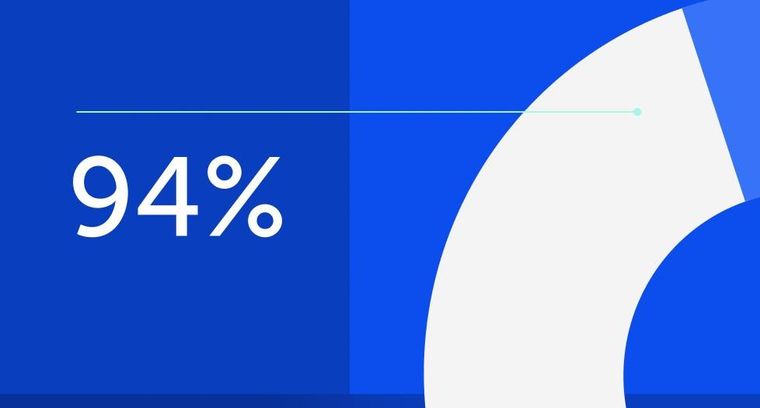
94% of researchers rate our articles as excellent or good
Learn more about the work of our research integrity team to safeguard the quality of each article we publish.
Find out more
REVIEW article
Front. Physiol., 08 October 2021
Sec. Membrane Physiology and Membrane Biophysics
Volume 12 - 2021 | https://doi.org/10.3389/fphys.2021.736681
This article is part of the Research TopicIon Channels and Transporters in Ca2+ -dependent Functions of LymphocytesView all 10 articles
Most cellular functions require of ion homeostasis and ion movement. Among others, ion channels play a crucial role in controlling the homeostasis of anions and cations concentration between the extracellular and intracellular compartments. Calcium (Ca2+) is one of the most relevant ions involved in regulating critical functions of immune cells, allowing the appropriate development of immune cell responses against pathogens and tumor cells. Due to the importance of Ca2+ in inducing the immune response, some viruses have evolved mechanisms to modulate intracellular Ca2+ concentrations and the mobilization of this cation through Ca2+ channels to increase their infectivity and to evade the immune system using different mechanisms. For instance, some viral infections require the influx of Ca2+ through ionic channels as a first step to enter the cell, as well as their replication and budding. Moreover, through the expression of viral proteins on the surface of infected cells, Ca2+ channels function can be altered, enhancing the pathogen evasion of the adaptive immune response. In this article, we review those ion channels and ion transporters that are essential for the function of immune cells. Specifically, cation channels and Ca2+ channels in the context of viral infections and their contribution to the modulation of adaptive immune responses.
Ion homeostasis must be strictly modulated in cells of the immune system since these charged atoms or molecules play critical roles in several different physiological aspects (Feske et al., 2015; Rubaiy, 2017). Cations are mainly represented by calcium (Ca2+), magnesium (Mg2+), zinc (Zn2+), potassium (K+), and sodium (Na+), and anions by chloride (Cl–), phosphate (HPO42–), and bicarbonate (HCO3–) (Feske et al., 2015; Rubaiy, 2017; Vaeth and Feske, 2018). Na+, K+, and Cl– usually maintain electrical gradients, and these cations contribute to the survival of the cell along with the movement of organelles, while Cl– concentration plays a role in the movement and the acidification process of organelles (Feske et al., 2012, 2015; Chakraborty et al., 2017; Murray et al., 2017; Rubaiy, 2017; Vaeth and Feske, 2018). Therefore, the mobilization of these charged atoms or molecules across the hydrophobic plasma membrane alters the electrochemical gradient in a localized fashion, inducing conformational changes that will influence the function of membrane proteins on those specific portions of the membrane (Feske et al., 2012, 2015; Chaigne-Delalande and Lenardo, 2014; Rubaiy, 2017; Vaeth and Feske, 2018). Given the relevance of ion channels and transporters in cellular functions, it is expected for immune cells, such as dendritic cells (DCs) and lymphocytes, to express several of these proteins for a specialized regulation of ion transport (Figure 1; Feske et al., 2012, 2015; Rubaiy, 2017; Vaeth and Feske, 2018). Overall, these channels are plasma membrane integral proteins that allow ions to transit from one side of the membrane to the other (Di Resta and Becchetti, 2010; Kim, 2014). Usually, cellular channels can be found in an open, resting closed, or inactivated closed state, in line with the physiological conditions and needs of the cell (Di Resta and Becchetti, 2010; Kim, 2014).
Figure 1. Ion channels and transporters expressed by dendritic cells and lymphocytes. The immune cells express on their surface various types of ion channels and transporters. CD4+ and CD8+ T cells express channels for Ca2+, Mg2+, K+, and Cl–, with fewer channels reported on CD8+ T cells. B cells express channels for Ca2+, Mg2+, and K+. Dendritic cells express Ca2+ channels.
Although currently there is not a thorough characterization regarding the modulation of ion channels and transporters in immune cells during infectious diseases, some reports have provided relevant information to understand better the contribution of these molecules during an infection (Cahalan and Chandy, 1997; Panyi et al., 2004; Feske et al., 2012, 2015; RamaKrishnan and Sankaranarayanan, 2016; Vaeth and Feske, 2018). It has been described that both, ion channels and transporters can regulate cation transport and modulate the immune response (Zweifach and Lewis, 1993; Lewis, 2001; Hogan et al., 2010; Feske et al., 2012). The most relevant ion channels and transporters include the Ca2+ release-activated Ca2+ (CRAC) channels, voltage-gated such as K+ channel (Kv)1.3 and Ca2+-activated K+ channel (KCa)3.1, transient receptor potential (TRP) channels, P2X receptors family and pannexin hemichannels, voltage-gated Ca2+, Na+, and H+ channels, and Mg2+ and Zn2+ channels, among others (Zweifach and Lewis, 1993; Lewis, 2001; Schenk et al., 2008; Franchi et al., 2009; Yip et al., 2009; Hogan et al., 2010; Woehrle et al., 2010; Junger, 2011; Feske et al., 2012; Prakriya and Lewis, 2015; Figure 2). One of the ion channels that regulates anion transport, and the immune response is the cystic fibrosis transmembrane conductance regulator (CFTR) and gamma-aminobutyric acid (GABA) receptors. In general, Ca2+ channel signaling starts with the influx of Ca2+ from the extracellular space, which triggers the activation of phospholipase Cβ (PLCβ) and the subsequent cleavage of phosphatidylinositol 4,5-bisphosphate (PIP2) into inositol (1,4,5) trisphosphate (IP3) and diacylglycerol (DAG) by PLCγ. After this, the binding of IP3 with to the IP3-receptor (IP3R) on the endoplasmic reticulum (ER) allows the diffusion of Ca2+ into the cytoplasm (Clapham, 2007). The following sections will discuss the contribution of ion channels and transporters to the interface between antigen-presenting cells, such as DCs and T cells, an interaction commonly known as immunological synapse. Further, the modulation of these channels during adaptive immune responses, diseases, channelopathies, and viral infections will be discussed.
Figure 2. Ion channels and signaling pathways described for T cells. Several channels responsible of regulating the ion homeostasis can be found on the surface of T cells. These channels maintain the different concentrations of ion in the extracellular and intracellular space and are permeable to specific ions. T cell receptor engagement with the pMHC molecule found on antigen presenting cells will induce a signaling cascade that will result on the influx of Ca2+ and eventual store-operated Ca2+ entry inside of T cells. All these events will result in the activation of different transcription factor that will activate the effector function of T cells.
T cells and B cells are the main players of the adaptive immune response and express various ion channels and transporters on their surface (Feske et al., 2012; Netea et al., 2019). These channels can regulate the concentration of different ions inside these cells and modulate their functions (Feske et al., 2012). This section will discuss the ion channels and transporters expressed by these lymphocytes.
T cells play an essential role at connecting the humoral and cellular arms of adaptive immunity, which is critical to properly response to infections by various pathogens (Jasenosky et al., 2015; Lee et al., 2020). T cells can be classified into two major types: CD4+ T cells that orchestrate the immune response against a specific pathogen, and CD8+ T cells that have cytotoxic properties and can induce the apoptosis of cells infected by a pathogen (Whitacre et al., 2012; Jasenosky et al., 2015). In general, these cells can be recognized for the T cell receptor found on their surface (Whitacre et al., 2012). Additionally, T cells express several ion channels and transporters that modulate the activation of these cells through the influx of Ca2+, Mg2+ and Zn2+, or the efflux of K+ and Cl– (Table 1; Feske, 2013; Feske et al., 2015).
CRAC channels contribute significantly to modulating immune cells, particularly the T cells, as these channels are mainly responsible for Ca2+ influx, promoting an increase in the concentration of this ion that can be maintained for hours after the initial opening of these channels (Lewis, 2001; Joseph et al., 2014). Ca2+ levels modulate the activation, proliferation, and cytokine secretion by T lymphocytes, among other relevant processes (reviewed later; Lewis, 2001). CRAC channels are located on the membrane of T cells and are composed of three proteins, ORAI1, ORAI2, and ORAI3 (Vaeth et al., 2020). CRAC channels are also the main Ca2+ channels activated on antigen-presenting cells upon antigen recognition, promoting DCs maturation in a Ca2+-controlled fashion (Prakriya and Lewis, 2015). Usually, Ca2+ concentration in the cytoplasm of resting lymphocytes is close to 50 to 100 nM (Figure 2). Upon T cell receptor or B cell receptor engagement, Ca2+ concentration in the cytoplasm can reach up to 1 μM (Lewis, 2001; Feske et al., 2012). The mechanisms underlying Ca2+ increase after T cell receptor and B cell receptor engagement involves the activation of PLCγ1 or PLCγ2 (Zweifach and Lewis, 1993; Hogan et al., 2003). The production of IP3 by PLCγ leads to the release of Ca2+ from the ER that will stimulate Ca2+ influx across the plasma membrane through the store-operated Ca2+ entry (Zweifach and Lewis, 1993; Hogan et al., 2010). CRAC channels are responsible for the store-operated Ca2+ entry, and they are activated upon binding to the stromal interaction molecule 1 (STIM1) and ORAI 1–3 proteins (Hogan et al., 2010). Notably, the activation of CRAC channels occurs after the ORAI proteins bind to STIM proteins at the ER-plasma membrane junction (ORAI binding site to STIM is located at the carboxyl terminus of the former; Feske et al., 2012; Joseph et al., 2014). Upon binding, STIM proteins sense Ca2+ depletion inside the ER, oligomerizing and redistributing toward these junctions where they communicate this Ca2+ decrease to CRAC channels (Hogan et al., 2003; Feske et al., 2012). The cytoplasmic domain of STIM1 interacts with the N and C termini in ORAI1, and CRAC channels are activated, leading to the store-operated Ca2+ entry (Feske et al., 2012). STIM2, another isoform of STIM proteins, is expressed in lymphocytes and can interact with ORAI1, although with slower kinetics than STIM1 (Feske et al., 2012). Activation of CRAC channels and the store-operated Ca2+ entry induction is key for cytokine production because CD4+ and CD8+ T cells from STIM1-deficient mice and ORAI1 cannot secrete cytokines, such as IL-2, IL-4, IL-17, TNF, and IFN-γ (Trebak and Kinet, 2019). In T cells, mitochondria work alongside CRAC channels and interact with them to maintain Ca2+ influx and regulate homeostasis (Joseph et al., 2014). After T cell receptor engagement and immunological synapsis formation, mitochondria moves closes to CRAC channels in response to the massive transportation of Ca2+, thus prolonging CRAC activity (Joseph et al., 2014). Figure 2 describes these events on T cells upon T cell receptor engagement by cognate peptide-MHC (pMHC) complexes on the antigen-presenting cells surface.
The modulation of the Ca2+ concentrations inside T cells is also mediated by plasma membrane Ca2+ ATPase pumps (PMCAs). During immunological synapsis, PMCAs are in close contact with CRAC channels, which modulates the activity of the ATPase pump. However, PMCAs efflux of Ca+2 from the T cell is reduced, to allow mitochondrial control of Ca+2 flux (Quintana and Hoth, 2012). PMCAs also promote the activation the sarco-endoplasmic reticulum Ca2+ ATPase (SERCA), which removes cytosolic Ca2+ into the ER and the mitochondria (Hogan et al., 2003; Korthals et al., 2017). In addition, ryanodine receptor channels also increase the efflux of Ca2+ from the ER to the cytoplasm (Fenninger and Jefferies, 2019). These channels are activated by the release of cyclic adenosine diphosphate (ADP) ribose (cADPR) induced upon the store-operated Ca2+ entry (Fenninger and Jefferies, 2019). PMCAs are activated after Ca2+-loaded calmodulin binds to their carboxyl terminus (Joseph et al., 2014; Fenninger and Jefferies, 2019). Although PMCAs have a high affinity for Ca2+, they also have low permeability for this cation, which is why they modulate Ca2+ concentration at basal levels (Joseph et al., 2014).
The P2X purinoreceptor ion-channel family (and specifically P2X1, P2X2, and P2X7) consists of another group of channels related to immunity and myeloid and lymphoid cell modulation (Junger, 2011). These channels require extracellular ATP for activation and are non-selective for cations, such as Ca2+ and Na+ (Junger, 2011). P2X7 receptors mainly control complex pathways that modulate Ca2+ and Na+ influx and K+ efflux and are primarily expressed by T lymphocytes and innate immune cells (Yip et al., 2009; Junger, 2011). Activation of P2X7 by ions such as K+, Na+, Ca2+, and Cl– results in significant stimulation of the NLRP3 inflammasome in several innate cells, such as in macrophages and monocytes (Yip et al., 2009; Junger, 2011). Furthermore, NLRP3 oligomerization and inflammasome activation will result in Caspase 1-induced cleavage of pro-IL-1β and pro-IL-18 into their mature forms, IL-1β and IL-18 (Franchi et al., 2009). These cytokines will activate additional signaling pathways to induce inflammation and immune cell function (Franchi et al., 2009). Remarkably, Pannexin 1 hemichannels (Panx1) has been reported to co-localize with P2X7 at the immunological synapsis, (Woehrle et al., 2010). Because Panx1 can release ATP into the extracellular space, it has been suggested that T cells can modulate P2X7 signaling (Woehrle et al., 2010). This modulation can work in an autocrine fashion to amplify gene expression, T cell effector functions, and T cell receptor signals upon pMHC engagement (Schenk et al., 2008; Woehrle et al., 2010; Junger, 2011; Figure 2). Activation of the inflammasome and Caspase-1 is also linked to programmed cell death (Martinon et al., 2002; Shi et al., 2017). Gasdermin D is a target of Caspase-1, -4, -5, and -11 and has been identified as a key component of the molecular mechanisms of pyroptosis (Lamkanfi et al., 2008; Shi et al., 2015). Pyroptosis is a cellular phenomenon leading to programmed cell death that increases the presence of inflammatory molecules in the extracellular space, such as IL-1β (Shi et al., 2017), which differs from non-inflammatory cell death triggered by apoptosis (Jorgensen and Miao, 2015). The inflammatory features of pyroptosis include the activation of PRRs and an increased transcription of pro-inflammatory cytokines, which classifies this process as an innate immunity-related mechanism (Jorgensen and Miao, 2015). Gasdermin D is usually found in an inactivated state and, upon cleavage by the aforementioned Caspases, can act as a pore-forming protein by disrupting the plasma membrane, cell swelling, and lysis, in a pyroptotic fashion (Ding et al., 2016; Liu et al., 2016). Remarkably, increased cytosolic Ca2+ leads to increased translocation of GSDMD into the membrane and enhanced LPS-induced pyroptosis, in a PLCγ1-dependent manner (Liu et al., 2020). Therefore, Ca2+ contributes to the modulation of programmed cell death.
