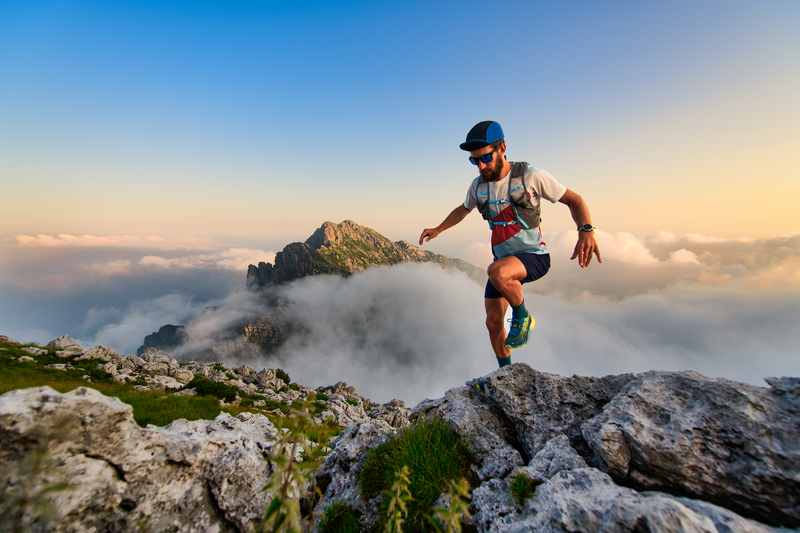
95% of researchers rate our articles as excellent or good
Learn more about the work of our research integrity team to safeguard the quality of each article we publish.
Find out more
BRIEF RESEARCH REPORT article
Front. Physiol. , 25 October 2021
Sec. Avian Physiology
Volume 12 - 2021 | https://doi.org/10.3389/fphys.2021.747473
This article is part of the Research Topic Fat metabolism and deposition in Poultry: Physiology, Genetics, Nutrition and Interdisciplinary Research, Volume I View all 13 articles
We recently discovered a novel cDNA encoding the precursor of a small secretory protein, neurosecretory protein GM (NPGM), in the mediobasal hypothalamus of chickens. Although our previous study showed that subcutaneous infusion of NPGM for 6 days increased body mass in chicks, the chronic effect of intracerebroventricular (i.c.v.) infusion of NPGM remains unknown. In this study, we performed i.c.v. administration of NPGM in eight-day-old layer chicks using osmotic pumps for 2 weeks. In the results, chronic i.c.v. infusion of NPGM significantly increased body mass, water intake, and the mass of abdominal and gizzard fat in chicks, whereas NPGM did not affect food intake, liver and muscle masses, or blood glucose concentration. Morphological analyses using Oil Red O and hematoxylin-eosin stainings revealed that fat accumulation occurred in both the liver and gizzard fat after NPGM infusion. The real-time PCR analysis showed that NPGM decreased the mRNA expression of peroxisome proliferator-activated receptor α, a lipolytic factor in the liver. These results indicate that NPGM may participate in fat storage in chicks.
Energy intake through feeding behavior is a necessary element for the maintenance of animal life and growth. However, excessive food intake can cause diseases, such as obesity (Moore et al., 2014). In general, it is known that animals have a complex endocrine system that controls their appetite to avoid obesity (Lawrence et al., 1999; Geary, 2000). Poultry, especially chickens, are an important agricultural species and have been repeatedly selected for meat and/or egg production, with emphasis on feed efficiency and growth rate (Abo Ghanima et al., 2020; Rehman et al., 2020; Batool et al., 2021). However, there is concern that excessive food intake and fat accumulation in broiler chickens can lead to reduced growth rates and metabolic diseases, resulting in lower meat production (Knowles et al., 2008). For layer chickens, fatty acid is required to product eggs. However, excessive fat accumulation in liver and abdomen may induce several health disorders and lead to a decline of egg production and reproductive performance (Trott et al., 2014; Cherian, 2015; Wang et al., 2019). This problem is undesirable from the standpoint of animal health and production efficiency. Therefore, it is important to control growth and fat accumulation in chickens to enable efficient meat and/or egg production. Understanding the mechanisms of fat accumulation in birds at the molecular and cellular levels will provide sufficient knowledge to improve these problems. In contrast, chicken fat is also one of healthy foods (Peña-Saldarriaga et al., 2020). Several studies have shown that the nutrient composition of diets affects adipose tissue development and lipid metabolism in chickens (Wang et al., 2017). However, the contribution of hormones to lipid metabolism in chickens and other birds has not yet been revealed. Therefore, it is likely that unknown factors are involved in these processes in avian species.
