- Department of Bioenergetics, Faculty of Biology, Institute of Molecular Biology and Biotechnology, Adam Mickiewicz University, Poznań, Poland
Voltage-dependent anion-selective channel (VDAC) allows the exchange of small metabolites and inorganic ions across the mitochondrial outer membrane. It is involved in complex interactions that regulate mitochondrial and cellular functioning. Many organisms have several VDAC paralogs that play distinct but poorly understood roles in the life and death of cells. It is assumed that such a large diversity of VDAC-encoding genes might cause physiological plasticity to cope with abiotic and biotic stresses known to impact mitochondrial function. Moreover, cysteine residues in mammalian VDAC paralogs may contribute to the reduction–oxidation (redox) sensor function based on disulfide bond formation and elimination, resulting in redox-sensitive VDAC (rsVDAC). Therefore, we analyzed whether rsVDAC is possible when only one VDAC variant is present in mitochondria and whether all VDAC paralogs present in mitochondria could be rsVDAC, using representatives of currently available VDAC amino acid sequences. The obtained results indicate that rsVDAC can occur when only one VDAC variant is present in mitochondria; however, the possibility of all VDAC paralogs in mitochondria being rsVDAC is very low. Moreover, the presence of rsVDAC may correlate with habitat conditions as rsVDAC appears to be prevalent in parasites. Thus, the channel may mediate detection and adaptation to environmental conditions.
Introduction
Environmental stress of varying severity always exists in any organism’s habitat. Given the diversity of organisms and their habitats, the diversity may be affected by different environmental conditions. The three main types of environments can be broadly distinguished as terrestrial, aquatic, and semi-terrestrial (semi-aquatic). The habitats available within these environments can be characterized by a set of parameters, including light, temperature, pH, atmospheric or hydrostatic pressure, salinity, and oxygen pressure, as well as individual combinations of these parameters (Edery, 2000; Rothschild and Mancinelli, 2001). An environmental factor is determined as stressful based on the organism’s tolerance against it. Abiotic factors such as temperature, radiation, oxygen pressure, and changes in water availability can exert stress via disturbances in gas exchange, water management, and nutrient production (Lesser, 2006; Lushchak, 2011; Sokolova et al., 2012; Wang and Komatsu, 2018). Biotic factors, including predators, competitors, and parasites (e.g., Forsman and Martin, 2009) can also be stressful to organisms. In the case of parasites, both the internal and external environments of a parasitic host dictate the outcome of their infection, resistance, susceptibility, and transmission (Prado et al., 2021). Moreover, the internal conditions of parasitic hosts may constitute a greater constraint upon survival than external conditions (Tinsley, 1999).
Unicellular organisms are exposed to stress conditions through their whole surface. Multicellular organisms regulate their response to stress in a more complex manner, but with cell response as the basis of all response types. Many different organisms are known for their resistance strategies to environmental stress, which indicate efficient cellular anti-stress mechanisms. These mechanisms may protect against intracellular oxidative stress imposed by environmental stress conditions, including increased temperature, not optimal oxygen pressure or high salinity (Laksanalamai and Robb, 2004; Wang et al., 2006; Bagnyukova et al., 2007; Sinha et al., 2013). The state of oxidative stress threatens the functioning of whole cells, especially that of the mitochondria. Reactive oxygen species (ROS), formed mainly during cellular respiration performed by mitochondria, are important signaling molecules but also markers of oxidative stress. Their excess is dangerous due to the direct impact of ROS or ROS-mediated regulation on cell structure and function (e.g., Chung, 2017).
The most common anti-oxidative stress cellular strategy involves maintaining ROS homeostasis. Available data indicate that the homeostasis may be provided by voltage-dependent anion-selective channel (VDAC) (e.g., Shoshan-Barmatz et al., 2010, 2020; De Pinto, 2021; Rostovtseva et al., 2021). This relatively simple, monomeric β-barrel channel at the interface between mitochondria and the cytosol is described as a highly conserved protein of the mitochondrial outer membrane, present in nearly all eukaryotic species examined to date (Colombini, 2012). VDAC performs and regulates inorganic ion and metabolite transport between mitochondria and the cytoplasm under both physiological and pathological conditions (Kroemer et al., 2007; Li et al., 2013; Rostovtseva et al., 2021). The contribution of VDAC in ROS homeostasis (Reina et al., 2010; Sanyal et al., 2020) is based on its transport of superoxide anion (Han et al., 2003), its important role in ROS production (Fang and Maldonado, 2018; Heslop et al., 2020), and its role in regulating the amount and activity of anti-oxidative enzymes (Gałgańska et al., 2008).
Voltage-dependent anion-selective channel is the most abundant protein in the mitochondrial outer membrane and has been relatively well studied since its discovery in 1976 (Schein et al., 1976). As summarized by Rostovtseva et al. (2021) in their comprehensive review, besides being a strictly regulated transport pathway between the mitochondrion and cytosol, VDAC also interacts with a numerous of mitochondrial and cytosolic proteins, which makes the channel a key element in and regulator of communications between mitochondria and cytosol. Moreover, VDAC forms homo- and hetero-complexes with additional functional subunits (e.g., Shoshan-Barmatz et al., 2010, 2018). However, the identification of VDAC paralogs indicates the presence of VDAC variants with slight amino acid differences that undoubtedly perform specified but not yet fully explained functions (De Pinto, 2021).
Three VDAC paralogs were identified in vertebrate mitochondria (Sampson et al., 1997; Messina et al., 2012). The presence of VDAC paralogs was also reported in other multicellular organisms such as invertebrates (Sardiello et al., 2003) and plants (Elkeles et al., 1997; Al Bitar et al., 2003; Wandrey et al., 2004; Lee et al., 2009; Wojtkowska et al., 2012; Hemono et al., 2020; Sanyal et al., 2020), as well as in unicellular organisms such as yeasts Saccharomyces cerevisiae (Blachly-Dyson et al., 1997; Di Rosa et al., 2021) and Candida glabrata (Wojtkowska et al., 2012) and protists Trypanosoma brucei (Flinner et al., 2012) and Cyanophora paradoxa (Wojtkowska et al., 2012). Thus, it is assumed that VDAC-encoding genes were duplicated independently in different lineages of eukaryotic organisms, several times during their evolution (Sampson et al., 1996; Saccone et al., 2003; Young et al., 2007). The resulting VDAC-encoding gene redundancy might indicate a need to innovate their existing function and a tendency to duplicate genetic material, as observed in invertebrates, plants, and vertebrates (Saccone et al., 2003). Thus, the following question arises: could the trigger factor be oxidative stress imposed by habitat conditions?
Identifying the function of individual VDAC paralogs is currently one of the main topics concerning VDAC research (De Pinto, 2021). One of the most important aspects of the research is the study of post-translational modification of VDAC paralogs by focusing mainly on cysteine residues. The significance of the number of cysteine residues, as well as their localization and oxidation state in individual mammalian VDAC paralogs, have been indicated by the De Pinto research group (Messina et al., 2012; De Pinto et al., 2016; Reina et al., 2020) and verified by other researchers (Okazaki et al., 2015; Karachitos et al., 2016; Queralt-Martín et al., 2020). The presence of ROS could result in the variable oxidation of cysteine residues exposed to the VDAC interior (including the flexible N-terminal region) or present in the connection loops between 19 β-strands forming the channel (Reina et al., 2010; Okazaki et al., 2015). As oxidative modifications of cysteine residues in VDAC proteins are not detected in other proteins of the mitochondrial outer membrane (Reina et al., 2020), it has been speculated that such modifications could have a regulatory function (including channel gating and conductance) as well as mitochondrial ROS buffering capacity (Okazaki et al., 2015; De Pinto, 2021).
Among the modifications occurring in cysteine residues, disulfide bond formation was shown to affect gating properties and conductance of VDAC (Okazaki et al., 2015; De Pinto et al., 2016; Reina et al., 2020). Accordingly, available data on human VDAC paralogs indicate that cysteine residues in the flexible N-terminal region are crucial for this bond formation. The same probably applies to Drosophila melanogaster VDAC paralogs (Komarov et al., 2004). Therefore, the presence of cysteine residues in the N-terminus could be a prerequisite for VDAC to serve as a sensor of the reduction–oxidation (redox) state (Queralt-Martín et al., 2020). Thus, the following questions arise: (1) is the sensor function possible when only one VDAC variant is present in mitochondria, and (2) is the sensor function possible for all VDAC paralogs present in mitochondria? To answer these questions, we used currently available VDAC amino acid sequences to analyze the number of cysteine residues and their location, with particular emphasis on the N-terminus. Next, we examined the relationship between the sequences and the studied species’ ecology. The obtained results suggest that the presence of redox-sensitive VDAC (rsVDAC) proteins may be important for adaptation to environmental conditions.