Transient receptor potential channels were initially described in Drosophila melanogaster mutants and constitute a superfamily including 6 subfamilies in humans: TRP canonical (TRCP), TRP vanilloid (TRPV), TRP melastatin (TRPM), TRP ankyrin (TRPA), TRP mulcolipin, and TRP polycystic (Minke and Cook, 2002; Wenning et al., 2011; Feske et al., 2012; Samanta et al., 2018). The most common TRP receptors expressed by T cells are TRPC and TRPM (Parenti et al., 2016; Froghi et al., 2021). TRPC channels are thought to be responsible for Ca2+ influx into T cells and are usually associated with PLCγ signaling (Feske et al., 2012). Further studies are required to elucidate if these channels contribute to the store-operated Ca2+ entry and T cell activation (Minke and Cook, 2002; Feske et al., 2012). TRP cation channel subfamily M member 2 (TRPM2) channels have been shown to regulate Ca2+ in a non-selective fashion in several immune cells (Yamamoto et al., 2010). These channels are regulated by several intracellular factors, mostly related to ADP, such as cADP and cADPR (Yamamoto et al., 2010; Huang et al., 2018). Other molecules, such as hydrogen peroxide, may modulate the permeability and activity of TRPM2 (Yamamoto et al., 2010; Sumoza-Toledo and Penner, 2011; Wenning et al., 2011). Different reports have shown that these channels can enhance T cell receptor stimulation in T cells and inhibit reactive oxygen species production in phagocytic cells (Di et al., 2011; Chung et al., 2015; Syed Mortadza et al., 2015; Morad et al., 2021). Furthermore, this channel can promote the secretion of cytokines such as IL-2, IL-17, and IFN-γ (Melzer et al., 2012). TRPM4, the most studied TRP channels in immune cells, are highly permeable to Na+ and K+ and partially to Ca2+ (Vennekens and Nilius, 2007). The influx of Ca2+ will induce the activation of TRPM4, which will negatively modulate store-operated Ca2+ entry and promote Na+ influx, membrane depolarization, and reduction of Ca2+ influx due to stabilizing electrical charges (Vennekens and Nilius, 2007; Feske et al., 2012; Bose et al., 2015). The modulation of Ca2+ levels regulates the Th1 and Th2 differentiation of T cells (Weber et al., 2010). Lastly, TRPM8 has been associated to T cell activation, increasing CD25 and CD69 expression and TNF-α secretion (Acharya et al., 2021). Although TRPM8 might not be necessary for T cell activation, inhibition of this channel impairs T cell proliferation (Acharya et al., 2021).
Cav channels induce specific Ca2+ influx in excitable cells and are divided into three subfamilies, Cav1, Cav2, and Cav3. T cells express only the Cav1 first subfamily (Feske et al., 2012, 2015), which comprises Cav1.1, Cav1.2, Cav1.3, Cav1.4, and the regulatory subunits β3 and β4 (Feske et al., 2012, 2015; Badou et al., 2013). Treatment of Jurkat T cells with a Cav channel antagonists reduces IL-2 production (Fenninger and Jefferies, 2019), suggesting the involvement of this channel in the secretion of this cytokine. The upregulation of Cav 1.2 and Cav 1.3 channels and the differentiation of T cells toward a Th2 profile are also associated (Cabral et al., 2010). Cav1.2 activity is also regulated via STIM, as upon inhibition of Cav 1.2, the interaction between STIM and ORAI occurs (Badou et al., 2013). The influx of Ca2+ generated by Cav 1.4 channels modulates the homeostasis of naïve T cells and is important for the generation of T cell responses (Omilusik et al., 2011).
Magnesium is another secondary messenger important for modulating the function of T cells, which has been associated with promoting the correct function and development of these cells (Feske et al., 2012). Magnesium transporter protein 1 (MAGT1, an Mg2+ transporter) is a highly selective Mg2+ channel that is not permeable to other divalent cations such as Ca2+, Zn2+, and Ni2+ (Figure 2; Goytain and Quamme, 2005; Ravell et al., 2020) and is involved in T cell development and function (Goytain and Quamme, 2005). Notably, MAGT1 will induce PLCγ1 activation and then store-operated Ca2+ entry, upon Mg2+ influx and T cell receptor stimulation (Ravell et al., 2020). T cells lacking MAGT1 channels exhibit an impaired capacity to be activated upon T cell receptor engagement and show a decreased Ca2+ influxes (Li et al., 2011; Brandao et al., 2013).
TRP cation channel subfamily M member 7 is a non-selective Mg2+ channel expressed by T cells, permeable also to Ca2+ (Bates-Withers et al., 2011; Antunes et al., 2016; Vaeth and Feske, 2018). TRPM7 channels regulate Mg2+ concentration and cell homeostasis in the entire organism (Bates-Withers et al., 2011; Antunes et al., 2016; Vaeth and Feske, 2018) and are fundamental for T cell development and maturation (Jin et al., 2008; Feske et al., 2012). The absence of this channel impairs the development of T cells, resulting in CD4– and CD8– T cells (Schmitz et al., 2003; Feske et al., 2012). However, it seems that the lack of TRPM7 does not modify the intracellular concentration of Mg2+ ([Mg2+]i) in T cells, but rather the primary function of this channel might be related to Ca2+ influx (Feske et al., 2012). The translocation of Ca2+ or Mg2+ into target cell leads to the phosphorylation of molecules involved in the signaling of tyrosine kinases-based receptors, promoting cell differentiation, growth and activation (Zou et al., 2019).
The Kv1.3 and KCa3.1 channels modulate K+ homeostasis (Chiang et al., 2017). These channels must control the efflux of K+, which leads to hyperpolarization of the plasma membrane (Lam and Wulff, 2011; Chiang et al., 2017). These channels inhibit membrane depolarization upon the influx of other cations, such as Mg2+ and Ca2+ (Lam and Wulff, 2011). The topology of Kv1.3 and KCa3.1 is very similar, as they are homotetramers with each subunit exhibiting six transmembrane segments (Di Lucente et al., 2018; Ji et al., 2018). However, these channels differ in the mechanism of activation (Wulff et al., 2009). While Kv1.3 is activated upon membrane depolarization leading to K+ efflux, KCa3.1 activation requires the release of Ca2+ and calmodulin. This process leads to repolarization of the plasma membrane upon K+ efflux and continuous influx of Ca2+ (Wulff et al., 2009). Interestingly, these channels are relevant for establishing central and effector memory phenotypes in T cells (Chiang et al., 2017).
N-methyl-D-aspartate receptors (NMDARs) and α-amino-3-hydroxy-5-methyl-4-isoxazole propionic acid receptors (AMPARs) are ionotropic glutamate receptors that deserve mention, as they are considered receptors linked to ion channels and are relevant during glutamatergic transmission in the central nervous system (CNS; Traynelis et al., 2010). Glutamate is considered one of the primary excitatory neurotransmitters of the CNS in mammals, and it can interact with ionotropic and metabotropic receptors (Traynelis et al., 2010). NMDARs and AMPARs are, as many of the channels mentioned so far, non-selective cations channels that are mostly permeable to K+ and Na+, and to some extent to Ca2+ (Traynelis et al., 2010). These receptors will produce excitatory post-synaptic responses upon activation (Fourgeaud et al., 2010; Traynelis et al., 2010; Kahlfuß et al., 2014). These receptors can also be found in several isoforms, depending on the subunit composition (Fourgeaud et al., 2010; Traynelis et al., 2010; Kahlfuß et al., 2014). Recent reports have described that immune cells, such as DCs are sensitive to and can release glutamate (Fourgeaud et al., 2010; Kahlfuß et al., 2014). Further, AMPAR and NMDAR are expressed by some immune cells, suggesting that these receptors can modulate the development, activation, or effector functions of these immune cells (Fourgeaud et al., 2010; Kahlfuß et al., 2014). However, more exhaustive studies are required to better define the contribution of these channels and the associated signaling pathways to the function of T cells and other immune cells.
Additional ion channels can be found on the surface of T cells, such as Zn2+ channels -i.e., ZIP3, ZIP6, ZIP8, ZIP10, ZIP14-, but the contribution of these channels to the function of T cells has not been defined (Feske et al., 2012).
CFTR is one of the anionic channels expressed by T cells (Feske et al., 2012). CFTR promotes the efflux of Cl– from the intracellular to the extracellular and its absence on CD4+ T cells induces a Th2 polarization leading to an increase in the secretion of IL-4 (Feske et al., 2012; Polverino et al., 2019). Another Cl– channel in T cells is GABA receptors, and the activation of this receptor has been described to inhibit the function and proliferation of these cells (Feske et al., 2012). Additionally, the treatment with GABA has prevented the secretion of IL-2 and IFN-γ on CD8+ T cells (Feske et al., 2012). Finally, the LRRC8-A channel has been identified on T cells and shown to be important for T cell development, function and survival (Kumar et al., 2014; Serra et al., 2021).
B cells can act as professional antigen-presenting cells that can prime T cells and can also differentiate into plasma cells to produce antibodies, which bind to specific antigens of pathogens, promoting their clearance (Whitacre et al., 2012; Häusser-Kinzel and Weber, 2019). B cells express the B cell receptor on their surface (Whitacre et al., 2012) and several ion channels and transporters that modulate their activation and function, through the influx of Ca2+, Mg2+, and Zn2+, or the efflux of K+ and Cl– (Scharenberg et al., 2007; Feske et al., 2015; Table 1).
Ca2 + ions act as a second messenger in B cells and are required for proliferation and antibody secretion (Vig and Kinet, 2009; Feske et al., 2012; Trebak and Kinet, 2019). Ca2+ influx in B cells is controlled by CRAC channels, which are activated upon antigen recognition (Feske et al., 2012, 2015). Therefore, CRAC channels contribute to the establishment of immunity against infections, modulating B cell proliferation and antibody secretion by these cells. The lack of ORAI1 in B cells can lead to immunodeficiency and also to autoimmunity (Feske et al., 2012). However, the absence of ORAI1 does not affect B cell development (Mahtani and Treanor, 2019). Autoimmune disorders described in the absence of ORAI1 are associated with inflammation of the CNS (Gauthier et al., 2006; Feske et al., 2012). For instance, multiple sclerosis has been related to reduced production of IL-10 by B cells that have mutations associated with CRAC channels (Gauthier et al., 2006; Feske et al., 2012).
Mg2+ is a secondary messenger essential in B cells because the lack of this cation prevents the proliferation of these cells (Feske et al., 2012). TRPM7 is the Mg2+ channel described in B cells, responsible for the entry of both Mg2+ and Ca2+ (Feske et al., 2012, 2015). Even though the role of Mg2+ has not been thoroughly studied, it is known that the lack of TRP7M in B cells induces cell death after 24 h, suggesting that the role of Mg2+ in B cells might be essential for cell survival (Brandao et al., 2013). The TRP7M might be essential for cell survival since the influx of Mg2+ induces the phosphorylation of targets from the signaling pathway involved with the tyrosine kinase-based receptors, leading to cell differentiation, growth and function (Zou et al., 2019). Interestingly, the lack of the channel TRPM7 has been associated with an increase of MAGT1 in B cells (Zou et al., 2019), a phenomenon that has not been described yet for T cells.
As seen for T cells, the influx of Ca2+ in B cells is highly dependent on the transmembrane electrostatic potential (Feske et al., 2012). K+ channels control the depolarization of the B cell membrane through the efflux of K+, and the channels involved in this process are Kv1.3 and Kca3.1 (Feske et al., 2012, 2015). Kv1.3 channels are expressed in proliferating B cells producing autoantibodies and switching to memory B cells (Wulff et al., 2004; Land et al., 2017). Blockade of this channel decreases the release of autoantibodies and has been related to inhibiting the proliferation of class-switched memory B cells (Land et al., 2017). Additionally, it has been suggested that blocking Kv1.3 channels might help controlling multiple sclerosis, a disease in which the proliferation of class-switched memory cells is detrimental (Beeton and Chandy, 2005; Feske et al., 2012).
Importantly, although B cells express additional ion channels such as Zn2+ channels -i.e., ZIP3, ZIP6, ZIP8, ZIP10, ZIP14, their contribution to B cell function remains unknown (Feske et al., 2015).
CFTR is the only Cl– channel described on the surface of B cells (Feske et al., 2012). Absence of this channel leads to the inhibition of conductance of Cl–, decreasing B cell activation and proliferation (Polverino et al., 2019). No other anionic channels have been described to be expressed by these cells.
One of the most relevant processes that initiate an adaptive immune response is the interaction between antigen-presenting cells (particularly DCs) and T cells (González et al., 2007; Carreño et al., 2010, 2011). This interaction requires ions to be mobilized from several compartments and considers the participation of ion channels and transporters (Quintana et al., 2011). The contribution of ion channels and transporters (mainly focused on Ca2+), and the function of these channels during the immunological synapsis and other processes such as T cell activation, proliferation, and differentiation, will be discussed in this section.
An immunological synapsis must be established to induce the activation of an adequate adaptive immune response (González et al., 2007; Franchi et al., 2009; Carreño et al., 2010, 2011). Ion channels are needed for the proper maturation of DCs, and several issues can arise when these channels are not working properly. For instance, inhibition of Kv1.3 results in an impairment in the expression of maturation markers, MHC-II expression, and secretion of cytokines (RamaKrishnan and Sankaranarayanan, 2016). Impairment of all these characteristics will cause a reduced activation and proliferation of T cells (RamaKrishnan and Sankaranarayanan, 2016). TRPM4 is required for the migration of peripheral DCs into the lymph nodes by activating PLC (Barbet et al., 2008). Therefore, a mutation or deletion of TRPM4 in DCs impairs the assembly of the immunological synapsis in lymph nodes, because DCs fail to reach this organ (Barbet et al., 2008). Cav1.2 will activate Ryanodine receptors, leading to the expression of MHC-II on the plasma membrane of DCs (Vukcevic et al., 2008). A mutation in the gene encoding for this channel would cause MHC-II not to be expressed on the DC membrane, antigen presentation would not occur, preventing the establishment of the immunological synapsis (RamaKrishnan and Sankaranarayanan, 2016).
Upon establishment of the immunological synapsis, regions known as central supramolecular activation clusters (cSMACs) are formed in the immediate vicinity of the T cell receptor-pMHC complex and concentrate most CD28 and T cell receptor molecules (Grakoui et al., 1999). Surrounding the immunological synapsis, peripheral supramolecular activation clusters emerge, where integrins such as CD2 and different intercellular adhesion molecules are located (Grakoui et al., 1999). At the most peripheric region, distant supramolecular activation clusters are formed, consisting mostly of CD45 and CD43 (Grakoui et al., 1999). One of the main indirect effects of the entry of Ca2+ into the T cell is the reorganization of actin on the three SMACs, modulating the intensity and the duration of T cell receptor signaling (Billadeau et al.,2007). After activation of T lymphocytes, there is a release of Ca2+ from the ER into the cytoplasm that leads to the activation of CRAC channel (Smith-Garvin et al., 2009). This will induce changes in the membrane potential, inducing the activation of voltage-dependent ion channels, such as Kv1.3. This will allow the exit of K+ to the extracellular space and maintain the T cell membrane potential (Grissmer et al., 1993). The primary function of intracellular Ca2+ influx is the mobilization of the centrosome to the cSMAC (Kloc et al., 2014). The centrosome is a cellular organelle that works as the microtubule-organizing center and is spatially close to the Golgi apparatus and several vesicles (Kloc et al., 2014). The mechanism by which the centrosome is mobilized due to Ca2+ flux is not entirely clear. Actin interaction with myosin generates a contraction that promotes that the centrosome, along with the Golgi apparatus, move toward the cSMAC (Billadeau et al., 2007). Because the centrosome moves toward the cSMAC, the T cell can release the proper cytokines in a localized and focused way toward the antigen-presenting cells or target cell because microtubule-organizing center carries with it the Golgi and vesicles containing cytokines (Martín-Cófreces et al., 2008; Kloc et al., 2014).
