- 1Department of Internal Medicine, Justus Liebig University Giessen, Universities of Giessen and Marburg Lung Center (UGMLC), Member of the German Center for Lung Research (DZL), Giessen, Germany
- 2The Cardio-Pulmonary Institute (CPI), Giessen, Germany
- 3Institute for Lung Health (ILH), Giessen, Germany
Protein transcription, translation, and folding occur continuously in every living cell and are essential for physiological functions. About one-third of all proteins of the cellular proteome interacts with the endoplasmic reticulum (ER). The ER is a large, dynamic cellular organelle that orchestrates synthesis, folding, and structural maturation of proteins, regulation of lipid metabolism and additionally functions as a calcium store. Recent evidence suggests that both acute and chronic hypercapnia (elevated levels of CO2) impair ER function by different mechanisms, leading to adaptive and maladaptive regulation of protein folding and maturation. In order to cope with ER stress, cells activate unfolded protein response (UPR) pathways. Initially, during the adaptive phase of ER stress, the UPR mainly functions to restore ER protein-folding homeostasis by decreasing protein synthesis and translation and by activation of ER-associated degradation (ERAD) and autophagy. However, if the initial UPR attempts for alleviating ER stress fail, a maladaptive response is triggered. In this review, we discuss the distinct mechanisms by which elevated CO2 levels affect these molecular pathways in the setting of acute and chronic pulmonary diseases associated with hypercapnia.
Introduction
Carbon dioxide (CO2) is a metabolic product of cellular oxidative respiration, and is primarily eliminated from the blood and tissues by the lungs under physiological conditions. An elevation in CO2 partial pressure in arterial blood over 45 mmHg is termed hypercapnia. Increased CO2 levels are often observed in conditions where an impairment of the alveolar-capillary barrier function or a decline in alveolar ventilation occurs (Vadasz et al., 2012b; Herold et al., 2013). Various acute and chronic lung diseases, such as acute respiratory distress syndrome (ARDS), chronic obstructive pulmonary disease (COPD), asthma and cystic fibrosis are frequently accompanied by hypercapnia (Vadasz et al., 2012b; Radermacher et al., 2017). Furthermore, elevated CO2 levels and intermittent hypoxia combined with hypercapnia play a role in the pathogenesis of obstructive sleep apnea, atherosclerosis and obesity (Kikuchi et al., 2017; Imamura et al., 2019; Xue et al., 2021).
It is increasingly evident, that various non-excitable cells, such as alveolar epithelial cells, fibroblasts and immune cells are sensitive to the changes in CO2 concentrations independently of intra- and extracellular pH, reactive oxygen species (ROS) and involvement of the carbonic anhydrases (Putnam et al., 2004; Shigemura et al., 2017; Cummins et al., 2019). In contrast to earlier reports, which suggested that hypercapnia might be tolerated or even beneficial in the setting of critically ill patients (Fuller et al., 2017; Roberts et al., 2018); more recent studies have shown that elevated CO2 levels are associated with higher complication rates, increased risk of exacerbations, more severe disease states, worse outcomes and an increased risk of mortality both for acute and chronic lung diseases (Yang et al., 2015; Nin et al., 2017; Husain-Syed et al., 2020; Shigemura et al., 2020). In addition, translational studies established that high CO2 levels impair alveolar fluid clearance, innate immunity and cellular host defense, decrease cytokine production, downregulate phagocytosis and macrophage activity. Hypercapnia also stimulates nitric oxide (NO) production, therefore negatively impacting on pulmonary metabolism, aggravates epithelial cell repair, alters cellular lipid metabolism, decreases muscle anabolism, increases smooth muscle airway contractility and muscle catabolism, thus contributing to disease states and impaired recovery (Lang et al., 2000; Vadasz et al., 2008; Gates et al., 2013; Jaitovich et al., 2015; Kikuchi et al., 2017; Shigemura et al., 2018; Korponay et al., 2019). In addition, recent studies suggest that elevated CO2 levels increase mortality in animal models of acute lung injury secondary to viral and bacterial insults (Gates et al., 2013; Casalino-Matsuda et al., 2020).
Protein transcription, translation, folding, and maturation continuously occur in each living cell and are essential for normal physiological function. In the cell, approximately one-third of the proteome and most of the secretory and membrane proteins are processed through the endoplasmic reticulum (ER) (Brodsky and Skach, 2011). In addition, the ER regulates lipid metabolism and serves as an intracellular calcium store (Hetz et al., 2015; Schwarz and Blower, 2016). The ER coordinates numerous co- and post-translational protein modifications, including N-linked glycosylation, formation of disulfide-bonds, sequence cleavage, chaperone-assisted protein folding, recognition and targeting of the ER-localized proteins for degradation (Ellgaard and Helenius, 2003; Araki and Nagata, 2011; Ellgaard et al., 2018). Numerous ER-resident chaperons, such as calnexin, calreticulin and binding immunoglobulin protein (BiP) orchestrate co-translational folding/refolding of nascent proteins. In addition, these chaperons play a central role in the removal of terminally misfolded proteins via ER-associated degradation (ERAD) and are key players of unfolded protein response (UPR) during ER stress (Hebert and Molinari, 2007; Halperin et al., 2014). Up to date, three main UPR pathways, named by ER-localized proteins have been characterized: inositol-requiring enzyme 1 (IRE1), protein kinase RNA-activated (PKR)-like ER kinase (PERK), and activating transcription factor-6 (ATF6). An increase of misfolded/unfolded proteins in the ER leads to dissociation of BiP from ER stress sensors, autophosphorylation of the sensors and subsequent activation of UPR (Wang and Kaufman, 2016; Almanza et al., 2019).
Of note, ER stress plays a pivotal role in the pathomechanism of various respiratory diseases, including but not limited to COPD (and in particular cigarette smoke exposure), viral and bacterial pneumonia, asthma, interstitial lung diseases and cystic fibrosis (Korfei et al., 2008; Lawson et al., 2011; Kenche et al., 2013; Kim et al., 2013; van ’t Wout et al., 2015; Lee et al., 2016; Marciniak, 2017; Tang et al., 2017; Schmoldt et al., 2019), many of which are accompanied by hypercapnia (Vadasz et al., 2012b; Shigemura et al., 2020). Notably, these disease states also often lead to hypoxia. Indeed, low oxygen levels have also been shown to negatively impact ER homeostasis, thus inducing ER stress (Chipurupalli et al., 2019; Bradley et al., 2021). Although the effects of hypoxia on the ER lie beyond the scope of the current manuscript, it is increasingly evident that hypoxia negatively affects ER function in alveolar epithelial cells and macrophages in the lung. These effects involve the downregulation of metabolic processes and disruption of the ER chaperone activity, which result in activation of key elements of the UPR, such as PERK, eIF2a, and IRE1α (Burman et al., 2018; Delbrel et al., 2018, 2019; Diaz-Bulnes et al., 2019; Bradley et al., 2021). Another cellular organelle that is tightly related to the ER is the peroxisome (Dimitrov et al., 2013). Of note, recent publications suggest that hypercapnia affects peroxisome signaling by modulation of the activity and expression of peroxisome proliferator-activated receptors (Huang et al., 2016; Kikuchi et al., 2017). At the molecular level, elevated CO2 has been shown to activate kinases and proteins that are known to regulate ER function and/or participate in UPR, such as c-Jun N-terminal kinase (JNK), extracellular signal-regulated kinase (ERK1/2), AMP-activated protein kinase (AMPK), B-cell lymphoma 2 (Bcl-2), and caspase-7 (Vadasz et al., 2008, 2012a; Welch et al., 2010; Casalino-Matsuda et al., 2015; Dada et al., 2015; Shigemura et al., 2018). Furthermore, recent reports suggest that CO2 can impact post-translational protein biochemistry by carbamate formation and subsequent protein carbamylation (Meigh et al., 2013; Linthwaite et al., 2018). In this review, we will focus on the molecular mechanisms by which hypercapnia impairs protein folding in the ER. Unfolding/misfolding of proteins in the ER by elevated CO2 levels result in enhanced protein retention or degradation, thereby impairing subsequent protein trafficking, and thus overall cellular and tissue function.
Hypercapnia and Endoplasmic Reticulum Homeostasis
It is well documented that protein maturation in the ER requires a specific milieu, including high Ca2+ levels, sufficient amounts of ATP, and an appropriate oxidizing environment (Jager et al., 2012; Almanza et al., 2019). In particular, in the past two decades, a number of studies revealed that disruption of the ER folding environment leads to accumulation of misfolded/unfolded proteins, induces ER stress and subsequent activation of the UPR (Araki and Nagata, 2011; Wang and Kaufman, 2016).