Materials and Methods
Construction of the Database
UniProt (The UniProt Consortium, 2021) was used to compile a list of VDAC paralogs of non-vertebrate and non-plant organisms (Supplementary File 1). Next, the database was enriched with records of paralogs from previously obtained organisms (using sequences of 250–380 amino acids) and with organisms from systematic groups missing in the list. Sequences containing “Fragment” annotations (except of Hydra vulgaris) and those whose amino acid sequences did not start with methionine were removed. The list was supplemented with the VDAC sequence predicted for the tardigrade Milnesium tardigradum, based on data kindly provided by Felix Bemm (Max Planck Institute for Developmental Biology, Tübingen, Germany). Finally, sequences were blasted using Blastp to determine whether different records for a given organism were paralogs or products of the same genes (Altschul et al., 1997).
Prediction of the Voltage-Dependent Anion-Selective Channel Structure
The SSPRo (Pollastri et al., 2002; Cheng et al., 2005) and DIpro (Baldi et al., 2004; Cheng et al., 2006) servers available at the Scratch Protein Predictor1 were used to estimate the secondary structure of the available VDAC sequences and predict the presence of disulfide bonds, respectively. 3D structures were predicted by applying the Iterative Threading ASSEmbly Refinement (I-TASSER) method (Roy et al., 2010; Yang et al., 2015; Yang and Zhang, 2015). The predicted solutions were visualized using YASARA.2
Results and Discussion
To perform all analysis, a database (Supplementary File 1) was built using a collection of species categorized by various parameters, such as a type of environment (terrestrial or aquatic), organism complexity (simple or complex), and the number of VDAC paralogs, including the number (poor or rich) and location (N-terminus free) of cysteine residues (Figure 1). We assumed that a complex organism contained multiple organ systems with different functions (Novikoff, 1945). As shown in Figure 1A, most of the studied organisms categorized by complexity and inhabited environment possess only one VDAC gene. This group mostly includes terrestrial and complex organisms. Due to the lack of VDAC paralogs, various aspects of mitochondrial functioning in these organisms depend on the properties of only one VDAC variant. Therefore, we checked the number of cysteine residues and their location in the secondary structure of VDAC proteins of simple and complex organisms (Figure 1B). We applied the following parameters to perform the operations: one VDAC or at least one VDAC paralog (if present) contained fewer than two cysteine residues in the primary structure (Cys-poor) and one VDAC or all VDAC paralogs (if present) had more than one cysteine residue in the primary structure (Cys-rich). Moreover, the presence of cysteine residue(s) in the N-terminal region upstream of the β1 strand was applied as an additional parameter, as the residue is obligatory for the possibility of disulfide bond formation in human VDAC paralogs (Okazaki et al., 2015; De Pinto et al., 2016; Reina et al., 2020). The results allowed us to distinguish organisms with VDAC(s), in which disulfide bonds could potentially form. Moreover, the possibility of this type of VDAC occurring was more frequent in complex organisms (42%) than in simple organisms (17%).
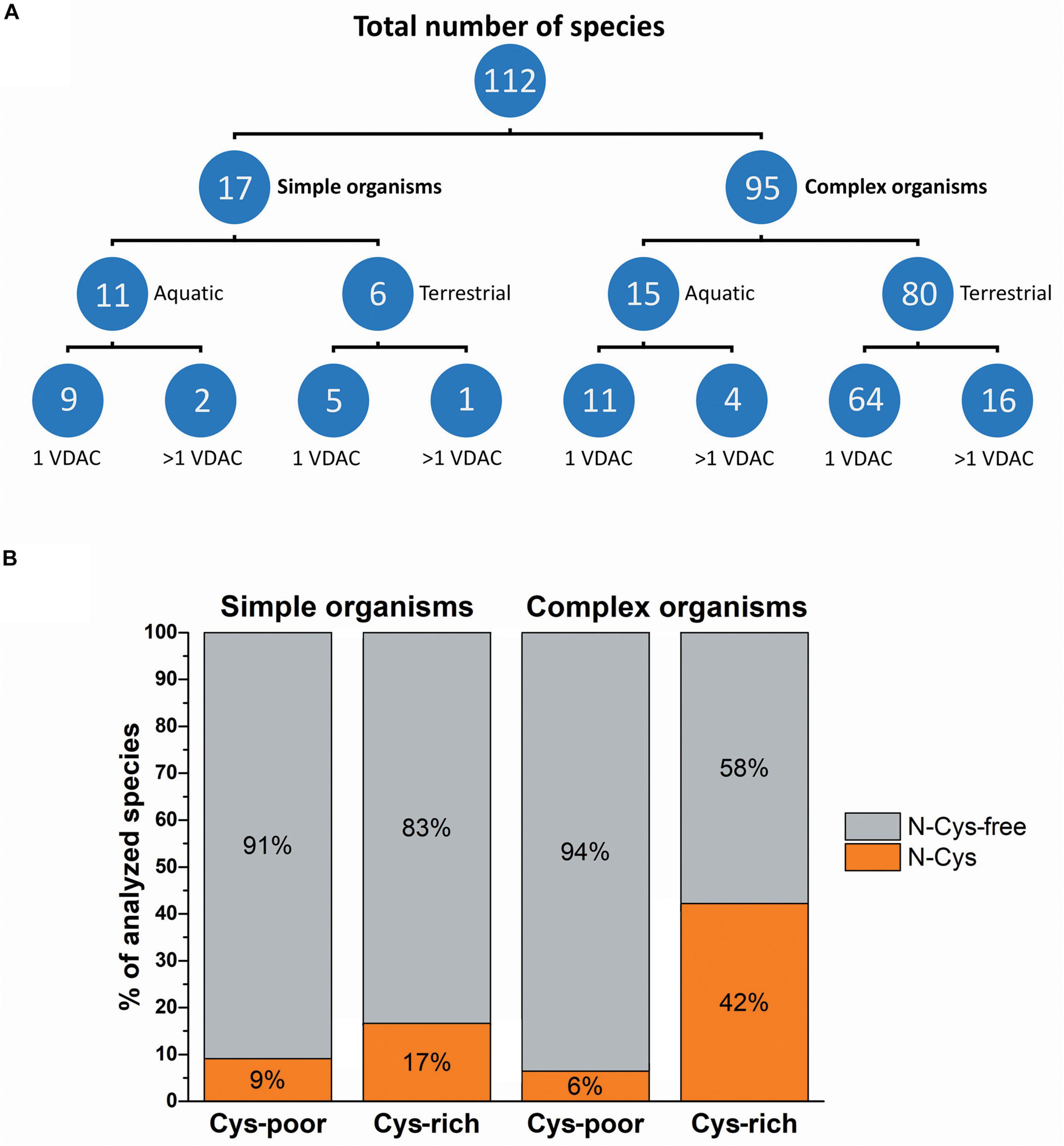
Figure 1. The complexity of organisms and their inhabited environment in relation to the number of VDAC variants and their cysteine residue content. (A) The number of organisms classified in terms of complexity, inhabited environment, and the number of genes encoding VDAC proteins. (B) Classification of organisms based on the number and localization of cysteine residues in VDAC proteins. Cys-poor: a set of organisms in which only one VDAC variant or at least one VDAC paralog (if present) contains fewer than two cysteine residues in the primary structure; Cys-rich: a set of organisms in which only one VDAC variant or all VDAC paralogs (if present) have more than one cysteine residue in the primary structure; N-Cys: a set of organisms in which only one VDAC variant or all VDAC paralogs (if present) have at least one N-terminal cysteine residue; N-Cys-free: a set of organisms that have at least one VDAC variant with no N-terminal cysteine residue.
