer and activator of transcription proteins (STAT), and mitogen-activated protein kinase pathways (Reyes-Becerril et al., 2011; Choi and Park, 2012; Li et al., 2020). Polyamines also regulate autophagy through the AMP-activated protein kinase (AMPK)-mammalian target of rapamycin (mTOR)-Unc-51 like autophagy activating kinase 1 signaling pathway (Liu et al., 2019c). In invertebrates, polyamines were first observed in Cionia intestinalisin in 1954 (Ackermann and Janka, 1954). Subsequently, polyamines have been detected in sea urchin, sea cucumber, sea squirt, oysters, short-necked clam, C. elegans, planarians, earthworms, mussel, and Bombyx mori (Hamana et al., 1991, 1995; Kournoutou et al., 2014; Yerra et al., 2016). In planarians and earthworms, the synthesis of Put is stimulated during regeneration, and under temperature and osmotic stresses, the levels of Put and Spd in C. elegans, planarians, and earthworms are temporarily increased (Hamana et al., 1995). The levels of polyamines fluctuate under Cd+ stress in mussels (Kournoutou et al., 2014). These results suggested that polyamines may be important regulatory molecules in stress response. However, changes in polyamines in bivalves after pathogen infection and their functions in innate immune response remain unknown.
The pearl oyster Pinctada fucata martensii is mainly distributed in the southern provinces of China and Japan. It is one of the most economically important species used in the production of seawater pearls. In this study, we evaluated the changes in the Put levels in pearl oysters under the LPS stress. We also investigated the immune regulation function and molecular mechanism of Put in LPS-induced inflammation. Results suggested that Put may have potential functions in oxidative stress and inflammation in pearl oysters. These results provided some data for exploring further the functions of polyamines as immunomodulators in bivalves.
Materials and Methods
Experimental Design and Sample Preparation
Pearl oysters (∼1.5 years old) were obtained from Houhong, Zhanjiang, Guangdong, China. The reagent was injected into the adductor muscle via a 100 μl microsyringe. The challenged group was injected with 100 μl of 0.5 mg/ml LPS (Sigma-Aldrich, St. Louis, MO, United States). The control group was injected with 100 μl of 1 × phosphate-buffered saline (1 × PBS) in the same way. In the Put group, 1 h before LPS injection, 100 μl of 1 mM Put dihydrochloride (Sigma-Aldrich, St. Louis, MO, United States) was injected. The pearl oysters injected with 100 μl of the 1 × PBS solution were used as the control group before LPS stimulation (i.e., LPS group). After LPS stimulation, the pearl oysters were maintained at (25 ± 1)°C in cistern with filtered seawater. Hemolymph was then extracted from eight pearl oysters in each group by using a 2 ml syringe at 6, 12, 24, and 48 h after LPS stimulation. Hemocytes and serum were separated from the hemolymph via centrifugation at 3,000 r/min for 5 min at 4°C. The collected hemocytes were resuspended in the TRIzol reagent (Invitrogen, Carlsbad, CA, United States). Both serum and hemocytes were quick-frozen in liquid nitrogen and stored at −80°C until use.
Detection of Put Content in Serum After Lipopolysaccharide Stimulation
Serum was collected from five individuals at different times post-LPS stimulation in each group. The Put levels were determined using kits (mlbio, Shanghai, China) following the instructions of the manufacturer. The procedure includes the following: 10 μl of the testing sample, 40 μl of the sample diluent, and 100 μl of the horseradish peroxidase-conjugate reagent were added to each well. Then, it was covered with an adhesive strip and incubated for 60 min at 37°C. Each well was aspirated and washed, repeating the process four times for a total of five washes. Notably, 50 μl of chromogen solution A and 50 μl of chromogen solution B were added to each well, gently mixed, incubated for 15 min at 37°C, and protected from light. Finally, 50 μl of stop solution was added to each well, and the OD450 value of each well at 37°C was determined using a microplate reader. Five samples of parallel tests were performed on each group.
Detection of Immune-Related Enzymes and Antibacterial Activities of Serum
After the Put treatment, the activities of antioxidant-related enzymes were detected in five individuals. SOD, CAT, activities of lysozyme (LYS), acid phosphatase (ACP), alkaline phosphatase (ALP), and GSH-Px were detected using kits (mlbio, Shanghai, China) in accordance with the instructions. The detection protocols are the same as the detection protocols of the Put level described in the “Detection of Put content in serum after LPS stimulation” section. The activity of iNOS was tested by kits (Nanjing Jiancheng Bioengineering Research Institute, Nanjing, China) following the protocol of the manufacturer. First, 30 μl serum and 40 μl reagent 4 were mixed. Then, 200 μl reagent 1, 10 μl reagent 2, and 100 μl reagent 3 were added to each tube. They were gently mixed and incubated at 37°C for 15 min. Finally, 100 μl of reagent 4 and 2 ml of reagent 5 were added, and the OD450 value was detected using a microplate reader.
The antibacterial activity of serum after the Put treatment was detected. Pseudomonas aeruginosa and Bacillus subtilis were cultured to the logarithmic growth phase with the LB medium. Afterward, 10 μl of the bacterial solution and 50 μl of serum were added to a sterile 96-well plate and mixed well. After incubating for 3 h at room temperature, 200 μl of LB medium was added to each well. The OD600 value of each well at 37°C was determined using a microplate reader (the test was performed once every 30 min and continued for 24 h). Five samples of parallel tests were performed on each group.
Real-Time PCR for Detection of the Expression of Immune-Related Genes After Put Treatment
The total RNA of all samples was isolated using the TRIzol method (Invitrogen, United States). The cDNA template was prepared with Moloney murine leukemia virus (M-MLV) reverse transcriptase (RT) (Takara, Clontech, Japan). The relative expression of target genes was detected via quantitative RT-PCR (qRT-PCR) performed on the Applied Biosystems 7500/7500 instrument. β-Actin was selected to assess the relative expression of genes. All the primers used herein were designed using the Primer Premier 5 software (Table 1).
Monodansylcadaverine Assay for Autophagy Evaluation
The autophagy levels in hemocytes were detected using the monodansylcadaverine (MDC) autophagy staining detection kit (Beijing Solarbio Science & Technology, Beijing, China). After 48 h of Put + LPS treatment, 200 μl of hemolymph was drawn using a 2 ml syringe, and then the hemolymph was spread on the cover glass. After 10 min, the cells were washed twice with 1× washing buffer and the supernatant was removed. Then, 90 μl of 1× washing buffer and 10 μl of MDC stain were added and the cells were incubated at room temperature for 1 h. Three samples of parallel tests were performed on each group. The hemocyte autophagy levels were observed and photographed using a fluorescence microscope (Nikon ECLIPSE Ni, DS-Ri2, Tokyo, Japan). Five fields of view were randomly selected for each sample to calculate the autophagy ratio of hemocytes.
Data Analysis
The data on qRT-PCR, detection of enzyme activity, and antibacterial activity were analyzed using the SPSS 22.2 software. Differences in enzyme activity and gene expression level at different time points in the same group were determined using ANOVA. Differences in enzyme activity, gene expression level, bacterial growth level, and hemocyte autophagy levels between different groups at the same time point were determined via t-test. P-values < 0.05 were considered statistically significant.
Results
Changes in Put Concentration in Serum After Lipopolysaccharide Stimulation
The concentration of Put in serum after 6, 12, and 24 h of LPS stimulation was quantified (Figure 1). In contrast to that in the in the PBS group, Put concentrations in serum significantly increased after 6 and 24 h by 1.24- and 1.32-fold, respectively, in the LPS stimulation group (P < 0.05). However, changes in Put concentrations in serum were not significant after 12 h of LPS stimulation (P > 0.05). Therefore, the Put concentration was sensitive to LPS stimulation in pearl oysters.
