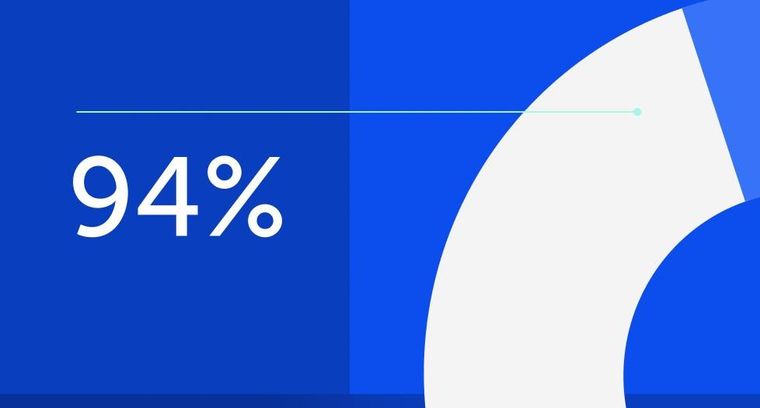
94% of researchers rate our articles as excellent or good
Learn more about the work of our research integrity team to safeguard the quality of each article we publish.
Find out more
ORIGINAL RESEARCH article
Front. Physiol., 28 September 2021
Sec. Membrane Physiology and Membrane Biophysics
Volume 12 - 2021 | https://doi.org/10.3389/fphys.2021.737291
This article is part of the Research TopicRegulatory Mechanisms of Ca2+-activated Ion Channels and Their Impacts on Physiological/Pathophysiological FunctionsView all 12 articles
Daily regulation of Ca2+– and voltage-activated BK K+ channel activity is required for action potential rhythmicity in the suprachiasmatic nucleus (SCN) of the hypothalamus, the brain's circadian clock. In SCN neurons, BK activation is dependent upon multiple types of Ca2+ channels in a circadian manner. Daytime BK current predominantly requires Ca2+ influx through L-type Ca2+ channels (LTCCs), a time when BK channels are closely coupled with their Ca2+ source. Here we show that daytime BK current is resistant to the Ca2+ chelator BAPTA. However, at night when LTCCs contribute little to BK activation, BK current decreases by a third in BAPTA compared to control EGTA conditions. In phase with this time-of-day specific effect on BK current activation, LTCC current is larger during the day. The specific Ca2+ channel subtypes underlying the LTCC current in SCN, as well as the subtypes contributing the Ca2+ influx relevant for BK current activation, have not been identified. SCN neurons express two LTCC subtypes, CaV1.2 and CaV1.3. While a role for CaV1.2 channels has been identified during the night, CaV1.3 channel modulation has also been suggested to contribute to daytime SCN action potential activity, as well as subthreshold Ca2+ oscillations. Here we characterize the role of CaV1.3 channels in LTCC and BK current activation in SCN neurons using a global deletion of CACNA1D in mouse (CaV1.3 KO). CaV1.3 KO SCN neurons had a 50% reduction in the daytime LTCC current, but not total Ca2+ current, with no difference in Ca2+ current levels at night. During the day, CaV1.3 KO neurons exhibited oscillations in membrane potential, and most neurons, although not all, also had BK currents. Changes in BK current activation were only detectable at the highest voltage tested. These data show that while CaV1.3 channels contribute to the daytime Ca2+ current, this does not translate into a major effect on the daytime BK current. These data suggest that BK current activation does not absolutely require CaV1.3 channels and may therefore also depend on other LTCC subtypes, such as CaV1.2.
The suprachiasmatic nucleus (SCN) is the central circadian clock of the brain in mammals (Hastings et al., 2018; Harvey et al., 2020). Membrane signaling via ion channels regulates the daily patterning of action potential activity and neurotransmitter release, which is critical for the expression of circadian behavioral rhythms. Calcium is an important regulator of SCN signaling (Ikeda, 2004; Hastings et al., 2018; McNally et al., 2020), and SCN neurons have several different Ca2+ influx pathways, including voltage-gated Ca2+ channels (Pennartz et al., 2002; Cloues and Sather, 2003; Harvey et al., 2020; McNally et al., 2020). Of the channels involved in SCN signaling, L-type Ca2+ channels (LTCCs) are abundantly expressed (Nahm et al., 2005; Harvey et al., 2020).
LTCC currents are generated by the CaV1 family of Ca2+ channel subtypes (Lipscombe et al., 2004; Striessnig et al., 2006). CaV1.1 is the skeletal muscle LTCC, while CaV1.2 (α1C, CACNA1C) and CaV1.3 (α1D, CACNA1D) are the predominant subtypes in neurons and are sensitive to inhibition by dihydropyridines such as nimodipine (Lipscombe et al., 2004; Striessnig et al., 2006). CaV1.2 plays a wide variety of roles in neuronal excitability and cell signaling, regulating action potential activity, secretion, and transcription (Striessnig et al., 2006), but global genetic deletion of CaV1.2 is lethal due to cardiac dysfunction (Seisenberger et al., 2000). CaV1.3 is also expressed in variety of neurons and neuroendocrine cells, often co-expressed with CaV1.2, but differs from CaV1.2 in its activation at lower voltages (Striessnig et al., 2006). A global deletion of CaV1.3 channels is viable and results in deafness and cardiac arrhythmias (Platzer et al., 2000; Zhang et al., 2005). SCN neurons express both CaV1.2 and CaV1.3 channels (Nahm et al., 2005; Huang et al., 2012; Kim et al., 2015; Cheng et al., 2018).
SCN LTCC current is diurnally modulated, with higher current during the day that decreases at night (Pennartz et al., 2002; Whitt et al., 2018; McNally et al., 2020). Correlated with the change in current magnitude, inhibition of LTCC current with nimodipine decreases SCN firing selectively during the day (Pennartz et al., 2002; Whitt et al., 2018; McNally et al., 2020). One mechanism for the effect on SCN firing is via LTCC-dependent activation of Ca2+-activated BK K+ channels (Plante et al., 2021). During the day, LTCCs are responsible for the majority of BK current activation (Jackson et al., 2004; Whitt et al., 2018; Plante et al., 2021). However, at night, their role is reduced, and BK current activation relies primarily on release of Ca2+ from intracellular stores (Whitt et al., 2018; Plante et al., 2021). Either CaV1.2 or CaV1.3 could contribute to the LTCC current that drives daytime activation of the BK current, as both channel subtypes are known to activate BK currents produced by BK channel variants from SCN (Plante et al., 2021) or within other excitable cells (Roberts et al., 1990; Wisgirda and Dryer, 1994; Prakriya and Lingle, 1999; Sun et al., 2003; Grunnet and Kaufmann, 2004; Berkefeld et al., 2006; Marcantoni et al., 2010; Hou et al., 2016; Bellono et al., 2017; Vivas et al., 2017; Plante et al., 2021). Little is known about the specific role of CaV1.2 on SCN firing, but CaV1.2 expression is higher at night and mouse knockouts display altered nighttime phase-shifting behavior (Schmutz et al., 2014). In contrast, CaV1.3 channels have been proposed to regulate firing rate, Ca2+ oscillations, and histamine signaling during the day (Huang et al., 2012; Kim et al., 2015, 2016). Current pharmacological tools cannot effectively distinguish between these LTCC subtypes, necessitating the use of transgenic mouse lines to address the relative contributions of these channel subtypes to neuronal excitability.