Recently, we discovered a novel cDNA encoding the precursor of a small secretory protein in the chick hypothalamus (Ukena et al., 2014; Shikano et al., 2018a). We named the small protein of 83-amino acid residues as neurosecretory protein GM (NPGM) (Ukena et al., 2014; Shikano et al., 2018a). The NPGM gene is highly conserved in vertebrates, including chickens, rats, and humans (Ukena et al., 2014). Our previous studies identified the localization of NPGM in the chick brain by in situ hybridization and immunostaining. NPGM-producing cells are localized in the infundibular nucleus (IN) and the medial mammillary nucleus (MM) of the hypothalamus in chicks (Shikano et al., 2018a). These nuclei are known to be feeding and metabolic centers in chicks. Furthermore, we found that the expression levels of NPGM mRNA gradually decreased during post-hatching development (Shikano et al., 2018a). These results suggest that NPGM is a novel hypothalamic factor involved in energy metabolism immediately after hatching in chicks. Subsequently, we performed chronic subcutaneous infusion to clarify the physiological functions of NPGM in chicks. Chronic subcutaneous infusion of NPGM for 6 days increased the body mass gain in chicks (Shikano et al., 2018c). It was not clear which parts of the body gained mass, although NPGM seemed to induce the largest increase in abdominal fat mass (Shikano et al., 2018c). In the present study, we analyzed the effects of chronic intracerebroventricular (i.c.v.) infusion of NPGM for 2 weeks on food intake, water intake, and body composition, including fat accumulation and lipid metabolism in the abdominal and gizzard fat and the liver.
One-day-old male layer chicks were purchased from a commercial company (Nihon layer, Gifu, Japan) and housed in a windowless room at 28°C on a 20–h light (4:00–24:00)/4–h dark (0:00–4:00) cycle. The chicks had ad libitum access to food and water.
Chicken NPGM was synthesized using fluorenylmethyloxycarbonyl (Fmoc) and a peptide synthesizer (Syro Wave; Biotage, Uppsala, Sweden) according to our previous method (Masuda et al., 2015; Shikano et al., 2019). The purity of the protein was >95%. Lyophilized NPGM was weighed using an analytical and precision balance (AP125WD; Shimadzu, Kyoto, Japan).
Chronic i.c.v. infusion of chicken NPGM was performed according to a previously reported method (Shikano et al., 2018b, 2019). NPGM was dissolved in absolute propylene glycol and adjusted to 30% propylene glycol at pH 8.0 as a vehicle solution. The administration period of NPGM was fixed to 13 days because our preliminary study showed that the chemical instability of NPGM occurred in the osmotic pump over 2 weeks. Eight-day-old chicks were i.c.v.-infused with 0 (vehicle control) or 15 nmol/day NPGM. This dose has been reported to be a physiological dose in rats and chicks (Stoyanovitch et al., 2005; Rich et al., 2007; Shikano et al., 2018b, 2019). Body mass, food intake, and water intake were measured daily (between 9:00 and 10:00) throughout the experiment. After 13 days of chronic i.c.v. infusion of NPGM, chicks were euthanized by decapitation, and the masses of the liver, abdominal and gizzard fat, subcutaneous fat, pectoralis major muscle, pectoralis minor muscle, and biceps femoris muscle were measured. Blood samples were taken at the endpoint and centrifuged at 800× g for 15 min at 4°C to separate the serum. The blood glucose levels were measured using a GLUCOCARD G + meter (Arkray, Kyoto, Japan). The method for identification and isolation of the mediobasal hypothalamus was performed according to a previously reported method (Ukena et al., 2014). The chicken pituitary gland lies within the sella turcia of the sphenoid bone. We removed sphenoid bone for isolation of the pituitary gland. The mediobasal hypothalamus, pituitary gland, abdominal and gizzard fat, and liver were immediately snap-frozen in liquid nitrogen and stored at −80°C for real-time PCR analysis.
Oil Red O staining of the livers and hematoxylin and eosin staining of the gizzard fat were performed according to a previously reported method (Shikano et al., 2019).
RNA extraction was performed as previously described (Shikano et al., 2019). PCR amplifications were performed using THUNDERBIRD SYBR qPCR Mix (TOYOBO, Osaka, Japan): 95°C for 20 s followed by 40 cycles at 95°C for 3 s and 60°C for 30 s using a real-time thermal cycler (CFX Connect; BioRad, Hercules, CA, United States).
The amplification of the lipid metabolic factors was performed using the primer sets listed in Supplementary Table 1. Acetyl-CoA carboxylase (ACC), fatty acid synthase (FAS), stearoyl-CoA desaturase 1 (SCD1), malic enzyme (ME), peroxisome proliferator-activated receptor γ (PPARγ), and fatty acid transporter 1 (FATP1) are lipogenic enzymes and related factors, and peroxisome proliferator-activated receptor α (PPARα), carnitine palmitoyltransferase 1a (CPT1a), lipoprotein lipase (LPL), adipose triglyceride lipase (ATGL), and comparative gene identification-58 (CGI-58) are lipolytic enzymes and related factors.