Next, we analyzed the interactions between the number of cysteine residues, their location in the secondary structure, and the number of VDAC paralogs present in the mitochondria of studied organisms (Figure 2). We found organisms that exclusively contained VDAC characterized by a few cysteine residues (including those within the N-terminus). We assumed that the number and distribution of cysteine residues allowed for their over-oxidation and, consequently, modulation of the channel under oxidative conditions. Therefore, we described this type of VDAC as a rsVDAC. This term refers to data on widely studied VDAC paralogs of humans and D. melanogaster. The latter has four VDAC paralogs: Dmel/porin, Dmel/porin2, Dmel/CG17140, and Dmel/CG17139. Dmel/porin is ubiquitous, while the remaining three paralogs are expressed exclusively in the male reproductive organ (Graham and Craigen, 2005). The knockout of D. melanogaster VDAC-encoding genes results in partial lethality, mitochondrial respiration defects, abnormal muscle mitochondrial morphology, synaptic dysfunction, and male infertility (Graham et al., 2010). All four paralogs were expressed in yeast Saccharomyces cerevisiae cells lacking yVDAC1 (Komarov et al., 2004), and only Dmel/porin and Dmel/porin2 complemented the absence of yVDAC1. Interestingly, the other two paralogs (Dmel/CG17140 and Dmel/CG17139) are characterized by the presence of several cysteine residues, one of which is located within their N-terminus (Figure 3A). Moreover, electrophysiological analysis showed that Dmel/CG17139 does not form a channel. Conversely, Dmel/CG17140 forms a channel in lipid membranes, but is far less voltage-dependent, unlike the canonical Dmel/porin or Dmel/porin2 (Komarov et al., 2004). Thus, Dmel/CG17140 only starts to close slightly at very high potential values (above 110 mV), whereas Dmel/porin2 closes at a potential of approximately 30 mV. Three paralogs, namely VDAC1, VDAC2, and VDAC3, have been detected in humans and other vertebrates. Human VDAC1 (hVDAC1) is ubiquitous and show the highest expression level, whereas hVDAC2 and hVDAC3 are highly abundant in the testes (Yamamoto et al., 2006). Accordingly, VDAC3 knockout in mice causes male infertility (Sampson et al., 2001). Although hVDAC1 and hVDAC2 can rescue the conditional lethal phenotype of yeast cells deficient for yVDAC1, hVDAC3 is almost unable to restore the wild-type phenotype (De Pinto et al., 2010) when the disulfide bond between cysteine residue 2 or 8 (Cys2/Cys8), located at the N-terminus region, and Cys122 is formed (Okazaki et al., 2015). The permanently reduced state of a cluster of close cysteine residues in hVDAC2 and hVDAC3 has been shown to sustain disulfide bond formation in the protein (Pittalà et al., 2020). Such a modification alters the electrophysiological properties of the formed channel, resulting in a lack of voltage dependence of the channel and consequently, the channel remains open. Interestingly, swapping the N-terminus of hVDAC1 with hVDAC3 (which eliminates the N-terminal cysteine residues in hVDAC3) restores the canonical activity of the formed channel and the ability to complement the lack of yVDAC1, as well as confers resistance to yeast against oxidative stress conditions (Reina et al., 2010).
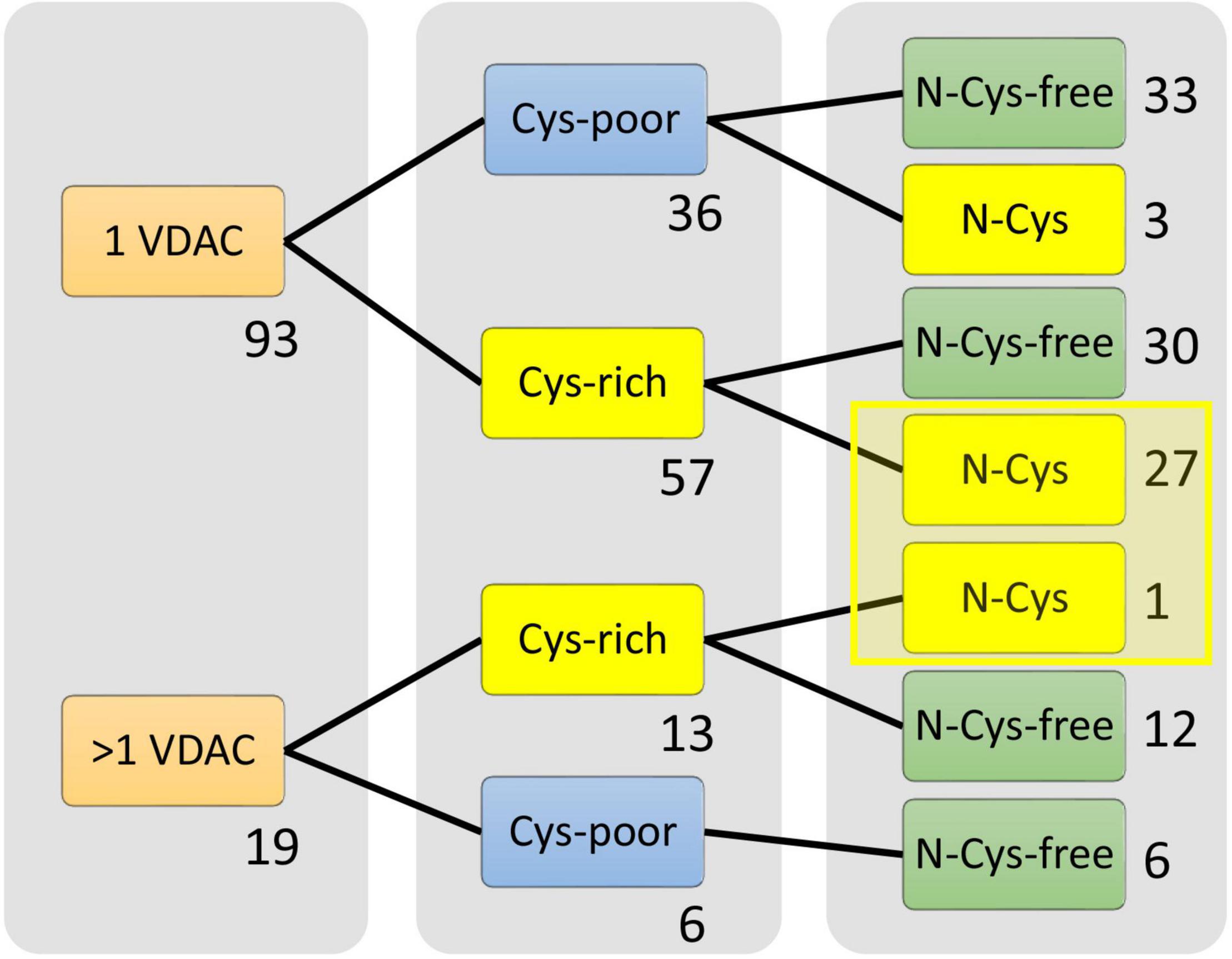
Figure 2. Selection of organisms that possess the only redox-sensitive VDAC (rsVDAC) variant in mitochondria. The analysis indicates the number of cysteine residues and their location in the VDAC secondary structure, as well as the absence or presence of VDAC paralogs, that is, the presence of only one VDAC variant (1 VDAC) or VDAC paralogs (>1 VDAC). Cys-poor: a set of organisms in which only one VDAC variant or at least one VDAC paralog (if present) has fewer than two cysteine residues in the primary structure; Cys-rich: a set of organisms in which only one VDAC variant or all VDAC paralogs (if present) have more than one cysteine residue in the primary structure; N- Cys-free: a set of organisms that have at least one VDAC variant with no N-terminal cysteine residue; rsVDAC, redox-sensitive VDAC.
