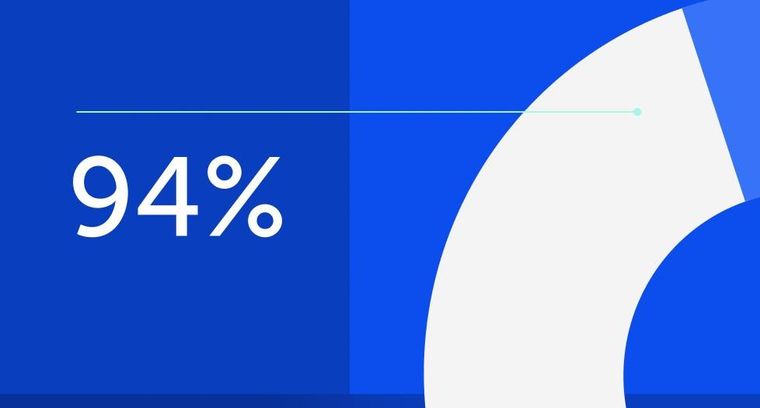
94% of researchers rate our articles as excellent or good
Learn more about the work of our research integrity team to safeguard the quality of each article we publish.
Find out more
ORIGINAL RESEARCH article
Front. Physiol., 29 September 2021
Sec. Environmental, Aviation and Space Physiology
Volume 12 - 2021 | https://doi.org/10.3389/fphys.2021.731633
This article is part of the Research TopicPhysiology and Physiopathology of Breath-Holding ActivityView all 18 articles
During breath holding after face immersion there develops an urge to breathe. The point that would initiate the termination of the breath hold, the “physiological breaking point,” is thought to be primarily due to changes in blood gases. However, we theorized that other factors, such as lung volume, also contributes significantly to terminating breath holds during face immersion. Accordingly, nine naïve subjects (controls) and seven underwater hockey players (divers) voluntarily initiated face immersions in room temperature water at Total Lung Capacity (TLC) and Functional Residual Capacity (FRC) after pre-breathing air, 100% O2, 15% O2 / 85% N2, or 5% CO2 / 95% O2. Heart rate (HR), arterial blood pressure (BP), end-tidal CO2 (etCO2), and breath hold durations (BHD) were monitored during all face immersions. The decrease in HR and increase in BP were not significantly different at the two lung volumes, although the increase in BP was usually greater at FRC. BHD was significantly longer at TLC (54 ± 2 s) than at FRC (30 ± 2 s). Also, with each pre-breathed gas BHD was always longer at TLC. We found no consistent etCO2 at which the breath holding terminated. BDHs were significantly longer in divers than in controls. We suggest that during breath holding with face immersion high lung volume acts directly within the brainstem to actively delay the attainment of the physiological breaking point, rather than acting indirectly as a sink to produce a slower build-up of PCO2.
The diving response, which is present in all mammals, including humans, includes a parasympathetically-mediated bradycardia that decreases cardiac output, a sympathetically-mediated increase in arterial blood pressure through selective restriction of blood flow to non-vital tissues, and apnea (for reviews see Gooden, 1994; Foster and Sheel, 2005; Lindholm and Lundgren, 2009; Castellini, 2012; Fitz-Clarke, 2018). Although voluntary breath holding in air can produce cardiovascular responses similar to that of the diving response, stimulation of trigeminal receptors on the face is necessary for full development of the diving response (Manley, 1990; Gooden, 1994; Andersson et al., 2000). The initiation of the diving response with immersion of the face into water, especially cold water, is well documented and is consistent with research in other animals and in humans with full body immersion (Kawakami et al., 1967; Moore et al., 1972; Fitz-Clarke, 2018).
The duration of a breath hold in either naïve subjects or experienced divers can be quite variable. Breath hold durations (BHDs) typically vary from 20 to 270 s (Lin, 1982), although voluntary breath holds during submergence reaching 11 min have been reported (Fitz-Clarke, 2018). After a voluntary inhibition of breathing and immersion of the face into water, a person can easily hold their breath, called the “easy-going” phase (see Lin, 1982). Throughout this time a physiological urge to breathe develops, primarily through changes in blood gases (i.e., increases in PaCO2 and/or decreases in PaO2). The point where these physiological parameters have built up to levels that initiate the termination of the breath hold is called the “physiological breaking point.” At the physiological breaking point there is an onset of involuntary ventilatory activity, such as an increase in diaphragmatic activity. Some subjects can continue to hold their breath past the physiological breaking point by consciously suppressing their urge to breathe, and overcoming the discomfort of hypoxia, hypercapnia, and acidosis, etc. (Fitz-Clarke, 2018). This phase is called the “struggle phase,” and may involve psychological and/or motivational factors that inhibit the termination of the breath hold. Strong involuntary breathing movements during the “struggle phase” may also increase brain blood flow (Dujic et al., 2009) and splenic contraction (Palada et al., 2008). The end of the struggle phase is the “conventional breaking point,” where the breath holding can no longer be continued, and the subject voluntarily terminates their breath hold, reemerges from the face submersion, and resumes normal ventilatory activity.