The interaction between DCs and T cells is also modulated by the intracellular concentration of Mg2+, Zn2+, and Ca2+ and the activity of ion channels and transporters (Feske et al., 2012; Figure 2). These cations can act as second messengers modulating various T cell functions, such as cytotoxicity, differentiation, and cytokine production (Feske et al., 2012). After T cell receptor activation, MAGT1 elicits a specific influx of Mg2+, which activates PLCγ1 and consequently the influx of Ca2+ after T cell receptor activation (Feske et al., 2012). The increase in Ca2+ in the cytosol after T cell receptor engagement will trigger various signaling pathways leading to the T cell activation and the induction of the immune response in a T cell-dependent manner (Joseph et al., 2014). However, the mechanisms involved in activating the MAGT1 transporter are not clear yet (Feske et al., 2012). Figure 2 describes the events occurring upon T cell receptor engagement by the cognate pMHC complex, focusing on Ca2+ signaling.
After T cell receptor engagement, there is an influx of Ca2+ producing a significant increase of intracellular Ca2+ concentration (around 50–100 fold increase as compared to resting values; Feske et al., 2012). This phenomenon promotes the activation of transcription factors and signal transduction molecules. Among the different channels that modulate this influx are TRP channels, Cav channels, P2X channels, and CRAC channels (Feske et al., 2012). To maintain the electrical gradient necessary for the transportation of this ion, Na+, Cl–, and K+ channels work alongside these Ca2+ channels (Feske et al., 2012; Vaeth et al., 2020; Figure 2).
T cell receptor engagement leads to the depletion of Ca2+ stores within the ER and the phosphorylation of PLCγ (Yang and Jafri, 2020). Although two isoforms of PLCγ have been described, namely PLCγ1 and PLCγ2 (Joseph et al., 2014) T cells mainly express PLCγ1 (Joseph et al., 2014). The activation of a tyrosine kinase promotes the PLCγ1 phosphorylation, and therein, its activation (Vaeth et al., 2020; Yang and Jafri, 2020). PLCγ1 hydrolyzes phosphatidylinositol 4,5-bisphosphate (PIP2) into DAG and IP3, molecules that act as second messengers in these cells (Vaeth et al., 2020; Yang and Jafri, 2020). DAG activates the protein kinase C (PKC) and the mitogen-activated protein kinase/extracellular signal-regulated kinase (MAPK/ERK) pathways, while IP3 binds to IP3R on the ER membrane. The IP3R is an ion channel permeable to Ca2+, which causes the release of this cation from this organelle as discussed above, with the ER later being refilled by the SERCA pump to recover original Ca2+ levels (Joseph et al., 2014; Vaeth et al., 2020; Yang and Jafri, 2020; Figure 2).
Phospholipase Cγ is composed of three SH domains needed to achieve proper phosphorylation of the phospholipase (Joseph et al., 2014). The activation of PLCγ occurs at microclusters in T cells, which are small protein aggregates promoted by the phosphorylation of the ζ-chain of the ZAP-70 protein (Joseph et al., 2014). The events occurring before phosphorylation are related to the activation of the ZAP-70 protein due to the phosphorylation of the immune receptor tyrosine activating motif, which is mediated by the Src family kinases (Joseph et al., 2014). This family of kinases is also in charge of the phosphorylation of NMDAR subunits in hippocampal neurons (Zainullina et al., 2011). Since Src proteins are expressed in both cell types, they might also regulate the activity of NMDAR in T cells (Zainullina et al., 2011). Following antigen stimulation, these receptors regulate T lymphocyte functions and contribute to the Ca2+ influx mediated by the store-operated Ca2+ entry (Zainullina et al., 2011; Joseph et al., 2014; Fenninger and Jefferies, 2019). Significantly, the activation of PLCγ at microclusters depends on the linker for activation of T cells, the SH domain-containing leukocyte protein of 76 KDa, Vav1, guanine nucleotide exchange factor, c-Cbl, and Itk (Joseph et al., 2014). In summary, PLCγ needs to be phosphorylated to begin the mobilization of Ca2+ from the ER Ca2+ stores into the cytoplasm, increasing the intracellular Ca2+concentration ([Ca2+]i).
As described above, the influx of Ca2+ into T cells is dependent on the transmembrane electrostatic potential (Feske et al., 2012). If the transmembrane electrostatic potential is negative, Ca2+ may enter the cell (Feske et al., 2012; Yang and Jafri, 2020). However, the influx of Ca2+ promotes a transient depolarization of the membrane, which does not favor ion entry (Feske et al., 2015). K+ channels contribute significantly to this process because they compensate this depolarization by maintaining a negative voltage in the T cell membrane and even hyperpolarizing it through the efflux of K+, providing a driving force that allows Ca2+ entry through CRAC channels (Fanger et al., 2001; Cahalan and Chandy, 2009; Lam and Wulff, 2011; Feske et al., 2012, 2015; Teisseyre et al., 2019; Yang and Jafri, 2020). There is some controversy about the role that K+ channels play with regards to Ca+2 influx and a clamp in membrane potential caused by the opening of K+ channels may attenuate Ca+2 entry (Cahalan and Chandy, 2009). The movement of ions through membranes depends on various factors, such as the concentration gradient, the membrane potential, and the permeable ions involved (Bowman and Baglioni, 1984). All of these factors can be grouped in the electro-diffusion theory, which is the basis of the Goldman-Hodgkin-Katz equation (Bowman and Baglioni, 1984). There is not much information regarding K+ efflux and Ca+2 influx in T cells, but these two ions have been studied in non-excitable cells (HeLa cells) using a path-clamp measurement to determine membrane potential while stimulating the cell with a femtosecond laser (Ando et al., 2009). One of the main conclusions was that after laser irradiation on HeLa cells, an hyperpolarization of membrane potential correlates with a rise in intracellular Ca+2 (Ando et al., 2009). The crucial role that K+ channels play in the modulation of Ca2+ entry through CRAC channels allows its influx and further signaling, promoting the proliferation of T cells.
In T cells, K+ efflux is controlled by the channels Kv1.3, KCa3.1, K2p3.1, K2p5.1, and K2p9.1 (Feske et al., 2015). The two most commonly expressed channels in the membrane of T cells are Kv1.3 and KCa3.1 (Feske et al., 2012; Conforti, 2017). Several studies have evaluated the impact of blocking these channels on T cell function and the effects vary depending on the channel that was inhibited (Petho et al., 2016). For example, the simultaneous blockage of the channels Kv1.3, KCa3.1, and CRAC can prevent T cell proliferation, while only blocking Kv1.3 alters the secretion of cytokines by CD4+ T cells (Petho et al., 2016; Veytia-Bucheli et al., 2018). Interestingly, the activation of KCa3.1 channels in CD8+ T cells modulates their chemotactic activity toward tumor cells, suggesting that the increased activity of this channel might promote CD8+ T cell infiltration (Chimote et al., 2018). Additionally, pharmacological inhibition of both K+ channels in human CD4+ T cells induces a decrease in IL-2 production (Valle-Reyes et al., 2018). This decrease in IL-2 correlates with the inhibition of T cell proliferation primarily in the early stages of T cell activation (Verheugen et al., 1997).
Naïve T cells express mainly Kv1.3 channels, and upon T cell receptor engagement, there is an upregulation and recruitment of KCa3.1 channel into the IS (Feske et al., 2015). Membrane depolarization by the influx of Ca2+ is sensed by a set of arginine residues in the transmembrane segment four of these channels, causing conformational changes and their opening. Interestingly, the activation of this channel is also regulated by Lck, PKC ζ, and PKA (Nicolaou et al., 2010; Feske et al., 2012, 2015).
The binding of Ca2+ and calmodulin inside T cells activates the KCa 3.1 channel, as the C terminus of this channel is constitutively linked to calmodulin (Feske et al., 2012). The activation of this channel is also dependent on PKA and the class 2 phosphatidylinositol-3-kinase (PI3K-C2β), which is activated after T cell receptor stimulation (Feske et al., 2012). The activation of PI3K-C2β increases PI3P levels in the plasma membrane, allowing the histidine kinase nucleoside diphosphate kinase B to activate the KCa 3.1 channel by phosphorylating histidine 358 in its carboxyl terminus (Feske et al., 2012, 2015). Accordingly, dephosphorylation of PI3P and KCa 3.1 inhibits the activity of this channel, T cell proliferation, and Ca2+ influx induced by T cell receptor signaling (Feske et al., 2012, 2015). Such an inhibition occurs through the PI3P phosphatase myotubularin-related protein 6 and the histidine phosphatase phosphohistidine phosphatase-1. KCa 3.1 channels can also be inhibited through the ubiquitination and inhibition of PI3K-C2β due to the E3 ubiquitin ligase tripartite motif-containing protein 27 (Feske et al., 2012, 2015).
The overall immune response must be tightly regulated to recognize and clear different pathogens. CD4+ T cells are usually in charge of orchestrating this response by polarizing into different Th profiles, with key cytokines secreted by each of these profiles (Gálvez et al., 2021). For instance, while a Th1 polarization induces the secretion of IFN-γ and will be optimal for the clearance of intracellular pathogens, a Th2 response induces the secretion of IL-4 and will be optimal for the clearance of extracellular bacteria and a Th17 response induces the secretion of IL-17 and will be optimal for the clearance of extracellular pathogens, such as parasites (Gálvez et al., 2021). Interestingly, the absence of TRPA1 induces the polarization of T cells into a Th1 profile, increasing the expression of T-bet and IFN-γ (Bertin et al., 2017). The role of ion channels modulating T cell profiles toward a Th2 response has been associated with the upregulation of Cav channels mentioned above (Feske et al., 2012). The role of some ion channels during Th17 polarization has been partially described up to date. The entry of Ca2+ through the P2 × 7 receptor activates ERK1 or ERK2, which suppresses the transcription of forkhead box P3 and promotes the expression of retinoic acid receptor-related orphan receptor-γt, which induces the polarization of T cells toward a Th17 phenotype, altogether inhibiting the regulatory T cells (Treg) polarization (Feske et al., 2012).
Translocation of different transcription factors, such as the nuclear factor of activated T cells (NFAT) and the nuclear factor kappa-light-chain-enhancer of activated B cells (NF-κB) into the nucleus will induce the transcription of genes encoding for cytokines, such as IL-2 (Vaeth et al., 2020; Yang and Jafri, 2020). Ca2+ influx through CRAC channels regulates the serine/threonine phosphatase calcineurin function, which coordinates three main signaling pathways (Vaeth et al., 2020; Yang and Jafri, 2020). The first pathway is related to NFAT signaling, the second one is associated with NF-κB signaling and the third one is related to the c-Jun NH2-terminal kinase (JNK) pathway (Vaeth et al., 2020; Yang and Jafri, 2020). For the first pathway, calcineurin dephosphorylates NFAT, specifically the regulatory domain in the N terminal (Hogan et al., 2003). This exposes nuclear localization signals, promoting the translocation of this complex toward the nucleus, eventually inducing the transcription of cytokine genes, such as IL-2 (Joseph et al., 2014; Yang and Jafri, 2020). To activate this particular transcription pathway, T cells need a low and extended intracellular Ca2+ influx (Joseph et al., 2014; Yang and Jafri, 2020). Certain distal regulatory elements in NFAT target genes from T cells (mainly enhancers) can be found in cytokine genes, including IL-3, GM-CSF, IL-4, IL-10, and IFN-γ genes (Hogan et al., 2003). It is also important to indicate that NFAT can interact with other transcription factors, such as the activator protein 1 (AP-1) transcription factor, ICER, EGR, and GATA (Hogan et al., 2003). Calcineurin also regulates NF-κB signaling pathway, as an increase in intracellular Ca2+ promotes PKCα, which along with protein kinase C theta (PKCθ; previously activated by the co-stimulation of the T cell receptor/CD3 and CD28) co-activates the Iκ kinase (IKK) and phosphorylates IκB (Yang and Jafri, 2020). Phosphorylation induces IκB dissociation from NF-κB, leading to IκB polyubiquitination and degradation by the proteasome (Yang and Jafri, 2020). Therefore, NF-κB is initially retained in the cytoplasm because of the association with the inhibitory IκB protein, but later on, after the dissociation from IκB, free NF-κB translocates into the nucleus and promotes gene transcription (Yang and Jafri, 2020). This mechanism for the activation of gene transcription is called the canonical NF-κB activation pathway (Brasier, 2006). To activate this transcription factor, unlike for NFAT signaling pathway, T cells need a high and short increase in Ca2+ ions in the cytoplasm (Joseph et al., 2014; Yang and Jafri, 2020; Figure 2). Using Hodgkin’s cell lines, seventeen novel genes regulated by NF-κB were described, such as IL-13, MDC, I-309, EMR, CD44, and the transcription factors STAT5a, IRF-1, Spi-B, and LITAF (Brasier, 2006).
Another pathway related to Ca2+ influx is the JNK pathway. Activation of c-Jun modulated by PKCθ regulates MAPK cascade of SEK1/MKK4 and phosphorylates JNK (Yang and Jafri, 2020). This will trigger JNK signaling, along with calcineurin, which also activates SEK (Yang and Jafri, 2020). SEK phosphorylates two serine residues in the activation domain of c-Jun, promoting the activation of this protein (Yang and Jafri, 2020). c-Jun, along with c-Fos establishes the AP-1 transcription factor complex, which plays a role in cell growth and IL-2 induction (Avraham et al., 1998; Yang and Jafri, 2020). The activation of JNK can be detected minutes after pMHC- T cell receptor interaction, along with the binding of B7-1 and -2 molecules to the CD28 receptor on T cells. Both signals are needed to strongly activate JNK as they synergize (Avraham et al., 1998).
All this coordinated activation of NFAT, NF-κB, and AP-1 will result in the activation of pathways that will induce the secretion of several cytokines, including IL-2, IL-8, IL-1β, IL-4, IL-6, IFN-γ, and TNF-α (Fiebich et al., 2012). Among all of these cytokines, the secretion of IL-2 is essential because it modulates the differentiation of CD4+ T cells toward a Th2 or Th1 profile while inhibiting Th17 differentiation (Fiebich et al., 2012).