Elevated CO2 Levels, Cellular ATP and Endoplasmic Reticulum Redox Balance
Protein translation and subsequent post-translational modification of ER-resident proteins are among the highest energy consuming cellular processes (Wieser and Krumschnabel, 2001). These ER processes, including folding, translocation, quality control and UPR require energy in form of ATP. The source of ATP depends on the cellular metabolic state. ATP is generated either by oxidative phosphorylation or by glycolysis (Depaoli et al., 2019). When ATP is derived from active mitochondrial respiration (oxidative phosphorylation), the ATP molecules are possibly transferred directly into the ER through mitochondria-associated ER membrane (MAM) sites (Depaoli et al., 2019; Fan and Simmen, 2019). However, when glycolysis is the major source of cellular energy, ATP enters the ER directly from the cytosol (Depaoli et al., 2019). Of note, most of the ER-folding chaperons of the HSP70 and HSP90 protein families are ATP-dependent, and thus require energy for proper function (Sala et al., 2017). A decline in the ER ATP levels has been shown to impair disulfide bond formation, negatively impacts protein glycosylation and provokes inappropriate calcium signaling (Bravo et al., 2013).
Several studies have demonstrated that hypercapnia aggravates cellular ATP production. For example, in a recent publication it was shown that epithelial and mesenchymal cells exposed to elevated CO2 levels exhibit mitochondrial dysfunction and decreased ATP production (Vohwinkel et al., 2011). The reduction in ATP levels is induced by CO2-dependent upregulation of miR-183, which in turn downregulates expression of isocitrate dehydrogenase 2 (IDH2), a key enzyme involved in the tricarboxylic acid (TCA) cycle. This inhibition of the TCA cycle impairs mitochondrial and thus metabolic function and leads to downregulation of cellular proliferation. Importantly, these deleterious effects of hypercapnia can be rescued by application of α-ketoglutaric acid (α-KG), an intermediate metabolite in the TCA cycle, or by overexpression of IDH2, further highlighting the central role of the impeded TCA cycle in the hypercapnia-induced metabolic dysfunction (Vohwinkel et al., 2011). In line with these findings, exposure of primary human airway epithelial and lung endothelial cells to hypercapnia has been shown to attenuate mitochondrial membrane potential, decrease ATP production, and induce mitochondrial dysfunction, thus decreasing reparative potential of the cell (Fergie et al., 2019).
Apart from ATP, protein folding and formation of disulfide bonds require a specific oxidizing environment of the ER (Araki and Nagata, 2011). The coordinated interaction between glutathione disulfide, hydrogen sulfide, hydrogen peroxide and NO maintains an optimal redox balance in the ER and mediates sulfenylation, sulfhydration and nitrosylation of the folded proteins (Banhegyi et al., 2012; Ellgaard et al., 2018). In addition, oxidative modifications in the ER are reduced by ER-resident oxidoreductases and protein disulfide isomerases, such as ER oxidoreductin 1 (Ero1), protein disulfide-isomerase (PDI), and fumarate reductase 2 (OSM1) (Tu et al., 2000; Araki and Nagata, 2011; Kim et al., 2018). Thus, perturbations of the ER redox balance [by e.g., dithiothreitol (DTT)] cause protein misfolding, activate ER stress and initiate UPR pathways, leading to cellular dysfunction or even cell death (Tatu et al., 1993; Bergmann and Molinari, 2018). Recent evidence suggests that elevated CO2 levels alter the oxidizing environment of the ER. Recently, we were able to show that elevated CO2 levels induce ER oxidation in hypercapnia-exposed alveolar epithelial cells (Kryvenko et al., 2020). One of the well-characterized types of oxidative protein modification is carbonylation of protein targets. This biochemical reaction is characterized by an irreversible non-enzymatic attachment of carbonyl groups to proteins, which disrupts normal protein folding in the ER by either modifying nascent proteins or by impairing the structure of ER chaperons (England and Cotter, 2004; Dalle-Donne et al., 2006). Interestingly, increased oxidation in the ER leads to ER retention and carbonylation of the Na,K-ATPase β-subunit (Kryvenko et al., 2020, 2021a), a protein that plays a central role in alveolar epithelial junctional function and clearance of alveolar edema, and function of which is impaired in the setting of acute lung injury and hypercapnia (Figure 1; Vadasz et al., 2007, 2008; Kryvenko and Vadasz, 2021). The influence of elevated CO2 levels on oxidative processes was also reported in another recent publication in which exposure of human bronchial epithelial cells to hypercapnia led to upregulation of genes involved in cellular responses to oxidative stress pathways (Casalino-Matsuda et al., 2018). Whether hypercapnia affects ER-resident oxidoreductases and protein disulfide isomerases (such as Ero1, PDI, and OSM1) is currently unknown and needs further investigation. Moreover, the ER, redox reactions and iron metabolism are tightly linked together (Banhegyi et al., 2012; Andreini et al., 2018; Hedison and Scrutton, 2019). Therefore, the role of the iron-proteome in CO2 sensing and hypercapnia-induced ER oxidation status changes needs further attention.
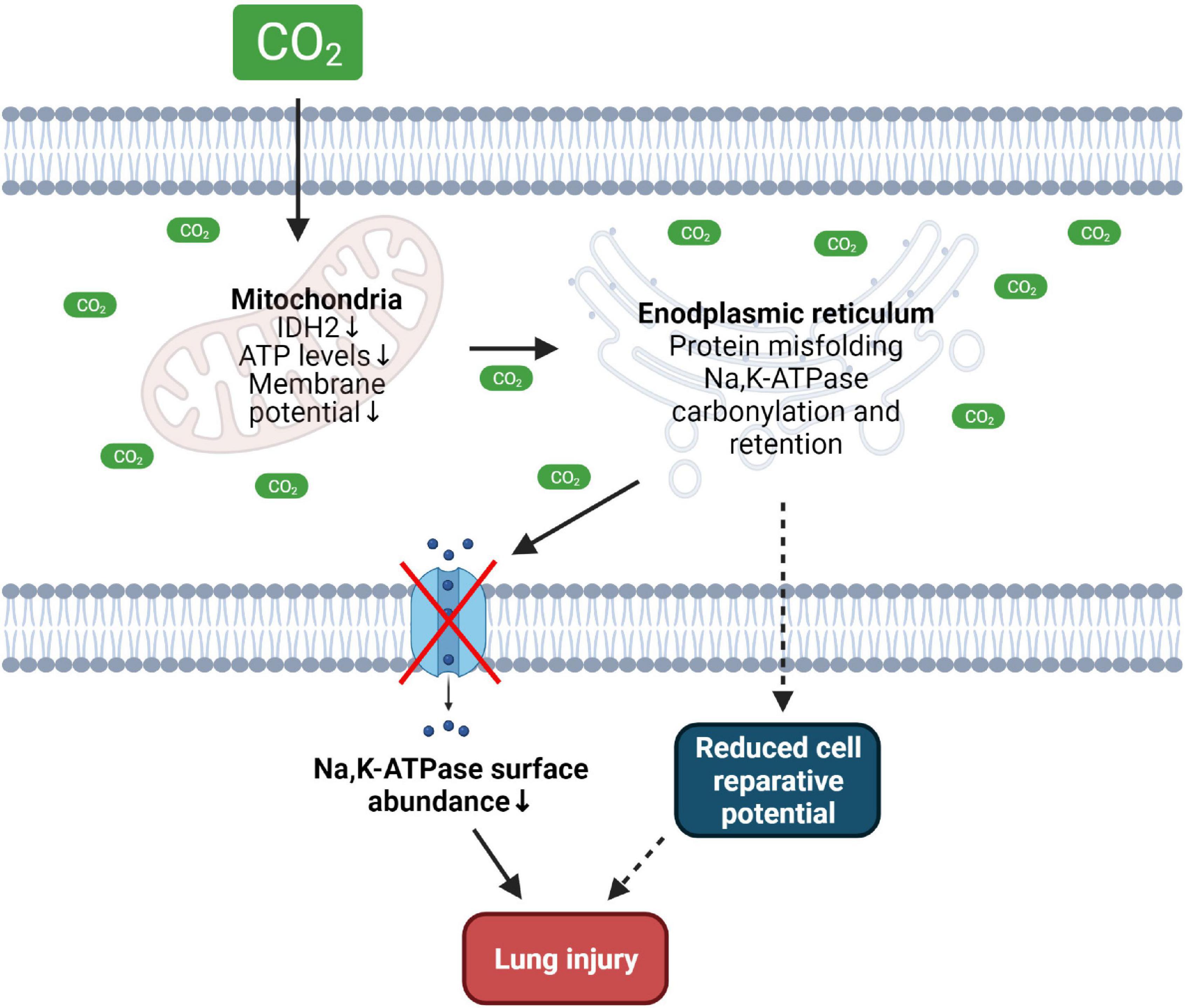
Figure 1. Effects of hypercapnia on mitochondrial and ER function driving lung injury. Increased CO2 levels cause mitochondrial dysfunction, reduce intracellular ATP levels thus altering ER function and promoting misfolding of the Na,K-ATPase protein leading to decreased plasma membrane abundance of the transporter. Hypercapnia-induced dysfunction of the mitochondria and ER may reduce cell reparative potential thus contributing to lung injury. IDH2, isocitrate dehydrogenase 2. Created with BioRender.com.