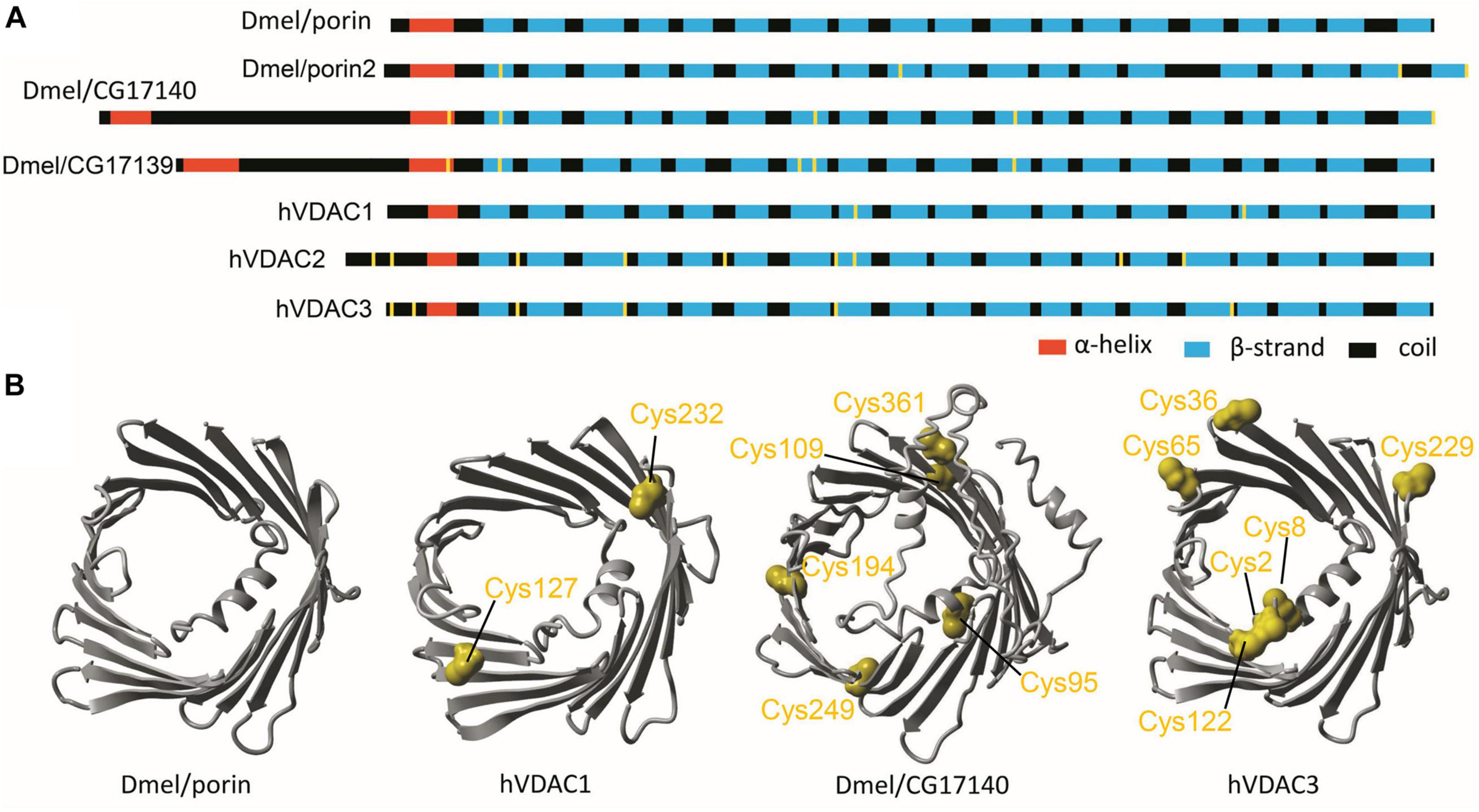
Figure 3. Drosophila melanogaster and human VDAC paralogs as models in studies of redox-sensitive VDAC. (A) The secondary structure of D. melanogaster and human VDAC paralogs with cysteine residues marked in yellow. (B) The tertiary structure predicted for ubiquitously expressed D. melanogaster and human paralogs (Dmel/porin and hVDAC1, respectively) as well as for paralogs with limited expression, mainly in D. melanogaster male reproductive tracts and human testes (Dmel/CG17140 and hVDAC3, respectively). Cysteine residues are marked in yellow.
Based on these correlating data, we assume that hVDAC3 and Dmel/CG17140 are orthologs due to their electrophysiological properties and tissue specificity. Both proteins were also used as our model of the assumed rsVDAC (Figure 3B), that is, VDAC containing multiple cysteine residues, with at least one within the N-terminus. The N-terminus is described as the most flexible segment of VDAC, which, in turn, facilitates interactions with other cysteine residues under oxidative conditions (Okazaki et al., 2015). However, it remains unclear if Dmel/CG17139 could be described as an rsVDAC. Based on the present analysis, the secondary structure and distribution of cysteine residues are very similar to Dmel/CG17140; however, limited available experimental data exclude the paralog channel activity.
Numerous parasitic species were found in the group of organisms that contained the assumed rsVDAC. As shown in Figure 4, 28 out of 112 studied species were assigned to the rsVDAC group, of which 21 were parasitic species, including obligatory ones (both internal and external) (Table 1). This finding suggests that metabolic and environmental conditions typical for parasitic organisms may support the presence of only one VDAC variant, which may be a redox sensor. In the case of free-living organisms, we noted the presence of at least one VDAC variant that displayed exceptionally low or no probability of cysteine residue oxidation, excluding the function of the redox sensor. Based on the human and D. melanogaster models, we believe this type of VDAC to be the most abundant and ubiquitous.
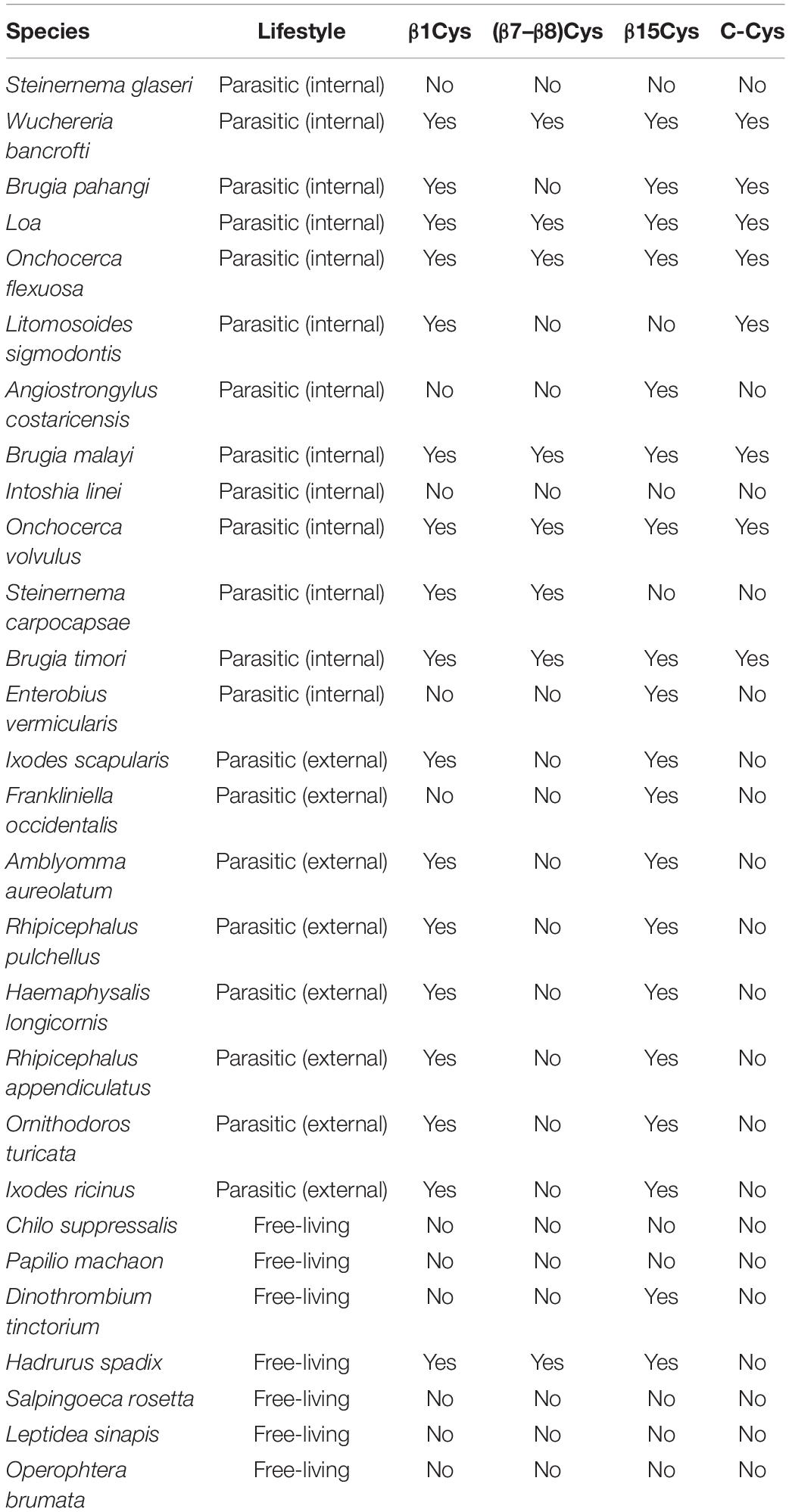
Table 1. List of organisms with redox-sensitive VDAC being the only VDAC variant, classified by their lifestyle and the presence or absence of cysteine residues in selected regions of the VDAC.
