- 1Department of Biology, Faculty of Marine and Environmental Sciences, Instituto Universitario de Investigación Marina (INMAR), Campus de Excelencia Internacional del Mar (CEI·MAR), University of Cádiz, Cádiz, Spain
- 2Centre of Marine Sciences (CCMar), Universidade do Algarve, Campus de Gambelas, Faro, Portugal
- 3Instituto de Ciencias Marinas de Andalucía, Consejo Superior de Investigaciones Científicas (ICMAN-CSIC), Cádiz, Spain
Aflatoxin B1 (AFB1) is a mycotoxin often present in food. This study aimed to understand the physiological effects of AFB1 on the seabream (Sparus aurata) gastrointestinal system. In a first in vitro approach, we investigated ion transport using the short-circuit current (Isc) technique in Ussing chambers in the anterior intestine (AI). Application of apical/luminal AFB1 concentrations of 8 and 16 μM to healthy tissues was without effect on tissue transepithelial electrical resistance (TER), and apparent tissue permeability (Papp) was measured using fluorescein FITC (4 kD). However, it resulted in dose-related effects on Isc. In a second approach, seabream juveniles fed with different AFB1 concentrations (1 and 2 mg AFB1 kg−1 fish feed) for 85 days showed significantly reduced gill Na+/K+-ATPase (NKA) and H+-ATPase (HA) activities in the posterior intestine (PI). Moreover, dietary AFB1 modified Isc in the AI and PI, significantly affecting TER in the AI. To understand this effect on TER, we analyzed the expression of nine claudins and three occludins as markers of intestinal architecture and permeability using qPCR. Around 80% of the genes presented significantly different relative mRNA expression between AI and PI and had concomitant sensitivity to dietary AFB1. Based on the results of our in vitro, in vivo, and molecular approaches, we conclude that the effects of dietary AFB1 in the gastrointestinal system are at the base of the previously reported growth impairment caused by AFB1 in fish.
Introduction
Fish farming accounted in 2018 for 52% of the total world fish used for food, excluding China, with an estimated production of 82 million metric tons (FAO, 2020). These values have steadily increased over the last decades. However, the present world challenges, such as the decreasing and availability of wild fishery stocks (Tacon and Metian, 2008) and the ongoing climate change, have changed the production paradigm. Therefore, the aquaculture industry is switching to a more sustainable model. The first step to achieve this is the generalized use of plant-based aquafeeds and fish feed additives (Tacon et al., 2011; Daniel, 2018). While this seems the right strategy, it raises new concerns on feed quality, such as pesticide use, market competition, deforestation, and mycotoxins presence.
Mycotoxin contamination and proliferation in aquafeeds represent increasing fish farming concerns (Nácher-Mestre et al., 2015, 2018). Recent numbers estimate contamination by molds and fungi in 25% of the crops (Pandya and Arade, 2016). Some mold species produce mycotoxins (including aflatoxins) as secondary metabolites (Zhang et al., 2014). Liposoluble aflatoxins have low molecular weight circa ~0.7 kD (Zhang et al., 2014) and readily cross the skin, and the gastrointestinal epithelium (Ditta et al., 2019). Aflatoxin B1 (AFB1) is the most potent carcinogen and genotoxic among aflatoxins (Flores-flores et al., 2015; Ostry et al., 2017).
Previous studies focused on the dietary effects of AFB1 in farmed fish, including the gilthead seabream (Sparus aurata), showed primarily species-specific growth impairment (Santacroce et al., 2012; Gonçalves et al., 2018a,b; Barany et al., 2020). Despite the growth inhibition, there is a lack of apparent pathological signs, and the adverse impact of AFB1 usually goes unnoticed in farmed fish (Anater et al., 2020). Especially interesting is the absence of AFB1 in fish fillets or liver in the gilthead seabream or salmonids after chronic dietary feeding (Nácher-Mestre et al., 2018; Barany et al., 2020), which contrasts with the findings in other species (Huang et al., 2014; Bedoya-Serna et al., 2018) and makes this issue more disturbing. We suggest that the putative absence of AFB1 residue in edible flesh could be due to high AFB1-tolerance implicit in intrinsic-species metabolism (Huang et al., 2011; Deng et al., 2018a,b) or that derived AFB1 bioactive metabolites are responsible for its effects. Such could be the case for AFB1 Exo-8,9-epoxide metabolite (Guengerich et al., 1998), for which commercial detection tests are yet undeveloped.
Anti-nutritional factors present in feeds or the environment (e.g., natural substances or toxicants) impact digestion, the intermediary metabolism, and cause inflammation (Kiron et al., 2020). Many types of anti-nutrient compounds appear in aquafeeds, especially in plant protein-rich feeds. Fermentation, heat treatments, antioxidants inclusion, and clay sequestrants are some methods used for anti-nutrient removal (Estensoro et al., 2016; Chakraborty et al., 2019). The effectiveness of the methods is dependent on the anti-nutritional compound nature. Specifically, several sequestrants or additives have been tested with aflatoxins to counteract their adverse effects (Rodrigues et al., 2019; de Freitas Souza et al., 2020).
Recent studies have pointed to the intestinal epithelium as a potential target of the actions of mycotoxins in humans, specifically in epithelial integrity (Akbari et al., 2017). Intestinal epithelia display two distinctive features: polarity across membranes and tightness among epithelial cells. The asymmetric distribution of proteins that regulate ion movements across the apical and basolateral membranes generates polarity. In contrast, tight junctions (TJs), which are multiprotein junctional complexes, prevent cell-to-cell leakage (Shen et al., 2011) and determine epithelial integrity and selectivity. The TJs permeability dynamic is mediated by claudins and occludins, among other elements (Mukendi et al., 2016). Both integral membrane proteins are the major structural and functional proteins within TJs to form the intercellular seal and permeability control (Claude, 1978; van Itallie and Anderson, 2006, 2013; Zhang and Guo, 2009).
According to their function, claudins are classified as barrier claudins that reduce permeability or pore claudins that promote selective ion permeability (van Itallie and Anderson, 2006; Hou et al., 2013). They can also interact with intracellular signaling molecules, linking extracellular transport, and intracellular signaling events (Collins et al., 2017). More than 63 genes encoding claudins exist in teleost fish (Kolosov et al., 2013; Lu et al., 2013). Of those, 30 claudins show some expression level in the gastrointestinal tract (GIT), but there is no evidence of their function (Tipsmark et al., 2010). The role of occludins is linked to ion selectivity and permeability, based on morphological, physiological, and mRNA expression results on studies performed in frog urinary bladder and in weaning piglet intestine (Claude, 1978; Zhang and Guo, 2009). Overall, information on occludins distribution and physiology is scarce at best.
Our recent work in seabream (Sparus aurata) showed that chronic ingestion of AFTB1 impairs growth and metabolism (Barany et al., 2020) as well as the physiological responses to additional stressors, such as crowding densities (Barany et al., 2021). In this line, we hypothesized that the gastrointestinal system is at the base of the previously reported growth impairment caused by AFB1 in fish since it establishes the first contact with food and its components. Therefore, this study aimed to characterize the direct effects of AFB1 on intestinal physiology by 1) comparing electrophysiological properties in vivo vs. in vitro expositions (i.e., short vs. long-term); 2) the morphological modifications caused in vivo after long-term exposure; and 3) the mRNA expression alterations in situ on putative proteins that keep cell-to-cell adhesion along with the gastrointestinal architecture.
Materials and Methods
Animals
Seabream juveniles (Sparus aurata, 100–200 g body mass) were obtained from the local stock of Ramalhete Marine Station (CCMar, Faro, Portugal) for the in vitro experiments or purchased from CUPIBAR SL, Cádiz, Spain for the in vivo experiments.
Series 1: AFB1 in Control Tissue in vitro
This series of experiments intended to clarify if the effect of AFB1 on the intestine was immediate or required of chronic exposure. Tissues from control fish (not exposed previously to AFB1) were collected and mounted in Ussing chambers (see below). The concentrations of AFB1 used (8 and 16 μM) were based on estimations of minimum and maximum presence of this compound in vivo that would leach from the feeding experiments. For these estimations, fish size, food ration, luminal fluid volume, and the content of AFB1 in the feeds were taken into account (Gilannejad et al., 2019; Barany et al., 2020).
Short-Circuit Current (Isc)
After anesthesia (1 mL 2-phenoxyethanol/L seawater) and decapitation, the anterior intestine was collected fresh, isolated, and mounted in Ussing chambers, as previously described (Gregorio et al., 2012). Tissues were open longitudinally, flattened, placed on a tissue holder of 0.25 cm2, and positioned between two half-chambers containing 2 mL of serosal physiological saline (e.g., symmetrical conditions) formulated for seabream (Fuentes et al., 2006), in mM: 160 NaCl, 1 MgSO4, 2 NaH2PO4, 1.5 CaCl2, 5 NaHCO3, 3 KCl, 5.5 Glucose, Hepes 5 mM; 300 mOsm/kg H2O and pH to 7.80). In vitro, the saline received a gas mixture of 99.7% O2 + 0.3% CO2, and the temperature was kept constant at 18°C according to the temperature maintained in the tanks with the fish when alive.
In vitro preparations were left undisturbed until reaching a steady state. After 1 h, enough AFB1 was sequentially added at 1-h intervals to give a final concentration of 8 and 16 μM, respectively. AFB1 was added apically to simulate leaching from food. Transepithelial electric resistance (TER, Ω cm2) calculations used the current deflections induced by a ± 1 mV pulse of 3 s every minute. Voltage clamping and current injections were performed using epithelial amplifiers VCC600 (Physiologic Instruments, San Diego, United States). All data were recorded onto a computer using a Lab-Trax-4 acquisition system (World Precision Instruments, Sarasota, FL, United States) using LabScribe3 (iWorx Systems Inc., Dover, NH, United States).
Intestinal Permeability
After tissue stabilization in vitro, saline solutions were replaced with new well-gassed solutions. Enough Fluorescein isothiocyanate–dextran (FITC, average mol wt ~4,000, Sigma, Madrid) prepared as concentrated stocks of 100 mg/mL were added to final concentrations of 0.5 mg/mL to the apical chamber. A sample (0.2 mL) was collected from both the apical and the basolateral compartments at time zero. Note that previously chambers were let for 15 min mixing with the oxygen bubbling in order to homogenize the serosal saline and the added FITC. New samples from both the donor and receiver compartments were collected into fresh vials after a 1-h control period (starting from time zero) and the subsequent 1-h periods in the presence of apical AFB1 (8 and 16 μM). The samples were stored at −20°C until analysis.
Fluoresce measurements were performed using a Multi-Mode Microplate Reader BioTek Synergy™ 4 (BioTek® Instruments, Winooski, VT, United States) set for excitation wavelength at 492 nm and emission wavelength at 520 nm for FITC. Standard concentrations were between 0.2 and 2000 ng/mL to calculate concentrations in both the apical and basolateral chambers. The apparent permeability (Papp) was estimated using the equation (Arnold et al., 2019): Papp = (dC/dT) x (V/A*C0) where Papp is the permeability in centimeters per second; dC/dT is the rate of concentration change (in ng/s) of FITC in the receiving chamber (basolateral) calculated from the slope of the concentration-time curve between 15 and 75 min; V is the volume of the receiver chamber in mL; A is the surface area of the tissue in square centimeter; and C0 is the starting concentration in the donor compartment (apical).
All animal experimentation was carried out in compliance with the European (Directive 2010/63/EU) and Portuguese legislation for the use of laboratory animals. All animal protocols were performed under Group-C licenses from the Direção-Geral de Alimentação e Veterinária, Ministério da Agricultura, do Mar, Ambiente e Ordenamento do Território, Portugal.