Hypercapnia and Endoplasmic Reticulum Calcium Homeostasis
The ER also represents a major calcium storage organelle, which regulates intracellular Ca2+ concentrations and oscillations (Krebs et al., 2015). Importantly, Ca2+ signaling is centrally involved in several intracellular pathways regulating protein synthesis, cell proliferation, metabolism and apoptosis (Bagur and Hajnoczky, 2017). The protein maturation process in the ER greatly relies on Ca2+-dependent chaperons, such as calnexin and calreticulin, two key players in the protein folding cycle (Araki and Nagata, 2011). Physiological Ca2+ concentrations are much higher in the ER than in the cytoplasm, which is achieved by sequestering of free Ca2+ and the coordinated action of the tissue-specific ATP-dependent Ca2+ pumps (SERCA2A and SERCA2B), ER membrane-localized inositol trisphosphate (InsP3R), and ryanodine (RyR) receptors (Schwarz and Blower, 2016; Almanza et al., 2019). Under physiological conditions, a sustained decrease of luminal ER Ca2+ levels upon Ca2+ release from the ER is prevented by store-operated calcium entry. This process is driven by oligomerization of stromal interaction molecule protein 1 and 2 (STIM1/STIM2) with the plasma-membrane localized calcium release-activated calcium channel protein 1 (ORAI1) and subsequent Ca2+ influx into the cell, followed by a SERCA-driven influx into the ER (Schwarz and Blower, 2016; Santulli et al., 2017). Thus, a depletion of the ER Ca2+ pool or inactivation of SERCA is associated with ER dysfunction and accumulation of unfolded/misfolded proteins (Sano and Reed, 2013).
Previous studies have reported that hypercapnia promotes elevation of intracellular Ca2+ levels in various cell types, thus causing different effects ranging from a decrease of the plasma membrane abundance of specific ion transporters to increased airway contractility (Vadasz et al., 2008; Cook et al., 2012; Turner et al., 2016; Shigemura et al., 2018). Interestingly, both short- and long-term hypercapnia modify intracellular Ca2+ levels, suggesting that several sources of the intracellular Ca2+ oscillations may exist. Previous reports have shown, that removal of Ca2+ from the extracellular medium, treatment with L- and T-type Ca2+ channel inhibitors or blocking SERCA activity by thapsigargin are not sufficient to prevent the elevation of intracellular Ca2+ concentrations upon hypercapnia, suggesting calcium mobilization from other stores (Nishio et al., 2001; Bouyer et al., 2003). In line with these findings, it was recently shown in alveolar epithelial cells and murine precision cut lung slices that the hypercapnia-induced increase in intracellular Ca2+ can be prevented by inhibition of InsP3R, indicating that elevated CO2 levels may enhance Ca2+ release from the ER (Kryvenko et al., 2021b). These results are also consistent with reports showing that ER-localized InsP3R receptors mediate Ca2+ release upon hypercapnia (Cook et al., 2012; Turner et al., 2016). Moreover, increased production of cAMP upon hypercapnia (Lecuona et al., 2013) may additionally stimulate protein kinase A and enhance subsequent release of calcium ions from the ER through InsP3R (Schmidt et al., 2008; Hofer, 2012). In a recent publication, a large-scale transcriptomic analysis of lung, muscle and respiratory cells exposed to hypercapnia revealed upregulation of canonical and non-canonical Wnt signaling pathways, including Fzd9, Wnt7a, Wnt4, and Wnt8b (Shigemura et al., 2019). The non-canonical Wnt/Ca2+ signaling cascade is tightly connected to the ER and plays an important role in the regulation of calcium release through InsP3R receptors and is linked to activities of calmodulin kinases activity and protein kinase C, which were previously found to be activated upon hypercapnia (Komiya and Habas, 2008; Vadasz et al., 2008). Interestingly, it has also been found that the Na,K-ATPase, a prominent target of hypercapnia, is involved in Ca2+ signaling as well by a direct interaction between the catalytic α-subunit of the Na,K-ATPase and InsP3R, thus modulating Ca2+ oscillations (Liu et al., 2008; Aperia et al., 2020). Thus, increasing evidence suggests that the ER is the primary source of increased intracellular Ca2+ upon hypercapnia and that enhanced release of Ca2+ from the ER may deplete the ER Ca2+ stores, which may impair the function of calcium-dependent chaperones, leading to compromised protein folding. These affects might be further aggravated by a marked downregulation of ATP-dependent transporters upon hypercapnia, including SERCA, thus impairing store-operated calcium entry mechanisms (Figure 2).
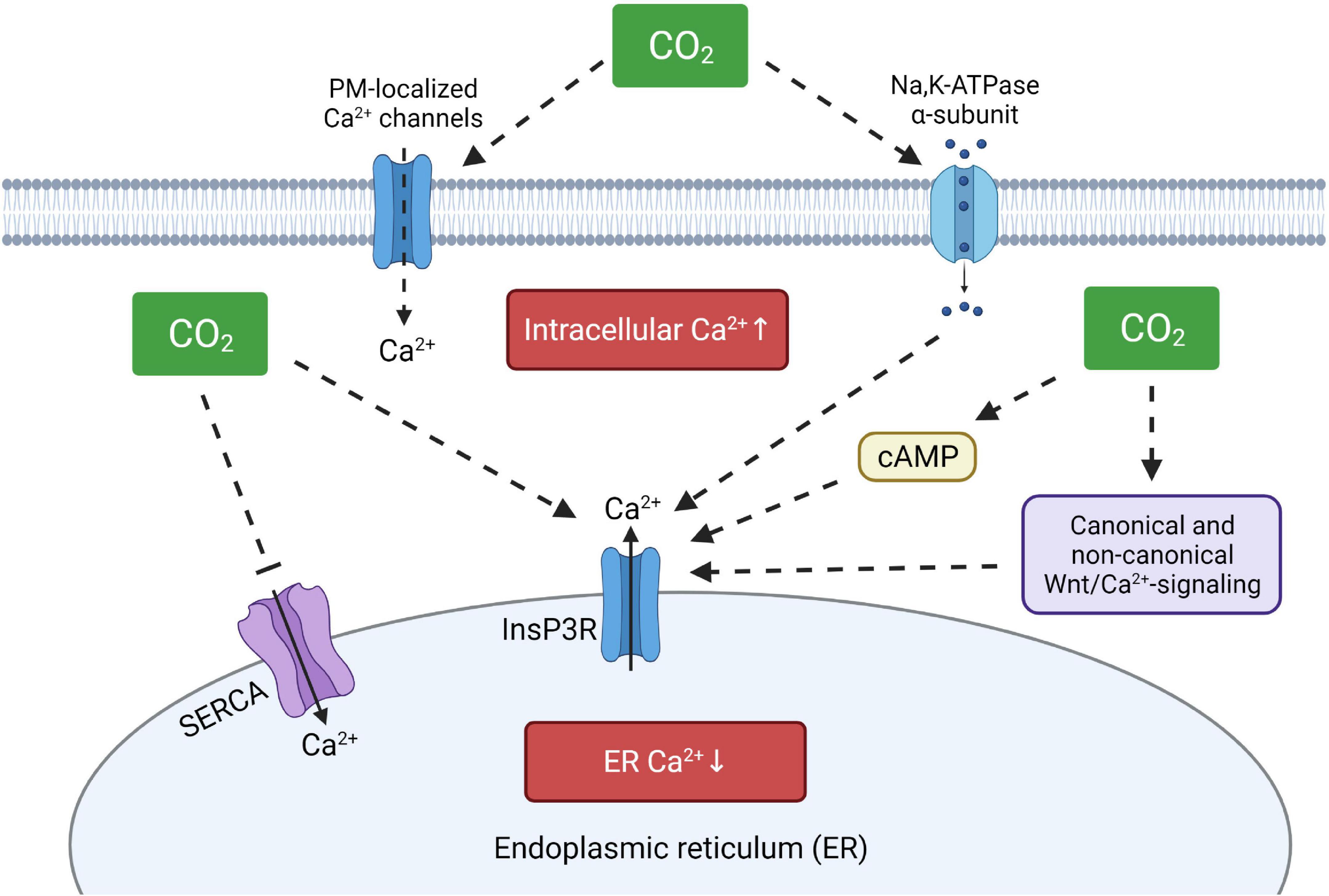
Figure 2. Proposed model of hypercapnia-induced intracellular Ca2+disturbances. Elevated CO2 levels may increase intracellular calcium concentrations by activation of plasma membrane-localized Ca2+ channels, by direct or indirect stimulation of ER-resident InsP3R and by modulating SERCA activity. ER, endoplasmic reticulum; InsP3R, ER membrane-localized inositol trisphosphate receptor; SERCA, sarco/endoplasmic reticulum Ca2+-ATPase, cAMP, cyclic adenosine monophosphate. Created with BioRender.com.
In addition, previous studies have reported that ER calcium and redox status are interconnected. Activity of RyR and SERCA2b are modified depending on the oxidative status of these molecules (Araki and Nagata, 2011). On the other hand, activation of InsP3R receptors and subsequent release of Ca2+ leads to a hyperoxidizing ER environment and apoptosis via CCAAT/enhancer-binding protein-homologous protein (CHOP) (Li et al., 2009). Thus, it may well be that a decrease in ATP production and increased ER protein oxidation upon elevated CO2 levels also contribute to alterations in ER Ca2+ homeostasis upon hypercapnia.