Diseases associated with ion channels and transporters mutations that change the molecular structure, topology, and functions of these proteins are called channelopathies (Kim, 2014; Schorge, 2018; Vaeth and Feske, 2018). These diseases are commonly caused by mutations in the alpha subunits or the accessory proteins of these channels (Kim, 2014; Schorge, 2018; Vaeth and Feske, 2018). Channelopathies have been linked to CNS diseases (i.e., epilepsy, ataxia, and migraine), heart diseases, lung diseases (i.e., cystic fibrosis), liver disease, and kidney diseases (Kim, 2014; Schorge, 2018; Vaeth and Feske, 2018). Channelopathies can also affect the immune system, causing immune alterations, such as autoimmune diseases or immunodeficiencies (Feske et al., 2015; Vaeth and Feske, 2018). For instance, mutations that abolish the functionality of CRAC channels, by loss of function of the ORAI1 gene or mutations in the STIM1 gene (components of this channel that we will further describe below), are responsible for a syndrome called CRAC channelopathy (Feske, 2010; Lacruz and Feske, 2015). This syndrome will lead to the establishment of combined immunodeficiency (CID), a disease that is directly linked to the absence of store-operated Ca2+ entry (Feske, 2010; Lacruz and Feske, 2015). CID patients, unlike severe combined immunodeficiency patients, exhibit normal counts of most immune cells, including T and B cells, and most myeloid populations, while displaying odd numbers of unconventional lymphocytic populations, such as Tregs, iNKT cells, and γδ T cells (Feske, 2010; Lacruz and Feske, 2015). Although low in number, these cell types are fundamental for establishing a proper immune response (La Cava, 2009; Nielsen et al., 2017; Gálvez et al., 2021). During their first years of life, CID infants require transplantation of hematopoietic stem cells to face the different severe diseases they develop due to their lack of these unconventional lymphocytes (Feske, 2010; Lacruz and Feske, 2015; Vaeth and Feske, 2018). These severe diseases will usually be caused by common and less pathogenic microorganisms like Candida albicans, Streptococcus pneumoniae, and cytomegalovirus (Feske, 2010; Lacruz and Feske, 2015; Vaeth and Feske, 2018). The lack of Tregs can also lead to the development of autoimmune hemolytic anemia, which originated from the presence of autoantibodies (Lian et al., 2018). Other diseases related to mutations in ion channels and transporters have been described, such as X-linked immunodeficiency with magnesium defects, Epstein-Barr virus infections, and neoplastic syndrome, which arise from the loss of function MAGT1 (Li et al., 2011; Ravell et al., 2014). Several other disorders have been described, such as agammaglobulinemia resulting from loss of function of LRRC8A (a Cl– channel) (Sawada et al., 2003) and transient neonatal zinc deficiency, which significantly impairs the activation of T cells and is originated by loss of function of ZIP4 (a Zn2+ channel) (Dufner-Beattie et al., 2005; Geiser et al., 2012). Additionally, channelopathies not only promote the infection, but it has also been described that viral infections can lead to the alteration of cation channels, such as Ca2+, K+, and Na+ (Charlton et al., 2020), creating dysregulation of the concentrations of Ca2+ along with other concentrations of cations. Furthermore, the immune response elicited against the infection can induce channelopathies, due to the binding of autoantibodies to IC (Vaeth and Feske, 2018). The proper function of these channels is fundamental for the immune system and the overall organism.
As described above, Ca2+ is essential for numerous functions of cells. Interestingly, viruses can take advantage of this by modulating the intracellular concentration of Ca2+ ([Ca2+]i) used in processes such as viral replication (Zhou et al., 2009). During viral infections, there are changes on [Ca2+]i that favor viral replication or the establishment of persistent infections on target cells, as discussed below (Zhou et al., 2009). In this section, we will describe the mechanisms used by RNA viruses, such as the human immunodeficiency virus (HIV), respiratory syncytial virus (hRSV), severe acute respiratory coronavirus 2 (SARS-CoV2), and DNA viruses, such as hepatitis B virus (HBV), and herpes simplex virus (HSV) to modulate the concentration of Ca2+ altering the cellular signaling.
Human immunodeficiency virus is a relevant virus transmitted through sexual intercourse, blood perfusion, needle sharing, and vertical transmission. This virus belongs to the Retroviridae family, and its genome is composed of two positive-sense single-stranded RNA molecules encoding for three polyproteins (Gag, Pol, and Env) and six accessory proteins (Tat, Rev, Nef, Vpr, Vif, and Vpu; Frankel and Young, 1998; German Advisory Committee Blood (Arbeitskreis Blut), 2016; Rossi et al., 2021). The main target of infection of HIV are CD4+ cells and CCR5- or CXCR4-positive cells, including DCs, macrophages, T helper cells, monocytes, microglia, and astrocytes (German Advisory Committee Blood (Arbeitskreis Blut), 2016; Rossi et al., 2021). Like other viruses, HIV modulates [Ca2+] and Ca2+-dependent channels during different stages of infection, including replication, assembly, budding, and also during immune response evasion (German Advisory Committee Blood (Arbeitskreis Blut), 2016; Chen et al., 2019). It has been described that HIV proteins have a role in Ca2+ modulation. Both HIV proteins gp120 and Tat can increase [Ca2+]i by modulating Ca2+ channels in several cell types, such as immune cells, astrocytes, neurons, and epithelial cells (Zocchi et al., 1998; Contreras et al., 2005; Zhou et al., 2009; Hu, 2016; Cai et al., 2020). In normal conditions, DCs need the Ca2+ mobilization for several function such as the engulfment of apoptotic bodies and antigen presentation (Poggi et al., 1998). Accordingly, the voltage-sensitive dihydropyridine receptor (DHPR) L-type calcium channel expressed in human monocyte-derived DCs (mo-DCs) plays an essential role in Ca2+ mobilization. This function was demonstrated by the use of nifedipine (NFP) which inhibit the DPHR function, affecting Ca2+ mobilization and engulfment of apoptotic bodies by mo-DCs (Figure 3; Poggi et al., 1998). The same phenomenon was observed when mo-DCs were stimulated with HIV Tat protein, which suggests that this protein can interact with DHPR, prevent the entry of Ca2+ into the cells, and affect essential functions, such as apoptotic body engulfment and IL-12 secretion (Poggi et al., 1998).
Figure 3. Viral pathogens modify biological processes on immune cells by increasing the Ca2+. (A) During normal conditions, various immune cells, such as dendritic cells, monocytes, macrophages, and neutrophils, regulate their concentrations of intracellular Ca2+. The activation of dihydropyridine receptor (DHPR) and Ca2+ channels promote the entry of Ca2+ into the cells. The interaction between the CRAC channel and STIM1 promotes the movement of Ca2+ into the cytosol. (B) During normal conditions, T cells regulate their concentrations of intracellular Ca2+ through the activation of the T cell receptor (TCR). (C) Different viruses can modulate different processes in various immune cells. The human immunodeficiency virus (HIV) can increase the Ca2+ concentration through the modulation of Ca2+ channels, and the activation of DHPR promotes the mobilization of Ca2+ that leads to the secretion of TNF-α. The human respiratory syncytial virus (hRSV) promotes the increase of Ca2+ as well. SARS-CoV-2 might be causing a hypoxic state and increase the infectivity of the virus through the increase Ca2+ concentration. The hepatitis B virus (HBV) and herpes simplex virus (HSV) can increase the concentration of intracellular Ca2+ through the interaction between the CRAC channel and STIM1. (D) RNA viruses can modulate different processes in T cells. HIV proteins activate the T cell receptor (TCR), and as a result, it impairs the domains of Ca2+, leading to spontaneous T cell proliferation. hRSV induces the influx of Ca2+ into T cells, which might decrease the secretion of IL-2. As for DNA viruses, such as HBV, the interaction between the CRAC channel and STIM1 might modulate the development of T cells.
Moreover, human NK cells express a functional phenylethylamine-sensitive (PAA) L-type calcium channel, which when blocked with verapamil (VPM; L-type calcium channel antagonist) the Ca2+ influx from the extracellular media, the tumor cell killing function was affected (Zocchi et al., 1998). The interaction between human NK cells and HIV Tat protein induces a decrease of Ca2+ influx, promoting the inhibition of Ca2+-dependent functions, such as NK cell cytotoxicity, as seen with VPM (Zocchi et al., 1998). Like other immune cells, human monocytes stimulated with Tat can mobilize Ca2+ from the extracellular media (Contreras et al., 2005). Additionally, human monocytes and other immune cells express a functional DHPR in the cellular membrane surface, which has an essential role in cytokine secretion (Contreras et al., 2005). HIV Tat protein uses DHPR to induce the Ca2+ mobilization, promoting the induction of TNF-α production by human monocytes during infections (Figure 3; Contreras et al., 2005).
On the other hand, because HIV can induce neurotoxicity, using a recombinant HIV gp120 protein in primary human cultures of astrocytes and neurons has been shown to increase [Ca2+]i, which occurs first in astrocytes and then in neurons, and also that NMDARs may mediate this effect in both cells types (Holden et al., 1999). Only in neurons there exists participation of the L-type calcium channel, as astrocytes do not express it. These results suggest that gp120 can modulate [Ca2+] influx through Ca2+ channels and NMDARs, promoting neurotoxicity (Holden et al., 1999).
Another important HIV protein is Nef, which induces T cell activation, which is necessary for viral replication in these cells and promotes hyperactivation in the absence of CD28 ligation (Manninen and Saksela, 2002; Fenard et al., 2005). In Jurkat cells, HIV Nef can induce an increase of Ca2+, mediated by the interaction with IP3R (Manninen and Saksela, 2002; Zhou et al., 2009). Furthermore, it has been described that Nef is found in lipid rafts, which are recruited into the IS space minutes after T cell receptor engagement (Figure 3; Fenard et al., 2005). Besides, HIV Nef can impair the formation of Ca2+ domains after T cell receptor engagement, suggesting that this viral protein can control the cellular distribution of Ca2+ levels, allowing hyperactivation, which implies a spontaneous T cells proliferation, including the expression of the T cells activation antigens and increased cytokines secretion, among other changes (Figure 3; Ott et al., 1997; Fenard et al., 2005).
One of the most prevalent viral agents that causes acute lower tract respiratory infections in infants under 2 years old, as wells as immunocompromised and elderly individuals, is hRSV (Tabor et al., 2021). Human RSV is an enveloped, negative-sense single-strand RNA virus that belongs to the Pneumoviridae family. This virus encodes for eleven proteins, including three in the virion surface (F, G, and SH) and four proteins found inside the viral structure (N, L, P, and M2.1; Collins et al., 2013; Cui et al., 2016). Decades ago, it was described that syncytia formation, characteristic of hRSV infection, in HEp-2 cells requires the presence of Ca2+ (Shahrabadi and Lee, 1988). Later, using a genetically-encoded Ca2+ indicator strategy in hRSV-infected MA104 cells, observing increases of [Ca2+]i and Ca2+ influx during cell fusions (Figure 3; Perry et al., 2015). Ca2+ concentrations increased first in the cytoplasm and then in the ER, and a rapid increase of Ca2+ influx before cell death was observed (Perry et al., 2015). Accordingly, the results suggest that hRSV may modulate Ca2+ levels. Additionally, hRSV infection requires the host Ca2+ pump SPCA1 located in the trans-Golgi network. A deficiency of SPCA1 has a relevant impact on hRSV spread and alters the susceptibility of the cells to infection, corroborating the crucial role of Ca2+ (Hoffmann et al., 2017). Additionally, hRSV infection of human epithelial cells, such as BEAS-2B, has been shown to increase upon expression of TRPV1, which is implicated in asthma predisposition (Omar et al., 2017; Harford et al., 2018). With regards to the ability of hRSV to modulate ions, it is known that the SH protein is a viroporin that forms pentameric cation-selective ion channel at the surface of the infected cells, allowing the entry of Na+ and K+ and promoting the activation of the NLRP3 inflammasome (Gan et al., 2012; Triantafilou et al., 2013).
As described above, the modulation of [Ca2+]i through the expression or the function of ion channel and pumps by hRSV can affect the immune response. It is known that hRSV can impair immunological synapsis by recruiting the N protein to the surface of the infected DCs (Gonzalez et al., 2008; Céspedes et al., 2014). Moreover, recent evidence shows that hRSV can infect human T cells (CD4+ and CD8+) and decrease IL-2 production, which may explain the absence of a long-lasting protective immunity (Figure 3; Raiden et al., 2017). Regarding the relevance of Ca2+ for hRSV infection, currently, there are no studies evaluating whether hRSV infection can modulate [Ca2+]i or Ca2+ signaling pathways in infected DCs or T cells.
The newly described coronavirus SARS-CoV-2 is the causative agent of an unprecedented pandemic in the last 100 years. This virus is an enveloped particle with a single-strand positive-sense RNA that encodes four structural proteins (S, E, M, and N) and sixteen non-structural proteins (nsp1-16; McClenaghan et al., 2020; Wang et al., 2020). Patients admitted to hospitals show low serum Ca2+, which is currently a severity indicator (Zhou et al., 2020). Moreover, it has been described that SARS-CoV-2 infection induces hypoxia in epithelial cells and innate immune cells (Serebrovska et al., 2020; Danta, 2021), and in a hypoxic state, there is an increase in [Ca2+]i, mediated by CRAC channels (Figure 3; Gusarova et al., 2011; Danta, 2021). Previously, it was described that SARS-CoV and MERS- CoV could alter Ca2+ concentration, which induced the viral entry into target cells, promoting viral fusion and, therein, increase their infectivity (Millet and Whittaker, 2018; Straus et al., 2020b). Furthermore, it was reported that the E protein of SARS-CoV is a viroporin that, like the hRSV SH protein, forms a pentameric ion channel that shows higher selectivity for Ca2+ than Na+ and K+ (Nieto-Torres et al., 2015). Indeed, the IC formed by the E protein can transport the Ca2+, which allows the subsequent activation of the NLRP3 inflammasome (Nieto-Torres et al., 2015). The SARS-CoV2-E (2-E) protein also forms an ion channel permeable to cations such as K+, Na+, and Ca2+ and causes cell death (Xia et al., 2020). Moreover, the 2-E protein by itself can induce the secretion of pro-inflammatory cytokines by macrophages (Xia et al., 2020).
The relevance of the Ca2+ channel for SARS-CoV-2 infection has been observed in Vero E6 and Calu-3 cells, and the use of calcium channels blockers, such as amlodipine, felodipine, and nifedipine, has been studied (Straus et al., 2020a). These Ca2+ channels blockers act by inhibiting the L-type Ca2+ channel, modulating viral entry into the cells, and viral replication, thus being a promising and potential therapeutic alternative for SARS-CoV-2 infection (Straus et al., 2020a). Although the main cellular targets for SARS-CoV-2 infection are epithelial cells, immune cells such as neutrophils, monocytes, and T cells are also susceptible to infection (Borsa and Mazet, 2020; Pontelli et al., 2020). How SARS-CoV-2 can modulate Ca2+ flux or interact with ion channels in infected immune cells remains to be elucidated.
Hepatitis B virus infection is a common cause of hepatic pathologies, such as hepatitis, cirrhosis, and hepatocellular cancer, which are public health problems worldwide (Hou et al., 2005; Liang, 2009). This virus is transmitted by contact with infected body fluids, such as blood (Hou et al., 2005). HBV is a small DNA enveloped virus with an icosahedral nucleocapsid that belongs to the Hepadnaviradae family (Venkatakrishnan and Zlotnick, 2016). This virus encodes for three envelop proteins (L, M, and S) and for the preCore, core, pol, and X (HBx; Seeger and Mason, 2015). Like other viruses, HBV modulates Ca2+ concentration to promote viral replication in hepatocytes. Remarkably, the HBx protein has been implicated in Ca2+ modulation and signaling (Bouchard et al., 2001; Casciano et al., 2017). In this context, it has been reported that HBx exerts effects on intracellular stored Ca2+, where it induces signal transduction pathways to allow HBV replication (Bouchard et al., 2001). Accordingly, HBx, not only by itself but in an HBV replication context, can interact with mitochondria and probably through the modulation of the mitochondrial permeability transition pore, control [Ca2+]i influx (McClain et al., 2007). Data obtained from rat primary hepatocytes showed that HBx could increase [Ca2+]i altering IP3-linked Ca2+ responses and modulate Ca2+ influx through the store-operated Ca2+ channels, which is necessary for HBV replication (Figure 3; Casciano et al., 2017). Furthermore, in HEK 293 cells, the interaction between HBx and ORAI1 is essential to maintain active the store-operated Ca2+ entry (Yao et al., 2018). According to this, the co-localization of HBx with ORAI1 suggests an upregulation of the STIM1-ORAI1 complex that modulates store-operated Ca2+channels positively, especially when Ca2+ concentrations are low, to induce an intracellular influx (Figure 3; Yao et al., 2018).