Amplification of feeding, water intake, and growth-related factors was performed using the primer sets listed in Supplementary Table 1. We chose neurosecretory protein GL (NPGL) as a paralogous gene of NPGM; neuropeptide Y (NPY) and agouti-related peptide (AGRP) as orexigenic factors; pro-opiomelanocortin (POMC), glucagon-like peptide-1 (GLP-1), and cholecystokinin (CCK) as anorexigenic factors; and angiotensinogen (AGT) and angiotensin-converting enzyme (ACE) as water intake-related factors in the hypothalamus. In the pituitary gland, we chose growth hormone (GH), prolactin (PRL), thyroid-stimulating hormone (TSH), and POMC. The relative quantification for each expression was determined by the 2–ΔΔCt method using β-actin (ACTB) as an internal control.
Data were analyzed with Student’s t-test for endpoint body mass, cumulative food intake, cumulative water intake, tissue mass, blood glucose level, and mRNA expression or two-way repeated-measures analysis of variance (ANOVA) followed by Bonferroni’s test for body mass gain and daily food and water intake. The significance level was set at P < 0.05. All results are expressed as the mean ± SEM.
To investigate the effect of chronic i.c.v. infusion of NPGM on energy metabolism, we measured body mass, food intake, and water intake for 2 weeks. The results showed that chronic infusion of NPGM significantly increased daily body mass gain and final body mass after 2 weeks (Figures 1A,B). However, daily and cumulative food intake remained unchanged (Figures 1C,D). The daily water intake was almost unchanged, but the cumulative water intake increased after 2 weeks of NPGM infusion (Figures 1E,F).
Figure 1. Effect of chronic i.c.v. infusion of NPGM on body mass gain, food intake, and water intake. The results were obtained by the infusion of the vehicle (control; CTL) and NPGM. The change in the body mass gain after surgery (A,B). The daily and cumulative food intake (C,D). The daily and cumulative water intake (E,F). Data are expressed as the mean ± SEM (n = 7–8). Data were analyzed using the student t-test and two-way repeated-measures analysis of variance (ANOVA). An asterisk indicates a statistically significant difference (*P < 0.05, **P < 0.01).
When we analyzed the effect of NPGM on body composition and blood glucose level, chronic infusion of NPGM increased the mass of the abdominal and gizzard fat and tended to increase the mass of the subcutaneous fat (Figure 2B). On the other hand, no change was observed in the liver mass and the muscle masses of the pectoralis major, pectoralis minor, and biceps femoris muscles after the infusion of NPGM (Figures 2A,C). Furthermore, the blood glucose levels were not altered by NPGM (Figure 2D).
Figure 2. Effect of chronic i.c.v. infusion of NPGM on body composition. The results were obtained by the infusion of the vehicle (control; CTL) and NPGM for 2 weeks. Ratio of the liver mass/body mass (A), ratio of the abdominal and gizzard fat mass/body mass, and the subcutaneous fat mass/body mass (B), ratio of pectoralis major, pectoralis minor, and biceps femoris muscle masses/body mass (C), and blood glucose level (D) were measured at the end of the experiment. Data are expressed as the mean ± SEM (n = 7–8). Data were analyzed by the Student’s t-test. Asterisks indicate statistically significant differences (***P < 0.005).
We elucidated the effect of fat accumulation by morphological analyses in the liver and gizzard fat. Oil Red O staining of the liver demonstrated that lipid droplets were deposited after chronic i.c.v. infusion of NPGM (Figure 3A).
Figure 3. Effect of chronic i.c.v. infusion of NPGM on lipid deposition in the liver and lipid droplets in the gizzard fat. The results were obtained 2 weeks after infusion of the vehicle (control; CTL) and NPGM. In the liver, representative photomicrographs of the sections were stained by Oil Red O (scale bar = 100 μm) (A). For the gizzard fat, exterior photographs around the gizzard (left panel, scale bar = 1 cm) and representative photographs of the sections stained by hematoxylin and eosin (right panel, scale bar = 100 μm) (B) were obtained.
We found an increase in the gizzard fat in NPGM-treated chicks compared with controls (Figure 3B, lower left panel). Hematoxylin-eosin staining revealed that the adipocytes in the gizzard fat of the NPGM-treated chicks were larger than those of the control group (Figure 3B, lower right panel).
To explore the molecular mechanism of fat accumulation in the liver and the abdominal and gizzard fat by chronic i.c.v. infusion of NPGM, we investigated the mRNA expression levels of the lipid metabolic factors. We analyzed the mRNA expression levels of ACC, FAS, SCD1, ME, PPARγ, FATP1, PPARα, CPT1a, LPL, ATGL, and CGI-58 after chronic infusion of NPGM. In the liver, NPGM decreased the mRNA expression of PPARα (Figure 4A), whereas in the abdominal and gizzard fat, the mRNA expression levels did not change (Figure 4B).