The physiological breaking point is sharply defined by chemical status (Lin, 1987, 1988). However, in addition to changes in blood gases, lung volume may also play a role in determining the duration of the easy-going phase. Both the easy going phase and total BHDs are longer when breath holding at total lung capacity (TLC) than at functional residual capacity (FRC) (Andersson and Schagatay, 1998). This may be because, with a larger lung volume, it could take longer for PaCO2 and PaO2 to reach levels that signal the physiological breaking point (Andersson and Schagatay, 1998), an effect perhaps aided through a potentiation of diving bradycardia (Manley, 1990). Alternatively, the level of lung inflation may provide afferent signals, through pulmonary stretch receptor activity, that could directly constitute a physiological signal that affects the onset of the physiological breaking point. Accordingly, we designed the present study to determine whether lung volume per se can affect the duration of the easy-going phase in either untrained or experienced divers (members of an underwater hockey team). Also, since chemical status can affect BHD, we had subjects pre-breath different gas mixtures to enhance or diminish chemoreceptor activity during the breath holds. We hypothesized that activation of pulmonary stretch receptors at large lung volumes act to prolong the attainment of the physiological breaking point.
This study was approved by the Midwestern University Institutional Review Board (IRB) prior to subject enrollment. All subjects gave informed consent and understood the procedures, risks, and voluntary nature of the study.
Subjects (ages 21–42) were recruited from Midwestern University in Downers Grove, IL (“controls”: N = 9; 4M, 5F) and a local underwater hockey club (“divers” N = 7; 4M, 3F). All subjects were healthy, non-smokers without apparent cardiovascular or respiratory disease and not taking any medication that would interfere with study parameters. In addition, subjects abstained from caffeine use on the day of testing. No adverse experiences were reported.
A total of eight trials were performed at two lung volumes—four at TLC and four at FRC. Before each breath hold trial, subjects were asked to breathe one of the following four gas mixtures to alter chemoreceptor activity. Standard Air (21% O2) was the control gas; 100% O2 was chosen to decrease peripheral chemoreceptor activity; 15% O2 / 85% N2 was chosen to increase peripheral chemoreceptor activity; and 5% CO2 / 95% O2 was chosen to increase central chemoreceptor activity and decrease peripheral chemoreceptor activity. The assignment of each gas was random, predetermined, and conducted in a single-blind fashion—subjects were blinded to the gas mixture administered before each trial.
Two practice trials were performed prior to recorded testing to acclimate the subjects to the testing format. Four trials with each of the four gas mixtures were conducted at TLC followed by four additional trials at FRC.
The subjects were seated and breathed the appropriate gas mixture for 5 min according to a randomization list, via a disposable facemask. After 3 min. of breathing the gas, an expired CO2 measurement was obtained by having the subject take a full breath, remove the face mask without releasing any air and blow into an end-tidal CO2 (etCO2) analyzer (see below). The subject then replaced the facemask without taking a breath and continued to breathe the gas mixture for an additional 1 min. At the end of the last minute the subject was given a verbal cue to halt their breathing at either TLC or FRC, remove the facemask, and immediately immerse their face in a basin of room temperature water. To ensure full stimulation of the diving response the forehead and eye areas were required to be submerged.
Subjects were instructed to hold their breath as long as comfortably possible, but to end their breath hold after they felt the urge to breathe. They were also instructed to refrain from hyperventilation before the breath hold, to refrain from movements of the chest or other skeletal muscles, including swallowing movements, during the breath hold, and not to release any air into the water. At the end of the breath hold, the subject removed their face from the water and immediately provided a second etCO2 measurement before taking a breath of room air. They were allowed to breathe normally once this measurement was obtained. Five-minute rest periods separated each trial.