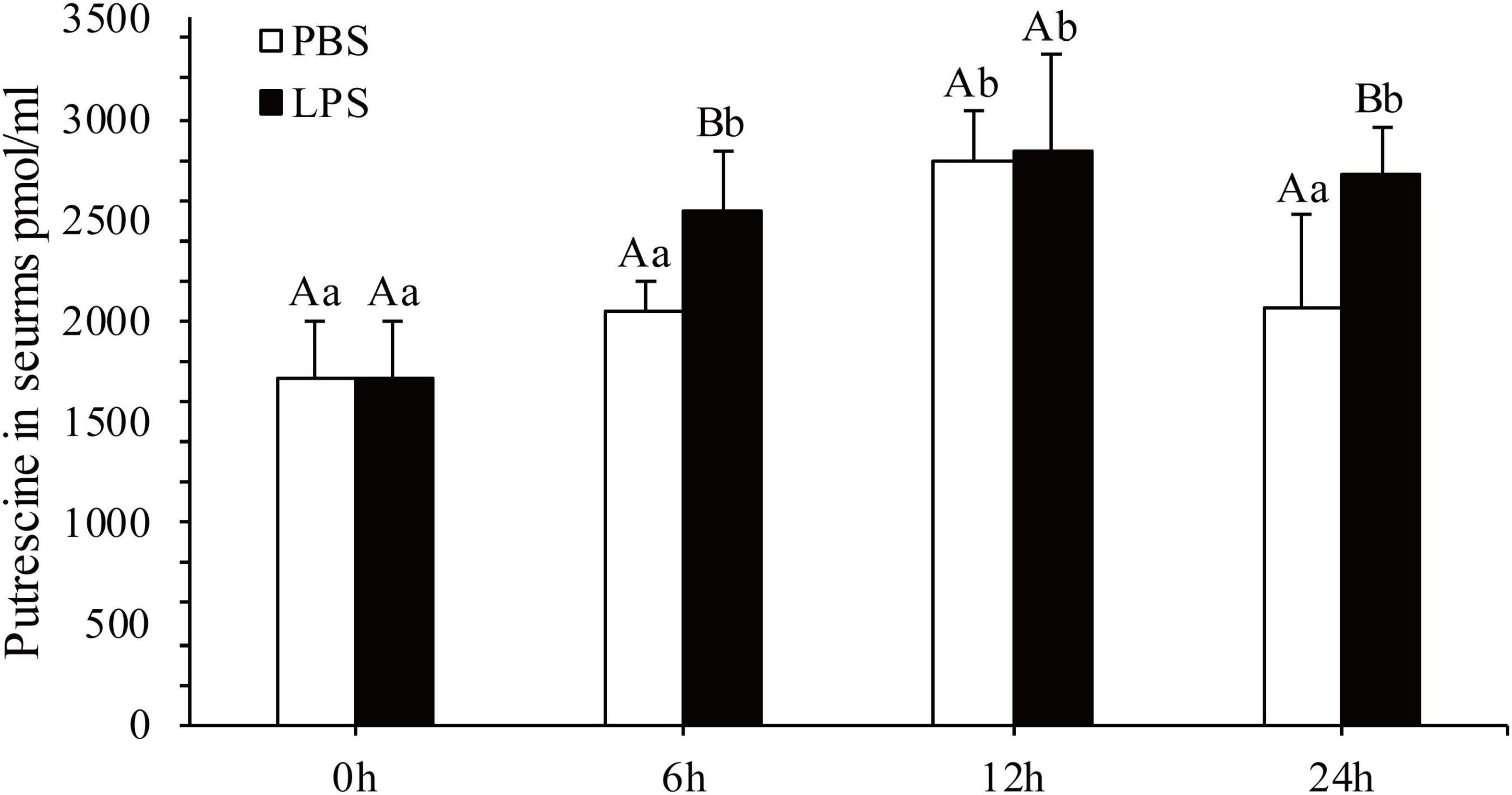
Figure 1. Detection of the putrescine level in serum after lipopolysaccharide (LPS) stimulation. Values are shown as mean ± SE (N = 5). Lowercase letters represent differences at different time points in the same groups, and capital letters represent differences in the different groups at same time points.
Immune- and Antioxidant-Related Enzymes and Antibacterial Activities
The regulatory functions of Put in LPS-induced humoral immunity were investigated by determining the LYS, ACP, ALP, SOD, CAT, GSH-Px, and inducible nitric oxide synthase (iNOS) in the Put group (i.e., combined treatment with Put and LPS) and the LPS group (i.e., combined treatment with PBS and LPS). As shown in Figures 2A–C, ACP and ALP activities were significantly inhibited by 1.24- and 1.21-fold, respectively, at 6 h in the Put group. However, the ACP activity was significantly induced at 48 h in the Put group compared with that in the LPS group (Figures 2B,C, P < 0.05). In contrast, the LYS activity did not significantly change (Figure 2A, P > 0.05). Furthermore, the SOD activity increased by 1.23-fold at 6 h in the Put group compared with that in the LPS group (Figure 2D, P < 0.05). In the Put group, the GSH-Px activity significantly increased by 1.25- to 1.42-fold at 6–48 h compared with that in the LPS group (Figure 2E, P < 0.05). The CAT activity was upregulated by 1.17-fold at 6 h and then downregulated at 24 and 48 h in the Put group compared with that in the LPS group (Figure 2F, P < 0.05). The iNOS activity was downregulated by 5.45- to 6.1-fold at 12–48 h in the Put group compared with that in the LPS group (Figure 3, P < 0.05). These results indicated that Put pretreatment remarkably attenuated oxidative stress.
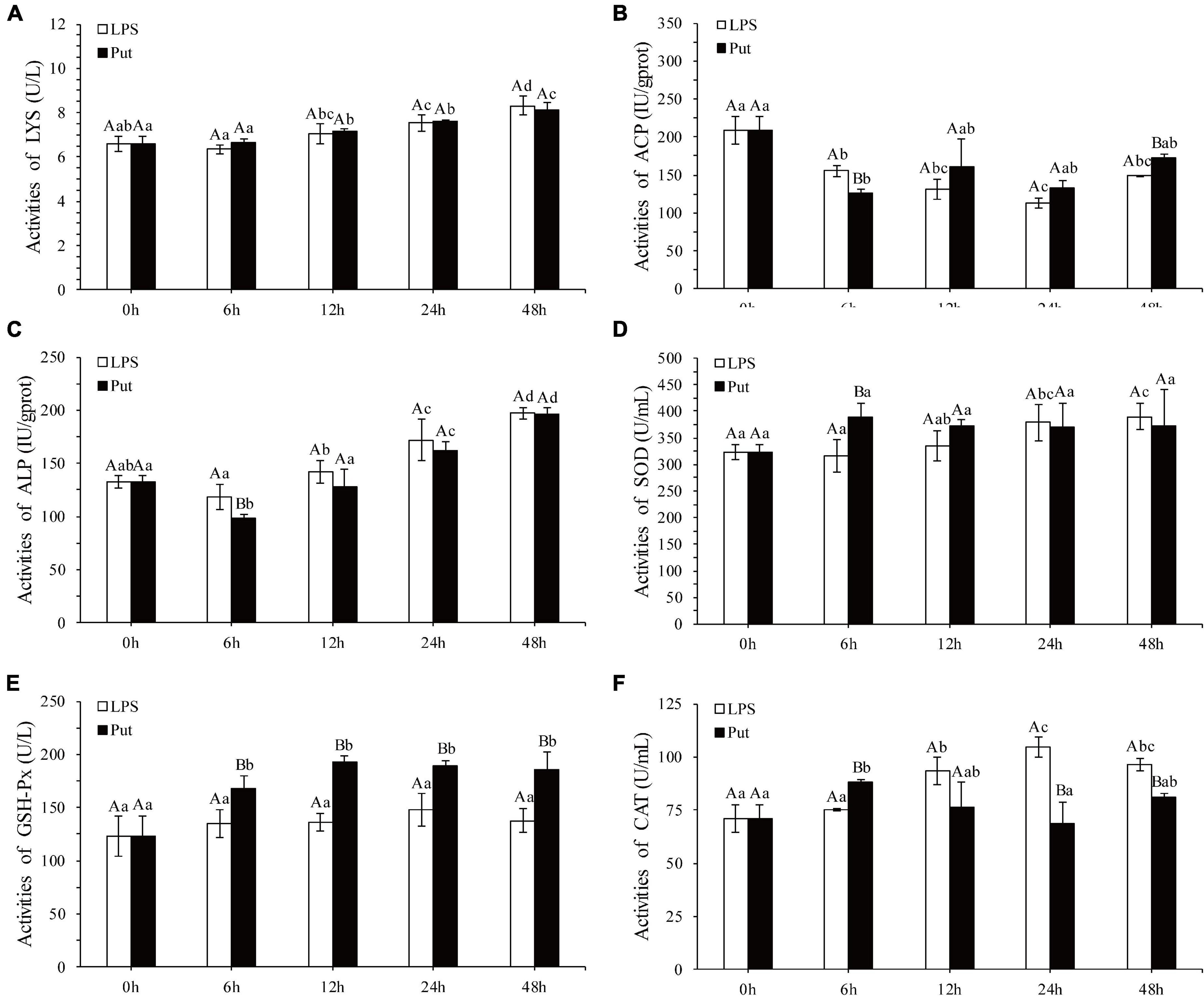
Figure 2. Detection of enzyme activity after the putrescine treatment. LYS, lysozyme; ACP, acid phosphatase; ALP, alkaline phosphatase; SOD, superoxide dismutase; GSH-Px, glutathione peroxidase; CAT, catalase. Values are shown as mean ± SE (N = 5). Lowercase letters represent differences at different time points in the same groups, and capital letters represent differences in the different groups at same time points. Lipopolysaccharide (LPS) group, PBS_LPS; Put group, Put dihydrochloride_LPS. (A–F) represent the activities of LYS/ACP/ALP/SOD/GSH-Px/CAT after the putrescine treatment, respectively.