Series 2: in vivo AFB1 Feeding
The influence of different dietary doses of AFB1 (1 and 2 mg AFB1 kg−1 fish feed) during 85 days on gilthead seabream (Sparus aurata) juveniles was previously assessed (Barany et al., 2020). Briefly, fish (176.79 ± 2.52 g body mass and 19.16 ± 0.09 cm furcal length) were acclimated to our facility at Servicio Central de Investigación en Cultivos Marinos (SCI-CM, CASEM, University of Cadiz, Spain; Operational Code REGA ES11028000312). Fish were homogenously distributed, in triplicates, in nine 500 L-fiber glass tanks continuously aerated in an open circuit flow-through seawater system. In each tank, the number of individuals was adjusted to achieve the same experimental density (4 g L−1; Barany et al., 2020, 2021) and number (n = 30) in the three experimental groups. Fish were fed for 85 days with: (i) fish feed without AFB1 (CT); (ii) 1 mg AFB1 kg−1 fish feed (D1); and (iii) 2 mg AFB1 kg−1 fish feed (D2). Parameters of the water, such as oxygen concentration, pH, and salinity, were analyzed daily (data not shown), and a 33% tank water was replenished daily to ensure good water quality. Fish were fed three times a day (distributed throughout the daylight) at 1.5% of their body mass using Eheim 3,581 Feed-Air digital automatic feeders (EHEIM GmbH & Co KG, Germany). All the experimental procedures followed the guidelines of the University of Cádiz (Spain) and the European Union (Directive 2010/63/EU) for the use of animals in research which were also previously approved (Junta de Andalucía reference number 28-04-15-241) by the Ethics and Animal Welfare Committee from the Spanish Government (RD53/2013).
Experimental Fish Feed
A commercial fish feed (Skretting, Burgos, Spain) was used as a basis to prepare the experimental diets to supply all essential nutritional requirements for the gilthead seabream (57% crude protein, 18% crude fat, 10% ash, 1.6% phosphorus, and 19.5 MJ kg−1 digestible energy). The inclusion of AFB1 (Sigma A6636) at levels of 0 (CT), 1 (D1), and 2 (D2) mg kg−1 fish feed was performed after grounding and re-pelleting. Prof. Francisco Javier Moyano’s Research group, from the University of Almería, made the experimental aquafeeds by extrusion at the Experimental Feed Service facilities.
Sampling Protocol
Fish were euthanized under anesthesia of 1 mL 2-phenoxyethanol L−1 seawater and sampled. Blood was collected from the caudal peduncle into 1 mL syringes rinsed with a solution containing 8,000 Units mL−1 0.9% NaCl (heparin ammonium salt, Sigma H6279) then fish were beheaded. Plasma was obtained by blood centrifugation (10,000 g, 3 min at 4°C), frozen in liquid nitrogen, and stored at −80°C until analysis. The second gill arch from both dorsal sides was excised, adherent blood was removed by blotting with absorbent paper, and biopsies of each branchial arch, consisting of a few filaments, were cut using a surgical blade.
Biopsies of intestinal mucosa were collected with fine-point scissors. Two sections of the intestine were distinguished: (1) anterior intestine, corresponding to 2–3 cm caudal to the stomach, and (2) the posterior intestine, corresponding to a section of 3–4 cm in length of distal intestine, delimited in the end by the rectal sphincter. Gill and intestinal biopsies were placed in ice-cold sucrose-EDTA-imidazole (SEI) buffer (150 mM sucrose, 10 mM EDTA, 50 mM imidazole, pH 7.3) and frozen in liquid nitrogen to estimate ATPase activity. Representative biopsies of the anterior intestine and posterior intestine were placed in RNAlater (Ambion®, Applied BioSystems). These samples were kept for 24 h at 4°C and then stored at −20°C until RNA isolation. Control and experimental groups were randomly sampled from tanks at random time points during each experiment to minimize tank effects.
Osmolality and Sodium
Plasma osmolality was measured in 20 μl samples with a Vapro 5,520 Osmometer (Wescor, United States). Sodium was measured by a flame photometer (BWB-XP Performance Plus, BWB Technologies, United Kingdom).
ATPases Activities
Gill and intestinal NKA and HA activities were analyzed using an NADH-linked kinetic assay in a 96-well microplate run at 25°C for 10 min, as described in McCormick (1993). Frozen tissues were homogenized on ice-cold SEID (0.1% sodium deoxycholate in SEI buffer, pH = 7.3) and centrifuged at 3,200 g for 5 min at 4°C. The supernatant was assayed for ATPase activity in the presence and absence of the NKA-specific inhibitor ouabain (0.5 mM) and HA-specific inhibitor bafilomycin A1 (100 nM), as previously described (Ruiz-Jarabo et al., 2017). NADH oxidation was determined spectrophotometrically at 340 nm. The difference in kinetics between the inhibited and uninhibited assay mixtures was used to calculate NKA and HA-specific (ouabain/bafilomycin A1-sensitive). The activity was expressed as μmol ADP/mg protein/h. Total protein was measured using the bicinchoninic acid protein assay (BCA) with a bovine serum albumin (BSA) standard (Thermo Scientific, Rockford, IL, United States). The assay was run on an automated microplate reader (PowerWave 340, BioTek Instrument Inc., Winooski, United States) controlled by KCjunior™ software.
Short-Circuit Current (Isc)
On sampling days, the anterior and posterior intestine were collected fresh, isolated, and mounted in Ussing chambers, as described for Series 1. Tissues were left undisturbed until Isc readings achieved a steady state, usually ~30 min after mounting. The transepithelial potential difference (TEP, in mV) was referenced to the apical side. Short-circuit current (Isc, μAmp/cm2) was monitored by clamping epithelia to 0 mV and expressed as negative for the absorption of anions. Transepithelial electric resistance (TER, Ω cm2) was calculated using the current deflections induced by a + 1 mV pulse of 3 s every minute using Ohm’s law. Voltage clamping and current injections were performed using epithelial amplifiers DVC1000 (World Precision Instruments, Sarasota, FL, United States). All data were recorded onto a computer using a Lab-Trax-4 acquisition system (World Precision Instruments, Sarasota, FL, United States) using LabScribe3 (iWorx Systems Inc., Dover, NH, United States).
RNA Isolation and Quantitative Real-Time PCR
Biopsies from the anterior and posterior intestines were individually processed. Tissues were homogenized using an Ultra-Turrax® T25 with an S25N-8G dispersing tool (IKA®-Werke), and total RNA was extracted using the NucleoSpin® RNA kits (Macherey Nagel) following the manufacturer instructions. Genomic DNA (gDNA) was removed via on-column DNase digestion at 37°C for 30 min using rDNase (RNase-free) included with the kits. RNA concentration was measured with a Qubit 2.0 fluorimeter and Qubit RNA BR assay kit (Life Technologies). RNA quality was assessed using a Bioanalyzer 2,100 with the RNA 6000 Nano kit (Agilent Technologies). Only those samples with an RNA integrity number greater than 8.0 were used in real-time quantitative PCR (qPCR). Total RNA (500 ng) from each sample was reverse-transcribed in a 20 μl reaction using the qScript™ cDNA synthesis kit (Quanta BioSciences) in a Mastercycler® proS (Eppendorf), as previously described by Mata-Sotres et al. (2016). A pool of cDNAs from all the samples for each tissue was used to construct calibration plots, using serial 1:10 dilutions from 10 ng to 100 fg, to assess qPCR linearity and efficiency as for use for inter-assay calibrations. RNase-free water (NTC) and RNA (NRT) were included in the analysis as control reactions to ensure the absence of primer-dimers and genomic DNA contaminations, respectively. For all primers pairs, amplification linearities (R2) and efficiencies ranged from 0.980–0.999 and 0.925–1.087, respectively. Reactions for qPCR were performed in triplicate with 10 ng of cDNA (estimated from the input of total RNA), forward and reverse primers for the named samples (optimum 200 or 400 nM each), and PerfeCTa™ SYBR® Green FastMix™ (Quanta BioSciences). Reactions were performed in a volume of 10 μl using Hard-Shell® Low-Profile Thin-Wall 96-Well Skirted PCR plates (Bio-Rad) covered with Microseal® B Adhesive Seals (Bio-Rad). Relative gene expressions were quantified in a CFX Connect™ Real-Time PCR Detection System under the control and analysis of CFX Manager™ software (Bio-Rad) using the ΔΔCT method (Livak and Schmittgen, 2001), corrected for efficiencies (Pfaffl, 2001). The amplification included an initial denaturation and polymerase activation step at 95°C for 10 min, followed by 40 cycles of denaturation for 15 s at 95°C, annealing, and extension at 60°C for 30 s. After amplification, individual melting curves from 60°C to 95°C (0.5°C every 5 s) were generated to confirm single amplicons and the absence of primer-dimer artifacts. Results were normalized to the geometric average expression of eef1a and actb (Vandesompele et al., 2002). The genes were selected due to their lower than 0.5 target stability M value and lower than 0.25 CVs (as indicated by BioRad CFX Manager Target Stability Value). Table 1 shows the primer sequences used for qPCR and the resulting amplicon sizes. Sequence confirmation of amplified fragments was performed by Sanger sequencing (CCMar Sequencing Facility). Claudin and occludin sequences were retrieved and identified from an in-house seabream intestinal transcriptome assembly (Fuentes Lab). Please refer to Supplementary Material for further details.
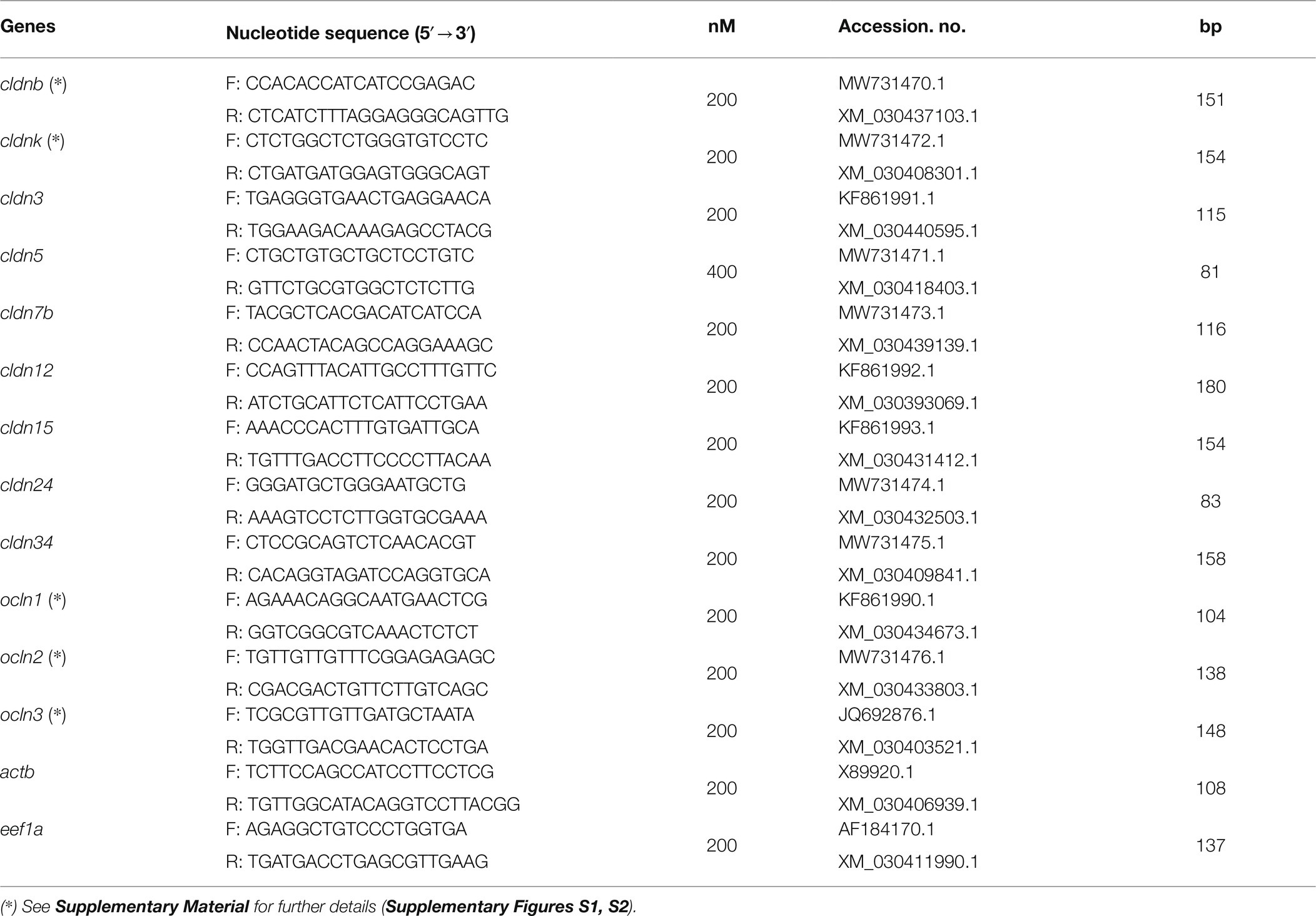
Table 1. Specific primers used for real-time qPCR expression analyses, primers final concentration (nM), GenBank accession numbers, and sizes of the amplified products in base pairs (bp).