Hepatitis B virus can infect and replicate in PBMCs, including T cells, affecting the immune response against this virus (Yan et al., 2016). As described early, for the development and function of T cells and DCs, the role of store-operated Ca2+ entry is crucial for establishing the immunological synapsis (Figure 3; Oh-Hora, 2009; Félix et al., 2013). However, there are no studies that evaluate the role of HBx in immune cells.
One of the most ubiquitous human infections in the mucocutaneous tissue is caused by herpes simplex viruses type 1 and type 2 (HSV-1 and HSV-2) (He et al., 2020). HSVs belong to the Herpesviradae family and have a linear double-stranded DNA genome packaged in an icosahedral capsid that is enveloped. The envelope has numerous proteins, and the virus encodes twelve different glycoproteins (gB, gC, gD, gE, gG, gH, gI, gJ, gK, gL, gM, and gN; Karasneh and Shukla, 2011; Retamal-Díaz et al., 2016; Madavaraju et al., 2021). HSVs can infect several cell types, including epithelial cells, fibroblasts, neurons, and DCs (Koelle et al., 1993; Karasneh and Shukla, 2011; Retamal-Díaz et al., 2016; Marcocci et al., 2020).
Herpes simplex viruses infection of epithelial cell lines induces a transient increase in [Ca2+]i, mediated by the ER and Ca2+ influx from extracellular space (Figure 3; Cheshenko et al., 2003). Additionally, IP3-sensitive Ca2+ stores, probably available through the opening of Ca2+ channels in the ER membrane, help HSV enter the cell. On the other hand, the chelation of Ca2+ and pharmacological inhibition of IP3R impairs viral infection (Cheshenko et al., 2003). ND7/23 cells (sensory-like neurons) express the Cav3.2 T-type Ca2+ channel that regulates the excitability of neurons. Significantly, the expression of this channel is reduced by HSV-1 infection, which depends on viral replication and protein synthesis (Zhang et al., 2019). HSV-2 infection in HeLa cells promotes an extracellular Ca2+ influx, which can be blocked by the use of Ca2+ channels blockers and, therein, suppresses viral infection. T-type, but not L-type Ca2+ channel, impact HSV-2 infection, as proven by the use of Ca2+ channels blockers (Ding et al., 2021). Regarding the effects of HSV-1 on immune cells, it has been reported that these cells express the herpesvirus entry mediator, which is a receptor for the HSV-1 gD, allowing viral entry into DCs and activated T cells (La et al., 2002). Besides, it is known that HSV infection of DCs can modulate the maturation and their capacity of activating T cells, and also cause DC death (Jones et al., 2003; Bosnjak et al., 2005; Retamal-Díaz et al., 2016), but there is no data to date regarding Ca2+ modulation and the role of Ca2+ channels involved in this process. On the other hand, T cell proliferation can be modulated by the HSV-1 gD protein (Bosnjak et al., 2005). Additionally, HSV infection of T cells, in which gB, gD, gH, and gL are necessary for viral entry, or contact with HSV-infected cells, such as fibroblasts, can impair T cell receptor-mediated activation by altering Ca2+ mobilization that is required for the T cell receptor signaling (Koelle et al., 1993; Sloan et al., 2006; Cao et al., 2008). The effects of HSV infection over Ca2+ channels in immune cells remains poorly understood and requires more studies to explore the potential development of new therapeutic strategies that target these components.
In all cell types, ion balance is crucial to maintain normal physiology and functions. In this context, ion channels play critical roles in controlling ion homeostasis. One of the most relevant ions is Ca2+, which acts as a second messenger, and is crucial for eliciting a proper immune response against pathogens. Immune cells, such as T cells, express a wide variety of ions channels on their surface, including four types of Ca2+ channels CRAC, TRP, Cav, and P2X (Feske, 2013; Feske et al., 2015). These channels are implicated in the proliferation, activation, and cytotoxicity of T cells, all crucial mechanisms for the adaptive immune response. It has been described that loss-of-function mutations in Ca2+ channel genes affect their function on immune cells, provoking combined immunodeficiency and increasing the susceptibility to infections (Vaeth and Feske, 2018). Importantly, viruses also need Ca2+ mobilization for entry, replication, and budding (Zhou et al., 2009).
Moreover, viruses such as HIV, hRSV, SARS-CoV2, HBV, and HSV, among others, have proteins that can interfere with the normal function of Ca2+ channels to increase [Ca2+]i and enhance their infectivity in target cells (Zhou et al., 2009). Viral modulation of Ca2+ channels expressed in immune cells allows viruses to impair the immune responses as an evasion mechanism (Fenard et al., 2005; Sloan et al., 2006). Despite this knowledge, further insights into the associated mechanism, the impact of using Ca2+ channels blockers, and their effects on viral infections are still required.
All authors listed have made a substantial, direct and intellectual contribution to the work, and approved it for publication.
This work was supported by the Millennium Institute of Immunology and Immunotherapy (P09/016-F, ICN09_016, AK); CORFO grant #13CTI-21526/P4 and P5; ANID/FONDECYT grants #3180570 (KB); #1190830 (AK), #1191300 (CR), #1190864 (PG); ANID scholarship #21190183 (NG) and #21210662 (CA); and Biomedical Research Consortium CTU06 (AK). COPEC-UC2019.R.1169. COPEC-UC2020.E.1. Regional Government of Antofagasta through the Innovation Fund for Competitiveness FIC-R 2017 (BIP Code: 30488811-0). AK is a Helen C. Levitt Visiting Professor at the Department of Microbiology and Immunology of the University of Iowa.
The authors declare that the research was conducted in the absence of any commercial or financial relationships that could be construed as a potential conflict of interest.
All claims expressed in this article are solely those of the authors and do not necessarily represent those of their affiliated organizations, or those of the publisher, the editors and the reviewers. Any product that may be evaluated in this article, or claim that may be made by its manufacturer, is not guaranteed or endorsed by the publisher.
Ca2+, Calcium; Mg2+, Magnesium; Zn2+, Zinc; K+, Potassium; Na+, Sodium; Cl–, Chloride; HPO42–, Phosphate; HCO3–, Bicarbonate; CRAC, Ca2+ release-activated Ca2+ channels; TRP, Transient receptor potential; CFTR, Cystic fibrosis transmembrane conductance regulator; GABA, Gamma-aminobutyric acid; PLC β, phospholipase C β; PIP2, Phosphatidylinositol 4,5-bisphosphate; IP3, inositol (1,4,5) trisphosphate; DAG, Diacylglycerol; IP3R, IP3 receptor; ER, Endoplasmic reticulum; MAGT1, Magnesium transporter protein 1; DCs, Dendritic cells; STIM1, Stromal interaction molecule 1; pMHC, Peptide-MHC; PMCAs, Plasma membrane Ca2+ ATPase pumps; SERCA, Sarco-endoplasmic reticulum Ca2+ ATPase; ADP, Adenosine diphosphate; cADPR, cyclic ADP ribose; Panx1, Pannexin 1 hemichannels; TRPM2, TRP cation channel subfamily M member 2; NMDARs, N-methyl -D-aspartate receptors; AMPARs, α-amino -3-hydroxy-5-methyl-4-isoxazole propionic acid receptors; cSMACs, Central supramolecular activation clusters; PKC, Protein kinase C; PI3K-C2 β, Phosphatidylinositol-3-kinase; NFAT, Nuclear factor of activated T cells; NF- κ B, Nuclear factor kappa-light-chain-enhancer of activated B cells; JNK, c-Jun NH2-terminal kinase; AP-1, Activator protein 1; PKC θ, Protein kinase C theta; IKK, I κ kinase; CNS, Central nervous system; CID, Combined immunodeficiency; HIV, Human immunodeficiency virus; hRSV, Human respiratory syncytial virus; SARS-CoV2, Severe acute respiratory coronavirus 2; HBV, Hepatitis B virus; HSV, Herpes simplex virus; DHPR, Voltage-sensitive dihydropyridine receptor; VPM, Verapamil.
Acharya, T. K., Tiwari, A., Majhi, R. K., and Goswami, C. (2021). Trpm8 channel augments T-cell activation and proliferation/. Cell Biol. Int. 45, 198–210. doi: 10.1002/cbin.11483
Ando, J., Smith, N. I., Fujita, K., and Kawata, S. (2009). Photogeneration of membrane potential hyperpolarization and depolarization in non-excitable cells. Eur. Biophys. J. 38, 255–262. doi: 10.1007/s00249-008-0397-6
Antunes, T. T., Callera, G. E., He, Y., Yogi, A., Ryazanov, A. G., Ryazanova, L. V., et al. (2016). Transient receptor potential melastatin 7 cation channel kinase: new player in angiotensin II-induced hypertension. Hypertens 67, 763–773. doi: 10.1161/HYPERTENSIONAHA.115.07021
Avraham, A., Jung, S., Samuels, Y., Seger, R., and Ben-Neriah, Y. (1998). Co-stimulation-dependent activation of a JNK-kinase in T lymphocytes. Eur. J. Immunol. 28, 2320–2330. doi: 10.1002/(SICI)1521-4141(199808)28:08<2320::AID-IMMU2320>3.0.CO;2-K
Badou, A., Jha, M. K., Matza, D., and Flavell, R. A. (2013). Emerging roles of L-type voltage-gated and other calcium channels in T lymphocytes. Front. Immunol. 4:243. doi: 10.3389/fimmu.2013.00243
Barbet, G., Demion, M., Moura, I. C., Serafini, N., Léger, T., Vrtovsnik, F., et al. (2008). The calcium-activated nonselective cation channel TRPM4 is essential for the migration but not the maturation of dendritic cells. Nat. Immunol. 9, 1148–1156. doi: 10.1038/ni.1648
Bates-Withers, C., Sah, R., and Clapham, D. E. (2011). TRPM7, the Mg(2+) inhibited channel and kinase. Adv. Exp. Med. Biol. 704, 173–183.
Beeton, C., and Chandy, K. G. (2005). Potassium channels, memory T cells, and multiple sclerosis. Neuroscientist 11, 550–562. doi: 10.1177/1073858405278016
Bertin, S., Aoki-Nonaka, Y., Lee, J., de Jong, P. R., Kim, P., Han, T., et al. (2017). The TRPA1 ion channel is expressed in CD4+ T cells and restrains T-cell-mediated colitis through inhibition of TRPV1. Gut 66, 1584–1596. doi: 10.1136/gutjnl-2015-310710
Billadeau, D. D., Nolz, J. C., and Gomez, T. S. (2007). Regulation of T-cell activation by the cytoskeleton. Nat. Rev. Immunol. 7, 131–143. doi: 10.1038/nri2021
Borsa, M., and Mazet, J. M. (2020). Attacking the defence: SARS-CoV-2 can infect immune cells. Nat. Rev. Immunol. 20:592. doi: 10.1038/s41577-020-00439-1
Bose, T., Cieślar-Pobuda, A., and Wiechec, E. (2015). Role of ion channels in regulating Ca2+ homeostasis during the interplay between immune and cancer cells. Cell Death Dis. 6:e1648. doi: 10.1038/cddis.2015.23
Bosnjak, L., Miranda-Saksena, M., Koelle, D. M., Boadle, R. A., Jones, C. A., and Cunningham, A. L. (2005). Herpes simplex virus infection of human dendritic cells induces apoptosis and allows cross-presentation via uninfected dendritic cells. J. Immunol. 174, 2220–2227. doi: 10.4049/jimmunol.174.4.2220
Bouchard, M. J., Wang, L. H., and Schneider, R. J. (2001). Calcium signaling by HBx protein in hepatitis B virus DNA replication. Science 294, 2376–2378. doi: 10.1126/science.294.5550.2376
Bowman, C. L., and Baglioni, A. (1984). Application of the Goldman-Hodgkin-Katz current equation to membrane current-voltage data. J. Theor. Biol. 108, 1–29. doi: 10.1016/S0022-5193(84)80165-4
Brandao, K., Deason-Towne, F., Perraud, A.-L., and Schmitz, C. (2013). The role of Mg2+ in immune cells. Immunol. Res. 55, 261–269. doi: 10.1007/s12026-012-8371-x
Brasier, A. R. (2006). The NF-κκ B regulatory network κ B. Cardiovasc. Toxicol. 6, 111–130. doi: 10.1385/CT:6:2:111
Cabral, M. D., Paulet, P. E., Robert, V., Gomes, B., Renoud, M. L., Savignac, M., et al. (2010). Knocking down Cav1 calcium channels implicated in Th2 cell activation prevents experimental asthma. Am. J. Respir. Crit. Care Med. 181, 1310–1317. doi: 10.1164/rccm.200907-1166OC
Cahalan, M. D., and Chandy, K. G. (1997). Ion channels in the immune system as targets for immunosuppression. Curr. Opin. Biotechnol. 8, 749–756. doi: 10.1016/S0958-1669(97)80130-9
Cahalan, M. D., and Chandy, K. G. (2009). The functional network of ion channels in T lymphocytes. Immunol. Rev. 231, 59–87. doi: 10.1111/j.1600-065X.2009.00816.x
Cai, S., Tuohy, P., Ma, C., Kitamura, N., Gomez, K., Zhou, Y., et al. (2020). A modulator of the low-voltage-activated T-type calcium channel that reverses HIV glycoprotein 120-, paclitaxel-, and spinal nerve ligation-induced peripheral neuropathies. Pain 161, 2551–2570. doi: 10.1097/j.pain.0000000000001955
Cao, Y.-J., Li, Y.-P., Zhang, Y.-C., and Zhang, C.-Z. (2008). T cell receptor signaling pathways: new targets for herpes simplex virus. Virol. Sin. 23, 429–437. doi: 10.1007/s12250-008-3000-5
Carreño, L. J., González, P. A., Bueno, S. M., Riedel, C. A., and Kalergis, A. M. (2011). Modulation of the dendritic cell-T-cell synapse to promote pathogen immunity and prevent autoimmunity. Immunotherapy 3, 6–11. doi: 10.2217/imt.11.38
Carreño, L. J., Riquelme, E. M., González, P. A., Espagnolle, N., Riedel, C. A., Valitutti, S., et al. (2010). T-cell antagonism by short half-life pMHC ligands can be mediated by an efficient trapping of T-cell polarization toward the APC. Proc. Natl. Acad. Sci. U. S. A. 107, 210–215. doi: 10.1073/pnas.0911258107
Casciano, J. C., Duchemin, N. J., Lamontagne, R. J., Steel, L. F., and Bouchard, M. J. (2017). Hepatitis B virus modulates store-operated calcium entry to enhance viral replication in primary hepatocytes. PLoS One 12:e0168328. doi: 10.1371/journal.pone.0168328
Céspedes, P. F., Bueno, S. M., Ramírez, B. A., Gomez, R. S., Riquelme, S. A., Palavecino, C. E., et al. (2014). Surface expression of the hRSV nucleoprotein impairs immunological synapse formation with T cells. Proc. Natl. Acad. Sci. U. S. A. 111, E3214–E3223. doi: 10.1073/pnas.1400760111
Chaigne-Delalande, B., and Lenardo, M. J. (2014). Divalent cation signaling in immune cells. Trends Immunol. 35, 332–344. doi: 10.1016/j.it.2014.05.001
Chakraborty, K., Leung, K. H., and Krishnan, Y. (2017). High lumenal chloride in the lysosome is critical for lysosome function. Elife 6:e28862. doi: 10.7554/eLife.28862.026
Charlton, F. W., Pearson, H. M., Hover, S., Lippiat, J. D., Fontana, J., Barr, J. N., et al. (2020). Ion channels as therapeutic targets for viral infections: further discoveries and future perspectives. Viruses 12:844. doi: 10.3390/v12080844