Marine eukaryotic organisms use redox-based mechanisms that mediate sensing and adaptation to environmental stress (Van Creveld et al., 2015). However, little is known about the role of ROS in the signaling of environmental stress conditions. ROS are toxic molecules that can cause severe damage to cells and, therefore, are strictly regulated by a wide range of antioxidant systems (Antonucci et al., 2021; Čapek and Roušar, 2021). However, it is crucial that a moderate amount of ROS act as second messenger molecules in a very complex network of signals in the cell (Tauffenberger and Magistretti, 2021). VDAC is involved in changes in the redox states of the cytosol and mitochondria (Gałgańska et al., 2008) and may act as a mitochondrial oxidative marker, participating in ROS signaling (Reina et al., 2016). Therefore, it is also possible that VDAC participates in the sensing of environmental stress conditions. If that is the case, the evolution of the VDAC structure would depend significantly on the inhabited environment.
Cyanidioschyzon merolae is a unicellular extremophilic eukaryotic organism adapted to high-sulfur acidic hot spring habitats. This organism has only one gene encoding VDAC, which contains four cysteine residues, all of which are located outside the N-terminus. By contrast, the tardigrade Hypsibius dujardini, which can survive under extreme conditions, also has one VDAC, with the protein containing only one cysteine residue located at the N-terminus. Both the VDAC proteins did not qualify as rsVDAC in our analysis. Instead, the group of organisms with the assumed rsVDAC was dominated by parasites. Using hVDAC3 as the rsVDAC model, we verified the presence and location of cysteine residues in assumed rsVDAC being the only VDAC variant in mitochondria. The regions containing cysteine residues included the N-terminus, the β1 strand, the segment containing β7 and β8 strands and the β15 strand (Figure 5A). Cysteine residues in the β1 and β15 strands turned out to be quite common in parasites, whereas those in the β7 and β8 segment were characteristic of internal parasites (Figure 5B and Table 1). In hVDAC3, the β7 and β8 segment is the location for Cys122, which is responsible for forming disulfide bonds with the N-terminal cysteine residues (Okazaki et al., 2015). A cysteine residue is also present in this region of Dmel/CG17140 (Figures 3, 5A), suggesting its potential role in redox sensitivity. Accordingly, the presence of two cysteine residues in modified mouse VDAC1, one at the N-terminus (Val3Cys) and the second one in β7 segment (Lys119Cys) resulted in the formation of disulfide bond and strong deviation from the typical native channel gating under oxidative condition (Mertins et al., 2012). In further studies, it was demonstrated that the N-terminal dynamics were essential for voltage gating (Zachariae et al., 2012; Zeth and Zachariae, 2018). We also indicated the presence of cysteine residue(s) at the C-terminus only in internal parasites (Table 1). It should be noted that cysteine residue(s) in the location is (or are) less common in VDAC of free-living organisms that have only one VDAC variant meeting our criteria of rsVDAC.
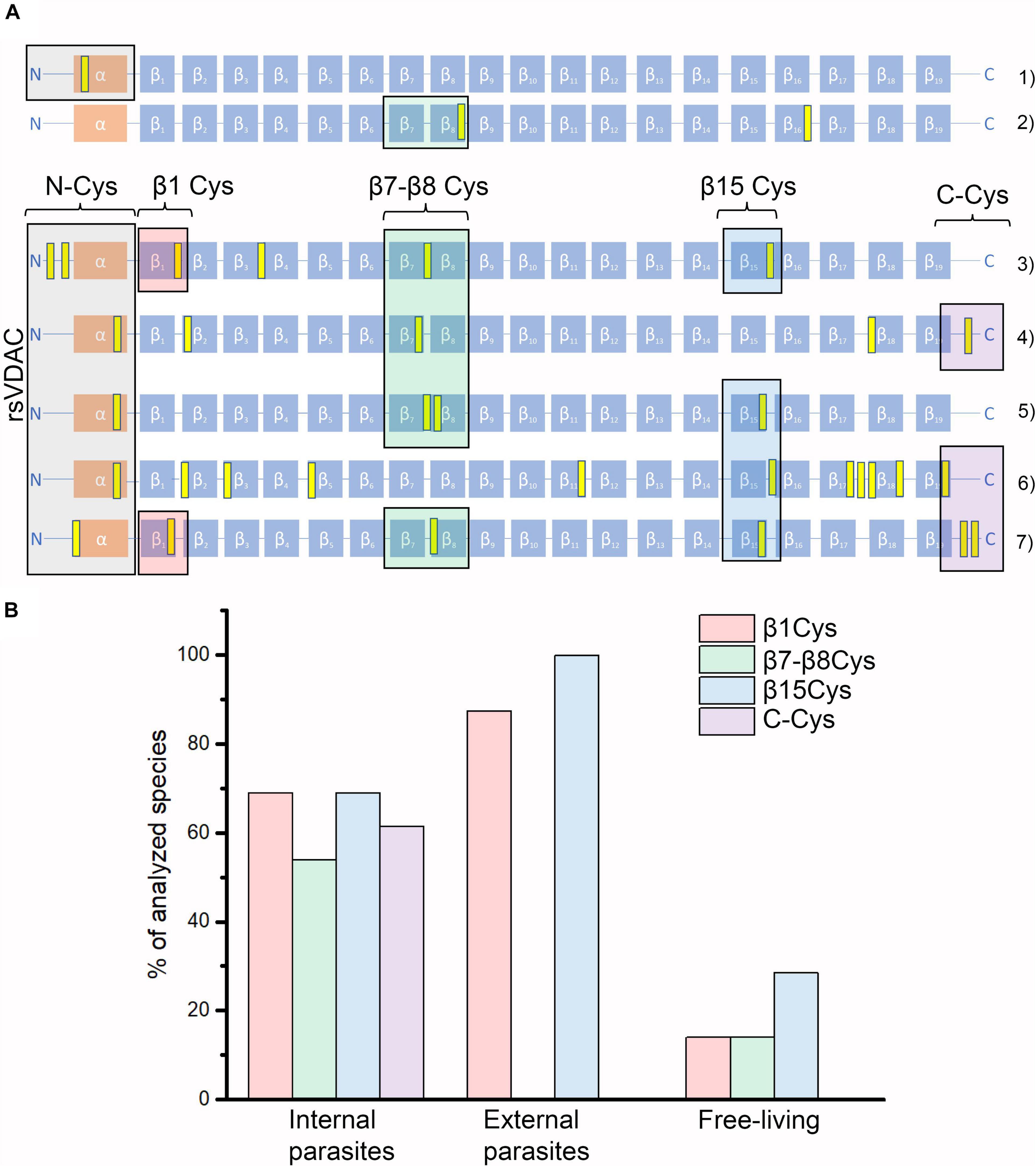
Figure 5. VDAC variants that differ in their number and location of cysteine residues. (A) Graphical representations of the studied organisms’ VDAC secondary structure with indicated locations of cysteine residues (marked in yellow). They represent “redox-insensitive” VDAC (1–2) and redox-sensitive VDAC (3–7). (1) Hypsibius dujardini (free-living); (2) human VDAC1; (3) human VDAC3; (4) Dmel\CG17140; (5) Aceria tosichella (free-living); (6) Folsomia candida (free-living); and (7) Onchocerca flexuosa (parasitic, internal). (B) The percentage of studied organisms with their assumed rsVDAC, being the only VDAC variant in mitochondria, including their lifestyle and the presence of cysteine residues in the selected regions of VDAC proteins (see also Table 1). Besides rsVDAC, A. tosichella and F. candida possess also “redox-insensitive” VDAC.