Histopathological Analysis
Intestinal samples collected from the CT and D2 groups (n = 9) were used for the analysis. The histological tissue samples were fixed in phosphate-buffered saline at pH 7.2 containing formalin (10%). Then the preparations were washed in running tap water, dehydrated in alcohol, cleared in xylene, and embedded in paraffin wax. Sections (6 μm) were cut and mounted on gelatinized slides using a rotary microtome. Sections were rehydrated in distilled water and stained with hematoxylin/eosin (H&E). Prepared slides were then examined and photographed (Jenoptik ProgRes CT5) under a light microscope (Nikon eclipse Ci-L).
Chemicals
All chemicals were purchased from Sigma-Aldrich (Madrid, Spain). Ouabain (03125) and bafilomycin A1 (B1793) were prepared as concentrated stocks in imidazole buffer (50 mM). Concentrated stocks of aflatoxin B1, AFB1 (A6636), and fluorescein isothiocyanate-dextran, FITC (46945) were prepared in dimethyl sulfoxide (DMSO). When added to the Ussing chambers, the final volume of DMSO never exceeded 0.5% of the total volume of mucosal saline. DMSO did not show an effect on tested control tissues (data not shown).
Statistical Analysis
All data are presented as the mean ± standard error of the mean (SEM). Statistical comparison for all given results was performed using ANOVA, after assessing normality and equal variance using Shapiro–Wilk and D’Agostino-Pearson tests, respectively. For all data sets, outliers were identified by the ROUT method at Q = 1%. All ANOVA analyses were followed either by Tukey’s or Bonferroni post hoc tests to identify specific significant differences. All statistical analyses were performed with GraphPad Prism 6.0 (GraphPad Software, La Jolla, CA, United States), and significance for all tests was set at p < 0.05.
Results
Dietary AFB1 did not significantly change plasma osmolality or Na+ levels (Table 2).
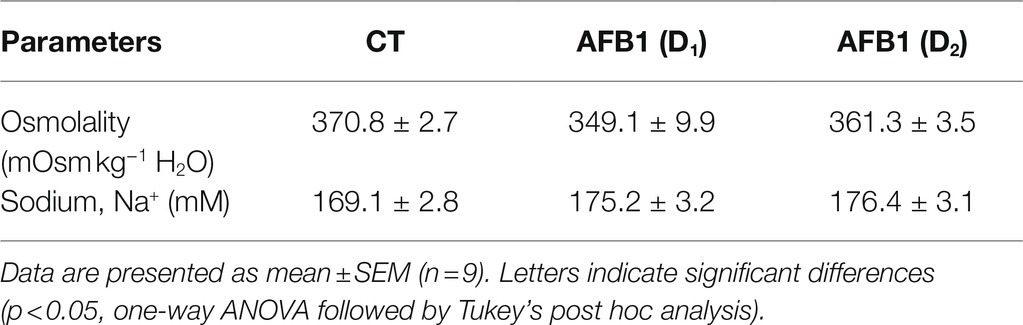
Table 2. Plasma osmolality and sodium in S. aurata juveniles fed with different experimental diets (CT, D1, and D2) for 85 days.
Gill and Intestinal ATPase
Gill NKA activity decreased significantly in the D2 group compared to CT and D1 groups (Figure 1A). In contrast, no significant differences were observed in NKA activity, neither in the anterior nor posterior intestine, although absolute activity values were lower in group D2 in the anterior intestine (Figure 1B). Additionally, HA activity showed no significant changes in the anterior intestine. However, this activity was significantly lower in the D2 group in the posterior intestine than in the control group or group D1 (Figure 1C).
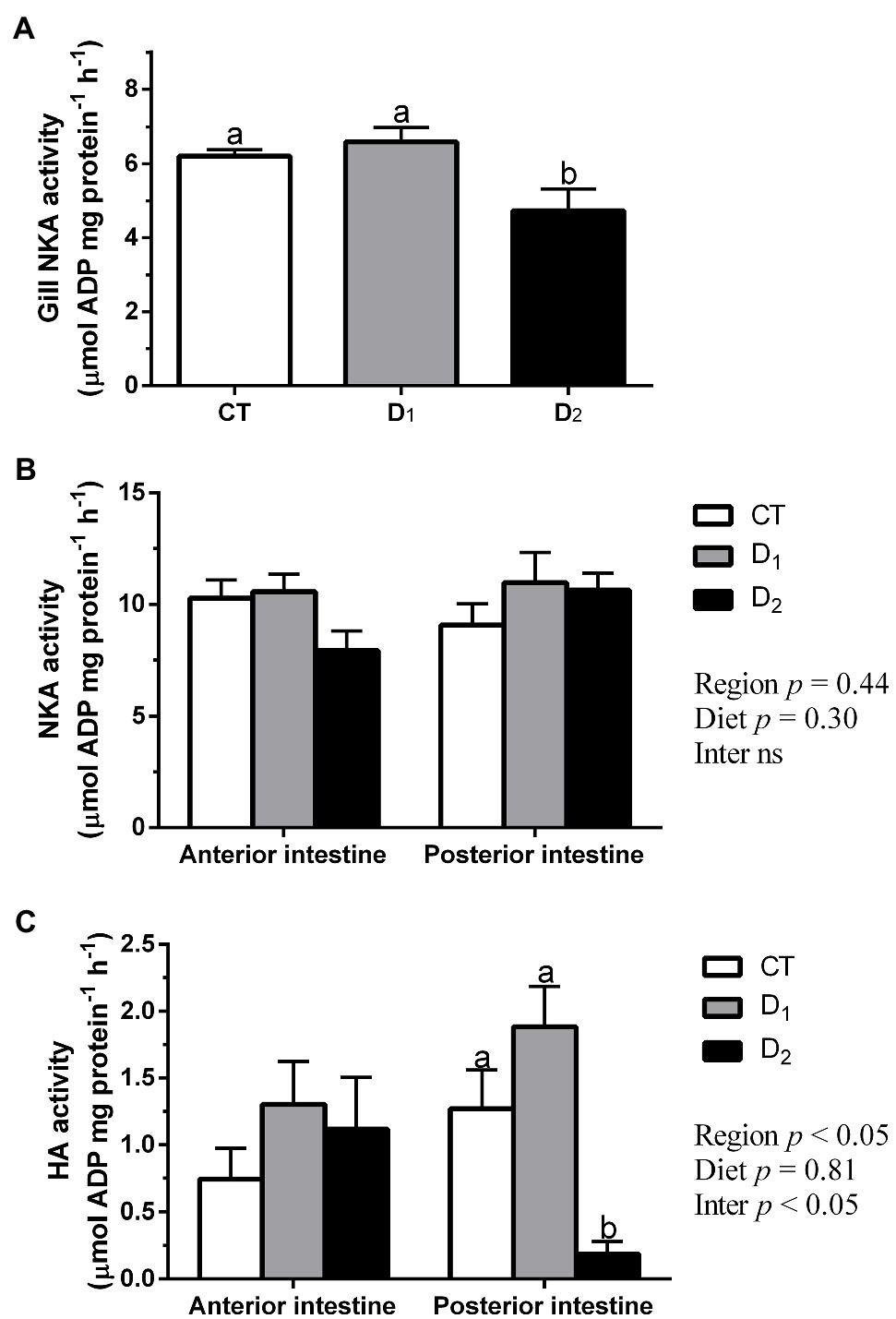
Figure 1. Gill NKA activity (A), intestinal NKA activity (B), and intestinal HA activity (C) in S. aurata juveniles fed with different experimental diets (CT, control; D1, 1 mg-; and D2, 2 mg AFB1 kg−1 fish feed) for 85 days. Data are presented as mean ± SEM (n = 6–10). Different letters indicate significant differences among the same intestinal regions. Abbreviation “Inter ns”: interaction not significant (p < 0.05, one or two-way ANOVA followed by Tukey’s post hoc analysis).
Dietary Effect of AFB1 on Intestinal Bioelectrical Properties
Series 1: AFB1 in Control Tissue in vitro
Under voltage clamp to 0 mV and symmetric conditions, the anterior intestine of the control seabream group mounted in Ussing chambers had an absorptive short-circuit current (Isc, μAmp cm−2) of −5.21 ± 1.59. However, after AFB1 (8 and 16 μM) was sequentially added at 1-h intervals, Isc became −4.45 ± 1.31 and −3.54 ± 1.26, respectively (Figure 2A). In contrast, neither the transepithelial electrical resistance (TER, Ω cm2) nor the apparent permeability (Papp) in the anterior intestine was significantly modified in any group in response to luminal addition of AFB1 (Figures 2B,C).
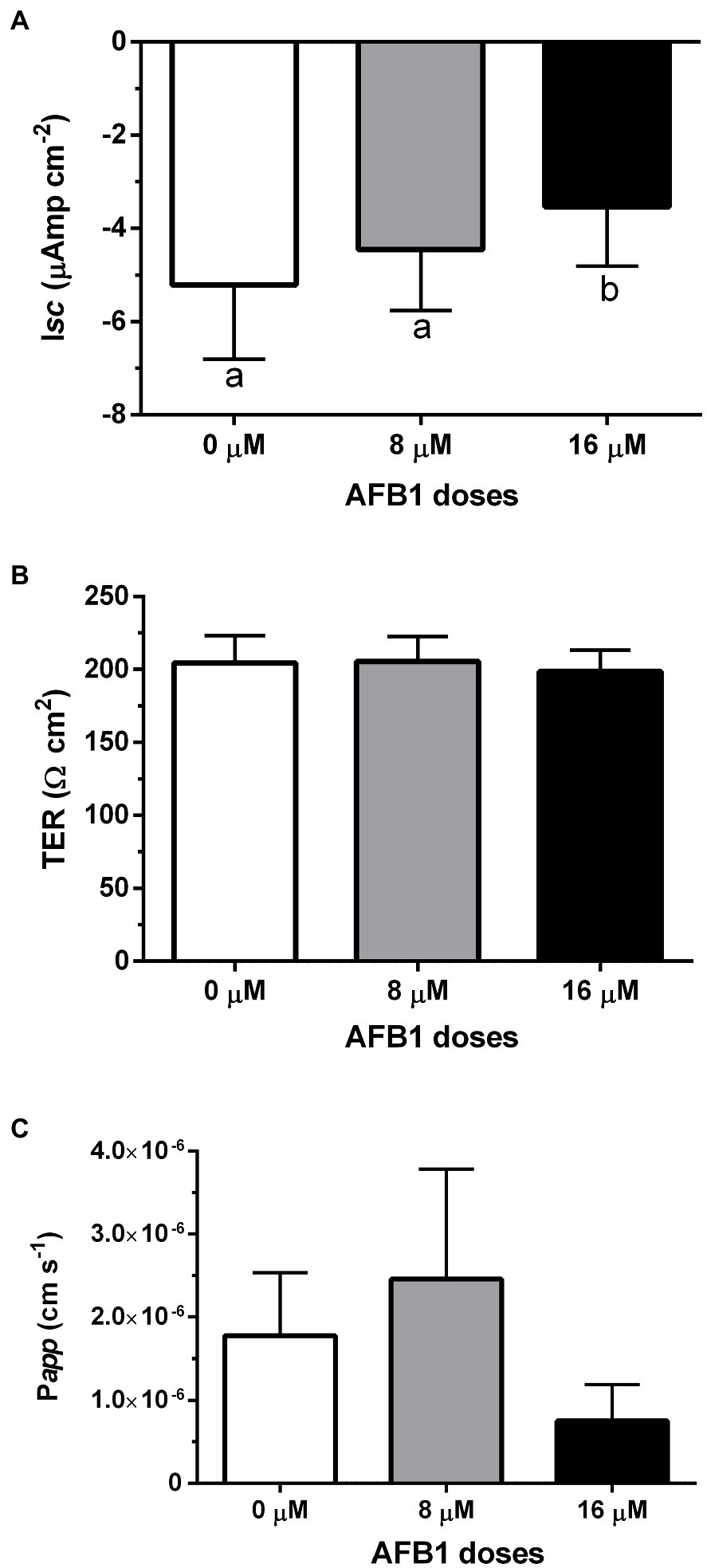
Figure 2. Isc (A), TER (B), and Papp (C) in response to increasing concentrations of luminal AFB1 (8 and 16 μM) in the anterior intestine of control S. aurata individuals mounted in Ussing chambers. Data are presented as mean ± SEM (n = 6; p < 0.05, matched-measures one-way ANOVA followed by Tukey’s post hoc analysis).