Figure 4. Effect of chronic i.c.v. infusion of NPGM on the mRNA expression of lipogenic and lipolytic factors [acetyl-CoA carboxylase (ACC), fatty acid synthase (FAS), stearoyl-CoA desaturase 1 (SCD1), malic enzyme (ME), peroxisome proliferator-activated receptor γ (PPARγ), fatty acid transporter 1 (FATP1), peroxisome proliferator-activated receptor α (PPARα), carnitine palmitoyltransferase 1a (CPT1a), lipoprotein lipase (LPL), adipose triglyceride lipase (ATGL), and comparative gene identification-58 (CGI-58)] in the liver (A) and the abdominal and gizzard fat (B). The results were obtained 2 weeks after infusion of the vehicle (control; CTL) and NPGM. Data are expressed as the mean ± SEM (n = 7–8). Data were analyzed by Student’s t-test. An asterisk indicates a statistically significant difference (**P < 0.01).
Based on the results of body mass gain and water intake, we investigated the mRNA expression levels of the endocrine hormones and related factors, which are involved in feeding, water intake, and growth in the hypothalamus and pituitary gland after chronic infusion of NPGM. NPGM did not change the mRNA expression levels in the hypothalamus (Supplementary Figure 1A), whereas NPGM decreased the expression of PRL mRNA in the pituitary gland (Supplementary Figure 1B).
In this study, we investigated the effects of a novel small protein, NPGM, on the body composition of chicks by chronic i.c.v. infusion for 2 weeks. Our data showed that the infusion of NPGM increased body mass and cumulative water intake without changes in food intake. Furthermore, morphological analyses showed that NPGM induced fat accumulation in the liver and gizzard fat. This study is the first to show that NPGM induces fat deposition in animals.
Several studies have been conducted on fat accumulation in mammals (Gesta et al., 2007; Peirce et al., 2014). However, the mechanism of lipid metabolism in birds has not been established. The biological structures of birds differ from those of mammals, and their endocrine actions are unique. For example, birds do not have heat-producing brown adipose tissue (BAT) and have only white adipose tissue (WAT) (Johnston, 1971). Moreover, birds are hyperglycemic animals that exhibit insulin resistance despite normal insulin activity (Dupont et al., 2008). In chickens, unlike mammals, insulin has little effect on glucose uptake in adipose tissue and does not inhibit lipolysis (Tokushima et al., 2005). Furthermore, glucagon is thought to be the main lipolytic hormone (Scanes et al., 1994). In mammals, leptin is a hormone secreted by adipocytes and acts on the hypothalamus to suppress eating and obesity (Halaas et al., 1995). Recently, the leptin gene has been identified in some bird and these findings suggest that avian leptin may have a different physiological function from that of lipid metabolism in mammals (Seroussi et al., 2017, 2019; Beauclair et al., 2019; Friedman-Einat and Seroussi, 2019). The present study showed that NPGM is a new player in regulating fat deposition in avian species, chicken.
We have also reported a paralog gene with NPGM, which is named neurosecretory protein GL (NPGL) in vertebrates (Ukena et al., 2014; Ukena, 2018). The NPGL gene is also highly conserved in vertebrates, including chickens, rats, mice, and humans (Ukena et al., 2014; Ukena, 2018). Sequence similarity between mature NPGM and NPGL is 54% in chickens (Shikano et al., 2018c). Our previous studies showed that NPGL-immunoreactive cells were localized in the IN and MM of the hypothalamus, and parts of NPGL-immunoreactive cells were identical to NPGM-immunoreactive cells in the MM in 1- and 15-day-old chicks and the IN in 15-day-old chicks (Shikano et al., 2018a). The expression levels of NPGL mRNA gradually increased during post-hatching development. In contrast, the expression level of NPGM mRNA gradually decreased after hatching, suggesting that NPGM and NPGL have different timings of action during the growth process (Shikano et al., 2018a). Functional analysis of NPGL has already been demonstrated in rats, mice, and chicks (Iwakoshi-Ukena et al., 2017; Matsuura et al., 2017; Shikano et al., 2018b, 2019, 2020; Fukumura et al., 2021). The results showed that the long-term administration of NPGL stimulated food intake and induced fat accumulation in rats, mice, and chicks. In contrast, NPGM treatment did not change food intake or the gene expression levels of feeding and growth regulators (NPY, AgRP, POMC, GLP-1, CCK, GH, and TSH) in the hypothalamus and pituitary gland in this study. Therefore, it is likely that NPGM does not directly regulate feeding behavior in chicks. In addition, the expression levels of water intake-regulating factors (AGT and ACE) did not change, although the amount of drinking water was increased by NPGM infusion. The future study is necessary to elucidate the regulatory mechanism of drinking behavior by NPGM.