Heart Rate [HR, in beats per minute (BPM)] and arterial blood oxygen saturation (SaO2, in percent saturation) were recorded throughout each trial using a cardiosync pulse oximeter (NoniTMn Medical, Model 8700) with toe clip sensor and contact thermal printer printing continuously in real time. The oximeter had a stated accuracy of ± 2% of full scale in the range of 70–100% SaO2. Systolic and diastolic blood pressure were measured using an automatic digital blood pressure monitor with printer (Omron®, Model HEM-703CP), and were used to calculate mean arterial blood pressure (BP, in mm Hg). EtCO2 (in percent) was measured using a CO2 analyzer (BIOPAC CO2100C). BHD (in s) was recorded using the touch-print feature of the oximeter. Lastly, an elastic chest band recorded chest excursion to evaluate lung volume and extraneous chest movements during each trial (BIOPAC TSD101B).
Blood pressure was obtained once approximately 30 s prior to the breath hold and once during the breath hold. However, BP readings may not have been obtained during the trials that lasted less than 30 s. EtCO2 was measured 1 min prior to the breath hold and again immediately after the breath hold was terminated. SaO2 and HR were measured throughout the 3 min prior to the breath hold until completion of the second CO2 measurement. Data points were collected at 10-s intervals as well as at the timepoints of breath hold initiation and termination. To minimize recording errors, the subjects were instructed to keep their foot still while the toe clip was in place. Water and air temperatures were recorded prior to the first trial. Finally, all subjects were questioned about adverse experiences.
All data is presented as mean ± standard error (SE). Statistical comparisons were made with a computer statistical package (SigmaStat v14) and p < 0.05 was used as the level of significance. For both the controls and divers, data for males and females were not significantly different, and so were grouped together. For all data, three-way ANOVAs compared controls vs. divers, TLC vs. FRC, and the four gases pre-breathed before the face immersions. Two-way ANOVAs compared TLC vs. FRC and the four gases pre-breathed before the face immersions for HR and SaO2 at specific time points during the face immersions. One-way repeated measures ANOVAs were used to compare HR and SaO2 during the face immersion to the control value before the face immersion. Even if the BHD lasted longer than 35 s, statistical analysis on HR and SaO2 was only done on the first 35 s of the face immersion to facilitate statistical comparisons between groups. If statistical significance was reached during comparisons, it was then followed by post hoc testing (Tukey’s multiple comparison procedure or Dunnett’s multiple comparison vs. control) to determine which values were different from the others. T-tests were used to compare BP and etCO2 before and after the face immersions.
Initial analysis combines data from control and divers to determine the effects of lung volume and pre-existing chemoreceptor stimulation on HR, BP, SaO2, etCO2 and BHD responses to face immersion. We then subsequently analyze data between the control and divers to determine whether breath hold diving experience affects any of these parameters.
Face immersion into room temperature water produced a significant decrease in HR when breath holding at both TLC (F = 80.109, p < 0.001) and FRC (F = 69.145, p < 0.001) (Figure 1). HRs were significantly higher when breath holding at TLC vs. FRC at pre-immersion (F = 5.230, p = 0.024), 5 s (F = 9.734, p = 0.002), and 15 s (F = 17.522, p < 0.001) (Figure 1). However, the magnitude of the bradycardias was not significantly different when breath holding at either TLC or FRC. Chemoreceptor stimulation by breathing different gases before face immersion had little effect on the decrease in HR (Table 1). There were no significant differences in the HR responses between the four TLC face immersions, nor between the four FRC face immersions. Additionally, for each of the four gases, there were no significant differences in the HR responses between TLC and FRC face immersions.
Figure 1. Face immersion into room temperature water produced a significant decrease in HR when breath holding at both TLC and FRC. 1, HR is significantly less than TLC pre-immersion HR; 2, HR is significantly less than FRC pre-immersion HR; 3, TLC HR is significantly greater than FRC HR.
Table 1. Chemoreceptor stimulation by breathing different gases before face immersion did not affect the HR response (presented as HR ± SE) to face immersion.
Face immersion produced a significant increase in BP when breath holding at both TLC (p < 0.001) and FRC (p < 0.001; Figure 2). BP increased by 6 ± 1 mm Hg when breath holding at TLC, and by a significantly greater 10 ± 2 mm Hg when breath holding at FRC (p = 0.028). Chemoreceptor stimulation by breathing different gases before face immersion had little effect on increase in BP (Table 2). There were no significant differences between the BPs for the four TLC face immersions, nor between the BPs for the four FRC face immersions.