Series 2: in vivo AFB1 Feeding
The anterior intestine of the control seabream group mounted in Ussing chambers had, under voltage clamp to 0 mV and symmetric conditions, an absorptive short-circuit current (Isc, μAmp cm−2) of −0.56 ± 1.23, while in the posterior intestine, this Isc was secretory (+3.60 ± 1.78). In fish from group AFB1 (D2), Isc was −3.20 ± 1.00 in the anterior intestine and −0.45 ± 1.66 μAmp cm−2 in the posterior intestine (Figure 3A).
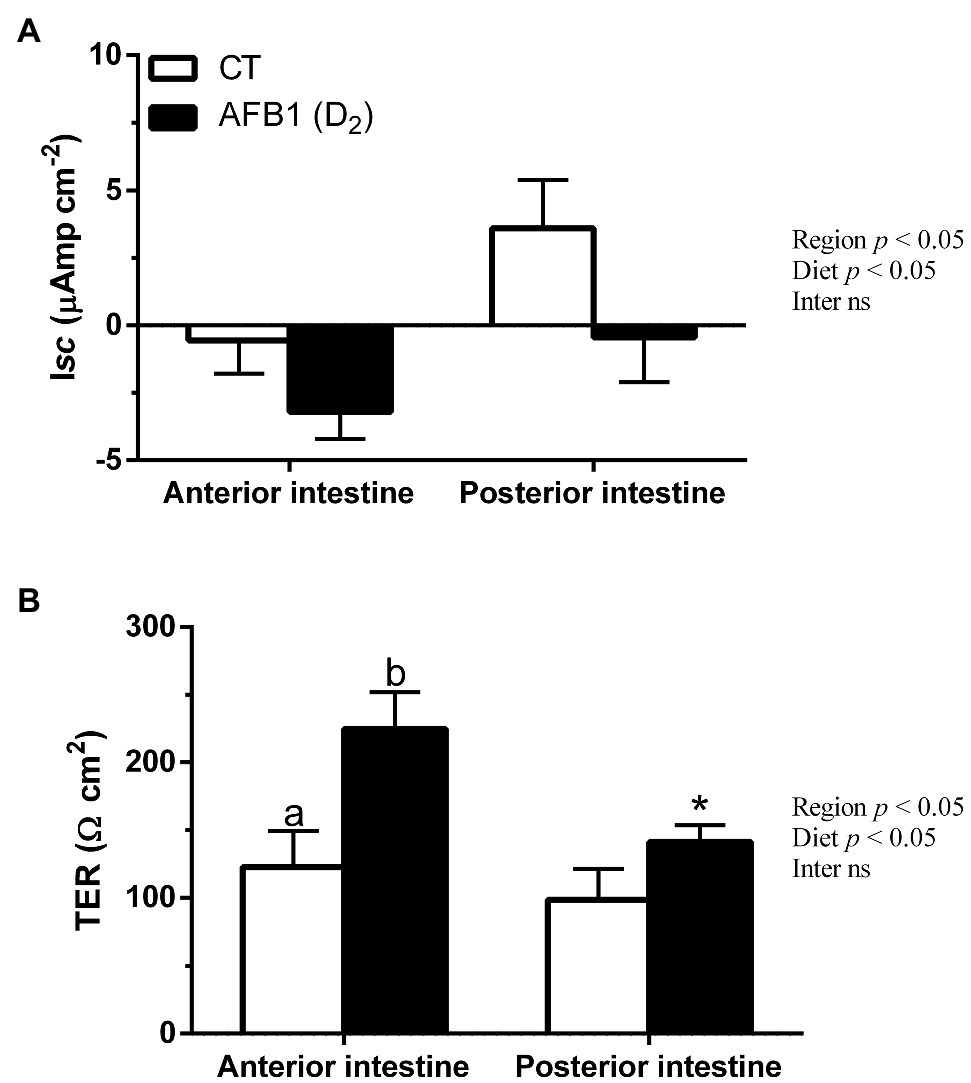
Figure 3. Isc (A) and TER (B) in anterior (AI) and posterior intestine (PI) of S. aurata juveniles fed with different experimental diets (CT, control; and D2, 2-mg AFB1 kg−1 fish feed) for 85 days. Data are presented as mean ± SEM (n = 8–11). For a given intestinal region, different letters indicate significant differences between experimental diets. Asterisks (*) indicate the significance level between different intestinal regions within the same experimental diet. Abbreviation “Inter ns”: interaction not significant (p < 0.05, two-way ANOVA followed by Tukey’s post hoc analysis).
Transepithelial electrical resistance (TER, Ω cm2) was ~123 Ω cm2 in the control seabream group’s anterior intestine and was ~99 Ω cm2 in the posterior intestine. However, the presence of AFB1 in the feed (group D2) significantly increased TER up to ~225 Ω cm2 in the anterior intestine while remained statistically unaffected in the posterior intestine (up to ~141 Ω cm2). Moreover, AFB1 in the feed highlighted significant differences between the anterior and posterior intestines (Figure 3B).
Gene Expression for Claudins and Occludins
The following claudins were amplified in the intestine of seabream: cldnb, cldnk, cldn3, cldn5, cldn7b, cldn12, cldn15, cldn24, and cldn34. Figure 4 shows the complete expression analysis of this set of claudins. Regional relative mRNA expression analysis of claudins in control fish revealed that five claudin genes out of 9 were significantly higher in anterior than posterior intestine, for example, cldn3, cldn5, cldn12, and cldn15. In contrast, only a single claudin of those analyzed, for example, cldn24, showed higher expression in posterior intestine, as no expression was detected in the anterior intestine (Figure 4H).
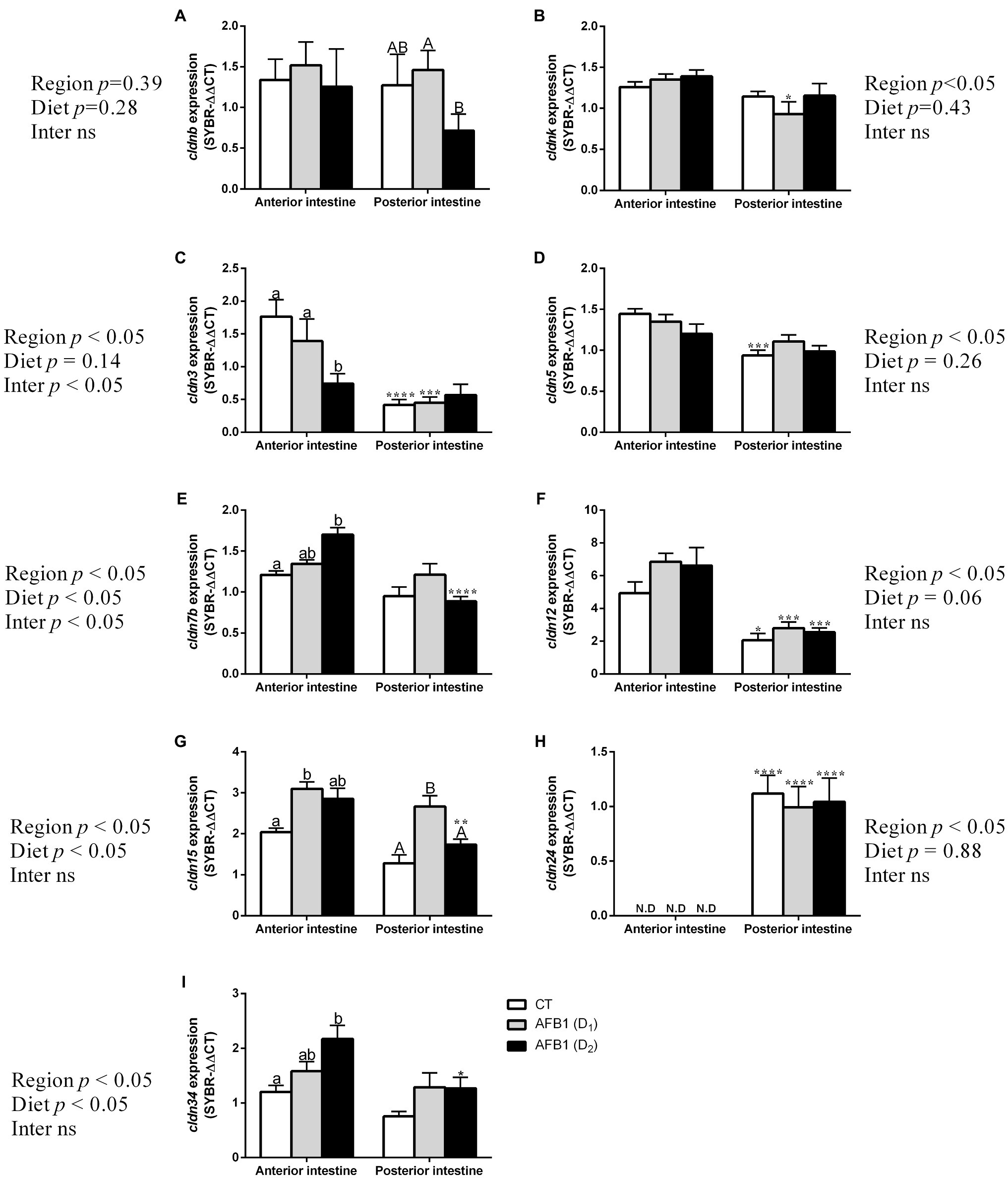
Figure 4. Gene expression of cldnb (A), cldnk (B), cldn3 (C), cldn5 (D), cldn7b (E), cldn12 (F), cldn15 (G), cldn24 (H), and cldn34 (I) in anterior (AI) and posterior intestine (PI) of S. aurata juveniles fed with different experimental diets (CT, control; D1, 1 mg- and D2, 2 mg-AFB1 kg−1 fish feed) for 85 days. Data are presented as mean ± SEM (n = 7–10). Different letters indicate significant differences among groups within the same intestinal region (lowercase letters: AI; capital letters: PI). Asterisks (*) indicate the significance level between different intestinal regions within the same experimental diet; Abbreviations, “ND”: not detected; “Inter ns”: interaction not significant (p < 0.05, two-way ANOVA followed by Tukey’s post hoc test).
AFB1 feeding induced several changes in claudin expression. Overall, the anterior intestine was more sensitive to dietary AFB1, and the effects included upregulation, downregulation, or no impact on relative claudin expression. Specifically, relative mRNA expression of cldnk, cldn5, cldn12, and cldn24 remained unchanged in response to dietary AFB1 both in the anterior or posterior intestine (Figure 4). Relative mRNA expression of cldn3 significantly decreased at the highest level of dietary AFB1 in the anterior but not in the posterior intestine (Figure 4C). Dietary AFB1 increased expression of cldn7b, cldn15, and cldn34 in the anterior intestine and downregulated mRNA expression of cldnb, cldn7b, and cldn15 in the posterior intestine but not in a concentration-dependent manner (Figures 4A,E,G,I).
Occludin expression, for example, ocln1, ocln2, and ocln3, were observed both in the anterior and posterior intestine of the seabream (Figure 5). There is no clear anterior–posterior pattern of expression in control fish, as seen for some claudins. However, dietary AFB1 induced several changes in occludin expression. In the anterior intestine, ocln1 expression was upregulated by AFB1 in a concentration-dependent manner, while in the posterior intestine showed an inverse U-shaped relationship with AFB1 doses (Figure 5A). However, the relative mRNA expression of ocln2 was insensitive to dietary AFB1, although there is a trend toward increased expression at the highest dose of AFB1 in the anterior intestine (Figure 5B). In contrast, mRNA expression of ocln3 was upregulated in anterior intestine but without significant changes in posterior intestine (Figure 5C).