Thus, what are the features that distinguish parasites from free-living organisms? It is suggested that hosts of parasites can be considered a safe environment, and the external environment to which parasites are exposed, for example, during transmission, as hostile ones (Tinsley, 2007). Conversely, many years of coexistence with the host body requires suitable adaptation, such as a strong antioxidant system, which may serve as a defense strategy against host-generated ROS (Chiumiento and Bruschi, 2009). Perhaps the reduced imbalance between ROS generated by the host and the antioxidant system requires the presence of stronger redox sensors in some parasites. The same idea may apply to mature spermatozoa enriched in rsVDAC (Reina et al., 2016). Spermatozoa are foreign to both the male who produces them and the female who receives them (Clark and Schust, 2013). The organs of the female reproductive tract are subject to being colonized by pathogens and, therefore, have developed multiple adaptations to impede the invasion and proliferation of such pathogens. In addition to physical (production of a cleansing outward flow of fluid and secretion of a viscoelastic mucus) and chemical (acidification of the vaginal fluid) impediments in the female tract, immunological barriers could include components of the innate immune system, including inflammatory responses, ROS, and antimicrobial peptides, that could potentially damage spermatozoa (Ford, 2004; Wigby et al., 2019).
Conclusion
The imbalance between ROS production and antioxidant capacity – which causes oxidative stress – is a common feature of cells exposed to environmental stress conditions. Our hypothesis was based on the assumption that, in some organisms, VDAC amino acid sequences form proteins sensitive to redox changes. Specifically, cysteine residues can potentially be oxidized and form disulfide bonds that alter the properties of the formed channel. The available data indicate that the bond formation requires the presence of cysteine residue in the flexible N-terminus. A large group of organisms, most often possessing only one VDAC variant, do not contain this type of VDAC that we termed “redox-sensitive.” Redox-sensitive VDACs may form adjacent to “redox-insensitive” ones in organisms with VDAC paralogs, such as D. melanogaster or humans. The redox-sensitive paralogs are often expressed in specific tissues and are not ubiquitous. Finally, our observations indicate that certain organisms – mainly parasites – have only one, but potentially redox-sensitive, VDAC variant. Thus, there is a possibility that VDAC evolution may depend on environmental conditions and that the channel may mediate detection and adaptation to environmental stress.
Data Availability Statement
The original contributions presented in the study are included in the article/Supplementary Material, further inquiries can be directed to the corresponding author.
Author Contributions
AK conceived, designed, and made the analyses. HK supervised the performed analyses. AK and HK wrote the final version of the manuscript. MB contributed to the database and wrote sections of the manuscript. WG created the database, helped in the analysis, and wrote sections of the manuscript. All authors have read and approved the final manuscript.
Funding
This work was supported by the research grants of National Science Centre, Poland, Grant Numbers 2017/26/D/NZ1/00075 and 2016/21/B/NZ4/00131.
Conflict of Interest
The authors declare that the research was conducted in the absence of any commercial or financial relationships that could be construed as a potential conflict of interest.
Publisher’s Note
All claims expressed in this article are solely those of the authors and do not necessarily represent those of their affiliated organizations, or those of the publisher, the editors and the reviewers. Any product that may be evaluated in this article, or claim that may be made by its manufacturer, is not guaranteed or endorsed by the publisher.
Supplementary Material
The Supplementary Material for this article can be found online at: https://www.frontiersin.org/articles/10.3389/fphys.2021.750627/full#supplementary-material
Footnotes
References
Al Bitar, F., Roosens, N., Smeyers, M., Vauterin, M., Van Boxtel, J., Jacobs, M., et al. (2003). Sequence analysis, transcriptional and posttranscriptional regulation of the rice VDAC family. Biochim. Biophys. Acta - Gene Struct. Expr. 1625, 43–51. doi: 10.1016/S0167-4781(02)00590-0
Altschul, S. F., Madden, T. L., Schäffer, A. A., Zhang, J., Zhang, Z., Miller, W., et al. (1997). Gapped BLAST and PSI-BLAST: a new generation of protein database search programs. Nucleic Acids Res. 25, 3389–3402. doi: 10.1093/nar/25.17.3389
Antonucci, S., Di Lisa, F., and Kaludercic, N. (2021). Mitochondrial reactive oxygen species in physiology and disease. Cell Calcium 94:102344. doi: 10.1016/j.ceca.2020.102344
Bagnyukova, T. V., Lushchak, O. V., Storey, K. B., and Lushchak, V. I. (2007). Oxidative stress and antioxidant defense responses by goldfish tissues to acute change of temperature from 3 to 23°C. J. Therm. Biol. 32, 227–234. doi: 10.1016/j.jtherbio.2007.01.004
Baldi, P., Cheng, J., and Vullo, A. (2004). “Large-scale prediction of disulphide bond connectivity,” in Proceedings of the 17th International Conference on Neural Information Processing Systems (Cambridge, MA: MIT Press), 97–104.
Blachly-Dyson, E., Song, J., Wolfgang, W. J., Colombini, M., and Forte, M. (1997). Multicopy suppressors of phenotypes resulting from the absence of yeast VDAC encode a VDAC-like protein. Mol. Cell Biol. 17, 5727–5738. doi: 10.1128/MCB.17.10.5727
Čapek, J., and Roušar, T. (2021). Detection of oxidative stress induced by nanomaterials in cells-the roles of reactive oxygen species and glutathione. Molecules 26:4710. doi: 10.3390/molecules26164710
Cheng, J., Randall, A. Z., Sweredoski, M. J., and Baldi, P. (2005). SCRATCH: a protein structure and structural feature prediction server. Nucleic Acids Res. 33, W72–W76. doi: 10.1093/nar/gki396
Cheng, J., Saigo, H., and Baldi, P. (2006). Large-scale prediction of disulphide bridges using kernel methods, two-dimensional recursive neural networks, and weighted graph matching. Proteins 62, 617–629. doi: 10.1002/prot.20787
Chiumiento, L., and Bruschi, F. (2009). Enzymatic antioxidant systems in helminth parasites. Parasitol. Res. 105:593. doi: 10.1007/s00436-009-1483-0
Chung, W. H. (2017). Unraveling new functions of superoxide dismutase using yeast model system: beyond its conventional role in superoxide radical scavenging. J. Microbiol. 55, 409–416. doi: 10.1007/s12275-017-6647-5
Clark, G. F., and Schust, D. J. (2013). Manifestations of immune tolerance in the human female reproductive tract. Front. Immunol. 4:26. doi: 10.3389/fimmu.2013.00026
Colombini, M. (2012). VDAC structure, selectivity, and dynamics. Biochim. Biophys. Acta Biomembr. 1818, 1457–1465. doi: 10.1016/j.bbamem.2011.12.026
De Pinto, V. (2021). Renaissance of VDAC: new insights on a protein family at the interface between mitochondria and cytosol. Biomolecules 11:107. doi: 10.3390/biom11010107
De Pinto, V., Guarino, F., Guarnera, A., Messina, A., Reina, S., Tomasello, S., et al. (2010). Characterization of human VDAC isoforms: a peculiar function for VDAC3? Biochim. Biophys. Acta Bioenerg. 1797, 1268–1275. doi: 10.1016/j.bbabio.2010.01.031
De Pinto, V., Reina, S., Gupta, A., Messina, A., and Mahalakshmi, R. (2016). Role of cysteines in mammalian VDAC isoforms’ function. Biochim. Biophys. Acta Bioenerg. 1857, 1219–1227. doi: 10.1016/j.bbabio.2016.02.020