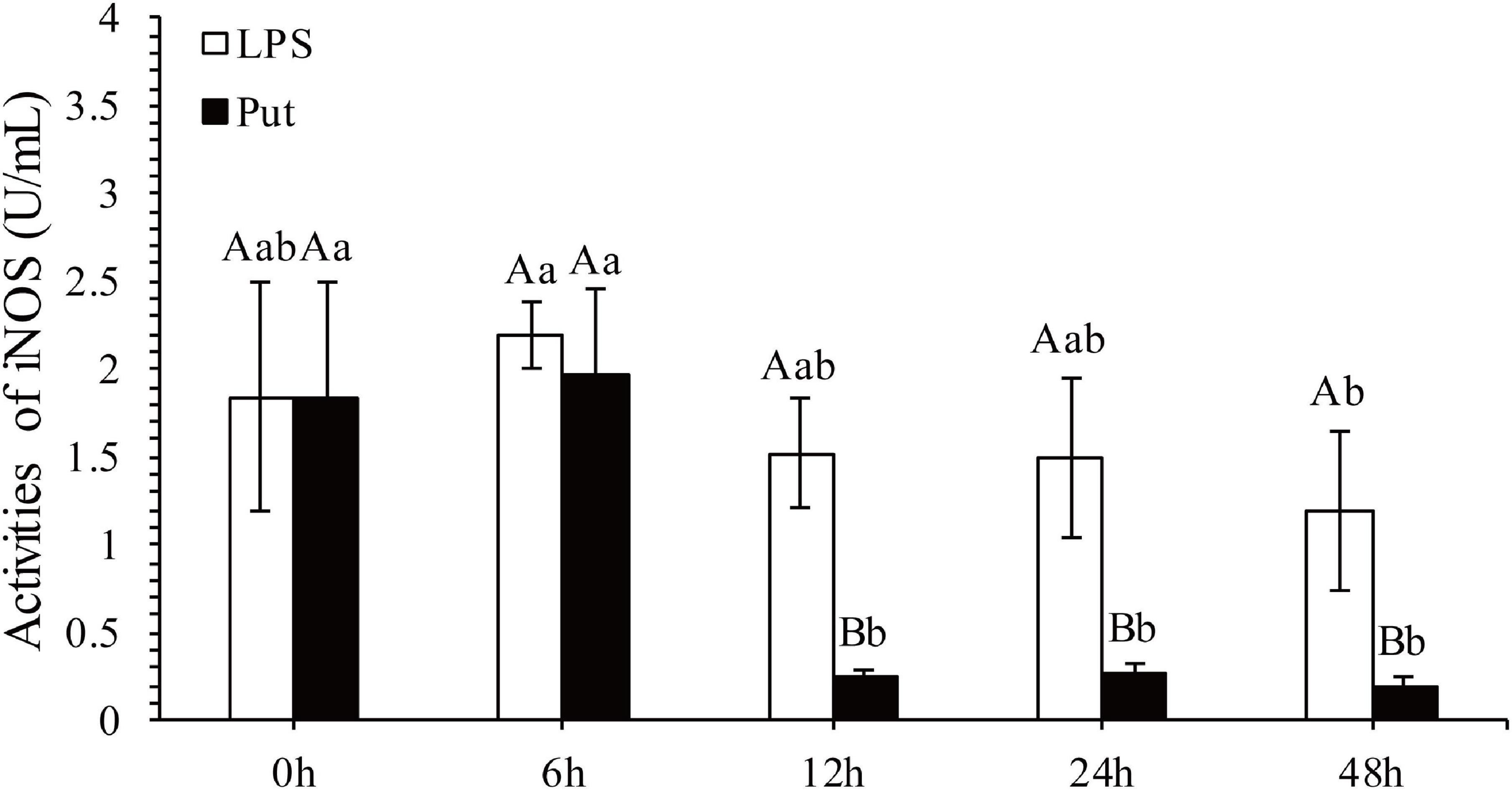
Figure 3. Detection of iNOS activity in serum after the putrescine treatment. Values are shown as mean ± SE (N = 5). iNOS, inducible nitric oxide synthase. Lowercase letters represent differences at different time points in the same groups, and capital letters represent differences in the different groups at same time points. Lipopolysaccharide (LPS) group, PBS_LPS; Put group, Put dihydrochloride_LPS.
The antibacterial activity of serum was determined using a microplate reader. As shown in Figure 4, P. aeruginosa and B. subtilis grew significantly better after co-incubation with the serum from the Put group at 48 h than that with the serum from the LPS group (P < 0.05, Figure 4).
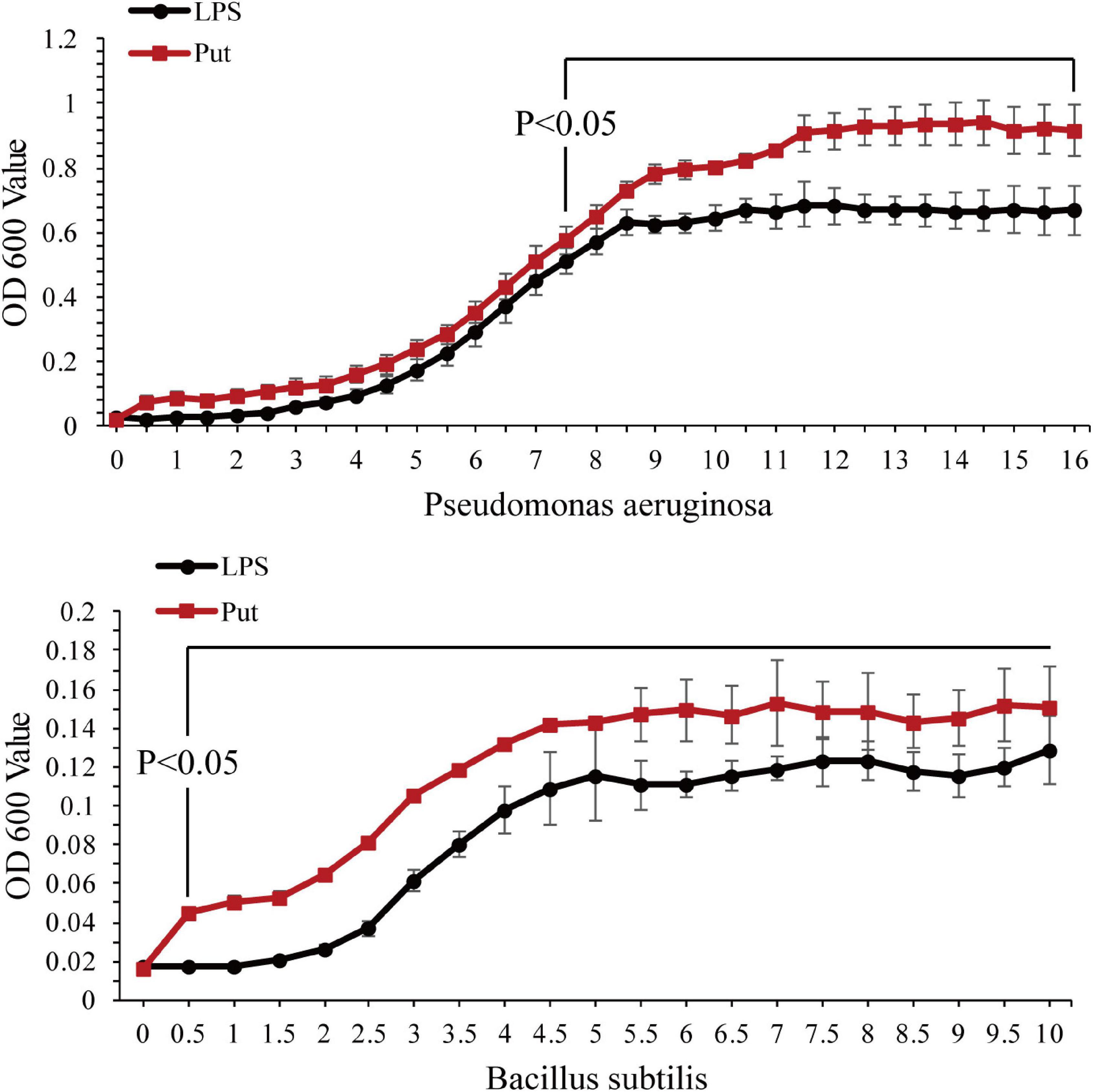
Figure 4. Antibacterial activity of serum after the putrescine treatment. Values are shown as mean ± SE (N = 5), and different superscripts indicate significant differences at P < 0.05. Lipopolysaccharide (LPS) group, PBS_LPS; Put group, Put dihydrochloride_LPS. Lowercase letters represent differences at different time points in the same groups, and capital letters represent differences in the different groups at same time points.