In this study, we focused on investigating a role for CaV1.3 channels in BK current activation, based on their proposed roles in SCN during the day and their regulation of spontaneous firing in other cell types via BK channel activation (Vandael et al., 2010; Bellono et al., 2017). Using a CaV1.3 knockout mouse line (CaV1.3 KO, also called α1D−/−, CACNA1D−/−)(Platzer et al., 2000), whole-cell Ca2+ and BK currents were recorded from day and night SCN neurons in acute brain slices. The results reveal that CaV1.3 channels contribute to the daytime LTCC current in SCN neurons, as well as BK current activation.
Experimental mice were 3–6 week old male and female wildtype (WT) C57BL/6J and CaV1.3 WT and KO littermates produced from heterozygous CaV1.3 breeding pairs on a mixed C57BL6:FVB background (Platzer et al., 2000). Tail or ear tissue samples were genotyped using “Cacna1d-1 WT” and neomycin probes in real-time PCR reactions at a commercial vendor (Transnetyx, Cordova, TN). All mice were group housed from birth on a standard 12:12 h light–dark cycle (for day timepoints) or a reverse 12:12 h light–dark cycle (night timepoints). All procedures involving mice were conducted in accordance with the University of Maryland School of Medicine Animal Care and Use Guidelines and approved by the Institutional Animal Care and Use Committee.
Mice were sacrificed at zeitgeber time (ZT) 1–3 h for day, or ZT 14–16 h for night, experiments. Brains were rapidly removed and placed into ice-cold sucrose-substituted saline containing (in mM): 1.2 MgSO4, 26 NaHCO3, 1.25 Na2HPO4, 3.5 KCl, 3.8 MgCl2, 10 glucose and 200 sucrose. Coronal slices (300 μm) were cut using a VT1000S vibratome (Leica Microsystems, Wetzlar, Germany) at 3–4°C. Slices containing SCN were incubated 1–2 h at 25°C in oxygenated artificial cerebrospinal fluid (ACSF) containing (in mM): 125 NaCl, 1.2 MgSO4, 26 NaHCO3, 1.25 Na2HPO4, 3.5 KCl, 2.5 CaCl2 and 10 D-glucose (300–305 mOsm/kg). Slices were transferred to the recording chamber and perfused via gravity flow at 1–2 ml min−1 with oxygenated ACSF.
Recordings were performed at the peak (ZT 4–8 h) and nadir (ZT 17–21 h) of the circadian rhythm in spontaneous action potential firing, corresponding to the “day” and “night” timepoints, respectively. Neurons within the center of the SCN were identified in whole-cell current-clamp mode by spontaneous action potential firing or firing following injection of 5–20 pA of current for silent neurons.
Macroscopic BK and Ca2+ currents were recorded in whole-cell voltage-clamp mode at 25°C as described previously (Whitt et al., 2018; McNally et al., 2020). For BK currents, electrodes (4–7 MΩ) were filled with intracellular solution (in mM): 123 K-methanesulfonate, 9 NaCl, 0.9 EGTA, 9 HEPES, 14 Tris-phosphocreatine, 2 Mg-ATP, 0.3 Tris-GTP, and 2 Na2-ATP, pH 7.3 (310–315 mOsm/kg). BAPTA (5 mM) was substituted for EGTA (0.9 mM) in some internal solutions as specified in figure legends. The bath ACSF was composed of (in mM): 125 NaCl, 1.2 MgSO4, 26 NaHCO3, 1.25 Na2HPO4, 3.5 KCl, 2.5 CaCl2 and 10 D-glucose (300–305 mOsm/kg). For Ca2+ currents, the internal solution was (in mM): 115 cesium gluconate, 10 tetraethylammonium chloride, 10 HEPES, 0.5 EGTA, 2 MgCl2, 20 sodium phosphocreatine, 2 Na2ATP, and 0.3 Na3 GTP (pH 7.3, 310–315 mOsm/kg). The bath ACSF was composed of (in mM): 68 NaCl, 3.5 KCl, 1 NaH2PO4, 26.2 NaHCO3, 1.3 MgSO4, 2.5 CaCl2, 10 D-glucose, 60 tetraethylammonium chloride, and 3 CsCl (300–305 mOsm/kg).
Total voltage-activated BK and Ca2+ currents were recorded in 1 μM tetrodotoxin (TTX) before and after focal perfusion (4-min wash-on) of the selective inhibitors paxilline (BK channels) or nimodipine (L-type Ca2+channels), respectively. A minimum 10–15-min wash-out period of focally and bath perfused ACSF was performed before recording from the next cell. BK and L-type Ca2+ currents were isolated by subtracting currents elicited in the presence of their respective inhibitors, Nimodipine and Paxilline respectively, from total baseline currents. Three currents were averaged per cell and normalized to cell capacitance (range of 5–10 pF). Ra was <25 MΩ with less than ± 5% change (on average ~15 MΩ). Rs was compensated at 60%. BK currents were elicited from a holding potential of −90 mV, stepping from −110 to 90 mV for 150 ms in 20-mV increments. Ca2+ currents were elicited from a holding potential of −90 mV, stepping from −90 to 50 mV for 150 ms in 10-mV increments. Only cells with a total current size of >100 pA were used in experiments. Voltage values were adjusted for the liquid junction potential (9 mV). Currents were post hoc filtered at 1 kHz.
Drugs used in these experiments were: L-type Ca2+ channel inhibitor nimodipine (Nim, 10 μM, Alomone Labs, Jerusalem, Israel, #N-150), Ryanodine Receptor inhibitor dantroline (Dan, 10 μM, Sigma, #D9175), BK current inhibitor Paxilline (Pax, 10 μM, Alomone Labs, Jerusalem, Israel, #P-450) and Sodium channel inhibitor tetrodotoxin (TTX, 1 μM, Alomone Labs, Jerusalem, Israel, #T-550). All drugs were dissolved in DMSO, except TTX, which was dissolved in water. Drugs were focally perfused to the bath at a flow rate of 1 ml min−1 by a computer-controlled pressurized perfusion system (ValveLink 8.2; Automate Scientific, Berkeley, CA, USA) at the concentrations indicated from 1,000× stocks.
In whole-cell current-clamp mode, membrane potential oscillations were recorded in TTX (1 μM) using the same solutions as BK current recordings (McNally et al., 2020). Cells with resting membrane potentials between −30 and 65 mV were included in the dataset. Data were acquired in 10-s sweeps at the cell resting membrane potential (spontaneous oscillations) and during a series of holding potentials stepping from −60 to 0 mV, in 10 mV increments (voltage-dependent oscillations). Oscillations were defined as at least a 5-mV change in membrane potential, with a frequency of 0.2 Hz. Representative traces in figures were at 2 kHz.