In our previous studies, NPGL increased the mass of WAT through de novo lipogenesis in rats and chicks, resulting in fast body mass gain (Iwakoshi-Ukena et al., 2017; Shikano et al., 2019). In general, birds are known to use the liver rather than adipose tissue as the primary site of de novo lipogenesis (Leveille et al., 1975). Glucose taken up by the liver is converted into fatty acids and cholesterol and, subsequently, fatty acids are converted into triacylglycerols (TAGs). Cholesterol and TAGs are transported by very low-density lipoproteins (VLDL) to the adipose tissue where they are stored (Hermier, 1997). However, we found that de novo lipogenesis by NPGL in chicks occurred in the adipose tissue but not in the liver (Shikano et al., 2019). This result indicates that NPGL is a neuropeptide that upregulates de novo lipogenesis in the adipose tissues of chicks. In the present study, chronic i.c.v. infusion of NPGM induced body mass gain in chicks, similar to NPGL. However, mRNA expression analysis of lipid metabolism factors involved in lipogenesis and lipolysis showed little change in the expression levels of lipid metabolism factors in the liver and adipose tissue, except for a decrease in the lipolytic factor PPARα in the liver. These results suggest that de novo lipogenesis by NPGM does not occur in these tissues. Therefore, the mechanism of action of NPGM and NPGL for fat accumulation may differ in the same animals. It is also known that lipogenesis and lipolysis in the adipose tissue are controlled by the hypothalamus via the sympathetic nervous system in mammals (Buettner et al., 2008; Scherer et al., 2011). Therefore, NPGM may be involved in the sympathetic control of fat accumulation in chickens. Future studies are needed to classify the target regions and neural networks of NPGM-producing neurons in chickens.
In this study, PRL mRNA expression was decreased in the pituitary gland after NPGM infusion. PRL is known to be involved in more than 300 actions in vertebrates (Bole-Feysot et al., 1998). In birds, the most studied role of PRL is involved in actions during the egg incubation phase (March et al., 1994). In addition, PRL has been reported to increase feeding behavior and activate LPL in adipocytes (Garrison and Scow, 1975; Das, 1991). In the present study, there was no change in food intake or LPL mRNA expression levels after NPGM infusion. However, NPGM may inhibit the increase in food intake and the activation of lipolysis through the suppression of PRL expression. In the future, it will be necessary to clarify the exact relationship between NPGM and PRL in chickens.
The present data on NPGM-induced fat accumulation in chickens may facilitate future applications in animal agriculture, such as controlling fat accumulation in poultry. The French food, foie gras, is a fatty liver, and the artificial production of foie gras is a problem related to animal welfare (Litt et al., 2020). In addition, chicken fat is becoming a healthy food because of the high amounts of unsaturated fatty acids (Peña-Saldarriaga et al., 2020) and these fatty acids are necessary constituents of the cell membrane and represent precursors to numerous different body components (Alagawany et al., 2021a,b). The application of NPGM to fat deposition in the liver and the abdominal and gizzard fat will have an impact on agricultural and livestock industries.
In conclusion, chronic i.c.v. administration of NPGM increased the body mass and caused fat accumulation in both the liver and the abdominal and gizzard fat. This is the first report describing the effect of NPGM on fat deposition in vertebrates, including birds. These results suggested that the biological functions of NPGM might affect egg production and reproductive performance in layers. In the future, we need to analyze the effects of NPGM in broilers for meat production. In addition, to clarify the exact role of NPGM action in the brain, the receptor for NPGM needs to be discovered. However, to date, receptors for NPGM and NPGL have not yet been discovered in vertebrates. It is known that fat accumulation is essential for birds, not only to sustain life but also to maintain bird-specific behaviors such as migration (Price, 2010; Guglielmo, 2018). Therefore, NPGM is considered an important factor in avian survival and reproduction. In the future, comparative analysis using other avian species and other vertebrates will help clarify the physiological role of NPGM in animals.
The raw data supporting the conclusions of this article will be made available by the authors, without undue reservation.
The animal study was reviewed and approved by the Guide for the Care and Use of Laboratory Animals prepared by Hiroshima University (Higashi-Hiroshima, Japan).
MK, EI-U, and KU: conceptualization and writing—review and editing. MK, EI-U, MF, and KU: methodology and investigation. MK: writing—original draft preparation and visualization. EI-U and KU: project administration and funding acquisition. All authors have read and agreed to the published version of the manuscript.
This work was supported by JSPS KAKENHI Grant (JP19K06768 to EI-U and JP19H03258 to KU), the Kieikai Research Foundation (KU).
The authors declare that the research was conducted in the absence of any commercial or financial relationships that could be construed as a potential conflict of interest.
All claims expressed in this article are solely those of the authors and do not necessarily represent those of their affiliated organizations, or those of the publisher, the editors and the reviewers. Any product that may be evaluated in this article, or claim that may be made by its manufacturer, is not guaranteed or endorsed by the publisher.
We would like to thank Kenshiro Shikano, Yuki Narimatsu, and Keisuke Fukumura for the experimental support.
The Supplementary Material for this article can be found online at: https://www.frontiersin.org/articles/10.3389/fphys.2021.747473/full#supplementary-material
Abo Ghanima, M. M., Alagawany, M., Abd El-Hack, M. E., Taha, A., Elnesr, S. S., Ajarem, J., et al. (2020). Consequences of various housing systems and dietary supplementation of thymol, carvacrol, and euganol on performance, egg quality, blood chemistry, and antioxidant parameters. Poult. Sci. 99, 4384–4397. doi: 10.1016/j.psj.2020.05.028
Alagawany, M., Elnesr, S. S., Farag, M. R., El-Sabrout, K., Alqaisi, O., Dawood, M. A., et al. (2021a). Nutritional significance and health benefits of omega-3, -6 and-9 fatty acids in animals. Anim. Biotechnol. doi: 10.1080/10495398.2020.1869562 [Epub ahead of print].