Chen, X., Cao, R., and Zhong, W. (2019). Host calcium channels and pumps in viral infections. Cells 9:94. doi: 10.3390/cells9010094
Cheshenko, N., Del Rosario, B., Woda, C., Marcellino, D., Satlin, L. M., and Herold, B. C. (2003). Herpes simplex virus triggers activation of calcium-signaling pathways. J. Cell Biol. 163, 283–293. doi: 10.1083/jcb.200301084
Chiang, E. Y., Li, T., Jeet, S., Peng, I., Zhang, J., Lee, W. P., et al. (2017). Potassium channels Kv1.3 and KCa3.1 cooperatively and compensatorily regulate antigen-specific memory T cell functions. Nat. Commun. 8:14644. doi: 10.1038/ncomms14644
Chimote, A. A., Balajthy, A., Arnold, M. J., Newton, H. S., Hajdu, P., Qualtieri, J., et al. (2018). A defect in KCa3.1 channel activity limits the ability of CD8+ T cells from cancer patients to infiltrate an adenosine-rich microenvironment. Sci. Signal. 11:aaq1616. doi: 10.1126/scisignal.aaq1616
Chung, M.-K., Asgar, J., Lee, J., Shim, M. S., Dumler, C., and Ro, J. Y. (2015). The role of TRPM2 in hydrogen peroxide-induced expression of inflammatory cytokine and chemokine in rat trigeminal ganglia. Neuroscience 297, 160–169. doi: 10.1016/j.neuroscience.2015.03.067
Collins, P. L., Fearns, R., and Graham, B. S. (2013). Respiratory syncytial virus: virology, reverse genetics, and pathogenesis of disease. Curr. Top. Microbiol. Immunol. 372, 3–38. doi: 10.1007/978-3-642-38919-1_1
Conforti, L. (2017). Potassium channels of T lymphocytes take center stage in the fight against cancer. J. Immunother. Cancer 5, 1–3. doi: 10.1186/s40425-016-0202-5
Contreras, X., Bennasser, Y., Chazal, N., Moreau, M., Leclerc, C., Tkaczuk, J., et al. (2005). Human immunodeficiency virus type 1 Tat protein induces an intracellular calcium increase in human monocytes that requires DHP receptors: involvement in TNF-alpha production. Virology 332, 316–328. doi: 10.1016/j.virol.2004.11.032
Cui, R., Wang, Y., Wang, L., Li, G., Lan, K., Altmeyer, R., et al. (2016). Cyclopiazonic acid, an inhibitor of calcium-dependent ATPases with antiviral activity against human respiratory syncytial virus. Antivir. Res. 132, 38–45. doi: 10.1016/j.antiviral.2016.05.010
Danta, C. C. (2021). SARS-CoV-2, hypoxia, and calcium signaling: the consequences and therapeutic options. ACS Pharmacol. Transl. Sci. 4, 400–402. doi: 10.1021/acsptsci.0c00219
Di Lucente, J., Nguyen, H. M., Wulff, H., Jin, L.-W., and Maezawa, I. (2018). The voltage-gated potassium channel Kv1.3 is required for microglial pro-inflammatory activation in vivo. Glia 66, 1881–1895. doi: 10.1002/glia.23457
Di Resta, C., and Becchetti, A. (2010). Introduction to ion channels. Adv. Exp. Med. Biol. 674, 9–21. doi: 10.1007/978-1-4419-6066-5_2
Di, A., Gao, X.-P., Qian, F., Kawamura, T., Han, J., Hecquet, C., et al. (2011). The redox-sensitive cation channel TRPM2 modulates phagocyte ROS production and inflammation. Nat. Immunol. 13, 29–34. doi: 10.1038/ni.2171
Ding, J., Wang, K., Liu, W., She, Y., Sun, Q., Shi, J., et al. (2016). Pore-forming activity and structural autoinhibition of the gasdermin family. Nature 535, 111–116. doi: 10.1038/nature18590
Ding, L., Jiang, P., Xu, X., Lu, W., Yang, C., Li, L., et al. (2021). T-type calcium channels blockers inhibit HSV-2 infection at the late stage of genome replication. Eur. J. Pharmacol. 892:173782. doi: 10.1016/j.ejphar.2020.173782
Dufner-Beattie, J., Huang, Z. L., Geiser, J., Xu, W., and Andrews, G. K. (2005). Generation and characterization of mice lacking the zinc uptake transporter ZIP3. Mol. Cell. Biol. 25, 5607–5615. doi: 10.1128/MCB.25.13.5607-5615.2005
Fanger, C. M., Rauer, H., Neben, A. L., Miller, M. J., Rauer, H., Wulff, H., et al. (2001). Calcium-activated potassium channels sustain calcium signaling in T lymphocytes. Selective blockers and manipulated channel expression levels. J. Biol. Chem. 276, 12249–12256. doi: 10.1074/jbc.M011342200
Félix, R., Crottès, D., Delalande, A., Fauconnier, J., Lebranchu, Y., Le Guennec, J.-Y., et al. (2013). The Orai-1 and STIM-1 complex controls human dendritic cell maturation. PLoS One 8:e61595. doi: 10.1371/journal.pone.0061595
Fenard, D., Yonemoto, W., de Noronha, C., Cavrois, M., Williams, S. A., and Greene, W. C. (2005). Nef is physically recruited into the immunological synapse and potentiates T cell activation early after TCR engagement. J. Immunol. 175, 6050–6057. doi: 10.4049/jimmunol.175.9.6050
Fenninger, F., and Jefferies, W. A. (2019). What’s bred in the bone: calcium channels in lymphocytes. J. Immunol. 202, 1021–1030. doi: 10.4049/jimmunol.1800837
Feske, S. (2013). Ca2+ influx in T cells: how many Ca2+ channels? Front. Immunol. 4:99. doi: 10.3389/fimmu.2013.00099
Feske, S., Skolnik, E. Y., and Prakriya, M. (2012). Ion channels and transporters in lymphocyte function and immunity. Nat. Rev. Immunol. 12, 532–547. doi: 10.1038/nri3233
Feske, S., Wulff, H., and Skolnik, E. Y. (2015). Ion channels in innate and adaptive immunity. Annu. Rev. Immunol. 33, 291–353. doi: 10.1146/annurev-immunol-032414-112212
Fiebich, B. L., Collado, J. A., Stratz, C., Valina, C., Hochholzer, W., Muñoz, E., et al. (2012). Pseudoephedrine inhibits T-cell activation by targeting NF-κB, NFAT and AP-1 signaling pathways. Immunopharmacol. Immunotoxicol. 34, 98–106. doi: 10.3109/08923973.2011.582118
Fourgeaud, L., Davenport, C. M., Tyler, C. M., Cheng, T. T., Spencer, M. B., and Boulanger, L. M. (2010). MHC class I modulates NMDA receptor function and AMPA receptor trafficking. Proc. Natl. Acad. Sci. U. S. A. 107, 22278–22283. doi: 10.1073/pnas.0914064107
Franchi, L., Eigenbrod, T., Muñoz-Planillo, R., and Nuñez, G. (2009). The inflammasome: a caspase-1-activation platform that regulates immune responses and disease pathogenesis. Nat. Immunol. 10, 241–273. doi: 10.1038/ni.1703
Frankel, A. D., and Young, J. A. T. (1998). HIV-1: fifteen proteins and an RNA. Annu. Rev. Biochem. 67, 1–25. doi: 10.1146/annurev.biochem.67.1.1
Froghi, S., Grant, C. R., Tandon, R., Quaglia, A., Davidson, B., and Fuller, B. (2021). New insights on the role of TRP channels in calcium signalling and immunomodulation: review of pathways and implications for clinical practice. Clin. Rev. Allergy Immunol. 60, 271–292. doi: 10.1007/s12016-020-08824-3
Gálvez, N. M. S., Bohmwald, K., Pacheco, G. A., Andrade, C. A., Carreño, L. J., and Kalergis, A. M. (2021). Type I natural killer T cells as key regulators of the immune response to infectious diseases. Clin. Microbiol. Rev. 34:e232-20. doi: 10.1128/CMR.00232-20
Gan, S. W., Tan, E., Lin, X., Yu, D., Wang, J., Tan, G. M. Y., et al. (2012). The small hydrophobic protein of the human respiratory syncytial virus forms pentameric ion channels. J. Biol. Chem. 287, 24671–24689. doi: 10.1074/jbc.M111.332791
Gauthier, S. A., Glanz, B. I., Mandel, M., and Weiner, H. L. (2006). A model for the comprehensive investigation of a chronic autoimmune disease: the multiple sclerosis CLIMB study. Autoimmun. Rev. 5, 532–536. doi: 10.1016/j.autrev.2006.02.012
Geiser, J., Venken, K. J. T., De Lisle, R. C., and Andrews, G. K. (2012). A mouse model of acrodermatitis enteropathica: loss of intestine zinc transporter ZIP4 (Slc39a4) disrupts the stem cell niche and intestine integrity. PLoS Genet. 8:e1002766. doi: 10.1371/journal.pgen.1002766
German Advisory Committee Blood (Arbeitskreis Blut) (2016). Human immunodeficiency virus (HIV). Transfus. Med. Hemother. 43, 203–222. doi: 10.1159/000445852
González, P. A., Carreño, L. J., Figueroa, C. A., and Kalergis, A. M. (2007). Modulation of immunological synapse by membrane-bound and soluble ligands. Cytokine Growth Factor Rev. 18, 19–31. doi: 10.1016/j.cytogfr.2007.01.003
Gonzalez, P. A., Prado, C. E., Leiva, E. D., Carreno, L. J., Bueno, S. M., Riedel, C. A., et al. (2008). Respiratory syncytial virus impairs T cell activation by preventing synapse assembly with dendritic cells. Proc. Natl. Acad. Sci. U S A. 105, 14999–15004. doi: 10.1073/pnas.0802555105
Goytain, A., and Quamme, G. A. (2005). Identification and characterization of a novel mammalian Mg2+ transporter with channel-like properties. BMC Genom. 6:48. doi: 10.1186/1471-2164-6-48
Grakoui, A., Bromley, S. K., Sumen, C., Davis, M. M., Shaw, A. S., Allen, P. M., et al. (1999). The immunological synapse: a molecular machine controlling T cell activation. Science 285, 221–227. doi: 10.1126/science.285.5425.221
Grissmer, S., Nguyen, A. N., and Cahalan, M. D. (1993). Calcium-activated potassium channels in resting and activated human T lymphocytes: expression levels, calcium dependence, ion selectivity, and pharmacology. J. Gen. Physiol. 102, 601–630. doi: 10.1085/jgp.102.4.601
Gusarova, G. A., Trejo, H. E., Dada, L. A., Briva, A., Welch, L. C., Hamanaka, R. B., et al. (2011). Hypoxia leads to Na,K-ATPase downregulation via Ca(2+) release-activated Ca(2+) channels and AMPK activation. Mol. Cell. Biol. 31, 3546–3556. doi: 10.1128/MCB.05114-11
Harford, T. J., Rezaee, F., Scheraga, R. G., Olman, M. A., and Piedimonte, G. (2018). Asthma predisposition and respiratory syncytial virus infection modulate transient receptor potential vanilloid 1 function in children’s airways. J. Allergy Clin. Immunol. 141, 414–416.e4. doi: 10.1016/j.jaci.2017.07.015
Häusser-Kinzel, S., and Weber, M. S. (2019). The role of B cells and antibodies in multiple sclerosis, neuromyelitis optica, and related disorders. Front. Immunol. 10:201. doi: 10.3389/fimmu.2019.00201
He, D., Mao, A., Li, Y., Tam, S., Zheng, Y., Yao, X., et al. (2020). TRPC1 participates in the HSV-1 infection process by facilitating viral entry. Sci. Adv. 6:eaaz3367. doi: 10.1126/sciadv.aaz3367
Hoffmann, H.-H., Schneider, W. M., Blomen, V. A., Scull, M. A., Hovnanian, A., Brummelkamp, T. R., et al. (2017). Diverse viruses require the calcium transporter SPCA1 for maturation and spread. Cell Host Microbe 22, 460–470.e5. doi: 10.1016/j.chom.2017.09.002
Hogan, P. G., Chen, L., Nardone, J., and Rao, A. (2003). Transcriptional regulation by calcium, calcineurin, and NFAT. Genes Dev. 17, 2205–2232. doi: 10.1101/gad.1102703
Hogan, P. G., Lewis, R. S., and Rao, A. (2010). Molecular basis of calcium signaling in lymphocytes: STIM and ORAI. Annu. Rev. Immunol. 28, 491–533. doi: 10.1146/annurev.immunol.021908.132550
Holden, C. P., Haughey, N. J., Nath, A., and Geiger, J. D. (1999). Role of Na+/H+ exchangers, excitatory amino acid receptors and voltage-operated Ca2+ channels in human immunodeficiency virus type 1 gp120-mediated increases in intracellular Ca2+ in human neurons and astrocytes. Neuroscience 91, 1369–1378. doi: 10.1016/S0306-4522(98)00714-3
Hou, J., Liu, Z., and Gu, F. (2005). Epidemiology and prevention of hepatitis B virus infection. Int. J. Med. Sci. 2, 50–57. doi: 10.7150/ijms.2.50
Hu, X.-T. (2016). HIV-1 tat-mediated calcium dysregulation and neuronal dysfunction in vulnerable brain regions. Curr. Drug Targets 17, 4–14. doi: 10.2174/1389450116666150531162212
Huang, Y., Winkler, P. A., Sun, W., Lü, W., and Du, J. (2018). Architecture of the TRPM2 channel and its activation mechanism by ADP-ribose and calcium. Nature 562, 145–163. doi: 10.1038/s41586-018-0558-4
Jasenosky, L. D., Scriba, T. J., Hanekom, W. A., and Goldfeld, A. E. (2015). T cells and adaptive immunity to Mycobacterium tuberculosis in humans. Immunol. Rev. 264, 74–87. doi: 10.1111/imr.12274
Ji, T., Corbalán-García, S., and Hubbard, S. R. (2018). Crystal structure of the C-terminal four-helix bundle of the potassium channel KCa3.1. PLoS One 13:e0199942. doi: 10.1371/journal.pone.0199942
Jin, J., Desai, B. N., Navarro, B., Donovan, A., Andrews, N. C., and Clapham, D. E. (2008). Deletion of Trpm7 disrupts embryonic development and thymopoiesis without altering Mg2+ homeostasis. Science 322, 756–760. doi: 10.1126/science.1163493
Jones, C. A., Fernandez, M., Herc, K., Bosnjak, L., Miranda-Saksena, M., Boadle, R. A., et al. (2003). Herpes simplex virus Type 2 induces rapid cell death and functional impairment of murine dendritic cells in vitro. J. Virol. 77, 11139–11149. doi: 10.1128/JVI.77.20.11139-11149.2003
Jorgensen, I., and Miao, E. A. (2015). Pyroptotic cell death defends against intracellular pathogens. Immunol. Rev. 265, 130–142. doi: 10.1111/imr.12287
Joseph, N., Reicher, B., and Barda-Saad, M. (2014). The calcium feedback loop and T cell activation: how cytoskeleton networks control intracellular calcium flux. Biochim. Biophys. Acta 1838, 557–568. doi: 10.1016/j.bbamem.2013.07.009
Junger, W. G. (2011). Immune cell regulation by autocrine purinergic signalling. Nat. Rev. Immunol. 11, 201–212. doi: 10.1038/nri2938
Kahlfuß, S., Simma, N., Mankiewicz, J., Bose, T., Lowinus, T., Klein-Hessling, S., et al. (2014). Immunosuppression by N-methyl-D-aspartate receptor antagonists is mediated through inhibition of Kv1.3 and KCa3.1 channels in T cells. Mol. Cell Biol. 34, 820–831. doi: 10.1128/MCB.01273-13
Karasneh, G. A., and Shukla, D. (2011). Herpes simplex virus infects most cell types in vitro: clues to its success. Virol. J. 8, 1–11. doi: 10.1186/1743-422X-8-481
Kloc, M., Kubiak, J. Z., Li, X. C., and Ghobrial, R. M. (2014). The newly found functions of MTOC in immunological response. J. Leukoc. Biol. 95, 417–430. doi: 10.1189/jlb.0813468
Koelle, D. M., Tigges, M. A., Burke, R. L., Symington, F. W., Riddell, S. R., Abbo, H., et al. (1993). Herpes simplex virus infection of human fibroblasts and keratinocytes inhibits recognition by cloned CD8+ cytotoxic T lymphocytes. J. Clin. Invest. 91, 961–968. doi: 10.1172/JCI116317
Korthals, M., Langnaese, K., Smalla, K.-H., Kähne, T., Herrera-Molina, R., Handschuh, J., et al. (2017). A complex of neuroplastin and plasma membrane Ca2+ ATPase controls T cell activation. Sci. Rep. 71, 1–13. doi: 10.1038/s41598-017-08519-4
Kumar, L., Chou, J., Yee, C. S. K., Borzutzky, A., Vollmann, E. H., Andrian, U. H., et al. (2014). Leucine-rich repeat containing 8A (LRRC8A) is essential for T lymphocyte development and function. J. Exp. Med. 211:929. doi: 10.1084/jem.20131379
La, S., Kim, J., Kwon, B. S., and Kwon, B. (2002). Herpes simplex virus type 1 glycoprotein D inhibits t-cell proliferation. Mol. Cells 14, 398–403.