BK and Ca2+ current-voltage plots were constructed from peak current level at each voltage step. Data are reported as group mean ± SEM. Numbers reported in figure legends are the number of neurons recorded, with 1–6 neurons per animal (one SCN slice per animal). Data for each condition was derived from a minimum of two animals. Statistical significance was determined at P < 0.05 using Prism v7 (GraphPad Software, Inc) using unpaired Welch's t tests, one-way ANOVA with Bonferroni's post hoc test, and Fisher's exact test for categorical data as indicated in results and figure legends. Representative current traces in figures were filtered at 500 Hz.
L-type Ca2+ current is larger during the day compared to night in SCN neurons (Pennartz et al., 2002; Whitt et al., 2018; McNally et al., 2020). In conjunction with the diurnal modulation of LTCC current, BK current is predominantly dependent upon LTCCs during the day. However, LTCCs do not significantly contribute to BK current activation at night, suggesting the coupling between BK channels and their Ca2+ channel sources could differ between day and night (Whitt et al., 2018). To examine this idea, C57BL6 WT BK currents were recorded in two Ca2+ buffering conditions. As a control, BK currents were first recorded under standard whole-cell voltage-clamp conditions with 0.9 mM EGTA in the internal solution, conditions which permit the endogenous Ca2+ sources to contribute to BK current activation (Fakler and Adelman, 2008; Whitt et al., 2018; Plante et al., 2021). These BK currents recorded with 0.9 mM EGTA were then compared to BK currents recorded with internal solution containing 5 mM BAPTA, a Ca2+ chelator with fast kinetics that disrupts the functional coupling of BK channels located >10–20 nm from their Ca2+ source (Berkefeld et al., 2006; Fakler and Adelman, 2008; Cox, 2014). If the coupling between BK channels and their Ca2+ channel sources differs between day and night, BAPTA would be expected to reduce BK current below levels recorded in the EGTA control conditions.
Daytime BK currents were not reduced in BAPTA compared to EGTA (Figures 1A,C,E), suggesting tight functional coupling and close spatial localization between BK channels and their daytime Ca2+ source. However, in contrast to daytime, at night BK current was reduced ~37% in BAPTA (Figures 1B,D,E). This suggests that some BK channels do not remain closely localized with their Ca2+ source at night. This change in BAPTA sensitivity was correlated with a change in the Ca2+ source that activates BK channels (Whitt et al., 2018). During the day, LTCCs are responsible for the majority of BK current activation. At night BK activation relies primarily on release of Ca2+ from intracellular stores through RyRs. This differential activation can be probed with inhibitors for these Ca2+ sources.
Figure 1. Effects of Ca2+ chelators and Ca2+ channel inhibitors on BK currents recorded from SCN neurons during the day and night. Paxilline-sensitive macroscopic BK currents were recorded from C57BL6 WT (A–E) and CaV1.3 WT (F) SCNs. All intracellular solutions in this study were made with 0.9 mM EGTA, except where 5 mM BAPTA was substituted (A–E). Currents were elicited from a holding potential of −90 mV by 150-ms voltage steps from −110 to +90 mV in +20-mV increments. (A,B) Representative BK currents from −90 to +90 mV are shown from day (A) and night (B) SCN neurons. (C,D) Current-voltage plot comparing BK current density recorded in either control EGTA or BAPTA during the day (C) and night (D). (E) Summary of BK current density at +90 mV recorded with control EGTA or BAPTA with Ca2+ channel inhibitors 10 μM nimodipine (Nim) during the day, or 10 μM dantrolene (Dan) at night. In EGTA, Nim decreased BK currents compared to controls (P = 0.0008), with no significant difference in BAPTA. At night, BK currents were decreased with BAPTA compared to control EGTA conditions (P < 0.0001). Then Dan decreased BK currents in control EGTA (P = 0.001), but BAPTA was not significant. (F) Ca2+ channel inhibitor sensitivity in CaV1.3 WT SCN neurons. Summary of BK current density at +90 mV recorded with EGTA in control (no drug) conditions, or in the presence of Nim or Dan in day and night. CaV1.3 WT BK currents were decreased in Nim during the day compared to controls (P = 0.01), but not Dan. At night, CaV1.3 WT BK currents were decreased in Dan compared to control (P = 0.008) and Nim (P < 0.0001), but Nim and control were not different. *P < 0.05, One-way ANOVA with Bonferroni's post hoc test between all conditions within day or night. N's represent individual cells recorded from C57BL6 WT (EGTA, BAPTA) day: control (11 neurons, three slices; six neurons, two slices); Nim (seven neurons, two slices; four neurons, one slice) and night: control (12 neurons, three slices; seven neurons, two slices); Dan (five neurons, two slices; three neurons, one slice). CaV1.3 WT (day, night): control (11 neurons, six slices; 12 neurons, three slices), Nim (14 neurons, four slices; 13 neurons, four slices), and Dan (five neurons, two slices; seven neurons, two slices). Data are mean ± SEM.
Consistent with previous studies, daytime BK current was sensitive to inhibition by nimodipine, a selective inhibitor of LTCCs, in control EGTA (Figure 1E) (Whitt et al., 2018). In the presence of BAPTA, the reduction in BK current was not statistically significant (Figure 1E). At night, BK current in control EGTA was sensitive to inhibition by dantrolene, a selective inhibitor of RyR Ca2+ release from intracellular stores. In BAPTA, a similar result to daytime recordings was observed, in that this condition also lacked significance (Figure 1E). Thus, taken together, the inhibitor sensitivity in BAPTA still leaves open whether co-localized BK-Ca2+ channel complexes contain either LTCCs during the day or RyRs at night. During the day, part of the lack of a definitive effect could be due to the potential for multiple CaV1 isoforms (CaV1.2 and CaV1.3) to contribute to the Ca2+ current in SCN neurons (Nahm et al., 2005; Huang et al., 2012; Kim et al., 2015; Cheng et al., 2018).
Given the influence of CaV1.3 channels on firing rate and membrane oscillations during the day (Huang et al., 2012), and BK's ability to partner with CaV1.3 without requiring other proteins in heterologous cells (Vivas et al., 2017; Plante et al., 2021), we first examined the specific contribution of CaV1.3 to BK current activation by recording from CaV1.3 KO SCNs (Platzer et al., 2000). CaV1.3 KO mice were obtained on a C57BL6:FVB background, and CaV1.3 WT littermates on this mixed strain background were used as controls. First, the changeover in Ca2+ source was verified in this strain from recordings in CaV1.3 WT SCNs. As with inbred C57BL6 SCN neurons (EGTA recordings, Figure 1E) (Whitt et al., 2018), CaV1.3 WT daytime BK currents were sensitive to inhibition by nimodipine, while dantrolene had a negligible effect (Figure 1F). At night, BK currents became nimodipine-insensitive, but dantrolene-sensitive (Figure 1F). This demonstrates that daily changeover in BK channel activation by its Ca2+ source occurs on the mixed strain background harboring the CaV1.3 transgene.
Next, the Ca2+ currents were characterized in CaV1.3 WT and KO SCNs to determine if loss of CaV1.3 channels results in decreased Ca2+ current (Figure 2). Ca2+ currents (Figures 2A,E) were recorded using the voltage protocol shown in Figure 2E. In daytime CaV1.3 WT SCN neurons, the peak nimodipine-sensitive current is 42% of the total current. At night, this decreases to 16% (Figures 2B,C). This differential contribution generated a day vs. night difference in the nimodipine-sensitive LTCC current (Figure 2D) that is consistent with previous studies on other strain backgrounds (Pennartz et al., 2002; Whitt et al., 2018; McNally et al., 2020).