Alagawany, M., Elnesr, S. S., Farag, M. R., Abd El-Hack, M. E., Barkat, R. A., Gabr, A. A., et al. (2021b). Potential role of important nutraceuticals in poultry performance and health-A comprehensive review. Res. Vet. Sci. 137, 9–29. doi: 10.1016/j.rvsc.2021.04.009
Batool, F., Bilal, R. M., Hassan, F. U., Nasir, T. A., Rafeeque, M., Elnesr, S. S., et al. (2021). An updated review on behavior of domestic quail with reference to the negative effect of heat stress. Anim. Biotechnol. doi: 10.1080/10495398.2021.1951281 [Epub ahead of print].
Beauclair, L., Ramé, C., Arensburger, P., Piégu, B., Guillou, F., Dupont, J., et al. (2019). Sequence properties of certain GC rich avian genes, their origins and absence from genome assemblies: case studies. BMC Genomics 20:734 doi: 10.1186/s12864-019-6131-1
Bole-Feysot, C., Goffin, V., Edery, M., Binart, N., and Kelly, P. A. (1998). Prolactin (PRL) and its receptor: actions, signal transduction pathways and phenotypes observed in PRL receptor knockout mice. Endocr. Rev. 19, 225–268. doi: 10.1210/edrv.19.3.0334
Buettner, C., Muse, E. D., Cheng, A., Chen, L., Scherer, T., Pocai, A., et al. (2008). Leptin controls adipose tissue lipogenesis via central, STAT3-independent mechanisms. Nat. Med. 14, 667–675. doi: 10.1038/nm1775
Cherian, G. (2015). Nutrition and metabolism in poultry: role of lipids in early diet. J. Anim. Sci. Biotechnol. 6:28 doi: 10.1186/s40104-015-0029-9
Das, K. (1991). Effects of testosterone propionate, prolactin and photoperiod on feeding behaviours of Indian male weaver birds. Indian J. Exp. Biol. 29, 1104–1108.
Dupont, J., Tesseraud, S., and Simon, J. (2008). Insulin signaling in chicken liver and muscle. Gen. Comp. Endocrinol. 163, 52–57. doi: 10.1016/j.ygcen.2008.10.016
Friedman-Einat, M., and Seroussi, E. (2019). Avian leptin: bird’s-eye view of the evolution of vertebrate energy-balance control. Trends Endocrinol. Metab. 30, 819–832. doi: 10.1016/j.tem.2019.07.007
Fukumura, K., Shikano, K., Narimatsu, Y., Iwakoshi-Ukena, E., Furumitsu, M., Naito, M., et al. (2021). Effects of neurosecretory protein GL on food intake and fat accumulation under different dietary nutrient compositions in rats. Biosci. Biotechnol. Biochem. 85, 1514–1520. doi: 10.1093/bbb/zbab064
Garrison, M. M., and Scow, R. O. (1975). Effect of prolactin on lipoprotein lipase in crop sac and adipose tissue of pigeons. Am. J. Physiol. 228, 1542–1544. doi: 10.1152/ajplegacy.1975.228.5.1542
Geary, N. (2000). Control-theory models of body-weight regulation and body-weight-regulatory appetite. Appetite 144:104440. doi: 10.1016/j.appet.2019.104440
Gesta, S., Tseng, Y. H., and Kahn, C. R. (2007). Developmental origin of fat: tracking obesity to its source. Cell 131, 242–256. doi: 10.1016/j.cell.2007.10.004
Guglielmo, C. G. (2018). Obese super athletes: fat-fueled migration in birds and bats. J. Exp. Biol. 221(Pt Suppl 1):jeb165753. doi: 10.1242/jeb.165753
Hermier, D. (1997). Lipoprotein metabolism and fattening in poultry. J. Nutr. 127, 805–808. doi: 10.1093/jn/127.5.805S
Halaas, J. L., Gajiwala, K. S., Maffei, M., Cohen, S. L., Chait, B. T., Rabinowitz, D., et al. (1995). Weight-reducing effects of the plasma protein encoded by the obese gene. Science 269, 543–546. doi: 10.1126/science.7624777
Iwakoshi-Ukena, E., Shikano, K., Kondo, K., Taniuchi, S., Furumitsu, M., Ochi, Y., et al. (2017). Neurosecretory protein GL stimulates food intake, de novo lipogenesis, and onset of obesity. eLife 6:e28527. doi: 10.7554/eLife.28527
Johnston, D. W. (1971). The absence of brown adipose tissue in birds. Comp. Biochem. Physiol. A Comp. Physiol. 40, 1107–1108 doi: 10.1016/0300-9629(71)90298-2
Knowles, T. G., Kestin, S. C., Haslam, S. M., Brown, S. N., Green, L. E., Butterworth, A., et al. (2008). Leg disorders in broiler chickens: prevalence, risk factors and prevention. PLoS One 3:e1545. doi: 10.1371/journal.pone.0001545