Hypercapnia, Endoplasmic Reticulum Stress and Adaptive vs. Maladaptive Unfolded Protein Response
A consequence of protein misfolding/unfolding in the ER, is ER stress and subsequent activation of IRE1α-, PERK-, and ATF6-mediated UPR pathways. The UPR response may be adaptive or maladaptive, depending on the markedness and duration of the stimulus (Wang and Kaufman, 2016). The adaptive mechanisms “aim” to restore the protein folding homeostasis in the ER by downregulating protein synthesis, activating ERAD and modulating function of specific ER chaperones. If the initial UPR response does not allow coping with ER stress, the maladaptive arm of UPR will be activated that may lead to cellular death, mostly via apoptosis (Wang and Kaufman, 2016).
A numbers of studies have shown that physiological ER stressors selectively activate UPR branches, thereby triggering non-classical stress responses within the ER, which do not lead to cellular death and have rather adaptive character (Raina et al., 2014; Bergmann et al., 2018). In line with this notion, we now know that exposure of alveolar epithelial cells to hypercapnia transiently activates IRE1α and induces ERAD of the ER-resident β-subunit of the Na,K-ATPase, thereby decreasing plasma membrane abundance of the transporter (Kryvenko et al., 2021b). Furthermore, enhanced protein degradation in the ER by ERAD is associated with increased ubiquitination of the target protein, which has been shown to occur upon hypercapnia (Gwozdzinska et al., 2017). Of note, a recent study identified the IRE1α interacting partner, TNF receptor-associated factor 2 (TRAF2), as a novel E3-ligase involved in the polyubiquitination of the Na,K-ATPase β-subunit (Gabrielli et al., 2021). However, whether TRAF2 is additionally required for ERAD of the Na,K-ATPase will need to be addressed in future studies. Interestingly, treatment of cells with CO2 levels of up to 120 mmHg for a duration of 5 days is not associated with increased apoptosis or cellular death in alveolar epithelial or mesenchymal cells (Vohwinkel et al., 2011), suggesting that at least in these settings of hypercapnia a rather adaptive type of UPR is activated.
It is well documented that elevated CO2 levels initiate specific signaling cascades in cells, including activation of ERK1/2, JNK, and AMPK-α1 that drive retrieval of the Na,K-ATPase and epithelial sodium channel (ENaC) from the plasma membrane, thereby causing alveolar epithelial barrier dysfunction and altering alveolar fluid balance (Vadasz et al., 2008; Welch et al., 2010; Gwozdzinska et al., 2017). Moreover, exposure of skeletal muscles to increased CO2 concentrations leads to stimulation of AMPK-α2 and is associated with a decrease in protein synthesis and increased muscles catabolism (Jaitovich et al., 2015; Ceco et al., 2017; Korponay et al., 2019). In general, AMPK activation is a response to metabolic stress by sensing AMP:ATP and ADP:ATP ratios, aiming to reestablish energy balance by reducing anabolic processes that require ATP and by promoting catabolic mechanisms that generate ATP (Garcia and Shaw, 2017). In contrast, in the setting of short-term hypercapnia, AMPK activation is independent of the metabolic status of the cell and is rather secondary to intracellular Ca2+ signaling (Vadasz et al., 2008). Notably, knockdown of AMPK in bronchial epithelial cells leads to a significant increase in CHOP levels resulting in ER stress and apoptosis (Liu et al., 2018). Moreover, AMPK activation downregulates BiP levels induced by tunicamycin or thapsigargin and has been found to regulate ER and mitochondrial morphology upon stress conditions, thus preventing mitochondrial fragmentation and apoptosis (Wikstrom et al., 2013; Kim et al., 2015).
Extracellular signal-regulated kinase, a member of the mitogen-activated protein kinase (MAPK) family, has been shown to play an essential role in UPR by interacting with IRE1α and by promoting transcription of pro-survival anti-apoptotic proteins, such as myeloid leukemia cell differentiation protein-1 (Mcl-1), Bcl-2 and B-cell lymphoma-extra large protein (Bcl-xL) (Darling and Cook, 2014). Furthermore, activation of ERK1/2 has been shown to be cytoprotective upon ER stress, by downregulating cellular apoptosis upon thapsigargin- and tunicamycin-induced UPR (Arai et al., 2004; Hu et al., 2004).
In addition, several other mechanisms may contribute to the adaptive or maladaptive signals upon hypercapnia. For example, hypercapnia has been found to inhibit autophagy in human macrophages by increasing expression of Bcl-2 and Bcl-xL, thus blocking Beclin-1 apoptotic complex formation (Casalino-Matsuda et al., 2015). Of note, Bcl-2 is involved in the regulation of ER calcium homeostasis und upregulation of the molecule may play a protective role upon ER stress by lowering steady-state levels of ER Ca2+ via InsP3R activation (Sano and Reed, 2013). However, the anti-apoptotic effects of Bcl-2 are inhibited by JNK (Sano and Reed, 2013) that is markedly upregulated in the setting of acute and chronic hypercapnia (Vadasz et al., 2012a; Dada et al., 2015; Gwozdzinska et al., 2017). In fact, the role of activated JNK, in contrast to AMPK and ERK1/2, is usually associated with an enhanced pro-apoptotic ER stress response. On the other hand, JNK is involved in the downstream cascade of IRE1α activation, the UPR branch responsible for preventing ER overload by ERAD (Maurel et al., 2014; Prischi et al., 2014; Almanza et al., 2019). Recently, hypercapnia has been associated with increased airway smooth muscle contractility in the setting of asthma, which is mediated by activation of caspase-7, an apoptosis-related cysteine peptidase (Shigemura et al., 2018). Interestingly, caspase-7 has also been found to be involved in the ER-stress mediated cell death upon thapsigargin treatment and caspase-7 ablation was able to reprogram the UPR and reduced JNK-induced apoptosis (Dahmer, 2005; Choudhury et al., 2013).
Thus, while activation of AMPK, ERK1/2, JNK, and caspase-7 drive clearly deleterious (maladaptive) signals leading to cellular dysfunction upon hypercapnia, activation of these signaling molecules may, at least in part, limit further injury by reducing the elevated CO2-induced ER stress, as part of an adaptive mechanism.
Conclusion
Protein maturation and folding in the ER require a specific milieu, which depends on Ca2+, ATP and an oxidative environment. Recent studies focusing on the pathophysiological effects of hypercapnia established that elevated CO2 levels alter the ER folding machinery. The molecular mechanisms driving ER dysfunction upon high CO2 concentrations include reduced cellular ATP levels, a Ca2+ disbalance in the ER, as well as altered redox homeostasis of the organelle (Figure 3). These events lead to ER stress, UPR, and ERAD of target proteins, potentially resulting in tissue and organ malfunction. To what extent these signals are adaptive or maladaptive depend on the extent and duration of hypercapnia and require further experimental assessment.
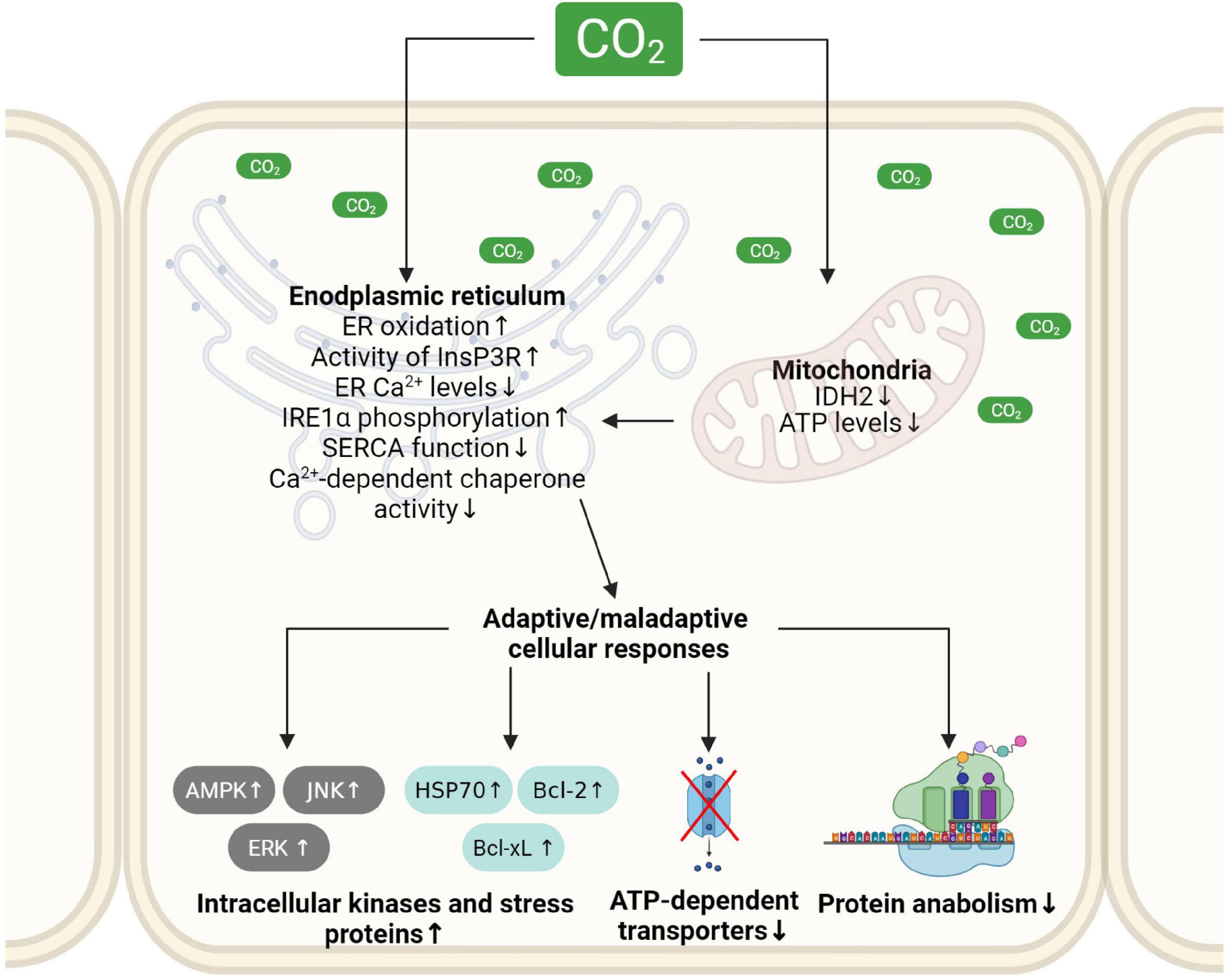
Figure 3. Schematic depiction of molecular mechanisms driving hypercapnia-induced ER dysfunction and subsequent adaptive and maladaptive cellular responses. Elevated CO2 levels reduce cellular ATP production, impair the oxidizing environment and alter calcium levels in the ER. These alterations in ER homeostasis cause ER stress and initiate adaptive and maladaptive cellular responses. ER, endoplasmic reticulum; IDH2, isocitrate dehydrogenase 2; InsP3R, ER membrane-localized inositol trisphosphate receptor; JNK, c-Jun N-terminal kinase; ERK1, extracellular signal-regulated kinase; AMPK, AMP-activated protein kinase; Bcl2, B-cell lymphoma 2 protein; Bcl-xL, B-cell lymphoma-extra large protein; HSP70, heat shock protein 70. Created with BioRender.com.