Figure 2. Nimodipine-sensitive Ca2+ currents from CaV1.3 WT and CaV1.3 KO SCN during the day and night. Macroscopic Ca2+ currents were elicited from a holding potential of −90 mV by 150-ms voltage steps from −90 to +50 mV in +10-mV increments. Nimodipine-sensitive LTCC currents were isolated by subtracting currents obtained in 10 μM nimodipine from the total cell current. (A,E) Representative nimodipine-sensitive (Nim-sens) current traces from CaV1.3 WT (A) and CaV1.3 KO (E) neurons during the day (top current traces) and night (bottom). (B,C) Current-voltage plot for CaV1.3 WT Ca2+ currents before nimodipine (total) and the nimodipine-sensitive (Nim-sens) current from day (B) and night (C) neurons. (D) Comparison of CaV1.3 WT nimodipine-sensitive normalized current density between day and night. CaV1.3 WT nimodipine-sensitive currents were larger during the day (at −10 mV) compared to night (at 0 mV) (P = 0.01). (E) Representative nimodipine-sensitive currents from CaV1.3 KO neurons during the day (top current traces) and night (bottom). (F,G) Current-voltage plot of CaV1.3 KO total and nimodipine-sensitive currents from day (F) and night (G) neurons. (H) Comparison of CaV1.3 KO nimodipine-sensitive normalized current density between day and night. CaV1.3 KO nimodipine-sensitive currents were not different between day (at 0 mV) and night (at −10 mV) (P = 0.2). (I,J) Comparisons of nimodipine-sensitive current densities from CaV1.3 WT and CaV1.3 KO SCN during the day (I) and night (J). CaV1.3 KO nimodipine-sensitive currents were smaller than CaV1.3 WT currents during the day (P = 0.009) but not at night (P = 0.5). P < 0.05, unpaired Welch's t tests. N's represent individual cells recorded from CaV1.3 WT (eight neurons, three slices day; 10 neurons, four slices night) and CaV1.3 KO (12 neurons, two slices day; nine neurons, one slice night). Data are mean ± SEM.
In the absence of CaV1.3 channels, there was no change in the total Ca2+ current in the CaV1.3 KO compared to WT (at −10 mV, P = 0.59 during the day and P = 0.76 at night, respectively; unpaired t-test) (Figures 2F,G). However, the peak nimodipine-sensitive current was reduced during the day in CaV1.3 KO cells compared to WT (Figure 2I), comprising only 23% of the total Ca2+ current (Figures 2E,F). In addition, the peak of the daytime current-voltage relationship for the nimodipine-sensitive current also shifts from −10 mV (CaV1.3 WT) to 0 mV (CaV1.3 KO), consistent with loss of the low voltage activating CaV1.3 channels (Figure 2I). Together these changes in the voltage-dependence and current magnitude identify that CaV1.3 channels contribute to the daytime LTCC current in SCN neurons.
At night, the contribution of the nimodipine-sensitive current to the total Ca2+ current in CaV1.3 KO neurons was similar to daytime contribution (22%, Figures 2G,H). Unlike CaV1.3 WT, the relative nimodipine-sensitive current to the total current was not smaller at night. Moreover, there was no shift toward depolarizing potentials of the peak voltage of the nimodipine-sensitive current-voltage relationship compared to CaV1.3 WT (Figure 2J). Taking into account the lack of change in both the total and nimodipine-sensitive currents in CaV1.3 KO neurons, the results raise the possibility that the loss of CaV1.3 channels might be homeostatically compensated by other LTCCs such as Cav1.2. Thus, the nighttime data are less conclusive and leave open the question of whether CaV1.3 contributes to the night LTCC Ca2+ current in SCN neurons.
CaV1.3 KO SCNs thus exhibited a different day vs. night profile for LTCC current magnitude. The daytime change in nimodipine-sensitive current magnitude eliminated the diurnal variation in LTCC current in CaV1.3 KO neurons. Whereas WT neurons had a larger LTCC current during the day (Figure 2D), CaV1.3 KO neurons showed an abrogation of this diurnal difference (Figure 2H). This observation primarily resulted from the decreased daytime nimodipine-sensitive current in CaV1.3 KO compared to WT, which was reduced by about half. The net effect was a loss of the diurnal variation in LTCC current in CaV1.3 KO SCNs.
Another property of SCN neurons involving LTCCs is the generation of spontaneous subthreshold membrane potential oscillations. During the day, the frequency of these oscillations is higher, and they are eliminated by nimodipine, demonstrating their dependence on LTCC function (Pennartz et al., 2002; Jackson et al., 2004; Huang et al., 2012; McNally et al., 2020). CaV1.3 channels have been proposed to underlie these oscillations due to their activation at subthreshold membrane potentials. Alteration of Ca2+-dependent inactivation of CaV1.3 channels is correlated with a decrease in the membrane potential oscillation frequency (Huang et al., 2012). In this study, membrane potential oscillations were revealed in CaV1.3 WT neurons during the day by application of TTX. SCN neurons exhibit a wide range of oscillatory behavior (Figure 3) (Pennartz et al., 2002; Jackson et al., 2004; McNally et al., 2020).
Figure 3. Membrane potential oscillations in CaV1.3 WT and CaV1.3 KO SCN neurons. (A,B) Representative current-clamp recordings of membrane potential oscillations from CaV1.3 WT (A) and CaV1.3 KO (B) neurons recorded during the day. Recordings were made in 1 μM TTX using the same ACSF and 0.9 mM control EGTA intracellular solution used to record BK currents. Dotted lines indicate −40 mV.
Just over half (56%) of CaV1.3 WT neurons exhibited membrane potential oscillations. Fifteen neurons had spontaneous oscillations, and three neurons had oscillations manifesting with voltage steps from −60 to 0 mV (out of 32 neurons from three slices; Figure 3A). The average resting membrane potential was −44.4 ± 1.8 mV (n = 47). CaV1.3 KO neurons also exhibited oscillatory membrane behavior (Figure 3B). Fifty seven percent of CaV1.3 KO neurons exhibited oscillations (six neurons with spontaneous and two neurons with oscillations from voltage-steps, out of 14 neurons total from one slice). The average CaV1.3 KO neuron resting membrane potential was −39.8 ± 2.2 mV (n = 14), which was not different from WT (P = 0.06, unpaired t-test). These results demonstrate that CaV1.3 is not the sole Ca2+ channel required to produce spontaneous membrane potential oscillations or regulate resting Ca2+-dependent K+ conductances in SCN neurons.