Figure 2. Face immersion into room temperature water produced a significant increase in BP when breath holding at both TLC and FRC. The increase in BP was significantly greater during FRC face immersions than TLC face immersions. 1, immersion BP is significantly greater than pre-immersion BP; 2, the increase in BP at FRC is significantly greater than the increase in BP at TLC.
Table 2. Chemoreceptor stimulation by breathing different gases before face immersion did not affect BP response (presented as BP ± SE) to face immersion.
During face immersion in room temperature water, SaO2 had a slight downward trend, but remained greater than 98% in both TLC and FRC face immersions. There were no differences in SaO2 between TLC and FRC during face immersion. When pre-breathing 100% O2 or 5% CO2 / 95% O2, SaO2 was statistically greater than when pre-breathing air or 15% O2 (F = 14.628, p < 0.001). However, pre-breathing different gases had little effect on SaO2 during face immersion, and even when pre-breathing 15% O2 before face immersion, SaO2 did not fall below 98% during face immersion.
During face immersion in room temperature water, etCO2 increased significantly from 6.13% ± 0.08 pre-immersion to 6.98% ± 0.09 post-immersion with TLC (p < 0.001), and from 5.92% ± 0.08 pre-immersion to 6.81% ± 0.09 post-immersion with FRC (p < 0.001) (Figure 3). Pre-breathing different gases did not change the pre-immersion etCO2, or the fact that there was a significant increase in etCO2 during the immersion (F = 10.52, p < 0.001). However, there was no consistent end-immersion etCO2 at which the breath holds terminated (Figure 4). After pre-breathing 5% CO2 / 95% O2 post-immersion etCO2 (7.24% ± 0.12) was significantly greater than for air (6.66% ± 0.12; F = 7.42, p = 0.003) or 15% O2 (6.59% ± 0.10; F = 7.42, p < 0.001). Also, after pre-breathing 100% O2, the post-immersion etCO2 (7.09% ± 0.13) was significantly greater than for air (F = 7.42, p = 0.028) or 15% O2, (F = 7.42, p = 0.013). In addition, there was no consistent increase in etCO2 after which the breath holds terminated. After breath holding at TLC, etCO2 increased by 1.21% ± 0.11 after pre-breathing 100% O2, which was a significantly greater increase in etCO2 (F = 7.39, p < 0.001) than that seen after pre-breathing air (0.66% ± 0.001; p < 0.001) or 15% O2 (0.64% ± 0.08; p < 0.001).
Figure 3. Face immersion into room temperature water produced a significant increase in etCO2 when breath holding at both TLC and FRC. 1, Post-immersion etCO2 is significantly greater than pre-immersion etCO2.
Figure 4. Breath holds after face immersion into room temperature water did not end at a consistent etCO2, and BHD was always longer at TLC than at FRC. There were significant differences between the post-immersion etCO2’s after pre-breathing different gases before breath holds. Thus there was no consistent etCO2 that initiated the termination of the breath holds. For each gas breathed before the breath hold, BHD at TLC was always longer than BHD at FRC. 1, Post-immersion etCO2 is significantly greater than post-immersion etCO2 after pre-breathing air at that lung volume. 2, Post-immersion etCO2 is significantly greater than post-immersion etCO2 after pre-breathing 15% O2 at that lung volume. 3, BDH at TLC is significantly longer than BHD at FRC after pre-breathing that specific gas. 4, BHD after pre-breathing 100% O2 at FRC is significantly longer than BHD after pre-breathing 15% O2 at FRC.
BHD after face immersion was significantly longer (F = 55.18, p < 0.001) at TLC (59 ± 3 s) than at FRC (32 ± 2 s), although it is notable that the post-immersion etCO2 at TLC and FRC was not significantly different from each other (F = 2.248, p = 0.137). Additionally, for each gas breathed before the breath hold, BHD when breath holding at TLC was always significantly longer than BHD when breath holding at FRC (air, p < 0.001; 15% O2, p < 0.001; 100% O2, p = 0.003; 5% CO2 / 95% O2, p = 0.003) (Figure 4).