Lacruz, R. S., and Feske, S. (2015). Diseases caused by mutations in ORAI1 and STIM1. Ann. N. Y. Acad. Sci. 1356, 45–79. doi: 10.1111/nyas.12938
Lam, J., and Wulff, H. (2011). The lymphocyte potassium channels Kv1.3 and KCa3.1 as targets for immunosuppression. Drug Dev. Res. 72, 573–584. doi: 10.1002/ddr.20467
Lamkanfi, M., Kanneganti, T.-D., Van Damme, P., Vanden Berghe, T., Vanoverberghe, I., Vandekerckhove, J., et al. (2008). Targeted peptidecentric proteomics reveals caspase-7 as a substrate of the caspase-1 inflammasomes. Mol. Cell. Proteomics 7, 2350–2363. doi: 10.1074/mcp.M800132-MCP200
Land, J., Lintermans, L. L., Stegeman, C. A., Muñoz-Elías, E. J., Tarcha, E. J., Iadonato, S. P., et al. (2017). Kv1.3 channel blockade modulates the effector function of B cells in granulomatosis with polyangiitis. Front. Immunol. 8:1205. doi: 10.3389/fimmu.2017.01205
Lee, H. G., Cho, M. Z., and Choi, J. M. (2020). Bystander CD4+ T cells: crossroads between innate and adaptive immunity. Exp. Mol. Med. 52, 1255–1263. doi: 10.1038/s12276-020-00486-7
Lewis, R. S. (2001). Calcium signaling mechanisms in T lymphocytes. Annu. Rev. Immunol. 19, 497–521. doi: 10.1146/annurev.immunol.19.1.497
Li, F.-Y., Chaigne-Delalande, B., Kanellopoulou, C., Davis, J. C., Matthews, H. F., Douek, D. C., et al. (2011). Second messenger role for Mg2+ revealed by human T-cell immunodeficiency. Nature 475, 471–476. doi: 10.1038/nature10246
Lian, J., Cuk, M., Kahlfuss, S., Kozhaya, L., Vaeth, M., Rieux-Laucat, F., et al. (2018). ORAI1 mutations abolishing store-operated Ca2+ entry cause anhidrotic ectodermal dysplasia with immunodeficiency. J. Allergy Clin. Immunol. 142, 1297–1310.e11. doi: 10.1016/j.jaci.2017.10.031
Liu, H., Tang, D., Zhou, X., Yang, X., and Chen, A. F. (2020). PhospholipaseCγ1/calcium-dependent membranous localization of Gsdmd-N drives endothelial pyroptosis, contributing to lipopolysaccharide-induced fatal outcome. Am. J. Physiol. Heart Circ. Physiol. 319, H1482–H1495. doi: 10.1152/ajpheart.00731.2019
Liu, X., Zhang, Z., Ruan, J., Pan, Y., Magupalli, V. G., Wu, H., et al. (2016). Inflammasome-activated gasdermin D causes pyroptosis by forming membrane pores. Nature 535, 153–158. doi: 10.1038/nature18629
Madavaraju, K., Koganti, R., Volety, I., Yadavalli, T., and Shukla, D. (2021). Herpes simplex virus cell entry mechanisms: an update. Front. Cell. Infect. Microbiol. 10:852. doi: 10.3389/fcimb.2020.617578
Mahtani, T., and Treanor, B. (2019). Beyond the CRAC: diversification of ion signaling in B cells. Immunol. Rev. 291, 104–122. doi: 10.1111/imr.12770
Manninen, A., and Saksela, K. (2002). HIV-1 Nef interacts with inositol trisphosphate receptor to activate calcium signaling in T cells. J. Exp. Med. 195, 1023–1032. doi: 10.1084/jem.20012039
Marcocci, M. E., Napoletani, G., Protto, V., Kolesova, O., Piacentini, R., Li Puma, D. D., et al. (2020). Herpes simplex Virus-1 in the brain: the dark side of a sneaky infection. Trends Microbiol. 28, 808–820. doi: 10.1016/j.tim.2020.03.003
Martín-Cófreces, N. B., Robles-Valero, J., Cabrero, J. R., Mittelbrunn, M., Gordón-Alonso, M., Sung, C. H., et al. (2008). MTOC translocation modulates IS formation and controls sustained T cell signaling. J. Cell Biol. 182, 951–962. doi: 10.1083/jcb.200801014
Martinon, F., Burns, K., and Tschopp, J. (2002). The inflammasome: a molecular platform triggering activation of inflammatory caspases and processing of proIL-beta. Mol. Cell 10, 417–426. doi: 10.1016/S1097-2765(02)00599-3
McClain, S. L., Clippinger, A. J., Lizzano, R., and Bouchard, M. J. (2007). Hepatitis B virus replication is associated with an HBx-dependent mitochondrion-regulated increase in cytosolic calcium levels. J. Virol. 81, 12061–12065. doi: 10.1128/JVI.00740-07
McClenaghan, C., Hanson, A., Lee, S. J., and Nichols, C. G. (2020). Coronavirus proteins as ion channels: current and potential research. Front. Immunol. 11:2651. doi: 10.3389/fimmu.2020.573339
Melzer, N., Hicking, G., Göbel, K., and Wiendl, H. (2012). TRPM2 cation channels modulate T cell effector functions and contribute to autoimmune CNS inflammation. PLoS One 7:e47617. doi: 10.1371/journal.pone.0047617
Millet, J. K., and Whittaker, G. R. (2018). Physiological and molecular triggers for SARS-CoV membrane fusion and entry into host cells. Virology 517, 3–8. doi: 10.1016/j.virol.2017.12.015
Minke, B., and Cook, B. (2002). TRP channel proteins and signal transduction. Physiol. Rev. 82, 429–472. doi: 10.1152/physrev.00001.2002
Morad, H., Luqman, S., Tan, C.-H., Swann, V., and McNaughton, P. A. (2021). TRPM2 ion channels steer neutrophils towards a source of hydrogen peroxide. Sci. Rep. 11:9339. doi: 10.1038/s41598-021-88224-5
Murray, J. W., Yin, D., and Wolkoff, A. W. (2017). Reduction of organelle motility by removal of potassium and other solutes. PLoS One 12:e0184898. doi: 10.1371/JOURNAL.PONE.0184898
Netea, M. G., Schlitzer, A., Placek, K., Joosten, L. A. B., and Schultze, J. L. (2019). Innate and adaptive immune memory: an evolutionary continuum in the host’s response to pathogens. Cell Host Microbe 25, 13–26. doi: 10.1016/j.chom.2018.12.006
Nicolaou, S. A., Neumeier, L., Takimoto, K., Lee, S. M., Duncan, H. J., Kant, S. K., et al. (2010). Differential calcium signaling and Kv1.3 trafficking to the immunological synapse in systemic lupus erythematosus. Cell Calcium 47, 19–28. doi: 10.1016/j.ceca.2009.11.001
Nielsen, M. M., Witherden, D. A., and Havran, W. L. (2017). γδ T cells in homeostasis and host defence of epithelial barrier tissues. Nat. Rev. Immunol. 17, 733–745. doi: 10.1038/nri.2017.101
Nieto-Torres, J. L., Verdiá-Báguena, C., Jimenez-Guardeño, J. M., Regla-Nava, J. A., Castaño-Rodriguez, C., Fernandez-Delgado, R., et al. (2015). Severe acute respiratory syndrome coronavirus E protein transports calcium ions and activates the NLRP3 inflammasome. Virology 485, 330–339. doi: 10.1016/j.virol.2015.08.010
Oh-Hora, M. (2009). Calcium signaling in the development and function of T-lineage cells. Immunol. Rev. 231, 210–224. doi: 10.1111/j.1600-065X.2009.00819.x
Omar, S., Clarke, R., Abdullah, H., Brady, C., Corry, J., Winter, H., et al. (2017). Respiratory virus infection up-regulates TRPV1, TRPA1 and ASICS3 receptors on airway cells. PLoS One 12:e0171681. doi: 10.1371/journal.pone.0171681
Omilusik, K., Priatel, J. J., Chen, X., Wang, Y. T., Xu, H., Choi, K. B., et al. (2011). The CaV1.4 calcium channel is a critical regulator of T cell receptor signaling and naive T Cell Homeostasis. Immunity 35, 349–360. doi: 10.1016/j.immuni.2011.07.011
Ott, M., Emiliani, S., Van Lint, C., Herbein, G., Lovett, J., Chirmule, N., et al. (1997). Immune hyperactivation of HIV-1-infected T cells mediated by Tat and the CD28 pathway. Science 275, 1481–1485. doi: 10.1126/science.275.5305.1481
Panyi, G., Varga, Z., and Gáspár, R. (2004). Ion channels and lymphocyte activation. Immunol. Lett. 92, 55–66. doi: 10.1016/j.imlet.2003.11.020
Parenti, A., De Logu, F., Geppetti, P., and Benemei, S. (2016). What is the evidence for the role of TRP channels in inflammatory and immune cells? Br. J. Pharmacol. 173, 953–969. doi: 10.1111/bph.13392
Perry, J. L., Ramachandran, N. K., Utama, B., and Hyser, J. M. (2015). Use of genetically-encoded calcium indicators for live cell calcium imaging and localization in virus-infected cells. Methods 90, 28–38. doi: 10.1016/j.ymeth.2015.09.004
Petho, Z., Balajthy, A., Bartok, A., Bene, K., Somodi, S., Szilagyi, O., et al. (2016). The anti-proliferative effect of cation channel blockers in T lymphocytes depends on the strength of mitogenic stimulation. Immunol. Lett. 171, 60–69. doi: 10.1016/j.imlet.2016.02.003
Poggi, A., Rubartelli, A., and Raffaella Zocchi, M. (1998). Involvement of dihydropyridine-sensitive calcium channels in human dendritic cell function competition bY HIV-1 Tat. J. Biol. Chem. 273, 7205–7209. doi: 10.1074/jbc.273.13.7205
Polverino, F., Lu, B., Quintero, J. R., Vargas, S. O., Patel, A. S., Owen, C. A., et al. (2019). CFTR regulates B cell activation and lymphoid follicle development. Respir. Res. 20:133. doi: 10.1186/s12931-019-1103-1
Pontelli, M. C., Castro, I. A., Martins, R. B., Veras, F. P., Serra, L., and La et al. (2020). Infection of human lymphomononuclear cells by SARS-CoV-2. bioRxiv [preprint] doi: 10.1101/2020.07.28.225912
Prakriya, M., and Lewis, R. S. (2015). Store-operated calcium channels. Physiol. Rev. 95, 1383–1436. doi: 10.1152/physrev.00020.2014
Quintana, A., and Hoth, M. (2012). Mitochondrial dynamics and their impact on T cell function. Cell Calcium 52, 57–63. doi: 10.1016/j.ceca.2012.02.005
Quintana, A., Pasche, M., Junker, C., Al-Ansary, D., Rieger, H., Kummerow, C., et al. (2011). Calcium microdomains at the immunological synapse: how ORAI channels, mitochondria and calcium pumps generate local calcium signals for efficient T-cell activation. EMBO J. 30, 3895–3912. doi: 10.1038/emboj.2011.289
Raiden, S., Sananez, I., Remes-Lenicov, F., Pandolfi, J., Romero, C., De Lillo, L., et al. (2017). Respiratory syncytial virus (RSV) infects CD4+ T cells: frequency of circulating CD4+ RSV+ T cells as a marker of disease severity in young children. J. Infect. Dis. 215, 1049–1058. doi: 10.1093/infdis/jix070
RamaKrishnan, A. M., and Sankaranarayanan, K. (2016). Understanding autoimmunity: the ion channel perspective. Autoimmun. Rev. 15, 585–620. doi: 10.1016/j.autrev.2016.02.004
Ravell, J. C., Chauvin, S. D., He, T., and Lenardo, M. (2020). An update on XMEN disease. J. Clin. Immunol. 40, 671–681. doi: 10.1007/s10875-020-00790-x
Ravell, J., Chaigne-Delalande, B., and Lenardo, M. (2014). X-linked immunodeficiency with magnesium defect, epstein-barr virus infection, and neoplasia disease: a combined immune deficiency with magnesium defect. Curr. Opin. Pediatr. 26, 713–719. doi: 10.1097/MOP.0000000000000156
Retamal-Díaz, A. R., Tognarelli, E., Kalergis, A. M., Bueno, S. M., and González, P. A. (2016). Immune Evasion by Herpes Simplex Viruses, Herpesviridae, Jozsef Ongradi, IntechOpen. doi: 10.5772/64128 Available online at: https://www.intechopen.com/chapters/51597
Rossi, E., Meuser, M. E., Cunanan, C. J., and Cocklin, S. (2021). Structure, function, and interactions of the hiv-1 capsid protein. Life 11, 1–25. doi: 10.3390/life11020100
Rubaiy, H. N. (2017). A short guide to electrophysiology and ion channels. J. Pharm. Pharm. Sci. 20, 48–67. doi: 10.18433/J32P6R
Samanta, A., Hughes, T. E. T., and Moiseenkova-Bell, V. Y. (2018). Transient receptor potential (TRP) channels. Subcell. Biochem. 87:1416. doi: 10.1007/978-981-10-7757-9_6
Sawada, A., Takihara, Y., Kim, J. Y., Matsuda-Hashii, Y., Tokimasa, S., Fujisaki, H., et al. (2003). A congenital mutation of the novel gene LRRC8 causes agammaglobulinemia in humans. J. Clin. Invest. 112, 1707–1713. doi: 10.1172/JCI18937
Scharenberg, A. M., Humphries, L. A., and Rawlings, D. J. (2007). Calcium signalling and cell-fate choice in B cells. Nat. Rev. Immunol. 7, 778–789. doi: 10.1038/nri2172
Schenk, U., Westendorf, A. M., Radaelli, E., Casati, A., Ferro, M., Fumagalli, M., et al. (2008). Purinergic control of T cell activation by ATP released through pannexin-1 hemichannels. Sci. Signal. 1:ra6. doi: 10.1126/scisignal.1160583
Schmitz, C., Perraud, A.-L., Johnson, C. O., Inabe, K., Smith, M. K., Penner, R., et al. (2003). Regulation of vertebrate cellular Mg2+ homeostasis by TRPM7. Cell 114, 191–200. doi: 10.1016/S0092-8674(03)00556-7