Changes in Expression of Immune-Related Genes
The NF-K B/JAK-STAT signaling pathway is a potential pathway to mediate the oxidative stress and inflammation. The expression levels of NF-K B, IKK, JAK, and STAT genes were determined via qRT-PCR (Figure 5). Compared with those in the LPS group, the expression levels of NF-κB and IKK from the NF-κB signaling pathway were significantly downregulated at 12 and 24 h by 3.16- to 8.23-fold in the Put group, respectively (P < 0.05, Figures 5A,B). The relative expressions of two genes from the JAK/STAT signaling pathway investigated herein were all significantly suppressed, declining by approximately 6.3- to 24.56-fold for JAK and STAT at 12 and 24 h, respectively (P < 0.05, Figures 5C,D).
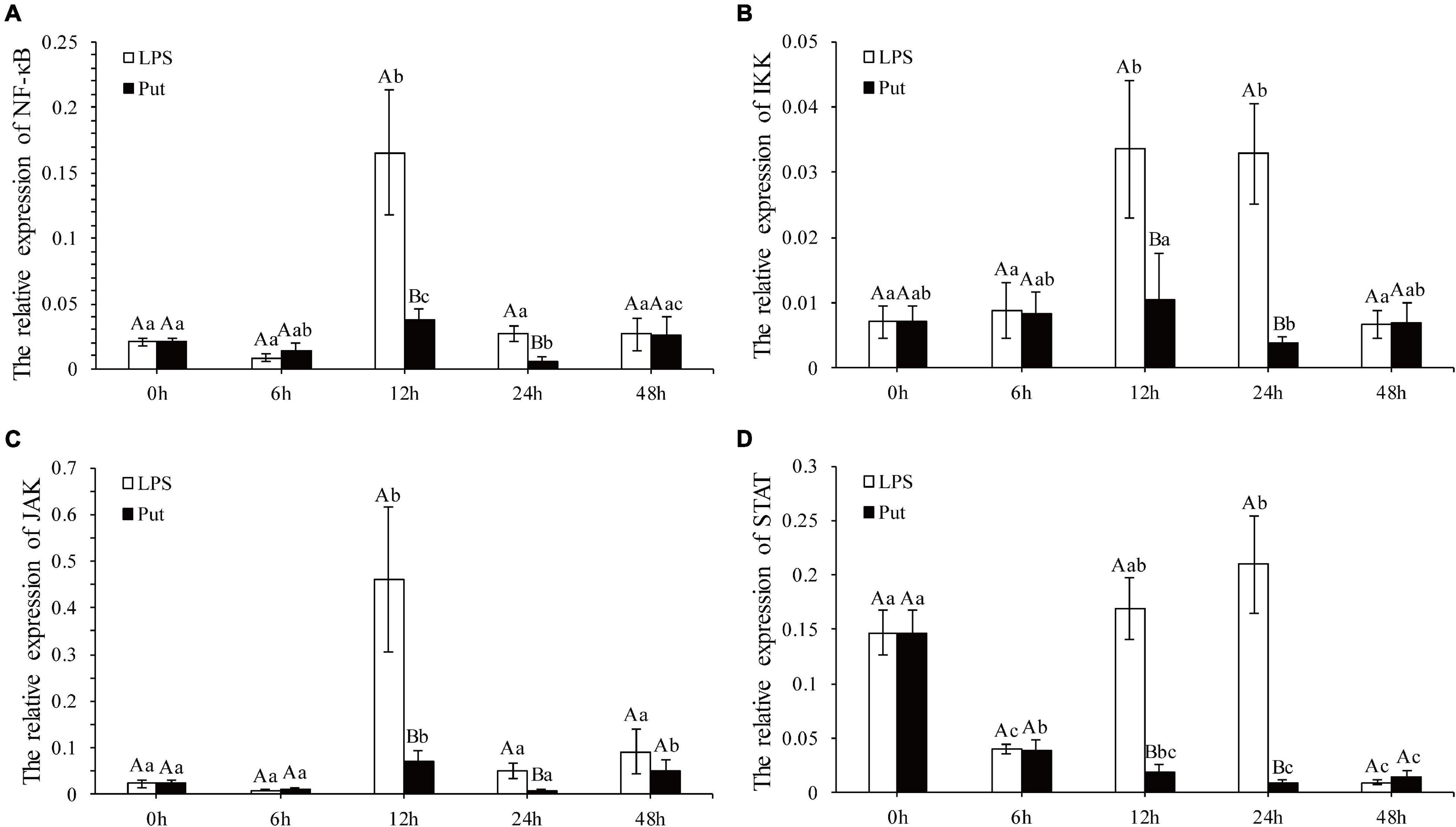
Figure 5. Expression of the nuclear factor kappa B (NF-κB), IκB kinase (IKK), Janus kinase (JAK), and signal transducer and activator of transcription proteins (STAT) genes after putrescine treatment. Values are shown as mean ± SE (N = 5). Lipopolysaccharide (LPS) group, PBS_LPS; Put group, Put dihydrochloride_LPS. Lowercase letters represent differences at different time points in the same groups, and capital letters represent differences in the different groups at same time points. (A–D) represent the expression of NF-κB/IKK/JAK/STAT after the putrescine treatment, respectively.
Detection of Hemocyte Autophagy Level
To achieve a better understanding of the underlying molecular mechanisms of Put-mediated autophagy inhibition, we explored the possible involvement of signaling pathways. Compared with the 0 h group, AMPK was significantly upregulated by 2.51- to 2.63-fold at 12 and 24 h by LPS stimulation, respectively (P < 0.05, Figure 6A). Moreover, mTOR was highly induced by 2.13- and 1.74-fold at 12 and 48 h, respectively (P < 0.05, Figure 6B). In the LPS group, Beclin-1 was highly induced by 4.63-fold at 48 h compared with 0 h (P < 0.05, Figure 6C). These results showed that autophagy was induced by LPS stimulation. Compared with that in the LPS group, the expression of AMPK was obviously decreased by 1.77- and 3.75-fold at 12 and 24 h, respectively, whereas that of mTOR increased by 1.75-fold at 48 h in the Put group (P < 0.05, Figures 6A,B). Compared with that in the LPS group, Beclin-1 expression decreased to 1.77- and 3.75-fold at 24 and 48 h, respectively, in the Put group (P < 0.05, Figure 6C).