Because CaV1.3 makes a clear contribution to the daytime nimodipine-sensitive current (Figure 2), corresponding to the time of day when BK current is more sensitive to nimodipine inhibition (Figure 1F), we investigated the role of this LTCC channel subtype in BK current activation (Figure 4). BK current levels were first quantified at +90 mV, where the largest diurnal difference is routinely quantified (Montgomery and Meredith, 2012; Whitt et al., 2016). We verified that BK current is larger at night in CaV1.3 WT SCN neurons on the mixed strain background (Figure 4A), consistent with previous studies on inbred mouse backgrounds (Pitts et al., 2006; Montgomery and Meredith, 2012; Montgomery et al., 2013). Most CaV1.3 WT neurons had a BK current, both during the day and at night (Figure 4B). In CaV1.3 KO neurons, the day vs. night difference in BK current level also persists in the absence of CaV1.3 channels (Figure 4A). Although the proportion of CaV1.3 KO neurons exhibiting a BK current was lower at 64% during the day, and was similar proportionally to CaV1.3 WT neurons with nimodipine applied, this difference compared to WT was not significant (Figure 4B). At night, all CaV1.3 KO neurons had a BK current. These data show that CaV1.3 is not absolutely required to activate BK currents in most neurons, or for the overall circadian difference in BK current magnitude in the SCN.
Figure 4. BK currents from CaV1.3 WT and CaV1.3 KO SCN during the day and night. Paxilline-sensitive macroscopic BK currents were recorded as in Figure 1. (A) Summary of BK current densities at +90 mV recorded from CaV1.3 WT and CaV1.3 KO neurons during the day and night. BK currents were increased at night for both CaV1.3 WT (P = 0.004) and CaV1.3 KO (P = 0.007) compared to the day. *P < 0.05, unpaired Welch's t tests. (B) Percentage of SCN neurons with BK current in CaV1.3 WT and CaV1.3 KO neurons recorded in control conditions or in 10 μM nimodipine (Nim) during the day and night. The number of neurons exhibiting BK currents was not different between CaV1.3 WT and CaV1.3 KO (P = 0.14, Fischer's exact test). N's: CaV1.3 WT, 12/13 (cell with BK current/total number recorded); CaV1.3 WT Nim, 10/14; CaV1.3 KO, 7/11 and night: CaV1.3 WT, 12/12; CaV1.3 WT Nim, 13/13; CaV1.3 KO, 6/6. (C,D) Current-voltage plot for normalized BK current densities from CaV1.3 WT and CaV1.3 KO neurons in control conditions (C) and after application of Nim (D) during the day. CaV1.3 WT BK currents were increased at +90 mV compared to CaV1.3 KO in control conditions (P = 0.03) but not in Nim (P = 0.6). *P < 0.05, Repeated measures ANOVA with Bonferroni's post hoc test between WT and KO across −10 to +90 mV. (E) Current-voltage plot for BK current densities from CaV1.3 WT and CaV1.3 KO at night, in control and after application of Nim. CaV1.3 KO BK currents were increased at +30 (P = 0.007), +50 (P = 0.002) and +70 mV (P = 0.01) compared to CaV1.3 WT in control conditions at night. N's represent individual cells recorded in (day, night): CaV1.3 WT (13 neurons, six slices; 12 neurons, three slices), CaV1.3 WT Nim (14 neurons, four slices; 13 neurons, four slices), and CaV1.3 KO (11 neurons, four slices; six neurons, two slices), and CaV1.3 KO Nim (three neurons, one slice; three neurons, two slices). Data are mean ± SEM.
In whole-cell recordings, multiple Ca2+ channel subtypes can contribute to BK current activation. Although CaV1.3 KO neurons with BK currents do not require CaV1.3 channels, these channels may still contribute to BK current activation in combination with other LTCC subtypes (such as CaV1.2). This possibility was addressed by examining the current-voltage relationships for CaV1.3 WT and KO BK currents. In this comparison for daytime SCN neurons, there was little difference in BK current levels across the voltage range, except at the highest voltage (Figure 4C). Although this reduction could suggest contribution from CaV1.3 channels to BK current activation, the peak Ca2+ influx due to CaV1.3 channels occurs around −20 to −10 mV. For BK channels activated directly by CaV1.3 Ca2+ currents, the largest relative BK current activation is thus observed between −10 and 0 mV (Vivas et al., 2017; Plante et al., 2021). Lack of a difference in BK current observed at these voltages suggests that CaV1.3 channels may not make a notable contribution to BK current activation in SCN neurons during the day.
When BK channels are coupled to the other neuronal L-type Ca2+ channel subtype, CaV1.2, the BK current activation profile is shifted to higher voltages (+30 mV) (Berkefeld et al., 2006; Plante et al., 2021). In the CaV1.3 KO, the contribution of CaV1.2 channels to BK current activation can be indirectly inferred by applying nimodipine. Inhibiting the remaining LTCC current in CaV1.3 KO neurons reduced BK current levels (at +50 mV, P = 0.006, two-way repeated measures ANOVA with Bonferroni's post hoc test) (Figure 4C vs. D), suggesting that it can be attributed to CaV1.2 channels. However, in the presence of nimodipine, BK current levels were not significantly different between CaV1.3 WT and KO neurons (Figure 4D). The reduction in BK current to similar levels with nimodipine in both genotypes indirectly suggests that there is little compensatory upregulation of CaV1.2 that affects BK current during the day.
At night, there was a paradoxical increase in BK current in CaV1.3 KO neurons compared to CaV1.3 WT (Figure 4E). However, in this case, the increase likely comes from compensatory upregulation of CaV1.2, since nimodipine reduces BK current back to WT levels. This result precludes formulating conclusions about the contribution of CaV1.3 to BK current activation in nighttime neurons in this study. Yet because nimodipine normally does not significantly decrease the nighttime BK current level in WT neurons in this (Figure 1F) and prior studies (Whitt et al., 2018), it is reasonable to conclude that there is no major role for CaV1.3 channels in BK current activation at night.
CaV1.3 has been proposed to play a role in circadian excitability based on its expression and functional modulation in SCN neurons (Huang et al., 2012; Kim et al., 2015; Cheng et al., 2018). Here we show that CaV1.3 channels contribute to the daytime LTCC current in SCN neurons. Their contribution may account for up to half of the LTCC current during the day, providing part of the basis for the diurnal difference in Ca2+ current levels. In contrast, it is not clear whether CaV1.3 contributes to daytime membrane potential oscillations or contributes to the nighttime LTCC current. Spontaneous Ca2+ oscillations are dependent on the function of LTCCs (Pennartz et al., 2002; Jackson et al., 2004; Huang et al., 2012; McNally et al., 2020). Although CaV1.3 has been proposed to be important for generating these oscillations (Huang et al., 2012; Comunanza et al., 2014), the presence of membrane oscillations in CaV1.3 KO neurons posits that other Ca2+ channels are also competent to generate them. Moreover, at night where there is no reduction of the nimodipine-sensitive Ca2+ current in CaV1.3 KO cells and no change in the total Ca2+ current, it suggests that CaV1.3 does not make a significant contribution to the nighttime LTCC current in SCN neurons. However, the data do not completely rule out the possibility of a compensatory upregulation of another LTCC channel subtype, such as CaV1.2, that is sufficient to maintain the nighttime Ca2+ current in CaV1.3 KO SCNs at a similar level to that observed for CaV1.3 WT. This compensation is further suggested by the increase in BK current observed in CaV1.3 KO neurons at night. In other tissues, compensation has been shown to occur via upregulation of CaV1.2 in the absence of CaV1.3 function (Namkung et al., 2001; Zhang et al., 2005; Marcantoni et al., 2010; Jurkovičová-Tarabová et al., 2012; Poetschke et al., 2015).