Lawrence, C. B., Turnbull, A. V., and Rothwell, N. J. (1999). Hypothalamic control of feeding. Curr. Opin. Neurobiol. 6, 778–783. doi: 10.1016/S0959-4388(99)00032-X
Leveille, G. A., Romsos, D. R., Yeh, Y., and O’Hea, E. K. (1975). Lipid biosynthesis in the chick. A consideration of site of synthesis, influence of diet and possible regulatory mechanisms. Poult. Sci. 54, 1075–1093. doi: 10.3382/ps.0541075
Litt, J., Leterrier, C., Savietto, D., and Fortun-Lamothe, L. (2020). Influence of dietary strategy on progression of health and behaviour in mule ducks reared for fatty liver production. Animal 14, 1258–1269. doi: 10.1017/S1751731119003367
March, J. B., Sharp, P. J., Wilson, P. W., and Sang, H. M. (1994). Effect of active immunization against recombinant-derived chicken prolactin fusion protein on the onset of broodiness and photoinduced egg laying in bantam hens. J. Reprod. Fertil. 101, 227–233. doi: 10.1530/jrf.0.1010227
Masuda, K., Ooyama, H., Shikano, K., Kondo, K., Furumitsu, M., Iwakoshi-Ukena, E., et al. (2015). Microwave-assisted solid-phase peptide synthesis of neurosecretory protein GL composed of 80 amino acid residues. J. Pept. Sci. 21, 454–460. doi: 10.1002/psc.2756
Matsuura, D., Shikano, K., Saito, T., Iwakoshi-Ukena, E., Furumitsu, M., Ochi, Y., et al. (2017). Neurosecretory protein GL, a hypothalamic small secretory protein, participates in energy homeostasis in male mice. Endocrinology 158, 1120–1129. doi: 10.1210/en.2017-00064
Moore, J. B., Gunn, P. J., and Fielding, B. A. (2014). The role of dietary sugars and de novo lipogenesis in non-alcoholic fatty liver disease. Nutrients 6, 5679–5703. doi: 10.3390/nu6125679
Peirce, V., Carobbio, S., and Vidal-Puig, A. (2014). The different shades of fat. Nature 510, 76–83. doi: 10.1038/nature13477
Peña-Saldarriaga, L. M., Fernández-López, J., and Pérez-Alvarez, J. A. (2020). Quality of chicken fat by-products: lipid profile and colour properties. Foods 9:1046. doi: 10.3390/foods9081046
Price, E. R. (2010). Dietary lipid composition and avian migratory flight performance: development of a theoretical framework for avian fat storage. Comp. Biochem. Physiol. A Mol. Integr. Physiol. 157, 297–309. doi: 10.1016/j.cbpa.2010.05.019
Rehman, A., Arif, M., Sajjad, N., Al-Ghadi, M. Q., Alagawany, M., Abd El-Hack, M. E., et al. (2020). Dietary effect of probiotics and prebiotics on broiler performance, carcass, and immunity. Poult. Sci. 99, 6946–6953. doi: 10.1016/j.psj.2020.09.043
Rich, N., Reyes, P., Reap, L., Goswami, R., and Fraley, G. S. (2007). Sex differences in the effect of prepubertal GALP infusion on growth, metabolism and LH secretion. Physiol. Behav. 92, 814–823. doi: 10.1016/j.physbeh.2007.06.003
Scanes, C. G., Peterla, T. A., Campbell, R. M. (1994). Influence of adenosine or adrenergic agonists on growth hormone stimulated lipolysis by chicken adipose tissue in vitro. Comp. Biochem. Physiol. Pharmacol. Toxicol. Endocrinol. 107, 243–248. doi: 10.1016/1367-8280(94)90047-7
Scherer, T., O’Hare, J., Diggs-Andrews, K., Schweiger, M., Cheng, B., Lindtner, C., et al. (2011). Brain insulin controls adipose tissue lipolysis and lipogenesis. Cell. Metab. 13, 183–194. doi: 10.1016/j.cmet.2011.01.008
Seroussi, E., Knytl, M., Pitel, F., Elleder, D., Krylov, V., Leroux, S., et al. (2019). Avian expression patterns and genomic mapping implicate leptin in digestion and TNF in immunity, suggesting that their interacting adipokine role has been acquired only in mammals. Int. J. Mol. Sci. 20:4489. doi: 10.3390/ijms20184489
Seroussi, E., Pitel, F., Leroux, S., Morisson, M., Bornelöv, S., Miyara, S., et al. (2017). Mapping of leptin and its syntenic genes to chicken chromosome 1p. BMC. Genet. 18:77. doi: 10.1186/s12863-017-0543-1
Shikano, K., Bessho, Y., Kato, M., Iwakoshi-Ukena, E., Taniuchi, S., Furumitsu, M., et al. (2018a). Localization and function of neurosecretory protein GM, a novel small secretory protein, in the chicken hypothalamus. Sci. Rep. 8:704. doi: 10.1038/s41598-018-24103-w