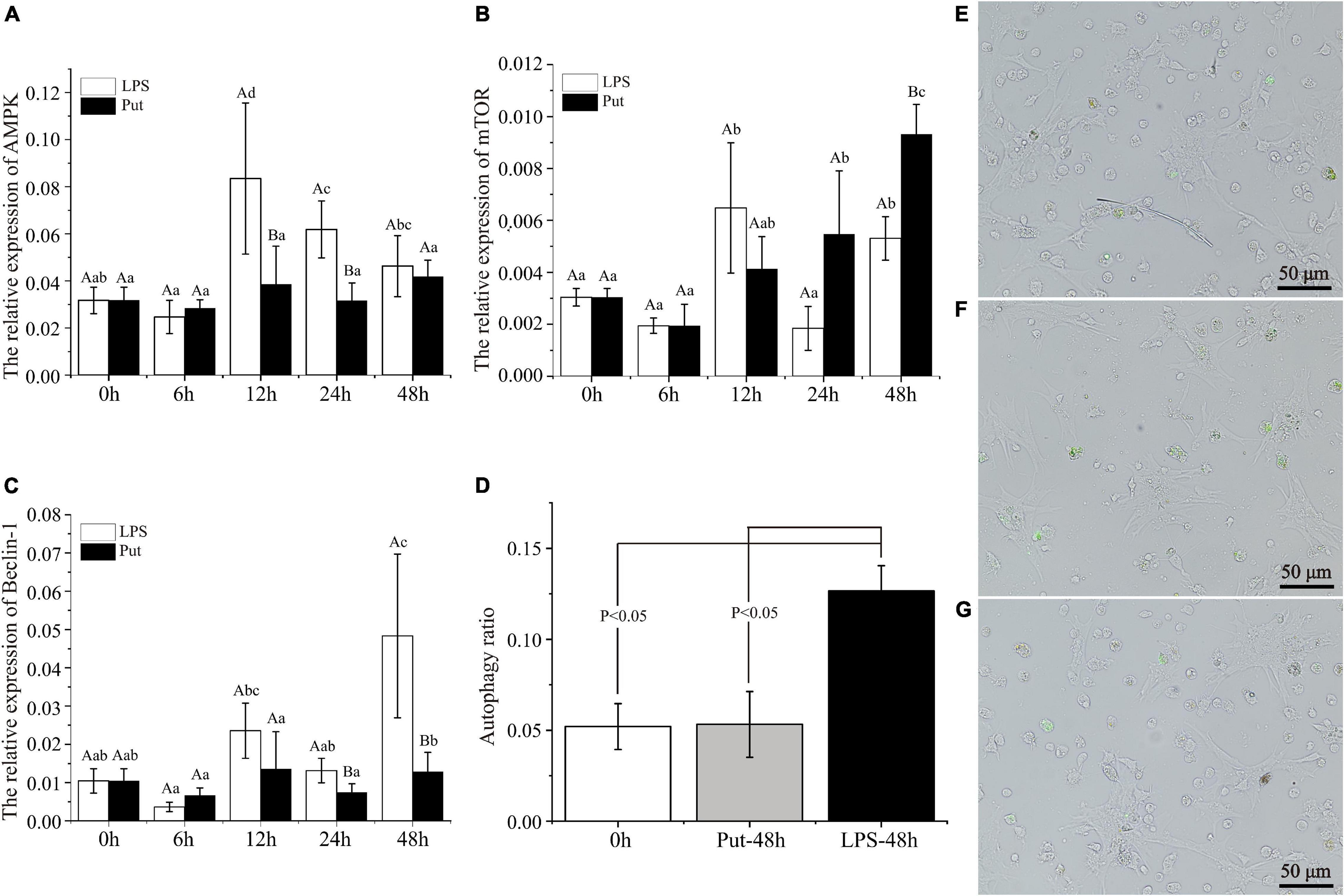
Figure 6. Putrescine treatment significantly inhibited hemocyte autophagy in pearl oysters. (A–C) mRNA expression of AMP-activated protein kinase (AMPK), mammalian target of rapamycin (mTOR), and Beclin-1 in hemocytes was analyzed. Values are shown as mean ± SE (N = 5). Lowercase letters represent differences at different time points in the same groups, and capital letters represent differences in the different groups at same time points. (D–G) Level of autophagy in hemocytes was detected via the monodansylcadaverine (MDC) method. Green fluorescence represents a positive signal. Lipopolysaccharide (LPS) group, PBS_LPS; Put group, Put dihydrochloride_LPS.
Subsequently, we adopted the MDC method to detect the autophagy level of pearl oyster hemocytes (Figures 6D–G). In the LPS group, the hemocyte autophagy level increased from 5.2 to 12.7% compared with that in the 0 h group (P < 0.05). Compared with that in the LPS group, the hemocyte autophagy level decreased from 12.7 to 5.3% at 48 h in the Put group (P < 0.05). Therefore, the Put pretreatment inhibited hemocytes autophagy via the AMPK-mTOR-Beclin-1 pathway upon LPS stimulation.
Discussion
Polyamines are a class of ubiquitous positively charged biogenic amines (Bae et al., 2018). In vertebrates, polyamines are important antioxidants and anti-inflammatory agents, and they regulate the immune response (Guérin et al., 2001; Shoji et al., 2005; Rider et al., 2007; Toro-Funes et al., 2013; Liu G. et al., 2016). Polyamines are also widespread in bivalve mollusks, suggesting that they may also be an important immune regulatory molecule (Hamana et al., 1991; Kournoutou et al., 2014). However, the immunomodulatory function of polyamines in the immune response of bivalves remains unknown. In this study, Put accumulation occurred at 6 and 24 h after LPS stimulation in pearl oysters. In eukaryotes, changes in the polyamine levels occur under various stresses, such as oxidative stress, pathogen infection, tissue damage, and drought/temperature stress (Wickström, 1991; Cipolla et al., 1993; Kournoutou et al., 2014; Adamipour et al., 2020; Tsaniklidis et al., 2021). In mussels, an increase in Put concentration under Cd+ stress may play an important role in the oxidative stress caused by this cation (Kournoutou et al., 2014). Polyamine accumulation and extensive changes in its levels under stress conditions indicate that they play a role in adaptive responses to various environmental stresses. Therefore, the accumulation of Put after LPS stimulation in pearl oysters indicated that it may play an important role in LPS-induced immune response.
To explore further the function of Put in LPS stress, we used this polyamine to treat pearl oysters before LPS stimulation. Owing to their lack of adaptive immunity, pearl oysters mainly rely on hemocytes and humoral immunity to resist pathogenic invasion (Pipe and Coles, 1995; Antunes et al., 2014; Song et al., 2015). LYS, ACP, and ALP are important antibacterial molecules in the humoral immunity of bivalves. In this study, the activities of ACP and ALP in pearl oyster were considerably inhibited in the Put group, which were consistent with those reported in mammals (Jing et al., 2021). Bacterial infections can cause oxidative stress. SOD, CAT, and GSH-Px are the main parameters of oxidative stress (Bilbao et al., 2009; Kolaiti et al., 2009; Venier et al., 2011). The increased activity of the antioxidant-related enzyme observed in our research suggested that oxidative stress weakened when treated with Put. In mammals, diets supplemented with Put substantially increase the activities of SOD, CAT, and GSH-Px (Liu et al., 2019a). Reactive nitrogen species, such as NO, are also free radicals that cause oxidative stress. iNOS is a critical enzyme that catalyzes the biosynthesis of NO. Our results showed that the iNOS activity was effectively inhibited after the Put treatment, similar to that observed in vertebrates (Choi and Park, 2012; Liu et al., 2019b). These results indicate that Put can effectively attenuate the oxidative stress response caused by LPS stimulation. Furthermore, the expression levels of NF-κB, IKK, JAK, and STAT genes were notably downregulated in hemocytes to be pretreated with Put before LPS stimulation. Previous studies have shown that inhibiting the NF-κB/JAK-STAT signaling pathway can remarkably reduce the oxidative stress and inflammation response (Choi and Park, 2012; Zhao et al., 2017; Liu et al., 2019b; Hu et al., 2020). Therefore, we speculated that Put may inhibit the LPS-stimulated oxidative stress by blocking the NF-κB and the JAK/STAT signaling pathways in the hemocytes of pearl oysters.