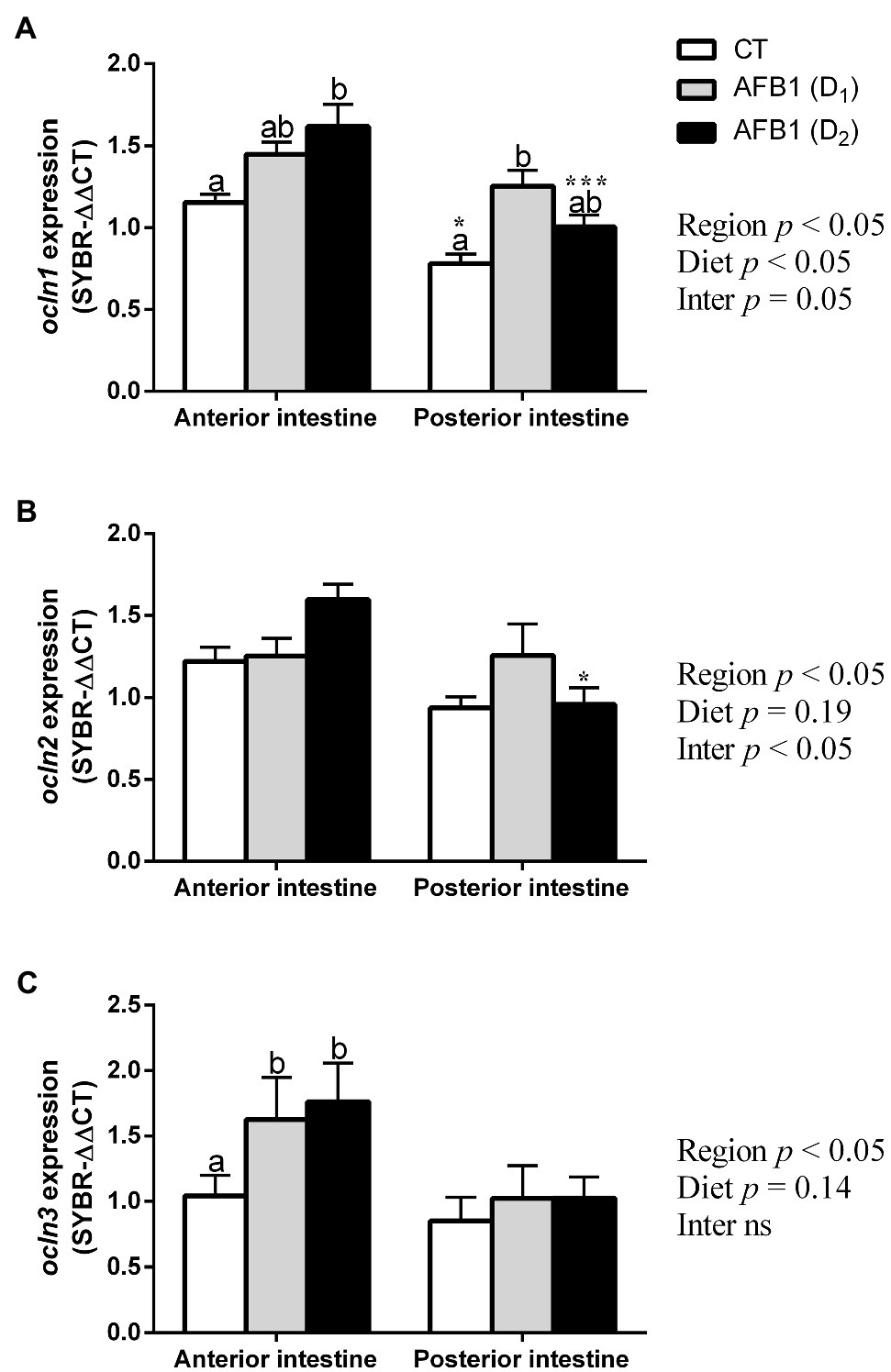
Figure 5. Gene expression of ocln1 (A), ocln2 (B), and ocln3 (C), in the anterior (AI) and posterior intestine (PI) of S. aurata juveniles fed with different experimental diets (CT, control; D1, 1 mg- and D2, 2 mg-AFB1 kg−1 fish feed) for 85 days. Data are presented as mean ± SEM (n = 8–10). Further details in legend Figure 4.
Histopathology
The microscopical examinations showed no apparent lesions in the control group (Figures 6A,B). However, the intestine of seabream with dietary exposure to AFB1 presented villi shortening, a diminishing or hypoplasia of goblet cells, and necrosis in the epithelial lining of the intestinal villi (Figures 6C,D). The microscopic examination of intestinal tissue also revealed villi sloughing with the presence of exudate in the intestinal lumen (Figure 6E), edema (Figure 6F), and infiltration of mononuclear cells in mucosa and submucosa layers (Figure 6G). Finally, separation of mucosa and submucosa layers with the development of subepithelial spaces was also observed (Figure 6H).
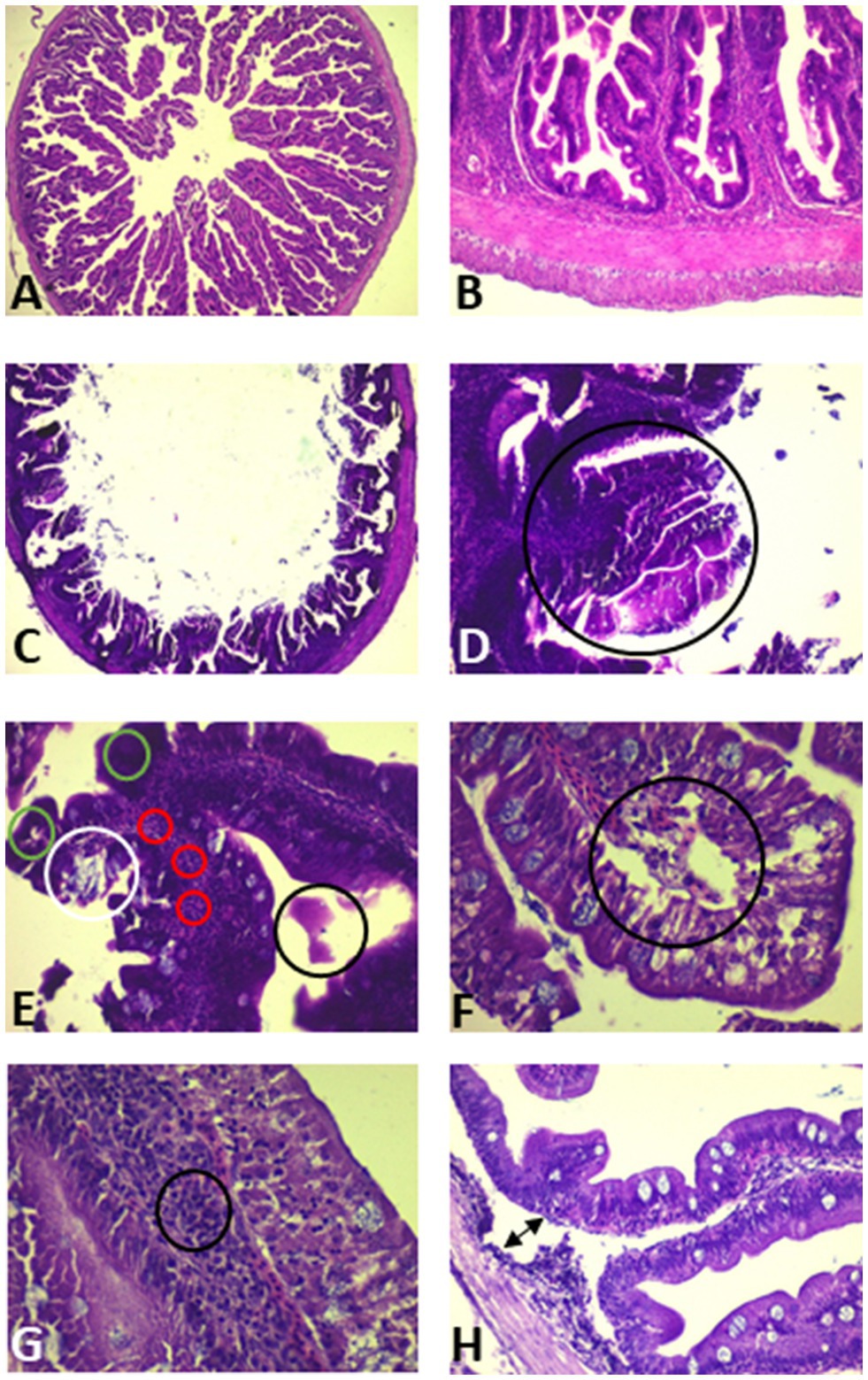
Figure 6. Photomicrograph of the anterior intestine (AI) of S. aurata juveniles fed with control diet (A,B) and with the highest dose of AFB1 (2 mg AFB1 kg−1 fish feed) for 85 days (C–H). (A) AI of the control group (H&E 2x). (B) AI showing different intestinal layers and villi in detail (H&E 10x). (C) AI of S. aurata juveniles exposed to AFB1 (H&E 2x). (D) AI showing in detail: necrosis, shortening of villi, and hypoplasia of goblet cells (GC; black circle; H&E 10x); (E) necrosis of epithelial villi (green circles), villus sloughing (white circle), hyperemia in submucosa layer (red circles), and exudate in the intestinal lumen (black circle; H&E 20x); (F) oedema in the apical end of the villus (black circle; H&E 40x); (G) infiltration of mononuclear cells in mucosa submucosa layer of a villus (H&E 40x); and (H) subepithelial spaces (arrow; H&E 20x).
Discussion
The present study shows that AFB1 has an impact on intestinal physiology in the seabream. An observation in line with our previous work in this species showing adverse effects of AFB1 on seabream growth, disruption of metabolism, and tissue integrity, for example, liver (Barany et al., 2020). Here, our experimental approach was twofold; on the one hand, we used tissues from healthy fish to assess the immediate effects of AFB1 in vitro in Ussing chambers. On the other hand, we assessed the intestinal response to dietary AFB1 using fish obtained from the same experimental procedure employed by Barany et al. (2020).
An in vitro experiment using Ussing chambers was devised to test the putative direct effects of AFB1 on the anterior intestine of fish without previous contact with the toxin. Although the advantage of this approach is that intestinal explants maintain the original function and architecture of the tissue, they have a limited life span of ~4 h (Fuentes et al., 2006). The concentrations used in vitro were in the low micromolar range (e.g., 8 and 16 μM of apical AFB1). These values were derived from calculations of the dietary leaching of AFB1 used in feeding experiments (Barany et al., 2020), considering feed AFB1 content (1 or 2 mg/kg of feed), feeding rates, intestinal fluid volume, and putative residence time of food (Gilannejad et al., 2019). Short-term exposure to apical AFB1 at these levels did not affect the primary markers of tissue integrity TER or intestinal permeability. Our results contrast with previous studies in human colon carcinoma Caco-2 cell cultures (Romero et al., 2016), where AFB1 exposure in vitro significantly reduced TER but at more prolonged periods. Therefore, this apparent disparity in effect might be related to the timing of exposure or apical AFB1 doses employed since the highest concentrations in our tests were about 6-fold lower (16 vs. 100 μM) than those used in Romero et al. (2016).
Furthermore, we measured apical to basolateral fluxes to determine putative changes in the paracellular pathway. The results with FITC (4 kD) showed no significant effects in permeability. This apparent lack of direct action on paracellular permeability might be related to unchanged permeability for larger organic solutes and/or the intrinsic biology of different intestinal regions. It has been previously shown how the permeability might differ from smaller to larger organic solutes and within other intestinal regions in mice (Tanaka et al., 2015). Although we did not find statistical differences in permeability, it appears that high concentrations of apical AFB1 (16 μM) may lower the paracellular fluxes for larger molecules according to the TER increase observed in the in vivo feeding experiments. Similarly, an inverse relationship between gut permeability and TER has been reported in parasitized fish intestines (Sitjà-Bobadilla et al., 2019) or intestinal mycotoxicosis (Gao et al., 2018, 2020).
In contrast, long-term feeding experiments showed that dietary AFB1 modified intestinal selectivity severely by increasing TER but was without effect on Isc. TER is the gold standard to assess barrier integrity and function (Wijtten et al., 2011). Interestingly, in vivo dietary AFB1 significantly enhanced TER in the anterior but not in the posterior intestine, showing up anterior-to-posterior differences in barrier function previously reported in seabream (Carvalho et al., 2012; Gregorio et al., 2012). To our knowledge, no other fish studies have been performed in this context. Although, the increase in tissue resistance in response to dietary AFB1 is somehow surprising considering that in Caco-2 cells, AFB1 in the culture medium decreased TER after 48 h of exposure (Gao et al., 2018). However, Galarza-Seeber et al. (2016), using gut leakage of FITC in an in vivo chicken model, showed that the integrity of the gut barrier function was unaffected by dietary AFB1. We suggest that these apparent disparities across studies in TER may be linked to intestinal inflammation due to a rebound effect caused by a secondary response in the long-term exposure to AFB1. Therefore, eliciting a putative biphasic inflammatory response characterized by an initial transient TER decrease that precedes a subsequently TER increase if the aflatoxicosis persists. Intestinal inflammation decreases the tissue capacity for absorption and might even revert to secretion, thus causing diarrheic disorders (Schulzke et al., 2009; Verkman et al., 2013) that ultimately impair growth performance (Barany et al., 2020). Rebound effects are recurrent physiological responses in order to adjust the organism to face a new situation or as a detrimental side effect (Fenwick et al., 1999; Montserrat et al., 2007).