Divers had a qualitatively similar cardiovascular diving response to the control subjects, and the differences between TLC and FRC face immersions that were seen in controls were also seen in divers. The pre-immersion HR of divers was significantly lower than the pre-immersion HR of controls (66 ± 1 for divers vs. 81 ± 1 for controls; F = 67.702, p < 0.001). Consequently, the face immersions HRs were significantly lower in divers than in controls (5 s, F = 54.868, p < 0.001; 15 s, F = 66.178, p < 0.001; 25 s, F = 100.817, p < 0.001; 35 s, F = 88.106, p < 0.001). However, the magnitude and time course of the bradycardias were similar between divers and controls. During face immersion divers showed a significant increase in BP (p < 0.001) that was similar in magnitude to that of controls (8 ± 2 mm Hg for controls vs. 7 ± 1 mm Hg for divers). As in controls, SaO2 had a slight downward trend during the breath holds in divers but did not decrease below 98% for any of the gases that were pre-breathed. The pre-immersion etCO2 of divers was significantly lower than that of controls (5.81% ± 0.10 for divers vs. 6.02% ± 0.06 for controls; F = 12.65, p < 0.001), although the end-immersion etCO2’s were not significantly different. Consequently, the increase in etCO2 during the face immersions was significantly greater for divers than for controls (0.97% ± 0.05 for divers vs. 0.79% ± 0.06 for controls; F = 6.38, p < 0.013). BHD in divers (50 ± 4 s) was significantly longer than BHD in controls (42 ± 2 s) (F = 4.34; p = 0.039), but not when specifically comparing at either TLC or FRC. In addition, for divers BHD at TLC (64 ± 6 s) was significantly longer (p < 0.001) than at FRC (35 ± 4 s). For controls BHD at TLC (54 ± 2 s) was significantly longer (p < 0.001) than at FRC (30 ± 2 s) (Figure 5).
Figure 5. Face immersion into room temperature water at TLC significantly increases BHD in both controls and divers. 1, BHD is significantly longer at TLC vs. FRC.
The most important finding from this study is that lung volume, and therefore presumably pulmonary stretch receptor activity, is an important physiological factor that contributes to the onset of the physiological breaking point during voluntary breath holding in room temperature water. BHD at TLC was always significantly longer than BHDs at FRC, even though the cardiovascular responses to face immersion at the two lung volumes were similar. Additionally, BHDs at TLC were always longer regardless of which gas was breathed before the breath hold or whether the subject had previous breath holding experience. Although PCO2 is the most important factor that contributes to the onset of the physiological breaking point (Lin et al., 1974; Fitz-Clarke, 2018), we found that there was no consistent etCO2 at which breath holds terminated.
Like previous studies investigating the human diving response, we found that face immersion in room temperature water produced bradycardia and an increase in blood pressure (Gooden, 1994; Foster and Sheel, 2005; Lindholm and Lundgren, 2009; Fitz-Clarke, 2018). Changing chemoreceptor stimulation by pre-breathing different gases before face immersion had little effect on the development of the bradycardia or the increase in BP. The HR response to breath holding after face immersion was similar at either TLC or FRC. Similar results were found by Andersson and Schagatay (1998) who reported that lung volume [60, 85, or 100% of Vital Capacity (VC)] does not appreciably change HR, skin capillary blood flow or blood pressure responses to breath holding. However, unlike Andersson and Schagatay (1998), we found that there was a greater increase in BP when breath holding at lower lung volumes (FRC) than at higher lung volumes (TLC). The intensification of peripheral vasoconstriction when breath holding at FRC would presumably lead to greater oxygen conservation during the breath hold (Andersson et al., 2000). Instead, however, BHD at FRC was significantly shorter than BHD at TLC.
The physiological breaking point signals the end of the easy-going phase of a voluntary breath hold. When physiological variables build up to the point where a signal to terminate the breath hold has been reached, the breath hold can only be continued through voluntary suppression of the drive to terminate the breath hold (Lin et al., 1974; Hentsch and Ulmer, 1984). The primary physiological variable that is thought to determine the physiological breaking point is an increase in chemoreceptor activity through an increase in PCO2 or a decrease in PO2 (Lin et al., 1974; Parkes, 2006). When breath holding with oxygen without face submersion, the physiological breaking point is reached when alveolar PCO2 reaches 48–54 mm Hg (Lin et al., 1974; Parkes, 2006), although the PCO2 will be lower if there is simultaneous hypoxia (Lin, 1987).