Autophagy is one of the main sensors of the oxidative stress signal (Lee et al., 2012). To respond to bacterial infections and prevent damage caused by oxidative stress, cells activate their autophagy system (Nakagawa et al., 2004; Travassos et al., 2010; Lee et al., 2012). In this study, the hemocyte autophagy level of pearl oysters decreased after the Put treatment. In vertebrates, the inhibition of iNOS by drug inhibitors can reduce autophagy in cardiomyocytes (Yuan et al., 2009). Therefore, the inhibition of the iNOS activity by the Put treatment may be one of the reasons for the decrease in hemocyte autophagy. Numerous key genes and signaling pathways involved in autophagy have been identified, such as mTOR, AMPK, and Beclin-1 (He and Klionsky, 2009; Booth et al., 2018). Autophagy-related genes are regulated by mTOR (Rosenbluth and Pietenpol, 2009). Inhibition of mTOR activity induces autophagy (Floto et al., 2007). Beclin-1 is a crucial protein that initiates autophagy (Booth et al., 2018). AMPK is an energy sensor that mediates autophagy (Harhaji-Trajkovic et al., 2009). Furthermore, it has been revealed that polyamines regulate autophagy through the AMPK-mTOR pathway (Pichiah et al., 2011; Liu et al., 2019c; Chen et al., 2021). To better understand the molecular mechanism of autophagy regulated by Put, we explored the possible involvement of signaling pathways. Results showed that Put inhibited the expression of AMPK and Beclin genes and induced that of mTOR, suggesting that Put may inhibit LPS-induced hemocyte autophagy through the AMPK-mTOR-Beclin-1 pathway.
Conclusion
The Put levels were induced after LPS stimulation in pearl oysters. Put weakened the oxidative stress in pearl oysters. The NF-κB/JAK-STAT pathways regulated by Put may be the potential pathways that regulate oxidative stress. Put inhibited hemocyte autophagy through the AMPK-mTOR-Beclin-1 pathway. Pearl oyster is one of the most economically important species for cultivating seawater pearls. During artificial pearl breeding, the damage and pathogenic infection caused during transplantation will cause strong immune rejection and even the death of pearl oysters. Therefore, the function of Put as an immunomodulator in pearl cultivation will be explored in a follow-up study.
Data Availability Statement
The original contributions presented in the study are included in the article/supplementary material, further inquiries can be directed to the corresponding author.
Ethics Statement
The pearl oyster Pinctada fucata martensii is a lower invertebrate, and therefore, the study was not subject to ethical approval.
Author Contributions
YJ, XD, and ZZ: conceptualization. YC: writing—original draft preparation. SZ, XL, ZL, and JC: validation. XX and ZG: formal analysis. ZZ and YJ: writing—review and editing. All authors have read and agreed to the published version of the manuscript.
Funding
This research was funded by the National Natural Science Foundation of China (32002369 and 32102817), the Guangdong Basic and Applied Basic Research Foundation (2021A1515011199 and 2020A1515011096), the Department of Education of Guangdong Province (2021KCXTD026, 2021KTSCX041 and 2020ZDZX1045), and the China Agriculture Research System of MOF and MARA.
Conflict of Interest
The authors declare that the research was conducted in the absence of any commercial or financial relationships that could be construed as a potential conflict of interest.
Publisher’s Note
All claims expressed in this article are solely those of the authors and do not necessarily represent those of their affiliated organizations, or those of the publisher, the editors and the reviewers. Any product that may be evaluated in this article, or claim that may be made by its manufacturer, is not guaranteed or endorsed by the publisher.
References
Ackermann, D., and Janka, R. (1954). First observation of spermine in invertebrate Cionia intestinalis. Hoppe Seylers Z. Physiol. Chem. 296, 279–282.
Adamipour, N., Khosh-Khui, M., Salehi, H., Razi, H., and Moghadam, A. (2020). Role of genes and metabolites involved in polyamines synthesis pathways and nitric oxide synthase in stomatal closure on Rosa damascena Mill. under drought stress. Plant Physiol. Biochem. 148, 53–61. doi: 10.1016/j.plaphy.2019.12.033
Allam, B., and Espinosa, E. P. (2016). Bivalve immunity and response to infections: are we looking at the right place. Fish Shellfish Immunol. 53, 4–12. doi: 10.1016/j.fsi.2016.03.037
Antunes, F., Hinzmann, M., Lopes-Lima, M., Vaz-Pires, P., Ferreira, S., Domingues, B., et al. (2014). Antibacterial effects of Anodonta cygnea fluids on Escherichia coli and enterococci multi-drug-resistant strains: environmental implications. Toxicol. Environ. Chem. Rev. 96, 880–889. doi: 10.1080/02772248.2014.989853
Bae, D. H., Lane, D. J. R., Jansson, P. J., and Richardson, D. R. (2018). The old and new biochemistry of polyamines. Biochim. Biophys. Acta Gen. Subj. 1862, 2053–2068. doi: 10.1016/j.bbagen.2018.06.004
Balbi, T., Cortese, K., and Ciacci, C. (2018). Autophagic processes in Mytilus galloprovincialis hemocytes: effects of Vibrio tapetis. Fish Shellfish Immunol. 73, 66–74. doi: 10.1016/j.fsi.2017.12.003
Bilbao, E., Cajaraville, M. P., and Cancio, I. (2009). Cloning and expression pattern of peroxisomal β-oxidation genes palmitoyl-CoA oxidase, multifunctional protein and 3-ketoacyl-CoA thiolase in mussel Mytilus galloprovincialis and thicklip grey mullet Chelon labrosus. Gene 443, 132–142. doi: 10.1016/j.gene.2009.05.008
Booth, L., Roberts, J. L., Poklepovic, A., Kirkwood, J., Sander, C., Avogadri-Connors, F., et al. (2018). The levels of mutant K-RAS and mutant N-RAS are rapidly reduced in a Beclin1 / ATG5 -dependent fashion by the irreversible ERBB1/2/4 inhibitor neratinib. Cancer Biol. Ther. 19, 132–137. doi: 10.1080/15384047.2017.1394556
Byrd, W. J., Jacobs, D. M., and Amoss, M. S. (1977). Synthetic polyamines added to cultures containing bovine sera reversibly inhibit in vitro parameters of immunity. Nature 267, 621–623. doi: 10.1038/267621a0
Canesi, L., Gallo, G., Gavioli, M., and Pruzzo, C. (2002). Bacteria-hemocyte interactions and phagocytosis in marine bivalves. Microsc. Res. Tech. 57, 469–476. doi: 10.1002/jemt.10100
Cao, Y. F., Jiao, Y., Zheng, C., Zhan, S. Z., Gu, Z. F., Xiong, X. W., et al. (2021). Transcriptome analysis provides insights into the function of the cholinergic system in Pearl oyster transplantation immunity. Aquaculture 15:737205. doi: 10.1016/j.aquaculture.2021.737205
Chen, K. Y., and Martynowicz, H. (1984). Lack of detectable polyamines in an extremely halophilic bacterium. Biochem. Biophys. Res. Commun. 124, 423–429. doi: 10.1016/0006-291X(84)91570-5
Chen, Q. S., Shen, A., Dai, J. W., Li, T. T., Huang, W. F., Shi, K., et al. (2021). IL37 overexpression inhibits autophagy and apoptosis induced by hepatic ischemia reperfusion injury via modulating AMPK/mTOR/ULLK1 signalling pathways. Life Sci. 276:119424. doi: 10.1016/j.lfs.2021.119424