Author Contributions
VK and IV drafted, edited, and approved final version of the manuscript.
Funding
This work was supported by grants from the Federal Ministry of Education and Research [German Center for Lung Research (DZL/ALI 1.5 and 3.4)], the Hessen State Ministry of Higher Education, Research and the Arts [Landes-Offensive zur Entwicklung Wissenschaftlich-ökonomischer Exzellenz (LOEWE)], the von Behring Röntgen Foundation (Project 66-LV07), the German Research Foundation (DFG/KFO309, P5; The Cardio-Pulmonary Institute) (EXC 2026; Project ID: 390649896) (to IV), and an MD/Ph.D. start-up grant (DFG/KFO309, MD/Ph.D.) (to VK).
Conflict of Interest
The authors declare that the research was conducted in the absence of any commercial or financial relationships that could be construed as a potential conflict of interest.
Publisher’s Note
All claims expressed in this article are solely those of the authors and do not necessarily represent those of their affiliated organizations, or those of the publisher, the editors and the reviewers. Any product that may be evaluated in this article, or claim that may be made by its manufacturer, is not guaranteed or endorsed by the publisher.
References
Almanza, A., Carlesso, A., Chintha, C., Creedican, S., Doultsinos, D., Leuzzi, B., et al. (2019). Endoplasmic reticulum stress signalling - from basic mechanisms to clinical applications. FEBS J. 286, 241–278. doi: 10.1111/febs.14608
Andreini, C., Putignano, V., Rosato, A., and Banci, L. (2018). The human iron-proteome. Metallomics 10, 1223–1231. doi: 10.1039/c8mt00146d
Aperia, A., Brismar, H., and Uhlen, P. (2020). Mending Fences: Na,K-ATPase signaling via Ca(2+) in the maintenance of epithelium integrity. Cell Calcium 88:102210. doi: 10.1016/j.ceca.2020.102210
Arai, K., Lee, S. R., van Leyen, K., Kurose, H., and Lo, E. H. (2004). Involvement of ERK MAP kinase in endoplasmic reticulum stress in SH-SY5Y human neuroblastoma cells. J. Neurochem. 89, 232–239. doi: 10.1111/j.1471-4159.2004.02317.x
Araki, K., and Nagata, K. (2011). Protein folding and quality control in the ER. Cold Spring Harb. Perspect. Biol. 3:a007526. doi: 10.1101/cshperspect.a007526
Bagur, R., and Hajnoczky, G. (2017). Intracellular Ca(2+) sensing: its role in calcium homeostasis and signaling. Mol. Cell 66, 780–788. doi: 10.1016/j.molcel.2017.05.028
Banhegyi, G., Margittai, E., Szarka, A., Mandl, J., and Csala, M. (2012). Crosstalk and barriers between the electron carriers of the endoplasmic reticulum. Antioxid Redox Signal. 16, 772–780. doi: 10.1089/ars.2011.4437
Bergmann, T. J., Fregno, I., Fumagalli, F., Rinaldi, A., Bertoni, F., Boersema, P. J., et al. (2018). Chemical stresses fail to mimic the unfolded protein response resulting from luminal load with unfolded polypeptides. J. Biol. Chem. 293, 5600–5612. doi: 10.1074/jbc.RA117.001484
Bergmann, T. J., and Molinari, M. (2018). Three branches to rule them all? UPR signalling in response to chemically versus misfolded proteins-induced ER stress. Biol. Cell 110, 197–204. doi: 10.1111/boc.201800029
Bouyer, P., Zhou, Y., and Boron, W. F. (2003). An increase in intracellular calcium concentration that is induced by basolateral CO2 in rabbit renal proximal tubule. Am. J. Physiol. Renal. Physiol. 285, F674–F687. doi: 10.1152/ajprenal.00107.2003
Bradley, K. L., Stokes, C. A., Marciniak, S. J., Parker, L. C., and Condliffe, A. M. (2021). Role of unfolded proteins in lung disease. Thorax 76, 92–99. doi: 10.1136/thoraxjnl-2019-213738
Bravo, R., Parra, V., Gatica, D., Rodriguez, A. E., Torrealba, N., Paredes, F., et al. (2013). Endoplasmic reticulum and the unfolded protein response: dynamics and metabolic integration. Int. Rev. Cell Mol. Biol. 301, 215–290. doi: 10.1016/B978-0-12-407704-1.00005-1
Brodsky, J. L., and Skach, W. R. (2011). Protein folding and quality control in the endoplasmic reticulum: recent lessons from yeast and mammalian cell systems. Curr. Opin. Cell Biol. 23, 464–475. doi: 10.1016/j.ceb.2011.05.004
Burman, A., Kropski, J. A., Calvi, C. L., Serezani, A. P., Pascoalino, B. D., Han, W., et al. (2018). Localized hypoxia links ER stress to lung fibrosis through induction of C/EBP homologous protein. JCI Insight 3:e99543. doi: 10.1172/jci.insight.99543
Casalino-Matsuda, S. M., Chen, F., Gonzalez-Gonzalez, F. J., Nair, A., Dib, S., Yemelyanov, A., et al. (2020). Hypercapnia suppresses macrophage antiviral activity and increases mortality of influenza A infection via Akt1. J. Immunol. 205, 489–501. doi: 10.4049/jimmunol.2000085
Casalino-Matsuda, S. M., Nair, A., Beitel, G. J., Gates, K. L., and Sporn, P. H. (2015). Hypercapnia inhibits autophagy and bacterial killing in human macrophages by increasing expression of Bcl-2 and Bcl-xL. J. Immunol. 194, 5388–5396. doi: 10.4049/jimmunol.1500150
Casalino-Matsuda, S. M., Wang, N., Ruhoff, P. T., Matsuda, H., Nlend, M. C., Nair, A., et al. (2018). Hypercapnia alters expression of immune response, nucleosome assembly and lipid metabolism genes in differentiated human bronchial epithelial cells. Sci. Rep. 8:13508. doi: 10.1038/s41598-018-32008-x
Ceco, E., Weinberg, S. E., Chandel, N. S., and Sznajder, J. I. (2017). Metabolism and skeletal muscle homeostasis in lung disease. Am. J. Respir Cell Mol. Biol. 57, 28–34. doi: 10.1165/rcmb.2016-0355TR
Chipurupalli, S., Kannan, E., Tergaonkar, V., D’Andrea, R., and Robinson, N. (2019). Hypoxia induced ER stress response as an adaptive mechanism in Cancer. Int. J. Mol. Sci. 20:749. doi: 10.3390/ijms20030749
Choudhury, S., Bhootada, Y., Gorbatyuk, O., and Gorbatyuk, M. (2013). Caspase-7 ablation modulates UPR, reprograms TRAF2-JNK apoptosis and protects T17M rhodopsin mice from severe retinal degeneration. Cell Death Dis. 4:e528. doi: 10.1038/cddis.2013.34
Cook, Z. C., Gray, M. A., and Cann, M. J. (2012). Elevated carbon dioxide blunts mammalian cAMP signaling dependent on inositol 1,4,5-triphosphate receptor-mediated Ca2+ release. J. Biol. Chem. 287, 26291–26301. doi: 10.1074/jbc.M112.349191
Cummins, E. P., Strowitzki, M. J., and Taylor, C. T. (2019). Mechanisms and consequences of oxygen- and carbon dioxide-sensing in mammals. Physiol. Rev. 100, 463–488. doi: 10.1152/physrev.00003.2019
Dada, L. A., Trejo Bittar, H. E., Welch, L. C., Vagin, O., Deiss-Yehiely, N., Kelly, A. M., et al. (2015). High CO2 Leads to Na,K-ATPase endocytosis via c-jun amino-terminal kinase-induced LMO7b phosphorylation. Mol. Cell Biol. 35, 3962–3973. doi: 10.1128/MCB.00813-15
Dahmer, M. K. (2005). Caspases-2, -3, and -7 are involved in thapsigargin-induced apoptosis of SH-SY5Y neuroblastoma cells. J. Neurosci. Res. 80, 576–583. doi: 10.1002/jnr.20471
Dalle-Donne, I., Aldini, G., Carini, M., Colombo, R., Rossi, R., and Milzani, A. (2006). Protein carbonylation, cellular dysfunction, and disease progression. J. Cell Mol. Med. 10, 389–406. doi: 10.1111/j.1582-4934.2006.tb00407.x