Shikano, K., Taniuchi, S., Iwakoshi-Ukena, E., Furumitsu, M., Bentley, G. E., Kriegsfeld, L. J., et al. (2018c). Chronic subcutaneous infusion of neurosecretory protein GM increases body mass gain in chicks. Gen. Comp. Endocrinol. 265, 71–76. doi: 10.1016/j.ygcen.2017.11.010
Shikano, K., Kato, M., Iwakoshi-Ukena, E., Furumitsu, M., Matsuura, D., Masuda, K., et al. (2018b). Effects of chronic intracerebroventricular infusion of neurosecretory protein GL on body mass and food and water intake in chicks. Gen. Comp. Endocrinol. 256, 37–42. doi: 10.1016/j.ygcen.2017.05.016
Shikano, K., Iwakoshi-Ukena, E., Kato, M., Furumitsu, M., Bentley, G. E., Kriegsfeld, L. J., et al. (2019). Neurosecretory protein GL induces fat accumulation in chicks. Front. Endocrinol. 10:392. doi: 10.3389/fendo.2019.00392
Shikano, K., Iwakoshi-Ukena, E., Saito, T., Narimatsu, Y., Kadota, A., Furumitsu, M., et al. (2020). Neurosecretory protein GL induces fat accumulation in mice. J. Endocrinol. 244, 1–12. doi: 10.1530/JOE-19-0112
Stoyanovitch, A. G., Johnson, M. A., Clifton, D. K., Steiner, R. A., and Fraley, G. S. (2005). Galanin-like peptide rescues reproductive function in the diabetic rat. Diabetes 54, 2471–2476. doi: 10.2337/diabetes.54.8.2471
Tokushima, Y., Takahashi, K., Sato, K., and Akiba, Y. (2005). Glucose uptake in vivo in skeletal muscles of insulin-injected chicks. Comp. Biochem. Physiol. B Biochem. Mol. Biol. 141, 43–48. doi: 10.1016/j.cbpc.2005.01.008
Trott, K. A., Giannitti, F., Rimoldi, G., Hill, A., Woods, L., Barr, B., et al. (2014). Fatty liver hemorrhagic syndrome in the backyard chicken. Vet. Pathol. 51, 787–795. doi: 10.1177/0300985813503569
Ukena, K. (2018). Avian and murine neurosecretory protein GL participates in the regulation of feeding and energy metabolism. Gen. Comp. Endocrinol. 260, 164–170. doi: 10.1016/j.ygcen.2017.09.019
Ukena, K., Iwakoshi-Ukena, E., Taniuchi, S., Bessho, Y., Maejima, S., Masuda, K., et al. (2014). Identification of a cDNA encoding a novel small secretory protein, neurosecretory protein GL, in the chicken hypothalamic infundibulum. Biochem. Biophys. Res. Commun. 446, 298–303. doi: 10.1016/j.bbrc.2014.02.090
Wang, G., Kim, W. K., Cline, M. A., Gilbert, E. R. (2017). Factors affecting adipose tissue development in chickens: a review. Poult. Sci. 96, 3687–3699. doi: 10.3382/ps/pex184
Keywords: neurosecretory protein, chicken, hypothalamus, fat deposition, chronic intracerebroventricular infusion
Citation: Kato M, Iwakoshi-Ukena E, Furumitsu M and Ukena K (2021) A Novel Hypothalamic Factor, Neurosecretory Protein GM, Causes Fat Deposition in Chicks. Front. Physiol. 12:747473. doi: 10.3389/fphys.2021.747473
Received: 26 July 2021; Accepted: 04 October 2021;
Published: 25 October 2021.
Edited by:
Sami Dridi, University of Arkansas, United StatesReviewed by:
Brian McCabe, University of Cambridge, United KingdomCopyright © 2021 Kato, Iwakoshi-Ukena, Furumitsu and Ukena. This is an open-access article distributed under the terms of the Creative Commons Attribution License (CC BY). The use, distribution or reproduction in other forums is permitted, provided the original author(s) and the copyright owner(s) are credited and that the original publication in this journal is cited, in accordance with accepted academic practice. No use, distribution or reproduction is permitted which does not comply with these terms.
*Correspondence: Kazuyoshi Ukena, dWtlbmFAaGlyb3NoaW1hLXUuYWMuanA=; Eiko Iwakoshi-Ukena, aXdha29zaGlAaGlyb3NoaW1hLXUuYWMuanA=
Disclaimer: All claims expressed in this article are solely those of the authors and do not necessarily represent those of their affiliated organizations, or those of the publisher, the editors and the reviewers. Any product that may be evaluated in this article or claim that may be made by its manufacturer is not guaranteed or endorsed by the publisher.
Research integrity at Frontiers
Learn more about the work of our research integrity team to safeguard the quality of each article we publish.