Choi, Y. H., and Park, H. Y. (2012). Anti-inflammatory effects of spermidine in lipopolysaccharide-stimulated BV2 microglial cells. J. Biomed. Sci. 19:31. doi: 10.1186/1423-0127-19-31
Cipolla, B., Guillé, F., Moulinoux, J. P., Quemener, V., Staerman, F., Corbel, L., et al. (1993). Polyamines and prostatic carcinoma: clinical and therapeutic implications. Eur. Urol. 24, 124–31. doi: 10.1159/000474279
David, M., Munaswamy, V., Halappa, R., and Marigoudar, S. R. (2008). Impact of sodium cyanide on catalase activity in the freshwater exotic carp, Cyprinus carpio (Linnaeus). Pestic. Biochem. Physiol. 92, 15–18. doi: 10.1016/j.pestbp.2008.03.013
Dong, W., Liu, Z., Qiu, L., Wang, W., Song, X., Wang, X., et al. (2017). The modulation role of serotonin in Pacific oyster Crassostrea gigas in response to air exposure. Fish Shellfish Immunol. 62, 341–348. doi: 10.1016/j.fsi.2017.01.043
Durmu, N., and Kadioğlu, A. (2005). Spermine and putrescine enhance oxidative stress tolerance in maize leaves. Acta Physiol. Plant. 27, 515–522. doi: 10.1007/s11738-005-0057-8
Fang, T., Jia, G., Zhao, H., Chen, X., Tang, J., Wang, J., et al. (2016). Effects of spermine supplementation on the morphology, digestive enzyme activities, and antioxidant capacity of intestine in weaning rats. Anim. Nutr. 2, 370–375. doi: 10.1016/j.aninu.2016.09.002
Ferrante, A., Maxwell, G. M., Rencis, V. O., Allison, A. C., and Morgan, D. (1986). Inhibition of the respiratory burst of human neutrophils by the polyamine oxidase-polyamine system. Int. J. Immunopharmacol. 8, 411–417. doi: 10.1016/0192-0561(86)90125-6
Floto, R. A., Sarkar, S., Perlstein, E. O., Kampmann, B., and Rubinsztein, D. C. (2007). Small molecule enhancers of rapamycin-induced TOR inhibition promote autophagy, reduce toxicity in Huntington’s disease models and enhance killing of mycobacteria by macrophages. Autophagy 3, 620–622. doi: 10.4161/auto.4898
Guérin, P., El, M. S., and Ménézo, Y. (2001). Oxidative stress and protection against reactive oxygen species in the pre-implantation embryo and its surroundings. Hum. Reprod. Upd. 7, 175–189. doi: 10.1093/humupd/7.2.175
Ha, H. C., Sirisoma, N. S., Kuppusamy, P., Zweier, J. L., Woster, P. M., and Casero, R. A. (1998). The natural polyamine spermine functions directly as a free radical scavenger. Proc. Natl. Acad. Sci. U. S. A. 95, 11140–11145. doi: 10.1073/pnas.95.19.11140
Hamana, K., Hamana, H., and Shinozawa, T. (1995). Alterations in polyamine levels of nematode, earthworm, leech and planarian during regeneration, temperature and osmotic stresses. Comp. Biochem. Physiol. B Biochem. Mol. Biol. 111, 91–97. doi: 10.1016/0305-0491(94)00222-G
Hamana, K., Niitsu, M., Samejima, K., and Matsuzaki, S. (1991). Novel tetraamines, pentaamines and hexaamines in sea urchin, sea cucumber, sea squirt and bivalves. Comp. Biochem. Physiol. B Comp. Biochem. 100, 59–62. doi: 10.1016/0305-0491(91)90084-Q
Hanington, P. C., Forys, M. A., Dragoo, J. W., Zhang, S. M., Adema, C. M., and Loker, E. S. (2010). Role for a somatically diversified lectin in resistance of an invertebrate to parasite infection. Proc. Natl. Acad. Sci. U. S. A. 107, 21087–21092. doi: 10.1073/pnas.1011242107
Harhaji-Trajkovic, L., Vilimanovich, U., Kravic-Stevovic, T., Bumbasirevic, V., and Trajkovic, V. (2009). AMPK-mediated autophagy inhibits apoptosis in cisplatin-treated tumor cells. J. Cell Mol. Med. 13, 3644–3654. doi: 10.1111/j.1582-4934.2008.00663.x
He, C., and Klionsky, D. J. (2009). Regulation Mechanisms and Signaling Pathways of Autophagy. Annu. Rev. Genet. 43, 67–93. doi: 10.1146/annurev-genet-102808-114910
Hu, X., Ding, C., Ding, X., Fan, P., and Li, Y. (2020). Inhibition of myeloid differentiation protein 2 attenuates renal ischemia/reperfusion-induced oxidative stress and inflammation via suppressing TLR4/TRAF6/NF-kB pathway. Life Sci. 256:117864. doi: 10.1016/j.lfs.2020.117864
Jing, W., Yxb, C., Jl, C., Ming, Q., and Bie, T. (2021). Serum biochemical parameters and amino acids metabolism are altered in piglets by early-weaning and proline and putrescine supplementations. Anim. Nutr. 7, 334–345. doi: 10.1016/j.aninu.2020.11.007
Kolaiti, R. M., Lucas, J. M., and Kouyanou-Koutsoukou, S. (2009). Molecular cloning of the ribosomal P-proteins MgP1, MgP2, MgP0, and superoxide dismutase (SOD) in the mussel Mytilus galloprovincialis and analysis of MgP0 at stress conditions. Gene 430, 77–85. doi: 10.1016/j.gene.2008.10.024
Kournoutou, G. G., Pytharopoulou, S., Leotsinidis, M., and Kalpaxis, D. L. (2014). Changes of polyamine pattern in digestive glands of mussel Mytilus galloprovincialis under exposure to cadmium. Comp. Biochem. Physiol. C Toxicol. Pharmacol. 165, 1–8. doi: 10.1016/j.cbpc.2014.05.005
Lee, J., Giordano, S., and Zhang, J. (2012). Autophagy, mitochondria and oxidative stress: cross-talk and redox signalling. Biochem. J. 441, 523–540. doi: 10.1042/BJ20111451
Li, G., Ding, H., Yu, X., Meng, Y., Li, J., Guo, Q., et al. (2020). Spermidine suppresses inflammatory DC function by activating the FOXO3 pathway and counteracts autoimmunity. iScience 23:100807. doi: 10.1016/j.isci.2019.100807
Li, M., Wang, L., Qiu, L., Wang, W., Xin, L., Xu, J., et al. (2016). A glutamic acid decarboxylase (CgGAD) highly expressed in hemocytes of Pacific oyster Crassostrea gigas. Dev. Comp. Immunol. 63, 56–65. doi: 10.1016/j.dci.2016.05.010
Liu, G., Liang, X., Wei, C., Fang, T., Gang, J., Chen, X., et al. (2016). Changes in the metabolome of rats after exposure to arginine and N-carbamylglutamate in combination with diquat, a compound that causes oxidative stress, assessed by 1H NMR spectroscopy. Food Funct. 7, 964–974. doi: 10.1039/C5FO01486G
Liu, Z., Zhou, Z., Wang, L., Dong, W., Qiu, L., and Song, L. (2016). The cholinergic immune regulation mediated by a novel muscarinic acetylcholine receptor through TNF pathway in oyster Crassostrea gigas. Dev. Comp. Immunol. 65, 139–148. doi: 10.1016/j.dci.2016.07.003
Liu, H., Dong, J., Song, S., Zhao, Y., and Yang, J. (2019c). Spermidine ameliorates liver ischaemia-reperfusion injury through the regulation of autophagy by the AMPK-mTOR-ULK1 signalling pathway. Biochem. Biophys. Res. Commun. 519, 227–233. doi: 10.1016/j.bbrc.2019.08.162
Liu, G., Mo, W., Xu, X., Wu, X., Jia, G., Zhao, H., et al. (2019a). Effects of putrescine on gene expression in relation to physical barriers and antioxidant capacity in organs of weaning piglets. RSC Adv. 9, 19584–19595. doi: 10.1039/C9RA02674F
Liu, G., Zheng, J., Wu, X., Xu, X., Jia, G., Zhao, H., et al. (2019b). Putrescine enhances intestinal immune function and regulates intestinal bacteria in weaning piglets. Food Funct. 10, 4134–4142. doi: 10.1039/C9FO00842J