Darling, N. J., and Cook, S. J. (2014). The role of MAPK signalling pathways in the response to endoplasmic reticulum stress. Biochim. Biophys. Acta 1843, 2150–2163. doi: 10.1016/j.bbamcr.2014.01.009
Delbrel, E., Soumare, A., Naguez, A., Label, R., Bernard, O., Bruhat, A., et al. (2018). HIF-1alpha triggers ER stress and CHOP-mediated apoptosis in alveolar epithelial cells, a key event in pulmonary fibrosis. Sci. Rep. 8:17939. doi: 10.1038/s41598-018-36063-2
Delbrel, E., Uzunhan, Y., Soumare, A., Gille, T., Marchant, D., Planes, C., et al. (2019). ER stress is involved in Epithelial-To-Mesenchymal transition of alveolar epithelial cells exposed to a hypoxic microenvironment. Int. J. Mol. Sci. 20:1299. doi: 10.3390/ijms20061299
Depaoli, M. R., Hay, J. C., Graier, W. F., and Malli, R. (2019). The enigmatic ATP supply of the endoplasmic reticulum. Biol. Rev. Camb. Philos. Soc. 94, 610–628. doi: 10.1111/brv.12469
Diaz-Bulnes, P., Saiz, M. L., Lopez-Larrea, C., and Rodriguez, R. M. (2019). Crosstalk between hypoxia and er stress response: a key regulator of macrophage polarization. Front. Immunol. 10:2951. doi: 10.3389/fimmu.2019.02951
Dimitrov, L., Lam, S. K., and Schekman, R. (2013). The role of the endoplasmic reticulum in peroxisome biogenesis. Cold Spring Harb. Perspect. Biol. 5:a013243. doi: 10.1101/cshperspect.a013243
Ellgaard, L., and Helenius, A. (2003). Quality control in the endoplasmic reticulum. Nat. Rev. Mol. Cell Biol. 4, 181–191. doi: 10.1038/nrm1052
Ellgaard, L., Sevier, C. S., and Bulleid, N. J. (2018). How are proteins reduced in the endoplasmic reticulum? Trends Biochem. Sci. 43, 32–43. doi: 10.1016/j.tibs.2017.10.006
England, K., and Cotter, T. (2004). Identification of carbonylated proteins by MALDI-TOF mass spectroscopy reveals susceptibility of ER. Biochem. Biophys. Res. Commun. 320, 123–130. doi: 10.1016/j.bbrc.2004.05.144
Fan, Y., and Simmen, T. (2019). Mechanistic connections between endoplasmic reticulum (ER) Redox control and mitochondrial metabolism. Cells 8:1071. doi: 10.3390/cells8091071
Fergie, N., Todd, N., McClements, L., McAuley, D., O’Kane, C., and Krasnodembskaya, A. (2019). Hypercapnic acidosis induces mitochondrial dysfunction and impairs the ability of mesenchymal stem cells to promote distal lung epithelial repair. FASEB J. 33, 5585–5598. doi: 10.1096/fj.201802056R
Fuller, B. M., Mohr, N. M., Drewry, A. M., Ferguson, I. T., Trzeciak, S., Kollef, M. H., et al. (2017). Partial pressure of arterial carbon dioxide and survival to hospital discharge among patients requiring acute mechanical ventilation: a cohort study. J. Crit Care 41, 29–35. doi: 10.1016/j.jcrc.2017.04.033
Gabrielli, N. M., Mazzocchi, L. C., Kryvenko, V., Tello, K., Herold, S., Morty, R. E., et al. (2021). TRAF2 is a novel ubiquitin E3 ligase for the Na,K-ATPase β-subunit that drives alveolar epithelial barrier dysfunction in hypercapnia. Front. Cell Dev. Biol. 9:689983. doi: 10.3389/fcell.2021.689983
Garcia, D., and Shaw, R. J. (2017). AMPK: mechanisms of cellular energy sensing and restoration of metabolic balance. Mol. Cell 66, 789–800. doi: 10.1016/j.molcel.2017.05.032
Gates, K. L., Howell, H. A., Nair, A., Vohwinkel, C. U., Welch, L. C., Beitel, G. J., et al. (2013). Hypercapnia impairs lung neutrophil function and increases mortality in murine pseudomonas pneumonia. Am. J. Respir. Cell Mol. Biol. 49, 821–828. doi: 10.1165/rcmb.2012-0487OC
Gwozdzinska, P., Buchbinder, B. A., Mayer, K., Herold, S., Morty, R. E., Seeger, W., et al. (2017). Hypercapnia Impairs ENaC Cell Surface Stability by Promoting Phosphorylation, Polyubiquitination and Endocytosis of beta-ENaC in a Human Alveolar Epithelial Cell Line. Front. Immunol. 8:591. doi: 10.3389/fimmu.2017.00591
Halperin, L., Jung, J., and Michalak, M. (2014). The many functions of the endoplasmic reticulum chaperones and folding enzymes. IUBMB Life 66, 318–326. doi: 10.1002/iub.1272
Hebert, D. N., and Molinari, M. (2007). In and out of the ER: protein folding, quality control, degradation, and related human diseases. Physiol. Rev. 87, 1377–1408. doi: 10.1152/physrev.00050.2006
Hedison, T. M., and Scrutton, N. S. (2019). Tripping the light fantastic in membrane redox biology: linking dynamic structures to function in ER electron transfer chains. FEBS J. 286, 2004–2017. doi: 10.1111/febs.14757
Herold, S., Gabrielli, N. M., and Vadasz, I. (2013). Novel concepts of acute lung injury and alveolar-capillary barrier dysfunction. Am. J. Physiol. Lung Cell Mol. Physiol. 305, L665–L681. doi: 10.1152/ajplung.00232.2013
Hetz, C., Chevet, E., and Oakes, S. A. (2015). Proteostasis control by the unfolded protein response. Nat. Cell Biol. 17, 829–838. doi: 10.1038/ncb3184
Hofer, A. M. (2012). Interactions between calcium and cAMP signaling. Curr. Med. Chem. 19, 5768–5773. doi: 10.2174/092986712804143286
Hu, P., Han, Z., Couvillon, A. D., and Exton, J. H. (2004). Critical role of endogenous Akt/IAPs and MEK1/ERK pathways in counteracting endoplasmic reticulum stress-induced cell death. J. Biol. Chem. 279, 49420–49429. doi: 10.1074/jbc.M407700200
Huang, S., Jin, L., Shen, J., Shang, P., Jiang, X., and Wang, X. (2016). Electrical stimulation influences chronic intermittent hypoxia-hypercapnia induction of muscle fibre transformation by regulating the microRNA/Sox6 pathway. Sci. Rep. 6:26415. doi: 10.1038/srep26415
Husain-Syed, F., Birk, H. W., Wilhelm, J., Ronco, C., Ranieri, V. M., Karle, B., et al. (2020). Extracorporeal carbon dioxide removal using a renal replacement therapy platform to enhance lung-protective ventilation in hypercapnic patients with Coronavirus Disease 2019-associated acute respiratory distress syndrome. Front. Med. 7:598379. doi: 10.3389/fmed.2020.598379
Imamura, T., Xue, J., Poulsen, O., Zhou, D., Karin, M., and Haddad, G. G. (2019). Intermittent hypoxia and hypercapnia induces inhibitor of nuclear factor-kappaB kinase subunit beta-dependent atherosclerosis in pulmonary arteries. Am. J. Physiol. Regul. Integr. Comp. Physiol. 317, R763–R769. doi: 10.1152/ajpregu.00056.2019
Jager, R., Bertrand, M. J., Gorman, A. M., Vandenabeele, P., and Samali, A. (2012). The unfolded protein response at the crossroads of cellular life and death during endoplasmic reticulum stress. Biol. Cell 104, 259–270. doi: 10.1111/boc.201100055
Jaitovich, A., Angulo, M., Lecuona, E., Dada, L. A., Welch, L. C., Cheng, Y., et al. (2015). High CO2 levels cause skeletal muscle atrophy via AMP-activated kinase (AMPK), FoxO3a protein, and muscle-specific Ring finger protein 1 (MuRF1). J. Biol. Chem. 290, 9183–9194. doi: 10.1074/jbc.M114.625715
Kenche, H., Baty, C. J., Vedagiri, K., Shapiro, S. D., and Blumental-Perry, A. (2013). Cigarette smoking affects oxidative protein folding in endoplasmic reticulum by modifying protein disulfide isomerase. FASEB J. 27, 965–977. doi: 10.1096/fj.12-216234
Kikuchi, R., Tsuji, T., Watanabe, O., Yamaguchi, K., Furukawa, K., Nakamura, H., et al. (2017). Hypercapnia accelerates adipogenesis: a novel role of high CO2 in exacerbating obesity. Am. J. Respir. Cell Mol. Biol. 57, 570–580. doi: 10.1165/rcmb.2016-0278OC