EtCO2 increased significantly during face immersions, and we found that breath holds at both TLC and FRC ended at an etCO2 of about 7%. Andersson and Schagatay (1998) found that breath holds at 60%, 85% and 100% of VC ended at an etCO2 of approximately 7.4%. When subjects pre-breathed different gases before breath holding, we found no post-immersion etCO2 at which all face immersions ended. Rather, pre-breathing 100% O2 or 5% CO2 / 95% O2 at FRC, or 5% CO2 / 95% O2 at TLC, significantly increased the post-immersion etCO2. Also, the difference between the pre-immersion etCO2 and post-immersion etCO2 was increased after pre-breathing 100% O2, at least at TLC. These data suggest that there is a synergistic effect between CO2 central chemoreceptor stimulation and O2 peripheral chemoreceptor stimulation (Fowler, 1954; Lin et al., 1974). Breathing a high O2 gas permits breath holds to terminate at higher etCO2’s. However, there is no absolute etCO2 that, when reached, terminates a breath hold.
In the present study, BHDs were so short that SaO2 decreased very little during face immersion. Hemoglobin remained above 98% saturated with oxygen, even after pre-breathing hypoxic gas. Consequently it was unlikely that arterial chemoreceptors were greatly stimulated through increasing hypoxemia during any of these breath holds. Therefore the peripheral chemoreflex, by itself, probably did not contribute to the cardiovascular (i.e., HR and BP) changes observed during the breath holds, or to the attainment of the physiological breaking point. However, even though oxygen saturations did not decrease substantially during the breath holds, PO2 had a synergistic effect with PCO2, as evidenced by significant differences in post-immersion etCO2 after pre-breathing different gases (Figure 4).
Although beginning a breath hold at either TLC or FRC did not appreciably change the cardiovascular responses to breath holding, breath holding at either TLC or FRC did significantly change BHD. BHDs were always longer at TLC than at FRC, even when subjects pre-breathed different gases. Andersson and Schagatay (1998) found that larger lung volumes increased the duration of both the easy-going phase and total breath holding duration. It is thought that a larger lung volume provides a sink that allows a build-up of CO2 and/or depletion of O2, thus allowing for a longer BHD (Sterba and Lundgren, 1985, 1988). However, even though there were significant differences in etCO2’s after pre-breathing different gases, there were no significant differences in BHD for the four face immersions at each of the two lung volumes (Figure 4). This suggests that lung volume, in addition to etCO2, is an important factor in determining physiological breaking point and breath holding capability.
The effect of lung volume on attainment of the physiological breaking point could stem from slowly adapting pulmonary stretch receptor (SAR) afferent activity. With greater expansion of the lungs with greater lung volumes, SAR activity will increase (Sant’Ambrogio, 1982; Schelegle and Green, 2001). When lung inflation is maintained, SARs characteristically show a long-lasting and sustained discharge (Sant’Ambrogio, 1982; Schelegle and Green, 2001). When breath holding at a large lung volume without face immersion, a Breuer–Hering like reflex may act to prolong maximal breath hold duration (Muxworthy, 1951; Chapin, 1955). We suggest that SAR activity during voluntary breath holding with face immersion at large lung volumes acts within the brainstem central rhythm generator to actively prolong phase II expiration and/or to inhibit generation of inspiration (D’Angelo and Agostoni, 1975; St. John and Zhou, 1990; Sant’Ambrogio and Sant’Ambrogio, 1991). The result of the SAR activity would be to extend the duration of the easy-going phase of a breath hold by suppressing attainment of the physiological breaking point that primarily results from an increase in PCO2. Thus at large lung volumes, inhibition of breath hold termination would occur due to neuronal interactions within the brainstem central rhythm generator, rather than due to a slower build-up of PCO2 within the lungs. Additionally, strong involuntary breathing movements are less frequent at high lung volumes, possibly explaining why higher lung volumes allows for longer BHDs (Fitz-Clarke, 2018).