BK channels are Ca2+-activated, and LTCCs are a major source of Ca2+ required for their activation during the day in SCN neurons (Whitt et al., 2018). Several factors motivated the specific investigation of CaV1.3 channels in BK current activation. In several types of excitable cells, in heterologous cells, and across diverse animal species, BK and CaV1.3 channels have been shown to functionally couple (Grunnet and Kaufmann, 2004; Berkefeld et al., 2006; Marcantoni et al., 2010; Bellono et al., 2017; Vivas et al., 2017; Plante et al., 2021). In this study, we found that while the contribution of CaV1.3 channels to the daytime LTCC current is significant, the effect on BK current is more limited. First, the day vs. night difference in BK current levels that is critical for maintaining proper circadian rhythm in SCN activity is still expressed in the CaV1.3 KO. This stands in contrast to the LTCC current recorded from CaV1.3 KO neurons, which does not show a diurnal difference in levels. Second, many SCN neurons still possess detectable BK currents and retain mostly normal BK current activation in the CaV1.3 KO. Lastly, in prior behavioral studies, daily patterns of locomotor activity were shown to be essentially normal in CaV1.3 KO mice on a regular light:dark cycle (Busquet et al., 2010). If CaV1.3 were a fundamental source of Ca2+ for BK channel activation, some disruption of locomotor activity might be expected, along the lines of the alterations in behavioral rhythms observed due to loss of BK channel function (Meredith et al., 2006). Taken together, these data suggest that CaV1.3 channels are not the predominant LTCCs contributing to the net steady-state BK current levels under these recording conditions.
Yet the contribution of CaV1.3 channels could be more subtle or underestimated. BK–CaV1.3 channel complexes expressed in heterologous cells were sensitive to low concentrations of BAPTA (0.1 mM) (Vivas et al., 2017), while the SCN BK currents in this study were resistant to higher concentrations of BAPTA (5 mM). This raises the possibility that the predominant BK—LTCC complex in SCN neurons is more tightly coupled than BK–CaV1.3 channel complexes, making their contribution harder to assess. There were also some minor changes in BK currents from daytime SCN neurons, such as a trend toward fewer neurons with detectable BK currents and a drop-off in the BK current at the peak voltage tested compared to WT. The lack of significance in this data could result from the variability in the Ca2+ currents recorded between SCN neurons (Whitt et al., 2018; McNally et al., 2020). Moreover, it is possible that the major contribution for CaV1.3 channels could be in the smaller population of neurons that had no BK currents in CaV1.3 KO neurons. Alternatively, the changes in BK current observed at +90 mV in the CaV1.3 KO could reflect an indirect role for the channel, such as in the gating of RyR-mediated Ca2+ release. A small portion of daytime SCN BK current has been previously reported to be sensitive to dantrolene (Whitt et al., 2018), but the pathway mediating the opening of RyRs is not currently understood in SCN neurons (Harvey et al., 2020). Lastly, CaV1.3 is less sensitive nimodipine than CaV1.2 (Xu and Lipscombe, 2001), raising the possibility that a small contribution to BK current could be lost within the variability of Ca2+ currents from cell to cell.
The data in this study indirectly suggest CaV1.2 as a more significant LTCC for providing the Ca2+ influx that activates BK channels in the daytime SCN. BK current activation is preserved to a large extent when CaV1.3 channels are absent, and a portion of that current is sensitive to nimodipine. BK channels are also well-described to couple to CaV1.2 channels in a variety of cell types, including in neurons and heterologous cells (Berkefeld et al., 2006, 2010; Berkefeld and Fakler, 2008; Hou et al., 2016; Plante et al., 2021). Besides CaV1.3, CaV1.2 is likely the relevant LTCC subtype to consider in SCN neurons, since CaV1.1 is restricted to skeletal muscle and CaV1.4 to retina (Lipscombe et al., 2004; Lee et al., 2015).
A factor complicating some interpretations in this study include the issue of LTCC compensation in the CaV1.3 KO. Substantia nigra and lateral superior olive neurons, as well as adrenal chromaffin cells, exhibit upregulation of other Ca2+ currents in CaV1.3 KOs (Marcantoni et al., 2010; Jurkovičová-Tarabová et al., 2012; Poetschke et al., 2015). A distinct CaV1.3 KO mouse line also exhibited compensatory upregulation of CaV1.2 channels in heart tissues and pancreatic β-cells (Namkung et al., 2001; Zhang et al., 2005), suggesting CaV1.3 and CaV1.2 have a connected expression relationship. In this study, the increased BK current at night in CaV1.3 KO neurons comes from LTCC compensation, since it is nimodipine sensitive. However, the total and nimodipine-sensitive Ca2+ currents did not increase compared to WT, as expected if there were compensation. This observation leaves open the question of whether LTCC compensation actually obscured a decrease in the CaV1.3 KO LTCC current at night. While this issue makes it difficult to assess the role for CaV1.3 in the nighttime Ca2+ current, it is unlikely to affect the conclusion that it does not play a role in BK current activation at night. Interestingly, the increase in BK current at night suggests that when extra LTCC channels are made, they can aberrantly couple to BK channels at the wrong time of day. Since it is not yet known how BK channels change their functional Ca2+ channel associations over the circadian cycle in SCN (Whitt et al., 2018; Harvey et al., 2020), the CaV1.3 KO model may provide a new context to test mechanisms for BK–CaV coupling.
The raw data supporting the conclusions of this article will be made available by the authors, without undue reservation.
The animal study was reviewed and approved by the Institutional Animal Care and Use Committee at the University of Maryland, Baltimore.
BM performed the experiments. BM, AP, and AM analyzed the data. AM wrote the manuscript. All authors contributed to the article and approved the submitted version.
The authors declare that the research was conducted in the absence of any commercial or financial relationships that could be construed as a potential conflict of interest.
All claims expressed in this article are solely those of the authors and do not necessarily represent those of their affiliated organizations, or those of the publisher, the editors and the reviewers. Any product that may be evaluated in this article, or claim that may be made by its manufacturer, is not guaranteed or endorsed by the publisher.
We thank Jörg Striessnig and Geoff Pitt for providing CaV1.3 heterozygous breeding pairs, and Ivy Dick for helpful advice and comments on the manuscript. This work was supported by grants from NHLBI R01-HL102758 (AM) and NIGMS T32GM008181 (AM and AP).