Liu, S., Wang, W., Liu, Y., Cao, W., Yuan, P., Li, J., et al. (2020). Protein kinase-like ER kinase (PERK) regulates autophagy of hemocytes in antiviral immunity of Pacific oyster Crassostrea gigas. Fish Shellfish Immunol. Rep. 1:100002. doi: 10.1016/j.fsirep.2020.100002
Løvaas, E., and Carlin, G. (1991). Spermine: an anti-oxidant and anti-inflammatory agent. Free Radic. Biol. Med. 11, 455–461. doi: 10.1016/0891-5849(91)90061-7
Mitta, G., Vandenbulcke, F., Hubert, F., Salzet, M., and Roch, P. (2000). Involvement of mytilins in mussel antimicrobial defense. J. Biol. Chem. 275, 12954–12962. doi: 10.1074/jbc.275.17.12954
Moreau, P., Moreau, K., Segarra, A., Tourbiez, D., Travers, M. A., Rubinsztein, D. C., et al. (2015). Autophagy plays an important role in protecting Pacific oysters from OsHV-1 and Vibrio aestuarianus infections. Autophagy 11, 516–526. doi: 10.1080/15548627.2015.1017188
Nakagawa, I., Amano, A., Mizushima, N., Yamamoto, A., Yamaguchi, H., Kamimoto, T., et al. (2004). Autophagy defends cells against invading group a streptococcus. Science 306, 1037–1040. doi: 10.1126/science.1103966
Pichiah, P., Suriyakalaa, U., Kamalakkannan, S., Kokilavani, P., and Achiraman, S. (2011). Spermidine may decrease ER stress in pancreatic beta cells and may reduce apoptosis via activating AMPK dependent autophagy pathway. Med. Hypotheses 77, 677–679. doi: 10.1016/j.mehy.2011.07.014
Pipe, R. K., and Coles, J. A. (1995). Environmental contaminants influencing immunefunction in marine bivalve molluscs. Fish Shellfish Immunol. 5, 581–595. doi: 10.1016/S1050-4648(95)80043-3
Pirinen, E., Kuulasmaa, T., Pietilä, M., Heikkinen, S., Tusa, M., Itkonen, P., et al. (2007). Enhanced polyamine catabolism alters homeostatic control of white adipose tissue mass, energy expenditure, and glucose metabolism. Mol. Cell. Biol. 27, 4953–4967. doi: 10.1128/MCB.02034-06
Quemener, V., Blanchard, Y., Chamaillard, L., Havouis, R., and Moulinoux, J. P. (1994). Polyamine deprivation: a new tool in cancer treatment. Anticancer Res. 14, 443–448. doi: 10.1007/BF02303562
Reyes-Becerril, M., Ascencio-Valle, F., Tovar-Ramírez, D., Meseguer, J., and Esteban, M. (2011). Effects of polyamines on cellular innate immune response and the expression of immune-relevant genes in gilthead seabream leucocytes. Fish Shellfish Immunol. 30, 248–254. doi: 10.1016/j.fsi.2010.10.011
Rider, J. E., Hacker, A., Mackintosh, C. A., Pegg, A. E., Woster, P. M., and Casero, R. Jr. (2007). Spermine and spermidine mediate protection against oxidative damage caused by hydrogen peroxide. Amino Acids 33, 231–240. doi: 10.1007/s00726-007-0513-4
Rosenbluth, J. M., and Pietenpol, J. A. (2009). mTOR regulates autophagy-associated genes downstream of p73. Autophagy 5, 114–116. doi: 10.4161/auto.5.1.7294
Seo, J. K., Stephenson, J., Crawford, J. M., Stone, K. L., and Noga, E. J. (2010). American Oyster, Crassostrea virginica, expresses a potent antibacterial histone H2B protein. Mar. Biotechnol. 12, 543–551. doi: 10.1007/s10126-009-9240-z
Shoji, H., Oguchi, S., Fujinaga, S., Shinohara, K., Kaneko, K., Shimizu, T., et al. (2005). Effects of human milk and spermine on hydrogen peroxide-induced oxidative damage in IEC-6 cells. J. Pediatr. Gastroenterol. Nutr. 41, 460–465. doi: 10.1093/carcin/bgp122
Song, L., Wang, L., Zhang, H., and Wang, M. (2015). The immune system and its modulation mechanism in scallop. Fish Shellfish Immunol. 46, 65–78. doi: 10.1016/j.fsi.2015.03.013
Tiscar, P. G., and Mosca, F. (2004). Defense mechanisms in farmed marine molluscs. Vet. Res. Commun. 28, 57–62. doi: 10.1023/B:VERC.0000045379.78547.23
Toro-Funes, N., Bosch-Fuste, J., Veciana-Nogues, M. T., Izquierdo-Pulido, M., and Vidal-Carou, M. C. (2013). In vitro antioxidant activity of dietary polyamines. Food Res. Int. 51, 141–147. doi: 10.1016/j.foodres.2012.11.036
Travassos, L. H., Carneiro, L. A., Ramjeet, M., Hussey, S., Kim, Y. G., Magalhães, J. G., et al. (2010). Nod1 and Nod2 direct autophagy by recruiting ATG16L1 to the plasma membrane at the site of bacterial entry. Nat. Immunol. 11, 55–62. doi: 10.4161/auto.6.3.11305
Tsaniklidis, G., Charova, S. N., Fanourakis, D., Tsafouros, A., Nikoloudakis, N., Goumenaki, E., et al. (2021). The role of temperature in mediating postharvest polyamine homeostasis in tomato fruit. Postharvest Biol. Technol. 179:111586. doi: 10.1016/j.postharvbio.2021.111586
Venier, P., Varotto, L., Rosani, U., Millino, C., and Pallavicini, A. (2011). Insights into the innate immunity of the Mediterranean mussel Mytilus galloprovincialis. BMC Genomics 12:69. doi: 10.1186/1471-2164-12-69
Wickström, K. (1991). Polyamines in rabbit aqueous humor after surgical trauma to the eye. Curr. Eye Res. 10, 463–469. doi: 10.3109/02713689109001753
Yerra, A., Challa, S., Valluri, S. V., and Mamillapalli, A. (2016). Spermidine alleviates oxidative stress in silk glands of Bombyx mori. J. Asia Pacific Entomol. 19, 1197–1202. doi: 10.1016/j.aspen.2016.10.014
Yuan, H., Perry, C. N., Huang, C., Iwai-Kanai, E., Carreira, R. S., Glembotski, C. C., et al. (2009). LPS-induced autophagy is mediated by oxidative signaling in cardiomyocytes and is associated with cytoprotection. Am. J. Physiol. Heart Circ. Physiol. 296, H470–H479. doi: 10.1152/ajpheart.01051.2008
Yuan, Q., Ray, R. M., Viar, M. J., and Johnson, L. R. (2001). Polyamine regulation of ornithine decarboxylase and its antizyme in intestinal epithelial cells. Am. J. Physiol. Gastrointest. Liver Physiol. 280, G130–G138. doi: 10.1152/ajpgi.2001.280.1.G130
Zhao, L., Wu, D., Sang, M., Xu, Y., Liu, Z., and Wu, Q. (2017). Stachydrine ameliorates isoproterenol-induced cardiac hypertrophy and fibrosis by suppressing inflammation and oxidative stress through inhibiting NF-κB and JAK/STAT signaling pathways in rats. Int. Immunopharmacol. 48, 102–109. doi: 10.1016/j.intimp.2017.05.002
Keywords: polyamines, putrescine, immune regulation, oxidative stress, mollusks, pearl oysters
Citation: Cao Y, Jiao Y, Zhan S, Liang X, Li Z, Chen J, Xiong X, Gu Z, Du X and Zheng Z (2021) Polyamine Putrescine Regulates Oxidative Stress and Autophagy of Hemocytes Induced by Lipopolysaccharides in Pearl Oyster Pinctada fucata martensii. Front. Physiol. 12:781324. doi: 10.3389/fphys.2021.781324
Received: 22 September 2021; Accepted: 11 November 2021;
Published: 10 December 2021.
Edited by:
Xiaotong Wang, Ludong University, ChinaCopyright © 2021 Cao, Jiao, Zhan, Liang, Li, Chen, Xiong, Gu, Du and Zheng. This is an open-access article distributed under the terms of the Creative Commons Attribution License (CC BY). The use, distribution or reproduction in other forums is permitted, provided the original author(s) and the copyright owner(s) are credited and that the original publication in this journal is cited, in accordance with accepted academic practice. No use, distribution or reproduction is permitted which does not comply with these terms.
*Correspondence: Zhe Zheng, emhlbmd6aGVAZ2RvdS5lZHUuY24=
†These authors have contributed equally to this work