The GIT is the first contact point with feed components. Therefore, dietary aflatoxins are promptly absorbed into the bloodstream from the GIT (Battacone et al., 2012; Rodrigues et al., 2019). In tetrapods, the small intestine (homologous to the anterior fish intestine) has a greater surface area due to the presence of villi (Kiela and Ghishan, 2016), while in fish, it has also been reported a greater number of villi and globet cells within this same region (Verdile et al., 2020). Note that the villi are conformed mostly by absorptive cells (Kiela and Ghishan, 2016). Thus, in our feeding experiments, we assumed the anterior intestine was exposed to much higher doses of AFB1 released from the feed than the posterior since it is in this region where the AFB1 is firstly absorbed (Rodrigues et al., 2019).
In the intestine, TJs separate the paracellular space from the intestinal lumen acting as a divider of apical and basolateral domains of plasma membranes (van Itallie and Anderson, 2013), thus regulating the paracellular movement of ions, water, and small molecules. Claudins and occludins are essential elements of a functional tight junction and determine the TER of the intestinal epithelium. Therefore, the modification in barrier properties reflected by TER is tightly linked with changes in these key structural proteins of the TJ (Curry et al., 2020). To understand the underlying molecular network of altered tissue resistance in response to dietary AFB1 observed in feeding experiments in the seabream, we performed a qPCR array that included several claudins and occludins. Bearing in mind that in most studies performed to date, claudin gene mRNA expression usually reflects protein levels and can be considered a proxy for their function (Alexandre et al., 2005; Tanaka et al., 2015). In our study, dietary AFB1 had a wide range of effects on claudin and occludin effects in expression (measured by qPCR) in the seabream intestine. Specifically, it seems that the anterior intestine is predictably more responsive than the posterior intestine to AFB1 exposure, in line with the observed histopathological effects (e.g., inflammation). This observation is unsurprising since AFB1 has a low molecular weight and is assumed to be rapidly absorbed first by the proximal intestine (Rodrigues et al., 2019).
Previous studies in grass carp showed that mycotoxins downregulated barrier-forming TJ proteins expression, such as zonula occludens 1, zonula occludens 2b, ocln, cldnc, cldnf, cldn7a, cldn7b, and cldn11, whereas cldn12 and cldn15a were upregulated (Huang et al., 2018). Based on several overexpression studies, certain claudins, including cldn1, cldn3, cldn4, and cldn15, increased TER, whereas cldn2 had opposite effects (Singh and Harris, 2004; Tamura et al., 2008; Milatz et al., 2010). Additionally, certain claudins’ functions have also been linked to intestinal crypt stem cell survival, self-renewal, and epithelial differentiation (Tamura et al., 2008; Xing et al., 2020). Remarkably, differential overexpression among claudins possibly modified ionic paracellular selectivity, for example, Cl− and Na+ (Alexandre et al., 2005), and organic solute fluxes (Tanaka et al., 2015).
In our study, dietary AFB1 in the seabream significantly downregulated cldn3 in the anterior intestine and cldnb in the posterior intestine, while cldn7b, cldn15, and cldn34 were upregulated in the anterior intestine. Thus, some of these changes in expression probably have physiological implications that may trigger a switch in tissue selectivity reflected by the in vivo TER increase and/or the apparent decrease in the intestinal tissue permeability properties for larger organic solutes (~4 kD) as previously shown by Curry et al. (2020). Similarly, previous studies have provided a relationship between TER decreased and permeability enhancement (Villarroel et al., 2009).
Few studies have investigated the role of occludin-proteins/genes in fish to the best of our knowledge. Overall, their distribution is tissue-specific, and they play a role in hydromineral balance through the regulation of epithelial tightness. Such is the case in fish, where occludins play an essential role in regulating paracellular solute movements (Chasiotis and Kelly, 2008; Tipsmark and Madsen, 2012) and show the unambiguous distribution to apical sections of osmoregulatory tissues, such as the gill, kidney, and GIT. Previous studies have correlated low protein expression with leaky epithelia, whereas high protein expression appears in tight epithelia (Chasiotis and Kelly, 2008). Occludin downregulation parallels decrease TER in Caco-2 epithelial monolayers (Musch et al., 2006; Zeissig et al., 2007).
Here we identified three different occludins, for example, ocln1, ocln2, and ocln3 in the GIT system of seabream, without an apparent pattern of anterior–posterior expression differentiation. On analyzing the dietary effects of AFB1, we found a significant increase of relative mRNA expression of ocln1 and ocln3 in the anterior intestine, likely associated with the TER enhancement in response to dietary AFB1 as previously reported in cells in vitro with androgens (Kaitu’u-Lino et al., 2020). Note that occludin 1b in the human distal intestine might also play an essential role in intestinal leakage across TJs (Muresan et al., 2000). In addition, corticosteroids (e.g., cortisol) and other hormones can also directly modulate epithelial permeability characteristics reflected by changes in TER (Chasiotis et al., 2010; Trubitt et al., 2015). We believe this is not the case in our study since we found no differences in plasma cortisol in response to dietary AFB1 (Barany et al., 2021). Thus, and besides endogenous hormones, anti-nutritional factors, such as AFB1, seem to alter the intestinal barrier function properties throughout diet alone. Similar suppressive effects on occludins relative expression have also been reported in the intestine of Atlantic salmon (Moldal et al., 2018). Specifically, and among other effects, deoxynivalenol was suggested to diminish the expression of occludins in the distal intestine.
Mycotoxins also alter the functions and histomorphology of the intestine (Liew and Mohd-Redzwan, 2018; Alassane-Kpembi et al., 2019). In the present study, dietary AFB1 induced desquamation with exudate in the intestinal lumen of the anterior region, while goblet cells (GC) decreased in the epithelial lining of the intestinal villi. Previous studies on AFB1 dietary effects on fishes also observed sloughing of surface epithelial in the intestine of Catla catla (Andleeb et al., 2015) and described hypoplasia of GC villi sloughing in carps exposed to AFB1 (Khan et al., 2019). In our study, dietary AFB1 caused in the seabream edema in the villi, necrosis of epithelial cells, and mononuclear cells infiltration in the submucosal layer of the villi. Also, subepithelial spaces were observed due to the separation of mucosa and submucosa layers (Akinrinmade et al., 2016). Thus, we suggest some of these gut-architectural affections might be caused by the specific toxic binding of AFB1 to constituents of TJs directly exposed to the toxicant (Sonoda et al., 1999), plus that observed claudins dysregulation may also interfere with TJs signaling to regulate local cell turnover (Tanaka et al., 2015; Xing et al., 2020).
In summary, our results showed that dietary AFB1 modifies fundamental mechanisms of intestinal physiology in the seabream. AFB1 impacts intestinal histomorphology and alters barrier function. Interestingly, no short-term effects were observed, indicating that chronic exposure, and molecular alterations, are needed to reveal its impact. Indeed, we reported that long-term dietary AFB1 modifies barrier function as shown by TER measurements and scrambles the expression of key components of the tight junction, claudins, and occludins. Based on these results, we conclude that dietary AFB1 in the gastrointestinal system is the base of the previously reported growth impairment of AFB1 in the seabream (Barany et al., 2020) that later led to altered physiological stress responses to crowding densities (Barany et al., 2021). Further studies regarding the specific physiological involvement of the described claudins and occludins in the current study are warranted to fully understand the likely complex combinatorial interactions linked to intestinal permeability regulation.
Data Availability Statement
All data generated or analyzed during this study are available as Supplementary Material for this article.
Ethics Statement
The animal study was reviewed and approved by Junta de Andalucía (reference number 28-04-15-241) and by the Ethics and Animal Welfare Committee from the Spanish Government (RD53/2013). In addition, all animal manipulations were carried out in compliance with the European (Directive 2010/63/EU) and Portuguese legislation for the use of laboratory animals. All animal protocols were performed under Group-C licenses from the Direção-Geral de Alimentação e Veterinária, Ministério da Agricultura, do Mar, Ambiente e Ordenamento do Território, Portugal.
Author Contributions
AB, JM, and JF contributed to the conception and design of the research. AB performed osmolality, enzyme activity, electrophysiology, molecular analysis, data curation, statistical analysis, and drafted manuscript. AB and SFG performed Na+ and permeability analysis. MO performed the histopathological examination. GM-R supervised molecular analyses and performed phylogenies. AB, MO, SFG, GM-R, JM, and JF edited and revised the manuscript. All authors contributed to the article and approved the submitted version.
Funding
This work was funded by the Spanish Ministry of Economy and Business-MINECO (AGL2016-76069-C2-1-R) awarded to JM. The authors (AB and JM) belong to the Fish Welfare and Stress Network (AGL2016-81808-REDT), supported by the Agencia Estatal de Investigación (MINECO, Spanish Government). AB was supported by the University of Cadiz Ph.D. scholarship (PIF UCA/REC02VIT/2014). CCMar is supported by national funds from the Portuguese Foundation for Science and Technology (FCT) through project UIDB/04326/2020.
Conflict of Interest
The authors declare that the research was conducted in the absence of any commercial or financial relationships that could be construed as a potential conflict of interest.
Publisher’s Note
All claims expressed in this article are solely those of the authors and do not necessarily represent those of their affiliated organizations, or those of the publisher, the editors and the reviewers. Any product that may be evaluated in this article, or claim that may be made by its manufacturer, is not guaranteed or endorsed by the publisher.
Acknowledgments
The authors wish to thank Maria Guilloto for sampling collection and Rosa Vázquez and staff for the use of the facilities of Servicios Centrales de Investigación en Cultivos Marinos SCI-CM, CASEM, University of Cádiz, Spain) to carry out the experiments. We also thank the group of Dr. Francisco Javier Moyano of the University of Almería, who made the experimental aquafeeds.
Supplementary Material
The Supplementary Material for this article can be found online at: https://www.frontiersin.org/articles/10.3389/fphys.2021.741192/full#supplementary-material
References
Akbari, P., Braber, S., Varasteh, S., and Alizadeh, A. (2017). The intestinal barrier as an emerging target in the toxicological assessment of mycotoxins. Arch. Toxicol. 91, 1007–1029. doi: 10.1007/s00204-016-1794-8
Akinrinmade, F. J., Akinrinde, A. S., and Amid, A. (2016). Changes in serum cytokine levels, hepatic and intestinal morphology in aflatoxin B1-induced injury: modulatory roles of melatonin and flavonoid-rich fractions from Chromolena odorata. Mycotoxin Res. 32, 53–60. doi: 10.1007/s12550-016-0239-9
Alassane-Kpembi, I., Pinton, P., and Oswald, I. P. (2019). Effects of mycotoxins on the intestine. Toxins 11:159. doi: 10.3390/toxins11030159
Alexandre, M. D., Lu, Q., and Chen, Y.-H. (2005). Overexpression of claudin-7 decreases the paracellular Cl− conductance and increases the paracellular Na+ conductance in LLC-PK1 cells. J. Cell Sci. 118, 2683–2693. doi: 10.1242/jcs.02406
Anater, A., Araújo, C. M. T. D., Rocha, D. C. C., Ostrensky, A., Filho, J. R. E., Ribeiro, D. R., et al. (2020). Evaluation of growth performance, hematological, biochemical and histopathological parameters of Rhamdia quelen fed with a feed artificially contaminated with aflatoxin B1. Aquac. Rep. 17:100326. doi: 10.1016/j.aqrep.2020.100326
Andleeb, S., Ashraf, M., Hafeez-Ur-Rehman, M., Jabbar, M. A., Abbas, F., and Younus, M. (2015). Effect of aflatoxin B1-contaminated feed on growth and vital organs of advance fry of, Catla catla. J. Anim. Plant Sci. 25, 816–824.