Bellono, N. W., Leitch, D. B., and Julius, D. (2017). Molecular basis of ancestral vertebrate electroreception. Nature 543, 391–396. doi: 10.1038/nature21401
Berkefeld, H., and Fakler, B. (2008). Repolarizing responses of BKCa-CaV complexes are distinctly shaped by their CaV subunits. J. Neurosci. 28, 8238–8245. doi: 10.1523/JNEUROSCI.2274-08.2008
Berkefeld, H., Fakler, B., and Schulte, U. (2010). Ca2+-activated K+ channels: from protein complexes to function. Physiol. Rev. 90, 1437–1459. doi: 10.1152/physrev.00049.2009
Berkefeld, H., Sailer, C. A., Bildl, W., Rohde, V., Thumfart, J. O., Eble, S., et al. (2006). BKCa-CaV channel complexes mediate rapid and localized Ca2+-activated K+ signaling. Science 314, 615–620. doi: 10.1126/science.1132915
Busquet, P., Nguyen, N. K., Schmid, E., Tanimoto, N., Seeliger, M. W., Ben-Yosef, T., et al. (2010). CaV1.3 L-type Ca2+ channels modulate depression-like behaviour in mice independent of deaf phenotype. Int. J. Neuropsychopharmacol. 13, 499–513. doi: 10.1017/S1461145709990368
Cheng, P. C., Wang, Y. C., Chen, Y. S., Cheng, R. C., Yang, J. J., and Huang, R. C. (2018). Differential regulation of nimodipine-sensitive and -insensitive Ca2+ influx by the Na+/Ca2+ exchanger and mitochondria in the rat suprachiasmatic nucleus neurons. J. Biomed. Sci. 25:44. doi: 10.1186/s12929-018-0447-z
Cloues, R. K., and Sather, W. A. (2003). Afterhyperpolarization regulates firing rate in neurons of the suprachiasmatic nucleus. J. Neurosci. 23, 1593–1604. doi: 10.1523/JNEUROSCI.23-05-01593.2003
Comunanza, V., Marcantoni, A., Vandael, D. H., Mahapatra, S., Gavello, D., Carabelli, V., et al. (2014). CaV1.3 as pacemaker channels in adrenal chromaffin cells: specific role on exo- and endocytosis? Channels 4, 440–446. doi: 10.4161/chan.4.6.12866
Cox, D. H. (2014). Modeling a Ca2+ channel/BKCa channel complex at the single-complex level. Biophys. J. 107, 2797–2814. doi: 10.1016/j.bpj.2014.10.069
Fakler, B., and Adelman, J. P. (2008). Control of KCa channels by calcium nano/microdomains. Neuron 59, 873–881. doi: 10.1016/j.neuron.2008.09.001
Grunnet, M., and Kaufmann, W. A. (2004). Coassembly of big conductance Ca2+-activated K+ channels and L-type voltage-gated Ca2+ channels in rat brain. J. Biol. Chem. 279, 36445–36453. doi: 10.1074/jbc.M402254200
Harvey, J. R. M., Plante, A. E., and Meredith, A. L. (2020). Ion channels controlling circadian rhythms in suprachiasmatic nucleus excitability. Physiol. Rev. 100, 1415–1454. doi: 10.1152/physrev.00027.2019
Hastings, M. H., Maywood, E. S., and Brancaccio, M. (2018). Generation of circadian rhythms in the suprachiasmatic nucleus. Nat. Rev. Neurosci. 19, 453–469. doi: 10.1038/s41583-018-0026-z
Hou, P., Xiao, F., Liu, H., Yuchi, M., Zhang, G., Wu, Y., et al. (2016). Extrapolating microdomain Ca2+ dynamics using BK channels as a Ca2+ sensor. Sci. Rep. 6:17343. doi: 10.1038/srep17343
Huang, H., Tan, B. Z., Shen, Y., Tao, J., Jiang, F., Sung, Y. Y., et al. (2012). RNA editing of the IQ domain in CaV1.3 channels modulates their Ca2+-dependent inactivation. Neuron 73, 304–316. doi: 10.1016/j.neuron.2011.11.022
Ikeda, M. (2004). Calcium dynamics and circadian rhythms in suprachiasmatic nucleus neurons. Neuroscientist 10, 315–324. doi: 10.1177/10738584031262149
Jackson, A. C., Yao, G. L., and Bean, B. P. (2004). Mechanism of spontaneous firing in dorsomedial suprachiasmatic nucleus neurons. J. Neurosci. 24, 7985–7998. doi: 10.1523/JNEUROSCI.2146-04.2004
Jurkovičová-Tarabová, B., Griesemer, D., Pirone, A., Sinnegger-Brauns, M. J., Striessnig, J., and Friauf, E. (2012). Repertoire of high voltage-activated Ca2+ channels in the lateral superior olive: functional analysis in wild-type, CaV1.3−/−, and CaV1.2DHP−/− mice. J. Neurophysiol. 108, 365–379. doi: 10.1152/jn.00948.2011
Kim, Y. S., Kim, Y. B., Kim, W. B., Lee, S. W., Oh, S. B., Han, H. C., et al. (2016). Histamine 1 receptor-Gβγ-cAMP/PKA-CFTR pathway mediates the histamine-induced resetting of the suprachiasmatic circadian clock. Mol. Brain 9:49. doi: 10.1186/s13041-016-0227-1
Kim, Y. S., Kim, Y. B., Kim, W. B., Yoon, B. E., Shen, F. Y., Lee, S. W., et al. (2015). Histamine resets the circadian clock in the suprachiasmatic nucleus through the H1R-CaV1.3-RyR pathway in the mouse. Eur. J. Neurosci. 42, 2467–2477. doi: 10.1111/ejn.13030
Lee, A., Wang, S., Williams, B., Hagen, J., Scheetz, T. E., and Haeseleer, F. (2015). Characterization of CaV1.4 complexes (α11.4, β2, and α2δ4) in HEK293T cells and in the retina. J. Biol. Chem. 290, 1505–1521. doi: 10.1074/jbc.M114.607465
Lipscombe, D., Helton, T. D., and Xu, W. (2004). L-type calcium channels: the low down. J. Neurophysiol. 92, 2633–2641. doi: 10.1152/jn.00486.2004
Marcantoni, A., Vandael, D. H., Mahapatra, S., Carabelli, V., Sinnegger-Brauns, M. J., Striessnig, J., et al. (2010). Loss of CaV1.3 channels reveals the critical role of L-type and BK channel coupling in pacemaking mouse adrenal chromaffin cells. J. Neurosci. 30, 491–504. doi: 10.1523/JNEUROSCI.4961-09.2010
McNally, B. A., Plante, A. E., and Meredith, A. L. (2020). Diurnal properties of voltage-gated Ca2+ currents in suprachiasmatic nucleus and roles in action potential firing. J. Physiol. 598, 1775–1790. doi: 10.1113/JP278327
Meredith, A. L., Wiler, S. W., Miller, B. H., Takahashi, J. S., Fodor, A. A., Ruby, N. F., et al. (2006). BK calcium-activated potassium channels regulate circadian behavioral rhythms and pacemaker output. Nat. Neurosci. 9, 1041–1049. doi: 10.1038/nn1740
Montgomery, J. R., and Meredith, A. L. (2012). Genetic activation of BK currents in vivo generates bidirectional effects on neuronal excitability. Proc. Natl. Acad. Sci. 109, 18997–19002. doi: 10.1073/pnas.1205573109
Montgomery, J. R., Whitt, J. P., Wright, B. N., Lai, M. H., and Meredith, A. L. (2013). Mis-expression of the BK K+ channel disrupts suprachiasmatic nucleus circuit rhythmicity and alters clock-controlled behavior- supplementary data. Am. J. Physiol. Cell Physiol. 304, C299–311. doi: 10.1152/ajpcell.00302.2012
Nahm, S. S., Farnell, Y. Z., Griffith, W., and Earnest, D. J. (2005). Circadian regulation and function of voltage-dependent calcium channels in the suprachiasmatic nucleus. J. Neurosci. 25, 9304–9308. doi: 10.1523/JNEUROSCI.2733-05.2005