Other explanations of the direct effect lung volume plays on BHD could be that the prolonged absence of SAR activity when breath holding at low lung volumes is, in itself, a respiratory stimulus that leads to the termination of the breath hold and reestablishment of eupnea (Fowler, 1954; Godfrey and Campbell, 1968, 1969). At low lung volumes there would be neither rhythmic nor static SAR afferent activity, because with eupnic breathing, and thus at lung volumes near to FRC, there is normally no SAR activity in humans (Sant’Ambrogio and Sant’Ambrogio, 1991). Additionally, the absence of any SAR input due to the voluntarily initiated apnea may act to terminate a breath hold (Mithoefer, 1959; Delapille et al., 2001). A technique that experienced breath hold divers sometimes use to prolong BHD is to engage in fictive breathing movements by pumping the thoracic cavity while keeping the glottis closed [personal observations; (Lin, 1982)]. This would have the effect of changing lung volume, and thus provide SAR input to the brainstem, even if no fresh air reaches the lungs. Rebreathing during a breath hold extends BHD, even though arterial blood gases do not improve (Fowler, 1954; Godfrey and Campbell, 1968, 1969). Another technique used to prolong breath holds is to open the epiglottis and swallow. Although this would not change lung volume or SAR activity, it would provide a rhythmic input into brainstem respiratory centers. However, swallowing or taking a “false breath” while breath holding reduces the magnitude of diving bradycardia (Gandevia et al., 1978), and involuntary breathing movements during breath holding are thought to be too small to influence the diving response (Andersson and Schagatay, 1998).
The experienced divers in our study were members of a local underwater hockey club. Qualitatively, the diving response of divers was similar to that of controls. However, the BHD of divers was significantly longer than that of controls, although not as long as that seen in experienced underwater hockey or rugby players (Davis et al., 1987; Schagatay and Andersson, 1998), or synchronized swimmers (Oldridge et al., 1978; Bjurström and Schoene, 1987). However, our divers were recreational underwater hockey players, rather than national caliber players as in the study by Davis et al. (1987). Additionally, through our instructions to them, divers ended their face immersions at or near their physiological breaking points, rather than near to their conventional breaking points. During the struggle phase of breath hold diving, the physiological drive to terminate the breath hold is consciously suppressed. Breath holding experience or apnea training can prolong total BHD by delaying the conventional breaking point (Davis et al., 1987; Schagatay et al., 1999, 2000). Thus, with our instructions to our subjects to end their breath holds when they felt the urge to breath and not to use fictive breathing techniques, we negated the training effects that could have given the trained divers a breath holding “advantage”.
We conclude many factors bring about the physiological breaking point during breath holding. These include increased PaCO2 (as measured by etCO2), although there is no absolute etCO2 or change in etCO2 that, when reached, will initiate the termination of the breath hold. We also conclude that high lung volume, or more specifically the increased pulmonary stretch receptor activity at high lung volume, is an important factor that contributes to the physiological breaking point. We suggest that high lung volume acts centrally to inhibit the termination of breath holds, and thus prolong the duration of the easy-going phase of voluntary breath holding. We also conclude that increased arterial chemoreceptor activity due to the development of hypoxia during the breath hold plays a limited, if any, role in terminating most short duration breath holds. Finally, we conclude that previous breath holding experiences increases breath hold durations without substantially changing cardiorespiratory (HR, BP SaO2, and etCO2) responses to face immersion.
The raw data supporting the conclusions of this article will be made available by the authors, without undue reservation.
The studies involving human participants were reviewed and approved by Midwestern University Institutional Review Board. The patients/participants provided their written informed consent to participate in this study.
PM and BG conceptualized and planned the study, formulated research protocols, and obtained IRB approval. BG and JS collected data. PM performed statistical analysis, created figures, and wrote the manuscript. All authors contributed to the article and approved the submitted version (except JS).
This work was supported by Midwestern University Intramural Research, and a Summer Research Fellowship to BG.
The authors declare that the research was conducted in the absence of any commercial or financial relationships that could be construed as a potential conflict of interest.
All claims expressed in this article are solely those of the authors and do not necessarily represent those of their affiliated organizations, or those of the publisher, the editors and the reviewers. Any product that may be evaluated in this article, or claim that may be made by its manufacturer, is not guaranteed or endorsed by the publisher.
We would like to thank all the subjects who participated in our study, and the Midwestern University Underwater Hockey Club.