Kim, H., Moon, S. Y., Kim, J. S., Baek, C. H., Kim, M., Min, J. Y., et al. (2015). Activation of AMP-activated protein kinase inhibits ER stress and renal fibrosis. Am. J. Physiol. Renal. Physiol. 308, F226–F236. doi: 10.1152/ajprenal.00495.2014
Kim, S., Kim, C. M., Son, Y. J., Choi, J. Y., Siegenthaler, R. K., Lee, Y., et al. (2018). Molecular basis of maintaining an oxidizing environment under anaerobiosis by soluble fumarate reductase. Nat. Commun. 9:4867. doi: 10.1038/s41467-018-07285-9
Kim, S. R., Kim, D. I., Kang, M. R., Lee, K. S., Park, S. Y., Jeong, J. S., et al. (2013). Endoplasmic reticulum stress influences bronchial asthma pathogenesis by modulating nuclear factor kappaB activation. J. Allergy Clin. Immunol. 132, 1397–1408. doi: 10.1016/j.jaci.2013.08.041
Komiya, Y., and Habas, R. (2008). Wnt signal transduction pathways. Organogenesis 4, 68–75. doi: 10.4161/org.4.2.5851
Korfei, M., Ruppert, C., Mahavadi, P., Henneke, I., Markart, P., Koch, M., et al. (2008). Epithelial endoplasmic reticulum stress and apoptosis in sporadic idiopathic pulmonary fibrosis. Am. J. Respir. Crit. Care Med. 178, 838–846. doi: 10.1164/rccm.200802-313OC
Korponay, T. C., Balnis, J., Vincent, C. E., Singer, D. V., Chopra, A., Adam, A. P., et al. (2019). High CO2 downregulates skeletal muscle protein anabolism via AMPKalpha2-mediated depressed ribosomal biogenesis. Am. J. Respir. Cell Mol. Biol. 62, 74–86. doi: 10.1165/rcmb.2019-0061OC
Krebs, J., Agellon, L. B., and Michalak, M. (2015). Ca(2+) homeostasis and endoplasmic reticulum (ER) stress: an integrated view of calcium signaling. Biochem. Biophys. Res. Commun. 460, 114–121. doi: 10.1016/j.bbrc.2015.02.004
Kryvenko, V., and Vadasz, I. (2021). Molecular mechanisms of Na,K-ATPase dysregulation driving alveolar epithelial barrier failure in severe COVID-19. Am. J. Physiol. Lung. Cell Mol. Physiol. 320, L1186–L1193. doi: 10.1152/ajplung.00056.2021
Kryvenko, V., Vagin, O., Dada, L. A., Sznajder, J. I., and Vadasz, I. (2021a). Maturation of the Na,K-ATPase in the endoplasmic reticulum in health and disease. J. Membr. Biol.1–11. doi: 10.1007/s00232-021-00184-z [Epub ahead of print].
Kryvenko, V., Wessendorf, M., Tello, K., Herold, S., Morty, R. E., Seeger, W., et al. (2021b). Hypercapnia induces IRE1alpha-driven endoplasmic reticulum-associated degradation of the Na,K-ATPase beta-subunit. Am. J. Respir. Cell Mol. Biol. doi: 10.1165/rcmb.2021-0114OC [Epub ahead of print].
Kryvenko, V., Wessendorf, M., Morty, R. E., Herold, S., Seeger, W., Vagin, O., et al. (2020). Hypercapnia Impairs Na,K-ATPase function by inducing endoplasmic reticulum retention of the beta-subunit of the enzyme in alveolar epithelial cells. Int. J. Mol. Sci. 21:1467. doi: 10.3390/ijms21041467
Lang, J. D. Jr., Chumley, P., Eiserich, J. P., Estevez, A., Bamberg, T., Adhami, A., et al. (2000). Hypercapnia induces injury to alveolar epithelial cells via a nitric oxide-dependent pathway. Am. J. Physiol. Lung. Cell Mol. Physiol. 279, L994–L1002. doi: 10.1152/ajplung.2000.279.5.L994
Lawson, W. E., Cheng, D. S., Degryse, A. L., Tanjore, H., Polosukhin, V. V., Xu, X. C., et al. (2011). Endoplasmic reticulum stress enhances fibrotic remodeling in the lungs. Proc. Natl. Acad. Sci. U.S.A. 108, 10562–10567. doi: 10.1073/pnas.1107559108
Lecuona, E., Sun, H., Chen, J., Trejo, H. E., Baker, M. A., and Sznajder, J. I. (2013). Protein kinase A-Ialpha regulates Na,K-ATPase endocytosis in alveolar epithelial cells exposed to high CO(2) concentrations. Am. J. Respir. Cell Mol. Biol. 48, 626–634. doi: 10.1165/rcmb.2012-0373OC
Lee, K. S., Jeong, J. S., Kim, S. R., Cho, S. H., Kolliputi, N., Ko, Y. H., et al. (2016). Phosphoinositide 3-kinase-delta regulates fungus-induced allergic lung inflammation through endoplasmic reticulum stress. Thorax 71, 52–63. doi: 10.1136/thoraxjnl-2015-207096
Li, G., Mongillo, M., Chin, K. T., Harding, H., Ron, D., Marks, A. R., et al. (2009). Role of ERO1-alpha-mediated stimulation of inositol 1,4,5-triphosphate receptor activity in endoplasmic reticulum stress-induced apoptosis. J. Cell Biol. 186, 783–792. doi: 10.1083/jcb.200904060
Linthwaite, V. L., Janus, J. M., Brown, A. P., Wong-Pascua, D., O’Donoghue, A. C., Porter, A., et al. (2018). The identification of carbon dioxide mediated protein post-translational modifications. Nat. Commun. 9:3092. doi: 10.1038/s41467-018-05475-z
Liu, J. Q., Zhang, L., Yao, J., Yao, S., and Yuan, T. (2018). AMPK alleviates endoplasmic reticulum stress by inducing the ER-chaperone ORP150 via FOXO1 to protect human bronchial cells from apoptosis. Biochem. Biophys. Res. Commun. 497, 564–570. doi: 10.1016/j.bbrc.2018.02.095
Liu, X., Spicarova, Z., Rydholm, S., Li, J., Brismar, H., and Aperia, A. (2008). Ankyrin B modulates the function of Na,K-ATPase/inositol 1,4,5-trisphosphate receptor signaling microdomain. J. Biol. Chem. 283, 11461–11468. doi: 10.1074/jbc.M706942200
Marciniak, S. J. (2017). Endoplasmic reticulum stress in lung disease. Eur. Respir. Rev. 26:170018. doi: 10.1183/16000617.0018-2017
Maurel, M., Chevet, E., Tavernier, J., and Gerlo, S. (2014). Getting RIDD of RNA: IRE1 in cell fate regulation. Trends Biochem. Sci. 39, 245–254. doi: 10.1016/j.tibs.2014.02.008
Meigh, L., Greenhalgh, S. A., Rodgers, T. L., Cann, M. J., Roper, D. I., and Dale, N. (2013). CO(2)directly modulates connexin 26 by formation of carbamate bridges between subunits. eLife 2:e01213. doi: 10.7554/eLife.01213
Nin, N., Muriel, A., Penuelas, O., Brochard, L., Lorente, J. A., Ferguson, N. D., et al. (2017). Severe hypercapnia and outcome of mechanically ventilated patients with moderate or severe acute respiratory distress syndrome. Intensive Care Med. 43, 200–208. doi: 10.1007/s00134-016-4611-1
Nishio, K., Suzuki, Y., Takeshita, K., Aoki, T., Kudo, H., Sato, N., et al. (2001). Effects of hypercapnia and hypocapnia on [Ca2+]i mobilization in human pulmonary artery endothelial cells. J. Appl. Physiol. 90, 2094–2100. doi: 10.1152/jappl.2001.90.6.2094
Prischi, F., Nowak, P. R., Carrara, M., and Ali, M. M. (2014). Phosphoregulation of Ire1 RNase splicing activity. Nat. Commun. 5:3554. doi: 10.1038/ncomms4554
Putnam, R. W., Filosa, J. A., and Ritucci, N. A. (2004). Cellular mechanisms involved in CO(2) and acid signaling in chemosensitive neurons. Am. J. Physiol. Cell Physiol. 287, C1493–C1526. doi: 10.1152/ajpcell.00282.2004
Radermacher, P., Maggiore, S. M., and Mercat, A. (2017). Fifty years of research in ARDS. Gas exchange in acute respiratory distress syndrome. Am. J. Respir. Crit. Care Med. 196, 964–984. doi: 10.1164/rccm.201610-2156SO
Raina, K., Noblin, D. J., Serebrenik, Y. V., Adams, A., Zhao, C., and Crews, C. M. (2014). Targeted protein destabilization reveals an estrogen-mediated ER stress response. Nat. Chem. Biol. 10, 957–962. doi: 10.1038/nchembio.1638