Schorge, S. (2018). Channelopathies go above and beyond the channels. Neuropharmacology 132, 1–2. doi: 10.1016/j.neuropharm.2018.02.011
Seeger, C., and Mason, W. S. (2015). Molecular biology of hepatitis B virus infection. Virology 479–480, 672–686. doi: 10.1016/j.virol.2015.02.031
Serebrovska, Z. O., Chong, E. Y., Serebrovska, T. V., Tumanovska, L. V., and Xi, L. (2020). Hypoxia, HIF-1α, and COVID-19: from pathogenic factors to potential therapeutic targets. Acta Pharmacol. Sin. 41, 1539–1546. doi: 10.1038/s41401-020-00554-8
Serra, S. A., Stojakovic, P., Amat, R., Rubio-Moscardo, F., Latorre, P., Seisenbacher, G., et al. (2021). LRRC8A-containing chloride channel is crucial for cell volume recovery and survival under hypertonic conditions. Proc. Natl. Acad. Sci. 118:e2025013118. doi: 10.1073/pnas.2025013118
Shahrabadi, M. S., and Lee, P. W. (1988). Calcium requirement for syncytium formation in HEp-2 cells by respiratory syncytial virus. J. Clin. Microbiol. 26, 139–141. doi: 10.1128/jcm.26.1.139-141.1988
Shi, J., Gao, W., and Shao, F. (2017). Pyroptosis: gasdermin-mediated programmed necrotic cell death. Trends Biochem. Sci. 42, 245–254. doi: 10.1016/j.tibs.2016.10.004
Shi, J., Zhao, Y., Wang, K., Shi, X., Wang, Y., Huang, H., et al. (2015). Cleavage of GSDMD by inflammatory caspases determines pyroptotic cell death. Nature 526, 660–665. doi: 10.1038/nature15514
Sloan, D. D., Han, J.-Y., Sandifer, T. K., Stewart, M., Hinz, A. J., Yoon, M., et al. (2006). Inhibition of TCR signaling by herpes simplex virus. J. Immunol. 176, 1825–1833. doi: 10.4049/jimmunol.176.3.1825
Smith-Garvin, J. E., Koretzky, G. A., and Jordan, M. S. (2009). T cell activation. Annu. Rev. Immunol. 27, 591–619. doi: 10.1146/annurev.immunol.021908.132706
Straus, M. R., Bidon, M., Tang, T., Whittaker, G. R., and Daniel, S. (2020a). FDA approved calcium channel blockers inhibit SARS-CoV-2 infectivity in epithelial lung cells. bioRxiv [Preprint] doi: 10.1101/2020.07.21.214577
Straus, M. R., Tang, T., Lai, A. L., Flegel, A., Bidon, M., Freed, J. H., et al. (2020b). Ca 2+ ions promote fusion of middle east respiratory syndrome coronavirus with host cells and increase infectivity. J. Virol. 94, 426–446. doi: 10.1128/JVI.00426-20
Sumoza-Toledo, A., and Penner, R. (2011). TRPM2: a multifunctional ion channel for calcium signalling. J. Physiol. 589, 1515–1525. doi: 10.1113/jphysiol.2010.201855
Syed Mortadza, S. A., Wang, L., Li, D., and Jiang, L.-H. (2015). TRPM2 channel-mediated ROS-sensitive Ca(2+) signaling mechanisms in immune cells. Front. Immunol. 6:407. doi: 10.3389/fimmu.2015.00407
Tabor, D. E., Fernandes, F., Langedijk, A. C., Wilkins, D., Lebbink, R. J., Tovchigrechko, A., et al. (2021). Global molecular epidemiology of respiratory syncytial virus from the 2017-2018 INFORM-RSV study. J. Clin. Microbiol. 59:e1828-20. doi: 10.1128/JCM.01828-20
Teisseyre, A., Palko-Labuz, A., Sroda-Pomianek, K., and Michalak, K. (2019). Voltage-gated potassium channel Kv1.3 as a target in therapy of cancer. Front. Oncol. 9:933. doi: 10.3389/fonc.2019.00933
Traynelis, S. F., Wollmuth, L. P., McBain, C. J., Menniti, F. S., Vance, K. M., Ogden, K. K., et al. (2010). Glutamate receptor ion channels: structure, regulation, and function. Pharmacol. Rev. 62, 405–496. doi: 10.1124/pr.109.002451
Trebak, M., and Kinet, J.-P. (2019). Calcium signalling in T cells. Nat. Rev. Immunol. 19, 154–169. doi: 10.1038/s41577-018-0110-7
Triantafilou, K., Kar, S., Vakakis, E., Kotecha, S., and Triantafilou, M. (2013). Human respiratory syncytial virus viroporin SH: a viral recognition pathway used by the host to signal inflammasome activation. Thorax 68, 66–75. doi: 10.1136/thoraxjnl-2012-202182
Vaeth, M., and Feske, S. (2018). Ion channelopathies of the immune system. Curr. Opin. Immunol. 52, 39–50. doi: 10.1016/j.coi.2018.03.021
Vaeth, M., Kahlfuss, S., and Feske, S. (2020). CRAC channels and calcium signaling in T cell-mediated immunity. Trends Immunol. 41, 878–901. doi: 10.1016/j.it.2020.06.012
Valle-Reyes, S., Valencia-Cruz, G., Liñan-Rico, L., Pottosin, I., and Dobrovinskaya, O. (2018). Differential activity of voltage- and Ca2+-dependent potassium channels in leukemic T cell lines: Jurkat cells represent an exceptional case. Front. Physiol. 9:499 doi: 10.3389/fphys.2018.00499
Venkatakrishnan, B., and Zlotnick, A. (2016). The structural biology of hepatitis B virus: form and function. Annu. Rev. Virol. 3, 429–451. doi: 10.1146/annurev-virology-110615-042238
Vennekens, R., and Nilius, B. (2007). Insights into TRPM4 function, regulation and physiological role. Handb. Exp. Pharmacol. 179, 269–285. doi: 10.1007/978-3-540-34891-7_16
Verheugen, J. A. H., Le Deist, F., Devignot, V., and Korn, H. (1997). Enhancement of calcium signaling and proliferation responses in activated human T lymphocytes. Cell Calcium 21, 1–17. doi: 10.1016/S0143-4160(97)90092-0
Veytia-Bucheli, J. I., Jiménez-Vargas, J. M., Melchy-Pérez, E. I., Sandoval-Hernández, M. A., Possani, L. D., and Rosenstein, Y. (2018). Kv1.3 channel blockade with the Vm24 scorpion toxin attenuates the CD4+ effector memory T cell response to TCR stimulation. Cell Commun. Signal. 16:45. doi: 10.1186/s12964-018-0257-7
Vig, M., and Kinet, J.-P. (2009). Calcium signaling in immune cells. Nat. Immunol. 10, 21–27. doi: 10.1038/ni.f.220
Vukcevic, M., Spagnoli, G. C., Iezzi, G., Zorzato, F., and Treves, S. (2008). Ryanodine receptor activation by Cav1.2 is involved in dendritic cell major histocompatibility complex class II surface expression. J. Biol. Chem. 283, 34913–34922. doi: 10.1074/jbc.M804472200
Wang, M.-Y., Zhao, R., Gao, L.-J., Gao, X.-F., Wang, D.-P., and Cao, J.-M. (2020). SARS-CoV-2: structure, biology, and structure-based therapeutics development. Front. Cell. Infect. Microbiol. 10:587269. doi: 10.3389/fcimb.2020.587269
Weber, K. S., Hildner, K., Murphy, K. M., and Allen, P. M. (2010). Trpm4 differentially regulates Th1 and Th2 function by altering calcium signaling and NFAT localization. J. Immunol. 185, 2836–2846. doi: 10.4049/jimmunol.1000880
Wenning, A. S., Neblung, K., Strauss, B., Wolfs, M.-J., Sappok, A., Hoth, M., et al. (2011). TRP expression pattern and the functional importance of TRPC3 in primary human T-cells. Biochim. Biophys. Acta 1813, 412–423. doi: 10.1016/j.bbamcr.2010.12.022
Whitacre, J. M., Lin, J., and Harding, A. (2012). T cell adaptive immunity proceeds through environment-induced adaptation from the exposure of cryptic genetic variation. Front. Genet. 3:5. doi: 10.3389/fgene.2012.00005
Woehrle, T., Yip, L., Elkhal, A., Sumi, Y., Chen, Y., Yao, Y., et al. (2010). Pannexin-1 hemichannel-mediated ATP release together with P2X1 and P2X4 receptors regulate T-cell activation at the immune synapse. Blood 116, 3475–3484. doi: 10.1182/blood-2010-04-277707
Wulff, H., Castle, N. A., and Pardo, L. A. (2009). Voltage-gated potassium channels as therapeutic targets. Nat. Rev. Drug Discov. 8, 982–1001. doi: 10.1038/nrd2983
Wulff, H., Knaus, H.-G., Pennington, M., and Chandy, K. G. (2004). K + channel expression during B cell differentiation: implications for immunomodulation and autoimmunity. J. Immunol. 173, 776–786. doi: 10.4049/jimmunol.173.2.776
Xia, B., Shen, X., He, Y., Pan, X., Wang, Y., Yang, F., et al. (2020). SARS-CoV-2 envelope protein causes acute respiratory distress syndrome (ARDS)-like pathological damage and constitutes an antiviral target. bioRxiv [Preprint] doi: 10.1101/2020.06.27.174953
Yamamoto, S., Takahashi, N., and Mori, Y. (2010). Chemical physiology of oxidative stress-activated TRPM2 and TRPC5 channels. Prog. Biophys. Mol. Biol. 103, 18–27. doi: 10.1016/j.pbiomolbio.2010.05.005
Yan, Q., Lan, Y.-H., Huang, Y.-X., Fan, R.-S., Liu, L., Song, S.-P., et al. (2016). Hepatitis B virus replication is upregulated in proliferated peripheral blood lymphocytes. Mol. Med. Rep. 13, 3581–3587. doi: 10.3892/mmr.2016.4973
Yang, P.-C. C., and Jafri, M. S. (2020). Ca2+ signaling in T lymphocytes: the interplay of the endoplasmic reticulum, mitochondria, membrane potential, and CRAC channels on transcription factor activation. Heliyon 6:e03526. doi: 10.1016/j.heliyon.2020.e03526
Yao, J.-H., Liu, Z.-J., Yi, J.-H., Wang, J., and Liu, Y.-N. (2018). Hepatitis B virus X protein upregulates intracellular calcium signaling by binding C-terminal of Orail protein. Curr. Med. Sci. 38, 26–34. doi: 10.1007/s11596-018-1843-z
Yip, L., Woehrle, T., Corriden, R., Hirsh, M., Chen, Y., Inoue, Y., et al. (2009). Autocrine regulation of T-cell activation by ATP release and P2X7 receptors. FASEB J. 23, 1685–1693. doi: 10.1096/fj.08-126458
Zainullina, L. F., Yamidanov, R. S., Vakhitov, V. A., and Vakhitova, Y. V. (2011). NMDA receptors as a possible component of store-operated Ca 2+ entry in human T-lymphocytes. Biochemistry 76, 1220–1226. doi: 10.1134/S0006297911110034
Zhang, Q., Hsia, S. C., and Martin-Caraballo, M. (2019). Regulation of T-type Ca2+ channel expression by interleukin-6 in sensory-like ND7/23 cells post-herpes simplex virus (HSV-1) infection. J. Neurochem. 151, 238–254. doi: 10.1111/jnc.14697
Zhou, X., Chen, D., Wang, L., Zhao, Y., Wei, L., Chen, Z., et al. (2020). Low serum calcium: a new, important indicator of COVID-19 patients from mild/moderate to severe/critical. Biosci. Rep. doi: 10.1042/BSR20202690 Online ahead of print.
Zhou, Y., Frey, T. K., and Yang, J. J. (2009). Viral calciomics: interplays between Ca2+ and virus. Cell Calcium 46, 1–17. doi: 10.1016/j.ceca.2009.05.005
Zocchi, M. R., Rubartelli, A., Morgavi, P., and Poggi, A. (1998). HIV-1 tat inhibits human natural killer cell function by blocking L-type calcium channels. J. Immunol. 161, 2938–2943.
Zou, Z.-G., Rios, F. J., Montezano, A. C., and Touyz, R. M. (2019). TRPM7, magnesium, and signaling. Int. J. Mol. Sci. 20:1877. doi: 10.3390/ijms20081877
Keywords: Ca2+, Ca2+ channels, T cells, immune response, viral infection
Citation: Bohmwald K, Gálvez NMS, Andrade CA, Mora VP, Muñoz JT, González PA, Riedel CA and Kalergis AM (2021) Modulation of Adaptive Immunity and Viral Infections by Ion Channels. Front. Physiol. 12:736681. doi: 10.3389/fphys.2021.736681
Received: 05 July 2021; Accepted: 10 September 2021;
Published: 08 October 2021.
Edited by:
Barbara Niemeyer, Saarland University, GermanyReviewed by:
Ossama Kashlan, University of Pittsburgh, United StatesCopyright © 2021 Bohmwald, Gálvez, Andrade, Mora, Muñoz, González, Riedel and Kalergis. This is an open-access article distributed under the terms of the Creative Commons Attribution License (CC BY). The use, distribution or reproduction in other forums is permitted, provided the original author(s) and the copyright owner(s) are credited and that the original publication in this journal is cited, in accordance with accepted academic practice. No use, distribution or reproduction is permitted which does not comply with these terms.
*Correspondence: Alexis M. Kalergis, YWthbGVyZ2lzQGJpby5wdWMuY2w=; YWthbGVyZ2lzQGljbG91ZC5jb20=
Disclaimer: All claims expressed in this article are solely those of the authors and do not necessarily represent those of their affiliated organizations, or those of the publisher, the editors and the reviewers. Any product that may be evaluated in this article or claim that may be made by its manufacturer is not guaranteed or endorsed by the publisher.
Research integrity at Frontiers
Learn more about the work of our research integrity team to safeguard the quality of each article we publish.