Di Rosa, M. C., Guarino, F., Conti Nibali, S., Magrì, A., and De Pinto, V. (2021). Voltage-dependent anion selective channel isoforms in yeast: expression, structure, and functions. Front. Physiol. 12:675708. doi: 10.3389/fphys.2021.675708
Edery, I. (2000). Circadian rhythms in a nutshell. Physiol. Genomics 3, 59–74. doi: 10.1152/physiolgenomics.2000.3.2.59
Elkeles, A., Breiman, A., and Zizi, M. (1997). Functional differences among wheat voltage-dependent anion channel (VDAC) isoforms expressed in yeast: indication for the presence of a novel VDAC-modulating protein? J. Biol. Chem. 272, 6252–6260. doi: 10.1074/jbc.272.10.6252
Fang, D., and Maldonado, E. N. (2018). VDAC regulation: a mitochondrial target to stop cell proliferation. Adv. Cancer Res. 138, 41–69. doi: 10.1016/bs.acr.2018.02.002
Flinner, N., Schleiff, E., and Mirus, O. (2012). Identification of two voltage-dependent anion channel-like protein sequences conserved in Kinetoplastida. Biol. Lett. 8, 446–449. doi: 10.1098/rsbl.2011.1121
Ford, W. C. (2004). Regulation of sperm function by reactive oxygen species. Hum. Reprod. Update 10, 387–399. doi: 10.1093/humupd/dmh034
Forsman, J. T., and Martin, T. E. (2009). Habitat selection for parasite-free space by hosts of parasitic cowbirds. Oikos 118, 464–470. doi: 10.1111/j.1600-0706.2008.17000.x
Gałgańska, H., Budzińska, M., Wojtkowska, M., and Kmita, H. (2008). Redox regulation of protein expression in Saccharomyces cerevisiae mitochondria: possible role of VDAC. Arch. Biochem. Biophys. 479, 39–45. doi: 10.1016/j.abb.2008.08.010
Graham, B. H., and Craigen, W. J. (2005). Mitochondrial voltage-dependent anion channel gene family in Drosophila melanogaster: complex patterns of evolution, genomic organization, and developmental expression. Mol. Genet. Metab. 85, 308–317. doi: 10.1016/j.ymgme.2005.03.009
Graham, B. H., Li, Z., Alesii, E. P., Versteken, P., Lee, C., Wang, J., et al. (2010). Neurologic dysfunction and male infertility in Drosophila porin mutants: a new model for mitochondrial dysfunction and disease. J. Biol. Chem. 285, 11143–11153. doi: 10.1074/jbc.M109.080317
Han, D., Antunes, F., Canali, R., Rettori, D., and Cadenas, E. (2003). Voltage-dependent anion channels control the release of the superoxide anion from mitochondria to cytosol. J. Biol. Chem. 278, 5557–5563. doi: 10.1074/jbc.M210269200
Hemono, M., Ubrig, É, Azeredo, K., Salinas-Giegé, T., Drouard, L., and Duchêne, A. M. (2020). Arabidopsis voltage-dependent anion channels (VDACs): overlapping and specific functions in mitochondria. Cells 9:1023. doi: 10.3390/cells9041023
Heslop, K. A., Rovini, A., Hunt, E. G., Fang, D., Morris, M. E., Christie, C. F., et al. (2020). JNK activation and translocation to mitochondria mediates mitochondrial dysfunction and cell death induced by VDAC opening and sorafenib in hepatocarcinoma cells. Biochem. Pharmacol. 171:113728. doi: 10.1016/j.bcp.2019.113728
Karachitos, A., Grobys, D., Antoniewicz, M., Jedut, S., Jordan, J., and Kmita, H. (2016). Human VDAC isoforms differ in their capability to interact with minocycline and to contribute to its cytoprotective activity. Mitochondrion 28, 38–48. doi: 10.1016/j.mito.2016.03.004
Komarov, A. G., Graham, B. H., Craigen, W. J., and Colombini, M. (2004). The physiological properties of a novel family of VDAC-like proteins from Drosophila melanogaster. Biophys. J. 86, 152–162. doi: 10.1016/S0006-3495(04)74093-X
Kroemer, G., Galluzzi, L., and Brenner, C. (2007). Mitochondrial membrane permeabilization in cell death. Physiol. Rev. 87, 99–163. doi: 10.1152/physrev.00013.2006
Laksanalamai, P., and Robb, F. T. (2004). Small heat shock proteins from extremophiles: a review. Extremophiles 8, 1–11. doi: 10.1007/s00792-003-0362-3
Lee, S. M., Hoang, M. H. T., Han, H. J., Kim, H. S., Lee, K., Kim, K. E., et al. (2009). Pathogen inducible voltage-dependent anion channel (AtVDAC) isoforms are localized to mitochondria membrane in Arabidopsis. Mol. Cells 27, 321–327. doi: 10.1007/s10059-009-0041-z
Lesser, M. P. (2006). Oxidative stress in marine environments: biochemistry and physiological ecology. Annu. Rev. Physiol. 68, 253–278. doi: 10.1146/annurev.physiol.68.040104.110001
Li, Z. Y., Xu, Z. S., He, G. Y., Yang, G. X., Chen, M., Li, L. C., et al. (2013). The voltage-dependent anion channel 1 (AtVDAC1) negatively regulates plant cold responses during germination and seedling development in Arabidopsis and interacts with calcium sensor CBL1. Int. J. Mol. Sci. 14, 701–713. doi: 10.3390/ijms14010701
Lushchak, V. I. (2011). Environmentally induced oxidative stress in aquatic animals. Aquat. Toxicol. 101, 13–30. doi: 10.1016/j.aquatox.2010.10.006
Mertins, B., Psakis, G., Grosse, W., Back, K. C., Salisowski, A., Reiss, P., et al. (2012). Flexibility of the N-terminal mVDAC1 segment controls the channel’s gating behavior. PLoS One 7:e47938. doi: 10.1371/journal.pone.0047938
Messina, A., Reina, S., Guarino, F., and De Pinto, V. (2012). VDAC isoforms in mammals. Biochim. Biophys. Acta Biomembr. 1818, 1466–1476. doi: 10.1016/j.bbamem.2011.10.005
Novikoff, A. B. (1945). The concept of integrative levels and biology. Science 101, 209–215. doi: 10.1126/science.101.2618.209
Okazaki, M., Kurabayashi, K., Asanuma, M., Saito, Y., Dodo, K., and Sodeoka, M. (2015). VDAC3 gating is activated by suppression of disulfide-bond formation between the N-terminal region and the bottom of the pore. Biochim. Biophys. Acta Biomembr. 1848, 3188–3196. doi: 10.1016/j.bbamem.2015.09.017
Pittalà, M., Saletti, R., Reina, S., Cunsolo, V., De Pinto, V., and Foti, S. (2020). A high resolution mass spectrometry study reveals the potential of disulfide formation in human mitochondrial voltage-dependent anion selective channel isoforms (hVDACs). Int. J. Mol. Sci. 21:1468. doi: 10.3390/ijms21041468
Pollastri, G., Przybylski, D., Rost, B., and Baldi, P. (2002). Improving the prediction of protein secondary structure in three and eight classes using recurrent neural networks and profiles. Proteins 47, 228–235. doi: 10.1002/prot.10082
Prado, A. M., Maia, B. M. C., Ueta, M. T., and Cabral, F. J. (2021). How much epigenetics and quantitative trait loci (QTL) mapping tell us about parasitism maintenance and resistance/susceptibility to hosts. Biochim. Biophys. Acta Mol. Basis Dis. 1864:166214. doi: 10.1016/j.bbadis.2021.166214
Queralt-Martín, M., Bergdoll, L., Teijido, O., Munshi, N., Jacobs, D., Kuszak, A. J., et al. (2020). A lower affinity to cytosolic proteins reveals VDAC3 isoform-specific role in mitochondrial biology. J. Gen. Physiol. 152:e012501. doi: 10.1085/JGP.201912501
Reina, S., Gaetana, M., Pittalà, G., Guarino, F., Messina, A., De Pinto, V., et al. (2020). Cysteine oxidations in mitochondrial membrane proteins?: the case of VDAC isoforms in mammals. Front. Cell Dev. Biol. 8:397. doi: 10.3389/fcell.2020.00397
Reina, S., Guarino, F., Magrì, A., and De Pinto, V. (2016). VDAC3 as a potential marker of mitochondrial status is involved in cancer and pathology. Front. Oncol. 6:264. doi: 10.3389/fonc.2016.00264
Reina, S., Palermo, V., Guarnera, A., Guarino, F., Messina, A., Mazzoni, C., et al. (2010). Swapping of the N-terminus of VDAC1 with VDAC3 restores full activity of the channel and confers anti-aging features to the cell. FEBS Lett. 584, 2837–2844. doi: 10.1016/j.febslet.2010.04.066