Roberts, B. W., Mohr, N. M., Ablordeppey, E., Drewry, A. M., Ferguson, T. I., Trzeciak, S., et al. (2018). Association between partial pressure of arterial carbon dioxide and survival to hospital discharge among patients diagnosed with sepsis in the emergency department. Crit. Care Med. 46, e213–e220. doi: 10.1097/CCM.0000000000002918
Sala, A. J., Bott, L. C., and Morimoto, R. I. (2017). Shaping proteostasis at the cellular, tissue, and organismal level. J. Cell Biol. 216, 1231–1241. doi: 10.1083/jcb.201612111
Sano, R., and Reed, J. C. (2013). ER stress-induced cell death mechanisms. Biochim. Biophys. Acta 1833, 3460–3470. doi: 10.1016/j.bbamcr.2013.06.028
Santulli, G., Nakashima, R., Yuan, Q., and Marks, A. R. (2017). Intracellular calcium release channels: an update. J. Physiol. 595, 3041–3051. doi: 10.1113/JP272781
Schmidt, R., Baumann, O., and Walz, B. (2008). cAMP potentiates InsP3-induced Ca2+ release from the endoplasmic reticulum in blowfly salivary glands. BMC Physiol. 8:10. doi: 10.1186/1472-6793-8-10
Schmoldt, C., Vazquez-Armendariz, A. I., Shalashova, I., Selvakumar, B., Bremer, C. M., Peteranderl, C., et al. (2019). IRE1 signaling as a putative therapeutic target in influenza virus-induced pneumonia. Am. J. Respir. Cell Mol. Biol. 61, 537–540. doi: 10.1165/rcmb.2019-0123LE
Schwarz, D. S., and Blower, M. D. (2016). The endoplasmic reticulum: structure, function and response to cellular signaling. Cell Mol. Life Sci. 73, 79–94. doi: 10.1007/s00018-015-2052-6
Shigemura, M., Homma, T., and Sznajder, J. I. (2020). Hypercapnia: an aggravating factor in asthma. J. Clin. Med. 9:3207. doi: 10.3390/jcm9103207
Shigemura, M., Lecuona, E., Angulo, M., Dada, L. A., Edwards, M. B., Welch, L. C., et al. (2019). Elevated CO2 regulates the Wnt signaling pathway in mammals, Drosophila melanogaster and Caenorhabditis elegans. Sci. Rep. 9:18251. doi: 10.1038/s41598-019-54683-0
Shigemura, M., Lecuona, E., Angulo, M., Homma, T., Rodriguez, D. A., Gonzalez-Gonzalez, F. J., et al. (2018). Hypercapnia increases airway smooth muscle contractility via caspase-7-mediated miR-133a-RhoA signaling. Sci. Transl. Med. 10:eaat1662. doi: 10.1126/scitranslmed.aat1662
Shigemura, M., Lecuona, E., and Sznajder, J. I. (2017). Effects of hypercapnia on the lung. J. Physiol. 595, 2431–2437. doi: 10.1113/JP273781
Tang, A. C., Saferali, A., He, G., Sandford, A. J., Strug, L. J., and Turvey, S. E. (2017). Endoplasmic reticulum stress and chemokine production in cystic fibrosis airway cells: regulation by STAT3 modulation. J. Infect. Dis. 215, 293–302. doi: 10.1093/infdis/jiw516
Tatu, U., Braakman, I., and Helenius, A. (1993). Membrane glycoprotein folding, oligomerization and intracellular transport: effects of dithiothreitol in living cells. EMBO J. 12, 2151–2157.
Tu, B. P., Ho-Schleyer, S. C., Travers, K. J., and Weissman, J. S. (2000). Biochemical basis of oxidative protein folding in the endoplasmic reticulum. Science 290, 1571–1574. doi: 10.1126/science.290.5496.1571
Turner, M. J., Saint-Criq, V., Patel, W., Ibrahim, S. H., Verdon, B., Ward, C., et al. (2016). Hypercapnia modulates cAMP signalling and cystic fibrosis transmembrane conductance regulator-dependent anion and fluid secretion in airway epithelia. J. Physiol. 594, 1643–1661. doi: 10.1113/JP271309
Vadasz, I., Hubmayr, R. D., Nin, N., Sporn, P. H., and Sznajder, J. I. (2012b). Hypercapnia: a nonpermissive environment for the lung. Am. J. Respir. Cell Mol. Biol. 46, 417–421. doi: 10.1165/rcmb.2011-0395PS
Vadasz, I., Dada, L. A., Briva, A., Helenius, I. T., Sharabi, K., Welch, L. C., et al. (2012a). Evolutionary conserved role of c-Jun-N-terminal kinase in CO2-induced epithelial dysfunction. PLoS One 7:e46696. doi: 10.1371/journal.pone.0046696
Vadasz, I., Dada, L. A., Briva, A., Trejo, H. E., Welch, L. C., Chen, J., et al. (2008). AMP-activated protein kinase regulates CO2-induced alveolar epithelial dysfunction in rats and human cells by promoting Na,K-ATPase endocytosis. J. Clin. Invest. 118, 752–762. doi: 10.1172/JCI29723
Vadasz, I., Raviv, S., and Sznajder, J. I. (2007). Alveolar epithelium and Na,K-ATPase in acute lung injury. Intensive Care Med. 33, 1243–1251. doi: 10.1007/s00134-007-0661-8
van ’t Wout, E. F., van Schadewijk, A., van Boxtel, R., Dalton, L. E., Clarke, H. J., Tommassen, J., et al. (2015). Virulence factors of pseudomonas aeruginosa induce both the unfolded protein and integrated stress responses in airway epithelial cells. PLoS Pathog. 11:e1004946. doi: 10.1371/journal.ppat.1004946
Vohwinkel, C. U., Lecuona, E., Sun, H., Sommer, N., Vadasz, I., Chandel, N. S., et al. (2011). Elevated CO(2) levels cause mitochondrial dysfunction and impair cell proliferation. J. Biol. Chem. 286, 37067–37076. doi: 10.1074/jbc.M111.290056
Wang, M., and Kaufman, R. J. (2016). Protein misfolding in the endoplasmic reticulum as a conduit to human disease. Nature 529, 326–335. doi: 10.1038/nature17041
Welch, L. C., Lecuona, E., Briva, A., Trejo, H. E., Dada, L. A., and Sznajder, J. I. (2010). Extracellular signal-regulated kinase (ERK) participates in the hypercapnia-induced Na,K-ATPase downregulation. FEBS Lett. 584, 3985–3989. doi: 10.1016/j.febslet.2010.08.002
Wieser, W., and Krumschnabel, G. (2001). Hierarchies of ATP-consuming processes: direct compared with indirect measurements, and comparative aspects. Biochem. J. 355(Pt 2), 389–395. doi: 10.1042/0264-6021:3550389
Wikstrom, J. D., Israeli, T., Bachar-Wikstrom, E., Swisa, A., Ariav, Y., Waiss, M., et al. (2013). AMPK regulates ER morphology and function in stressed pancreatic beta-cells via phosphorylation of DRP1. Mol. Endocrinol. 27, 1706–1723. doi: 10.1210/me.2013-1109
Xue, J., Allaband, C., Zhou, D., Poulsen, O., Martino, C., Jiang, L., et al. (2021). Influence of intermittent Hypoxia/Hypercapnia on atherosclerosis, gut microbiome, and metabolome. Front. Physiol. 12:663950. doi: 10.3389/fphys.2021.663950
Keywords: hypercapnia, carbon dioxide, endoplasmic reticulum, protein folding, unfolded protein response
Citation: Kryvenko V and Vadász I (2021) Mechanisms of Hypercapnia-Induced Endoplasmic Reticulum Dysfunction. Front. Physiol. 12:735580. doi: 10.3389/fphys.2021.735580
Received: 02 July 2021; Accepted: 27 October 2021;
Published: 19 November 2021.
Edited by:
Sabah Hussain, McGill University, CanadaReviewed by:
Jonathan E. Elliott, Oregon Health and Science University, United StatesSonali P. Barwe, Alfred I. duPont Hospital for Children, United States
Michael Chvanov, University of Liverpool, United Kingdom
Copyright © 2021 Kryvenko and Vadász. This is an open-access article distributed under the terms of the Creative Commons Attribution License (CC BY). The use, distribution or reproduction in other forums is permitted, provided the original author(s) and the copyright owner(s) are credited and that the original publication in this journal is cited, in accordance with accepted academic practice. No use, distribution or reproduction is permitted which does not comply with these terms.
*Correspondence: István Vadász, istvan.vadasz@innere.med.uni-giessen.de