Arnold, Y. E., Thorens, J., Bernard, S., and Kalia, Y. N. (2019). Drug transport across porcine intestine using an Ussing chamber system: regional differences and the effect of P-glycoprotein and CYP3A4 activity on drug absorption. Pharmaceutics 11:139. doi: 10.3390/pharmaceutics11030139
Barany, A., Fuentes, J., Martínez-Rodríguez, G., and Mancera, J. M. (2021). Aflatoxicosis dysregulates the physiological responses to crowding densities in the marine teleost gilthead seabream (Sparus aurata). Animals 11:753. doi: 10.3390/ani11030753
Barany, A., Guilloto, M., Cosano, J., de Boevre, M., Oliva, M., de Saeger, S., et al. (2020). Dietary aflatoxin B1 (AFB1) reduces growth performance, impacting growth axis, metabolism, and tissue integrity in juvenile gilthead sea bream (Sparus aurata). Aquaculture 536:736510. doi: 10.1016/j.aquaculture.2020.736189
Battacone, G., Nudda, A., Rassu, S. P. G., Decandia, M., and Pulina, G. (2012). Excretion pattern of aflatoxin M1 in milk of goats fed a single dose of aflatoxin B1. J. Dairy Sci. 95, 2656–2661. doi: 10.3168/jds.2011-5003
Bedoya-Serna, C. M., Michelin, E. C., Massocco, M. M., Carrion, L. C. S., Godoy, S. H. S., Lima, C. G., et al. (2018). Effects of dietary aflatoxin B1 on accumulation and performance in matrinxã fish (Brycon cephalus). PLoS One 13:e0201812. doi: 10.1371/journal.pone.0201812
Carvalho, E. S. M., Gregório, S. F., Power, D. M., Canário, A. V. M., and Fuentes, J. (2012). Water absorption and bicarbonate secretion in the intestine of the sea bream are regulated by transmembrane and soluble adenylyl cyclase stimulation. J. Comp. Physiol. B Biochem. Syst. Environ. Physiol. 182, 1069–1080. doi: 10.1007/s00360-012-0685-4
Chakraborty, P., Mallik, A., Sarang, N., and Lingam, S. S. (2019). A review on alternative plant protein sources available for future sustainable aqua feed production. Int. J. Chem. Stud. 7, 1399–1404.
Chasiotis, H., and Kelly, S. P. (2008). Occludin immunolocalization and protein expression in goldfish. J. Exp. Biol. 211, 1524–1534. doi: 10.1242/jeb.014894
Chasiotis, H., Wood, C. M., and Kelly, S. P. (2010). Molecular and cellular endocrinology cortisol reduces paracellular permeability and increases occludin abundance in cultured trout gill epithelia. Mol. Cell. Endocrinol. 323, 232–238. doi: 10.1016/j.mce.2010.02.030
Claude, P. (1978). Morphological factors influencing transepithelial permeability: A model for the resistance of the Zonula Occludens. J. Membr. Biol. 39, 219–232. doi: 10.1007/BF01870332
Collins, F. L., Rios-Arce, N. D., Atkinson, S., Bierhalter, H., Schoenherr, D., Bazil, J. N., et al. (2017). Temporal and regional intestinal changes in permeability, tight junction, and cytokine gene expression following ovariectomy-induced estrogen deficiency. Phys. Rep. 5:e13263. doi: 10.14814/phy2.13263
Curry, J. N., Tokuda, S., McAnulty, P., and Yu, A. S. L. (2020). Combinatorial expression of claudins in the proximal renal tubule and its functional consequences. Am. J. Physiol. Ren. Physiol. 318, F1138–F1146. doi: 10.1152/ajprenal.00057.2019
Daniel, D. (2018). A review on replacing fish meal in aqua feeds using plant protein sources. Int. J. Fish. Aquat. Stud. 6, 164–179.
de Freitas Souza, C., Baldissera, M. D., Baldisserotto, B., Petrolli, T. G., da Glória, E. M., Zanette, R. A., et al. (2020). Dietary vegetable choline improves hepatic health of Nile tilapia (Oreochromis niloticus) fed aflatoxin-contaminated diet. Comp. Biochem. Physiol. Part C Toxicol. Pharmacol. 227:108614. doi: 10.1016/j.cbpc.2019.108614
Deng, H., Su, X., and Wang, H. (2018a). Simultaneous determination of aflatoxin B1, bisphenol A, and 4-nonylphenol in peanut oils by liquid-liquid extraction combined with solid-phase extraction and ultra-high performance liquid chromatography-tandem mass spectrometry. Food Anal. Methods 11, 1303–1311. doi: 10.1007/s12161-017-1113-x
Deng, J., Zhao, L., Zhang, N. Y., Karrow, N. A., Krumm, C. S., Qi, D. S., et al. (2018b). Aflatoxin B 1 metabolism: regulation by phase I and II metabolizing enzymes and chemoprotective agents. Mutat. Res. Rev. Mutat. Res. 778, 79–89. doi: 10.1016/j.mrrev.2018.10.002
Ditta, Y. A., Mahad, S., and Bacha, U. (2019). “Aflatoxins: their toxic effect on poultry and recent advances in their treatment,” in Mycotoxins. P. B. Njobeh and F. Stepman (Eds.) (Rijeka: IntechOpen)
Estensoro, I., Ballester-Lozano, G., Benedito-Palos, L., Grammes, F., Martos-Sitcha, J. A., Mydland, L. T., et al. (2016). Dietary butyrate helps to restore the intestinal status of a marine teleost (Sparus aurata) fed extreme diets low in fish meal and fish oil. PLoS One 11:e0166564. doi: 10.1371/journal.pone.0166564
FAO (2020). The State of World Fisheries and Aquaculture (SOFIA). Available at: http://doi.org/10.4060/ca9229en
Fenwick, J. C., Wendelaar Bonga, S. E., and Flik, G. (1999). In vivo bafilomycin-sensitive Na+ uptake in young freshwater fish. J. Exp. Biol. 202, 3659–3666. doi: 10.1242/jeb.202.24.3659
Flores-Flores, M. E., Lizarraga, E., Adela, L., and Gonz, E. (2015). Presence of mycotoxins in animal milk: A review. Food Control 53, 163–176. doi: 10.1016/j.foodcont.2015.01.020
Fuentes, J., Figueiredo, J., Power, D. M., and Canário, A. V. M. (2006). Parathyroid hormone-related protein regulates intestinal calcium transport in sea bream (Sparus auratus). Am. J. Physiol. Integr. Comp. Physiol. 291, R1499–R1506. doi: 10.1152/ajpregu.00892.2005
Galarza-Seeber, R., Latorre, J. D., Bielke, L. R., Kuttappan, V. A., Wolfenden, A. D., Hernandez-Velasco, X., et al. (2016). Leaky gut and mycotoxins: Aflatoxin B1 does not increase gut permeability in broiler chickens. Front. Vet. Sci. 3:10. doi: 10.3389/fvets.2016.00010
Gao, Y., Li, S., Wang, J., Luo, C., Zhao, S., and Zheng, N. (2018). Modulation of intestinal epithelial permeability in differentiated Caco-2 cells exposed to aflatoxin M1 and ochratoxin a individually or collectively. Toxins 10, 13. doi: 10.3390/toxins10010013
Gao, Y., Meng, L., Liu, H., Wang, J., and Zheng, N. (2020). The compromised intestinal barrier induced by mycotoxins. Toxins 12:619. doi: 10.3390/toxins12100619
Gilannejad, N., Silva, T., Martínez-Rodríguez, G., and Yúfera, M. (2019). Effect of feeding time and frequency on gut transit and feed digestibility in two fish species with different feeding behaviours, gilthead seabream and Senegalese sole. Aquaculture 513:734438. doi: 10.1016/j.aquaculture.2019.734438
Gonçalves, R. A., Do Cam, T., Tri, N. N., Santos, G. A., Encarnação, P., and Hung, L. T. (2018a). Aflatoxin B1 (AFB1) reduces growth performance, physiological response, and disease resistance in Tra catfish (Pangasius hypophthalmus). Aquac. Int. 26, 921–936. doi: 10.1007/s10499-018-0259-x
Gonçalves, R. A., Naehrer, K., and Santos, G. A. (2018b). Occurrence of mycotoxins in commercial aquafeeds in Asia and Europe: A real risk to aquaculture? Rev. Aquac. 10, 263–280. doi: 10.1111/raq.12159
Gregorio, S. F., Carvalho, E. S. M., Encarnacao, S., Wilson, J. M., Power, D. M., Canario, A. V. M., et al. (2012). Adaptation to different salinities exposes functional specialization in the intestine of the sea bream (Sparus aurata L.). J. Exp. Biol. 216, 470–479. doi: 10.1242/jeb.073742
Guengerich, F. P., Johnson, W. W., Shimada, T., Ueng, Y. F., Yamazaki, H., and Langouët, S. (1998). Activation and detoxication of aflatoxin B1. Mutat. Res. Fundam. Mol. Mech. Mutagen. 402, 121–128. doi: 10.1016/S0027-5107(97)00289-3
Hou, J., Rajagopal, M., and Yu, A. S. L. (2013). Claudins and the kidney. Annu. Rev. Physiol. 75, 479–501. doi: 10.1146/annurev-physiol-030212-183705
Huang, Y., Han, D., Xiao, X., Zhu, X., Yang, Y., Jin, J., et al. (2014). Effect of dietary aflatoxin B1 on growth, fecundity and tissue accumulation in gibel carp during the stage of gonad development. Aquaculture 428–429, 236–242. doi: 10.1016/j.aquaculture.2014.03.010
Huang, Y., Han, D., Zhu, X., Yang, Y., Jin, J., Chen, Y., et al. (2011). Response and recovery of gibel carp from subchronic oral administration of aflatoxin B1. Aquaculture 319, 89–97. doi: 10.1016/j.aquaculture.2011.06.024
Huang, C., Wu, P., Jiang, W. D., Liu, Y., Zeng, Y. Y., Jiang, J., et al. (2018). Deoxynivalenol decreased the growth performance and impaired intestinal physical barrier in juvenile grass carp (Ctenopharyngodon idella). Fish Shellfish Immunol. 80, 376–391. doi: 10.1016/j.fsi.2018.06.013
Kaitu’u-Lino, T. J., Sluka, P., Foo, C. F. H., and Stanton, P. G. (2020). Claudin-11 expression and localisation is regulated by androgens in rat Sertoli cells in vitro. Reproduction 133, 1169–1179. doi: 10.1530/REP-06-0385
Khan, H., Khan, F. A., Sadique, U., Ahmad, S., Ul Hassan, Z., Ahmad, F., et al. (2019). Genotoxic and toxicopathological effect of aflatoxin B1 in grass carp (Ctenopharyngodon idella). Kafkas Univ. Vet. Fak. Derg. 25, 841–848. doi: 10.9775/kvfd.2019.21988
Kiela, P. R., and Ghishan, F. K. (2016). Physiology of intestinal absorption and secretion. Best Pract. Res. Clin. Gastroenterol. 30, 145–159. doi: 10.1016/j.bpg.2016.02.007
Kiron, V., Park, Y., Siriyappagouder, P., Dahle, D., Vasanth, G. K., Dias, J., et al. (2020). Intestinal transcriptome reveals soy derivatives-linked changes in Atlantic salmon. Front. Immunol. 11:3013. doi: 10.3389/fimmu.2020.596514
Kolosov, D., Bui, P., Chasiotis, H., and Kelly, S. P. (2013). Claudins in teleost fishes. Tissue Barriers 1:e25391. doi: 10.4161/tisb.25391
Liew, W. P. P., and Mohd-Redzwan, S. (2018). Mycotoxin: its impact on gut health and microbiota. Front. Cell. Infect. Microbiol. 8:60. doi: 10.3389/fcimb.2018.00060
Livak, K. J., and Schmittgen, T. D. (2001). Analysis of relative gene expression data using real-time quantitative PCR and the 2-ΔΔCT method. Methods 25, 402–408. doi: 10.1006/meth.2001.1262
Lu, Z., Ding, L., Lu, Q., and Chen, Y.-H. (2013). Claudins in intestines. Tissue Barriers 1:e24978. doi: 10.4161/tisb.24978
Mata-Sotres, J. A., Martos-Sitcha, J. A., Astola, A., Yúfera, M., and Martínez-Rodríguez, G. (2016). Cloning and molecular ontogeny of digestive enzymes in fed and food-deprived developing gilthead seabream (Sparus aurata) larvae. Comp. Biochem. Physiol. Part B Biochem. Mol. Biol. 191, 53–65. doi: 10.1016/j.cbpb.2015.09.006
McCormick, S. D. (1993). Methods for nonlethal gill biopsy and measurement of Na+, K+ -ATPase activity. Can. J. Fish. Aquat. Sci. 50, 656–658. doi: 10.1139/f93-075
Milatz, S., Krug, S. M., Rosenthal, R., Günzel, D., Müller, D., Schulzke, J. D., et al. (2010). Claudin-3 acts as a sealing component of the tight junction for ions of either charge and uncharged solutes. Biochim. Biophys. Acta Biomembr. 1798, 2048–2057. doi: 10.1016/j.bbamem.2010.07.014
Moldal, T., Bernhoft, A., Rosenlund, G., Kaldhusdal, M., and Koppang, E. O. (2018). Dietary deoxynivalenol (DON) may impair the epithelial barrier and modulate the cytokine signaling in the intestine of Atlantic salmon (Salmo salar). Toxins 10:376. doi: 10.3390/toxins10090376
Montserrat, N., Gabillard, J. C., Capilla, E., Navarro, M. I., and Gutiérrez, J. (2007). Role of insulin, insulin-like growth factors, and muscle regulatory factors in the compensatory growth of the trout (Oncorhynchus mykiss). Gen. Comp. Endocrinol. 150, 462–472. doi: 10.1016/j.ygcen.2006.11.009
Mukendi, C., Dean, N., Lala, R., Smith, J., Bronner, M. E., and Nikitina, N. V. (2016). Evolution of the vertebrate claudin gene family: insights from a basal vertebrate, the sea lamprey. Int. J. Dev. Biol. 60, 39–51. doi: 10.1387/ijdb.150364nn
Muresan, Z., Paul, D. L., and Goodenough, D. A. (2000). Occludin 1B, a variant of the tight junction protein occludin. Mol. Biol. Cell 11, 627–634. doi: 10.1091/mbc.11.2.627
Musch, M. W., Walsh-Reitz, M. M., and Chang, E. B. (2006). Roles of ZO-1, occludin, and actin in oxidant-induced barrier disruption. Am. J. Physiol. Gastrointest. Liver Physiol. 290, G222–G231. doi: 10.1152/ajpgi.00301.2005
Nácher-Mestre, J., Ballester-Lozano, G. F., Garlito, B., Portolés, T., Calduch-Giner, J., Serrano, R., et al. (2018). Comprehensive overview of feed-to-fillet transfer of new and traditional contaminants in Atlantic salmon and gilthead sea bream fed plant-based diets. Aquac. Nutr. 24, 1782–1795. doi: 10.1111/anu.12817
Nácher-Mestre, J., Serrano, R., Beltrán, E., Pérez-Sánchez, J., Silva, J., Karalazos, V., et al. (2015). Occurrence and potential transfer of mycotoxins in gilthead sea bream and Atlantic salmon by use of novel alternative feed ingredients. Chemosphere 128, 314–320. doi: 10.1016/j.chemosphere.2015.02.021
Ostry, V., Malir, F., Toman, J., and Grosse, Y. (2017). Mycotoxins as human carcinogens—the IARC monographs classification. Mycotoxin Res. 33, 65–73. doi: 10.1007/s12550-016-0265-7
Pandya, J. P., and Arade, P. C. (2016). Mycotoxin: A devil of human, animal and crop health. Adv. Life Sci. 5, 3937–3941.