Rostovtseva, T. K., Bezrukov, S. M., and Hoogerheide, D. P. (2021). Regulation of mitochondrial respiration by VDAC is enhanced by membrane-bound inhibitors with disordered polyanionic C-terminal domains. Int. J. Mol. Sci. 22:7358. doi: 10.3390/ijms22147358
Rothschild, L. J., and Mancinelli, R. L. (2001). Life in extreme environments. Nature 409, 1092–1101. doi: 10.1038/35059215
Roy, A., Kucukural, A., and Zhang, Y. (2010). I-TASSER: a unified platform for automated protein structure and function prediction. Nat. Protoc. 5, 725–738. doi: 10.1038/nprot.2010.5
Saccone, C., Caggese, C., D’Erchia, A. M., Lanave, C., Oliva, M., and Pesole, G. (2003). Molecular clock and gene function. J. Mol. Evol. 57, S277–S285. doi: 10.1007/s00239-003-0037-9
Sampson, M. J., Decker, W. K., Beaudet, A. L., Ruitenbeek, W., Armstrong, D., Hicks, M. J., et al. (2001). Immotile sperm and infertility in mice lacking mitochondrial voltage-dependent anion channel type 3. J. Biol. Chem. 276, 39206–39212. doi: 10.1074/jbc.M104724200
Sampson, M. J., Lovell, R. S., and Craigen, W. J. (1997). The murine voltage-dependent anion channel gene family. Conserved structure and function. J Biol Chem. 272, 18966–18973. doi: 10.1074/jbc.272.30.18966
Sampson, M. J., Lovell, R. S., Davison, D. B., and Craigen, W. J. (1996). A novel mouse mitochondrial voltage-dependent anion channel gene localizes to chromosome 8. Genomics 36, 192–196. doi: 10.1006/geno.1996.0445
Sanyal, S. K., Kanwar, P., Fernandes, J. L., Mahiwal, S., Yadav, A. K., Samtani, H., et al. (2020). Arabidopsis mitochondrial voltage-dependent anion channels are involved in maintaining reactive oxygen species homeostasis, oxidative and salt stress tolerance in yeast. Front. Plant Sci. 11:50. doi: 10.3389/fpls.2020.00050
Sardiello, M., Tripoli, G., Oliva, M., Santolamazza, F., Moschetti, R., Barsanti, P., et al. (2003). A comparative study of the porin genes encoding VDAC, a voltage-dependent anion channel protein, in Anopheles gambiae and Drosophila melanogaster. Gene 317, 111–115. doi: 10.1016/S0378-1119(03)00658-9
Schein, S. J., Colombini, M., and Finkelstein, A. (1976). Reconstitution in planar lipid bilayers of a voltage-dependent anion-selective channel obtained from paramecium mitochondria. J. Membrain Biol. 30, 99–120. doi: 10.1007/BF01869662
Shoshan-Barmatz, V., De Pinto, V., Zweckstetter, M., Raviv, Z., Keinan, N., and Arbel, N. (2010). VDAC, a multi-functional mitochondrial protein regulating cell life and death. Mol. Aspects Med. 31, 227–285. doi: 10.1016/j.mam.2010.03.002
Shoshan-Barmatz, V., Krelin, Y., and Shteinfer-Kuzmine, A. (2018). VDAC1 functions in Ca2+ homeostasis and cell life and death in health and disease. Cell Calcium 69, 81–100. doi: 10.1016/j.ceca.2017.06.007
Shoshan-Barmatz, V., Shteinfer-Kuzmine, A., and Verma, A. (2020). VDAC1 at the intersection of cell metabolism, apoptosis, and diseases. Biomolecules 10:1485. doi: 10.3390/biom10111485
Sinha, K., Das, J., Pal, P. B., and Sil, P. C. (2013). Oxidative stress: the mitochondria-dependent and mitochondria-independent pathways of apoptosis. Arch. Toxicol. 87, 1157–1180. doi: 10.1007/s00204-013-1034-4
Sokolova, I. M., Frederich, M., Bagwe, R., Lannig, G., and Sukhotin, A. (2012). A Energy homeostasis as an integrative tool for assessing limits of environmental stress tolerance in aquatic invertebrates. Mar. Environ. Res. 79, 1–15. doi: 10.1016/j.marenvres.2012.04.003
Tauffenberger, A., and Magistretti, P. J. (2021). Reactive oxygen species: beyond their reactive behavior. Neurochem. Res. 46, 77–87. doi: 10.1007/s11064-020-03208-7
The UniProt Consortium (2021). UniProt: the universal protein knowledgebase in 2021. Nucleic Acids Res. 49, D480–D489. doi: 10.1093/nar/gkaa1100
Tinsley, R. C. (1999). Parasite adaptation to extreme conditions in a desert environment. Parasitology 119, S31–S56. doi: 10.1017/S0031182000084638
Tinsley, R. C. (2007). “Parasitism and hostile environments,” in Parasitism and Ecosystems, eds R. F. Thomas, É Thomas, F. Thomas, F. Renaud, and J.-F. Guegan (Oxford: Oxford University Press).
Van Creveld, S. G., Rosenwasser, S., Schatz, D., Koren, I., and Vardi, A. (2015). Early perturbation in mitochondria redox homeostasis in response to environmental stress predicts cell fate in diatoms. ISME J. 9, 385–395. doi: 10.1038/ismej.2014.136
Wandrey, M., Trevaskis, B., Brewin, N., and Udvardi, M. K. (2004). Molecular and cell biology of a family of voltage-dependent anion channel porins in Lotus japonicus. Plant Physiol. 134, 182–193. doi: 10.1104/pp.103.031484
Wang, H. C., Susko, E., and Roger, A. J. (2006). On the correlation between genomic G+ C content and optimal growth temperature in prokaryotes: data quality and confounding factors. Biochem. Biophys. Res. Commun. 342, 681–684. doi: 10.1016/j.bbrc.2006.02.037
Wang, X., and Komatsu, S. (2018). Proteomic approaches to uncover the flooding and drought stress response mechanisms in soybean. J. Proteomics 172, 201–215. doi: 10.1016/j.jprot.2017.11.006
Wigby, S., Suarez, S. S., Lazzaro, B. P., Pizzari, T., and Wolfner, M. F. (2019). Sperm success and immunity. Curr. Top. Dev. Biol. 135, 287–313. doi: 10.1016/bs.ctdb.2019.04.002
Wojtkowska, M., Jaa̧kalski, M., Pieńkowska, J. R., Stobienia, O., Karachitos, A., Przytycka, T. M., et al. (2012). Phylogenetic analysis of mitochondrial outer membrane β-barrel channels. Genome Biol. Evol. 4, 110–125. doi: 10.1093/gbe/evr130
Yamamoto, T., Yamada, A., Watanabe, M., Yoshimura, Y., and Yamazaki, N. (2006). VDAC1, having a shorter N-terminus than VDAC2 but showing the same migration in an SDS- polyacrylamide gel, is the predominant form expressed in mitochondria of various tissues. J. Proteome Res. 5, 3336–3344. doi: 10.1021/pr060291w
Yang, J., Yan, R., Roy, A., Xu, D., Poisson, J., and Zhang, Y. (2015). The I-TASSER suite: protein structure and function prediction. Nat. Methods 12, 7–8. doi: 10.1038/nmeth.3213
Yang, J., and Zhang, Y. (2015). I-TASSER server: new development for protein structure and function predictions. Nucleic Acids Res. 43, W174–W181. doi: 10.1093/nar/gkv342
Young, M. J., Bay, D. C., Hausner, G., and Court, D. A. (2007). The evolutionary history of mitochondrial porins. BMC Evol. Biol. 7:31. doi: 10.1186/1471-2148-7-31
Zachariae, U., Schneider, R., Briones, R., Gattin, Z., Demers, J. P., Giller, K., et al. (2012). β-Barrel mobility underlies closure of the voltage-dependent anion channel. Structure 20, 1540–1549. doi: 10.1016/j.str.2012.06.015
Keywords: VDAC, parasite, cysteine oxidation, redox sensor, environmental stress, spermatozoa
Citation: Karachitos A, Grabiński W, Baranek M and Kmita H (2021) Redox-Sensitive VDAC: A Possible Function as an Environmental Stress Sensor Revealed by Bioinformatic Analysis. Front. Physiol. 12:750627. doi: 10.3389/fphys.2021.750627
Received: 30 July 2021; Accepted: 12 November 2021;
Published: 13 December 2021.
Edited by:
Vito De Pinto, University of Catania, ItalyReviewed by:
Carmen A. Mannella, University of Maryland Medical Center, United StatesGiovanna Lippe, University of Udine, Italy
Copyright © 2021 Karachitos, Grabiński, Baranek and Kmita. This is an open-access article distributed under the terms of the Creative Commons Attribution License (CC BY). The use, distribution or reproduction in other forums is permitted, provided the original author(s) and the copyright owner(s) are credited and that the original publication in this journal is cited, in accordance with accepted academic practice. No use, distribution or reproduction is permitted which does not comply with these terms.
*Correspondence: Andonis Karachitos, YW5kb25pcy5rYXJhY2hpdG9zQGFtdS5lZHUucGw=