Namkung, Y., Skrypnyk, N., Jeong, M. J., Lee, T., Lee, M. S., Kim, H. L., et al. (2001). Requirement for the L-type Ca2+ channel alpha1D subunit in postnatal pancreatic beta cell generation. J. Clin. Invest. 108, 1015–1022. doi: 10.1172/JCI200113310
Pennartz, C. M., De Jeu, M. T., Bos, N. P., Schaap, J., and Geurtsen, A. M. (2002). Diurnal modulation of pacemaker potentials and calcium current in the mammalian circadian clock. Nature 416, 286–290. doi: 10.1038/nature728
Pitts, G. R., Ohta, H., and Mcmahon, D. G. (2006). Daily rhythmicity of large-conductance Ca2+-activated K+ currents in suprachiasmatic nucleus neurons. Brain Res. 1071, 54–62. doi: 10.1016/j.brainres.2005.11.078
Plante, A. E., Whitt, J. P., and Meredith, A. L. (2021). BK channel activation by L-type Ca2+ channels CaV1.2 and CaV1.3 during the subthreshold phase of an action potential. J. Neurophysiol. 126, 427–439. doi: 10.1152/jn.00089.2021
Platzer, J., Engel, J., Schrott-Fischer, A., Stephan, K., Bova, S., Chen, H., et al. (2000). Congenital deafness and sinoatrial node dysfunction in mice lacking class D L-type Ca2+ channels. Cell 102, 89–97. doi: 10.1016/S0092-8674(00)00013-1
Poetschke, C., Dragicevic, E., Duda, J., Benkert, J., Dougalis, A., Dezio, R., et al. (2015). Compensatory T-type Ca2+ channel activity alters D2-autoreceptor responses of substantia nigra dopamine neurons from CaV1.3 L-type Ca2+ channel KO mice. Sci. Rep. 5:13688. doi: 10.1038/srep13688
Prakriya, M., and Lingle, C. J. (1999). BK channel activation by brief depolarizations requires Ca2+ influx through L- and Q-type Ca2+ channels in rat chromaffin cells. J. Neurophysiol. 81, 2267–2278. doi: 10.1152/jn.1999.81.5.2267
Roberts, W. M., Jacobs, R. A., and Hudspeth, A. J. (1990). Colocalization of ion channels involved in frequency selectivity and synaptic transmission at presynaptic active zones of hair cells. J. Neurosci. 10, 3664–3684. doi: 10.1523/JNEUROSCI.10-11-03664.1990
Schmutz, I., Chavan, R., Ripperger, J. A., Maywood, E. S., Langwieser, N., Jurik, A., et al. (2014). A specific role for the REV-ERBalpha-controlled L-type voltage-gated calcium channel CaV1.2 in resetting the circadian clock in the late night. J. Biol. Rhythms 29, 288–298. doi: 10.1177/0748730414540453
Seisenberger, C., Specht, V., Welling, A., Platzer, J., Pfeifer, A., Kuhbandner, S., et al. (2000). Functional embryonic cardiomyocytes after disruption of the L-type alpha1C (CaV1.2) calcium channel gene in the mouse. J. Biol. Chem. 275, 39193–39199. doi: 10.1074/jbc.M006467200
Striessnig, J., Koschak, A., Sinnegger-Brauns, M. J., Hetzenauer, A., Nguyen, N. K., Busquet, P., et al. (2006). Role of voltage-gated L-type Ca2+ channel isoforms for brain function. Biochem. Soc. Trans. 34, 903–909. doi: 10.1042/BST0340903
Sun, X., Gu, X. Q., and Haddad, G. G. (2003). Calcium influx via L- and N-type calcium channels activates a transient large-conductance Ca2+-activated K+ current in mouse neocortical pyramidal neurons. J. Neurosci. 23, 3639–3648. doi: 10.1523/JNEUROSCI.23-09-03639.2003
Vandael, D. H., Marcantoni, A., Mahapatra, S., Caro, A., Ruth, P., Zuccotti, A., et al. (2010). CaV1.3 and BK channels for timing and regulating cell firing. Mol. Neurobiol. 42, 185–198. doi: 10.1007/s12035-010-8151-3
Vivas, O., Moreno, C. M., Santana, L. F., and Hille, B. (2017). Proximal clustering between BK and CaV1.3 channels promotes functional coupling and BK channel activation at low voltage. Elife 6:e28029. doi: 10.7554/eLife.28029
Whitt, J. P., McNally, B. A., and Meredith, A. L. (2018). Differential contribution of Ca2+ sources to day and night BK current activation in the circadian clock. J. Gen. Physiol. 150, 259–275. doi: 10.1085/jgp.201711945
Whitt, J. P., Montgomery, J. R., and Meredith, A. L. (2016). BK channel inactivation gates daytime excitability in the circadian clock. Nat. Commun. 7:10837. doi: 10.1038/ncomms10837
Wisgirda, M. E., and Dryer, S. E. (1994). Functional dependence of Ca2+-activated K+ current on L- and N-type Ca2+ channels: differences between chicken sympathetic and parasympathetic neurons suggest different regulatory mechanisms. Proc. Natl. Acad. Sci. 91, 2858–2862. doi: 10.1073/pnas.91.7.2858
Xu, W., and Lipscombe, D. (2001). Neuronal CaV1.3alpha1 L-type channels activate at relatively hyperpolarized membrane potentials and are incompletely inhibited by dihydropyridines. J. Neurosci. 21, 5944–5951. doi: 10.1523/JNEUROSCI.21-16-05944.2001
Keywords: CACNA1D, KCNMA1, BK channel, L-type Ca2+ channel, circadian rhythm, Ca2+-activated K+ channel
Citation: McNally BA, Plante AE and Meredith AL (2021) Contributions of CaV1.3 Channels to Ca2+ Current and Ca2+-Activated BK Current in the Suprachiasmatic Nucleus. Front. Physiol. 12:737291. doi: 10.3389/fphys.2021.737291
Received: 06 July 2021; Accepted: 30 August 2021;
Published: 28 September 2021.
Edited by:
Yoshiaki Suzuki, Nagoya City University, JapanReviewed by:
Pietro Mesirca, INSERM U1191 Institut de Génomique Fonctionnelle (IGF), FranceCopyright © 2021 McNally, Plante and Meredith. This is an open-access article distributed under the terms of the Creative Commons Attribution License (CC BY). The use, distribution or reproduction in other forums is permitted, provided the original author(s) and the copyright owner(s) are credited and that the original publication in this journal is cited, in accordance with accepted academic practice. No use, distribution or reproduction is permitted which does not comply with these terms.
*Correspondence: Andrea L. Meredith, YW1lcmVkaXRoQHNvbS51bWFyeWxhbmQuZWR1
Disclaimer: All claims expressed in this article are solely those of the authors and do not necessarily represent those of their affiliated organizations, or those of the publisher, the editors and the reviewers. Any product that may be evaluated in this article or claim that may be made by its manufacturer is not guaranteed or endorsed by the publisher.
Research integrity at Frontiers
Learn more about the work of our research integrity team to safeguard the quality of each article we publish.