Pfaffl, M. W. (2001). A new mathematical model for relative quantification in real-time RT-PCR. Nucleic Acids Res. 29:e45. doi: 10.1093/nar/29.9.e45
Rodrigues, R. O., Rodrigues, R. O., Ledoux, D. R., Rottinghaus, G. E., Borutova, R., Averkieva, O., et al. (2019). Feed additives containing sequestrant clay minerals and inactivated yeast reduce aflatoxin excretion in milk of dairy cows. J. Dairy Sci. 102, 6614–6623. doi: 10.3168/jds.2018-16151
Romero, A., Ares, I., Ramos, E., Castellano, V., Martínez, M., Martínez-Larrañaga, M.-R., et al. (2016). Mycotoxins modify the barrier function of Caco-2 cells through differential gene expression of specific claudin isoforms: protective effect of illite mineral clay. Toxicology 353–354, 21–33. doi: 10.1016/j.tox.2016.05.003
Ruiz-Jarabo, I., Barany, A., Jerez-Cepa, I., Mancera, J. M., and Fuentes, J. (2017). Intestinal response to salinity challenge in the Senegalese sole. (Solea senegalensis). Comp. Biochem. Physiol. -Part A Mol. Integr. Physiol. 204, 57–64. doi: 10.1016/j.cbpa.2016.11.009
Santacroce, M. P., Iaffaldano, N., Zacchino, V., Rosato, M. P., Casalino, E., and Centoducati, G. (2012). Effects of Aflatoxin bi on liver phase I and phase II enzymes induced in vitro on Sparus aurata hepatocytes primary culture. Ital. J. Anim. Sci. 11:e60. doi: 10.4081/ijas.2012.e60
Schulzke, J. D., Ploeger, S., Amasheh, M., Fromm, A., Zeissig, S., Troeger, H., et al. (2009). Epithelial tight junctions in intestinal inflammation. Ann. N. Y. Acad. Sci. 1165, 294–300. doi: 10.1111/j.1749-6632.2009.04062.x
Shen, L., Weber, C. R., Raleigh, D. R., Yu, D., and Turner, J. R. (2011). Tight junction pore and leak pathways: A dynamic duo. Annu. Rev. Physiol. 73, 283–309. doi: 10.1146/annurev-physiol-012110-142150
Singh, A. B., and Harris, R. C. (2004). Epidermal growth factor receptor activation differentially regulates claudin expression and enhances transepithelial resistance in Madin-Darby canine kidney cells. J. Biol. Chem. 279, 3543–3552. doi: 10.1074/jbc.M308682200
Sitjà-Bobadilla, A., Gil-Solsona, R., Estensoro, I., Piazzon, M. C., Martos-Sitcha, J. A., Picard-Sánchez, A., et al. (2019). Disruption of gut integrity and permeability contributes to enteritis in a fish-parasite model: A story told from serum metabolomics. Parasit. Vectors 12:486. doi: 10.1186/s13071-019-3746-7
Sonoda, N., Furuse, M., Sasaki, H., Yonemura, S., Katahira, J., Horiguchi, Y., et al. (1999). Clostridium perfringens enterotoxin fragment removes specific claudins from tight junction strands. J. Cell Biol. 147, 195–204. doi: 10.1083/jcb.147.1.195
Tacon, A. G. J., Hasan, M. R., and Metian, M. (2011). Demand and supply of feed ingredients for farmed fish and crustaceans: Trends and prospects. Available at: http://www.fao.org/docrep/015/ba0002e/ba0002e.pdf
Tacon, A. G. J., and Metian, M. (2008). Global overview on the use of fish meal and fish oil in industrially compounded aquafeeds: trends and future prospects. Aquaculture 285, 146–158. doi: 10.1016/j.aquaculture.2008.08.015
Tamura, A., Kitano, Y., Hata, M., Katsuno, T., Moriwaki, K., Sasaki, H., et al. (2008). Megaintestine in claudin-15-deficient mice. Gastroenterology 134, 523–534. doi: 10.1053/j.gastro.2007.11.040
Tanaka, H., Takechi, M., Kiyonari, H., Shioi, G., Tamura, A., and Tsukita, S. (2015). Intestinal deletion of claudin-7 enhances paracellular organic solute flux and initiates colonic inflammation in mice. Gut 64, 1529–1538. doi: 10.1136/gutjnl-2014-308419
Tipsmark, C. K., and Madsen, S. S. (2012). Tricellulin, occludin and claudin-3 expression in salmon intestine and kidney during salinity adaptation. Comp. Biochem. Physiol. Part A Mol. Integr. Physiol. 162, 378–385. doi: 10.1016/j.cbpa.2012.04.020
Tipsmark, C. K., Sørensen, K. J., and Madsen, S. S. (2010). Aquaporin expression dynamics in osmoregulatory tissues of Atlantic salmon durings moltification and seawater acclimation. J. Exp. Biol. 213, 368–379. doi: 10.1242/jeb.034785
Trubitt, R. T., Rabeneck, D. B., Bujak, J. K., Bossus, M. C., Madsen, S. S., and Tipsmark, C. K. (2015). Transepithelial resistance and claudin expression in trout RTgill-W1 cell line: effects of osmoregulatory hormones. Comp. Biochem. Physiol. Part A Mol. Integr. Physiol. 182, 45–52. doi: 10.1016/j.cbpa.2014.12.005
van Itallie, C. M., and Anderson, J. M. (2006). Claudins and epithelial paracellular transport. Annu. Rev. Physiol. 68, 403–429. doi: 10.1146/annurev.physiol.68.040104.131404
van Itallie, C. M., and Anderson, J. M. (2013). Claudin interactions in and out of the tight junction. Tissue Barriers 1:e25247. doi: 10.4161/tisb.25247
Vandesompele, J., De Preter, K., Pattyn, F., Poppe, B., Van Roy, N., De Paepe, A., et al. (2002). Accurate normalization of real-time quantitative RT-PCR data by geometric averaging of multiple internal control genes. Genome Biol. 3:research0034.1. doi: 10.1186/gb-2002-3-7-research0034
Verdile, N., Pasquariello, R., Scolari, M., Scirè, G., Brevini, T. A. L., and Gandolfi, F. (2020). A detailed study of rainbow trout (Onchorhynchus mykiss) intestine revealed that digestive and absorptive functions are not linearly distributed along its length. Animals 10:745. doi: 10.3390/ani10040745
Verkman, A. S., Synder, D., Tradtrantip, L., Thiagarajah, J. R., and Anderson, M. O. (2013). CFTR inhibitors. Curr. Pharm. Des. 19, 3529–3541. doi: 10.2174/13816128113199990321
Villarroel, M., García-Ramírez, M., Corraliza, L., Hernández, C., and Simó, R. (2009). High glucose concentration leads to differential expression of tight junction proteins in human retinal pigment epithelial cells. Endocrinol. Nutr. 56, 53–58. doi: 10.1016/S1575-0922(09)70552-2
Wijtten, P. J. A., van der Meulen, J., and Verstegen, M. W. A. (2011). Intestinal barrier function and absorption in pigs after weaning: A review. Br. J. Nutr. 105, 967–981. doi: 10.1017/S0007114510005660
Xing, T., Benderman, L. J., Sabu, S., Parker, J., Yang, J., Lu, Q., et al. (2020). Tight junction protein claudin-7 is essential for intestinal epithelial stem cell self-renewal and differentiation. Cell. Mol. Gastroenterol. Hepatol. 9, 641–659. doi: 10.1016/j.jcmgh.2019.12.005
Zeissig, S., Bürgel, N., Günzel, D., Richter, J., Mankertz, J., Wahnschaffe, U., et al. (2007). Changes in expression and distribution of claudin 2, 5 and 8 lead to discontinuous tight junctions and barrier dysfunction in active Crohn’s disease. Gut 56, 61–72. doi: 10.1136/gut.2006.094375
Zhang, B., and Guo, Y. (2009). Supplemental zinc reduced intestinal permeability by enhancing occludin and zonula occludens protein-1 (ZO-1) expression in weaning piglets. Br. J. Nutr. 102, 687–693. doi: 10.1017/S0007114509289033
Keywords: epithelial barrier, gastrointestinal tract, mycotoxin, tight junctions, Ussing chamber
Citation: Barany A, Oliva M, Gregório SF, Martínez-Rodríguez G, Mancera JM and Fuentes J (2021) Dysregulation of Intestinal Physiology by Aflatoxicosis in the Gilthead Seabream (Sparus aurata). Front. Physiol. 12:741192. doi: 10.3389/fphys.2021.741192
Edited by:
Carol Bucking, York University, CanadaReviewed by:
Subrata Dasgupta, Central Institute of Fisheries Education (ICAR), IndiaM. C. Subhash Peter, University of Kerala, India
Copyright © 2021 Barany, Oliva, Gregório, Martínez-Rodríguez, Mancera and Fuentes. This is an open-access article distributed under the terms of the Creative Commons Attribution License (CC BY). The use, distribution or reproduction in other forums is permitted, provided the original author(s) and the copyright owner(s) are credited and that the original publication in this journal is cited, in accordance with accepted academic practice. No use, distribution or reproduction is permitted which does not comply with these terms.
*Correspondence: Juan Fuentes, jfuentes@ualg.pt