Andersson, J., and Schagatay, E. (1998). Effects of lung volume and involuntary breathing movements on the human diving response. Eur. J. Appl. Physiol. 77, 19–24. doi: 10.1007/s004210050294
Andersson, J., Schagatay, E., Gislen, A., and Holm, B. (2000). Cardiovascular responses to cold-water immersions of the forearm and face, and their relationship to apnoea. Eur. J. Appl. Physiol. 83, 566–572. doi: 10.1007/s004210000317
Bjurström, R. L., and Schoene, R. B. (1987). Control of ventilation in elite synchronized swimmers. J. Appl. Physiol. 63, 1019–1024. doi: 10.1152/jappl.1987.63.3.1019
Castellini, M. (2012). Life under water: physiological adaptations to diving and living at sea. Comprehen. Physiol. 2, 1889–1919. doi: 10.1002/cphy.c110013
Chapin, J. L. (1955). Relationship between lung volume and breath-holding breaking point. J. Appl. Physiol. 8, 88–90. doi: 10.1152/jappl.1955.8.1.88
D’Angelo, E., and Agostoni, E. (1975). Tonic vagal influences on inspiratory duration. Respir. Physiol. 24, 287–302. doi: 10.1016/0034-5687(75)90019-5
Davis, F. M., Graves, M. P., Guy, H. J., Prisk, G. K., and Tanner, T. E. (1987). Carbon dioxide response and breath-hold times in underwater hockey players. Unders. Biomed. Res. 14, 527–534.
Delapille, P., Verin, E., Tourny-Chollet, C., and Pasquis, P. (2001). Breath-holding time: effects of non-chemical factors in divers and non-divers. Eur. J. Physiol. 442, 588–594. doi: 10.1007/s004240100568
Dujic, Z., Uglesic, L., Breskovic, T., Valic, Z., Heusser, K., Marinovic, J., et al. (2009). Involuntary breathing movements improve cerebral oxygenation during apnea struggle phase in elite divers. J. Appl. Physiol. 107, 1840–1846. doi: 10.1152/japplphysiol.00334.2009
Fitz-Clarke, J. R. (2018). Breath-Hold Diving. Compreh. Physiol. 8, 585–630. doi: 10.1002/cphy.c160008
Foster, G. E., and Sheel, A. W. (2005). The human diving response, its function, and its control. Scand. J. Med. Sci. Sports 15, 3–12. doi: 10.1111/j.1600-0838.2005.00440.x
Fowler, W. S. (1954). Breaking point of breath-holding. J. Appl. Physiol. 6, 539–545. doi: 10.1152/jappl.1954.6.9.539
Gandevia, S. C., Mccloskey, D. I., and Potter, E. K. (1978). Reflex bradycardia occurring in response to diving, nasopharyngeal stimulation and ocular pressure, and its modification by respiration and swallowing. J. Physiol. 276, 383–394. doi: 10.1113/jphysiol.1978.sp012241
Godfrey, S., and Campbell, E. J. (1968). The control of breath holding. Respir. Physiol. 5, 385–400. doi: 10.1016/0034-5687(68)90030-3
Godfrey, S., and Campbell, E. J. (1969). Mechanical and chemical control of breath holding. Q. J. Exp. Physiol. Cogn. Med. Sci. 54, 117–128. doi: 10.1113/expphysiol.1969.sp002011
Gooden, B. A. (1994). Mechanism of the human diving response. Integr. Physiol. Behav. Sci. 29, 6–16. doi: 10.1007/BF02691277
Hentsch, U., and Ulmer, H. V. (1984). Trainability of underwater breath-holding time. Int. J. Sports Med. 5, 343–347. doi: 10.1055/s-2008-1025930
Kawakami, Y., Natelson, B. H., and Dubois, A. B. (1967). Cardiovascular effects of face immersion and factors affecting diving reflex in man. J. Appl. Physiol. 23, 964–970. doi: 10.1152/jappl.1967.23.6.964
Lin, Y. C. (1982). Breath-Hold Diving in Terrestrial Mammals. Philadelphia: Franklin press. doi: 10.1249/00003677-198201000-00009
Lin, Y. C. (1987). Effect of O2 and CO2 on Breath-Hold Breaking Point. Bethesda MD: Undersea and Hyperbaric Medical Society.
Lin, Y. C. (1988). Applied physiology of diving. Sports Med. 5, 41–56. doi: 10.2165/00007256-198805010-00004
Lin, Y. C., Lally, D. A., Moore, T. O., and Hong, S. K. (1974). Physiological and conventional breath-hold breaking points. J. Appl. Physiol. 37, 291–296. doi: 10.1152/jappl.1974.37.3.291
Lindholm, P., and Lundgren, C. E. G. (2009). The physiology and pathophysiology of human breath-hold diving. J. Appl. Physiol. 106, 284–292. doi: 10.1152/japplphysiol.90991.2008
Manley, L. (1990). Apnoeic heart rate responses in humans. Sports Med. 9, 286–310. doi: 10.2165/00007256-199009050-00004
Mithoefer, J. C. (1959). Lung volume restriction as a ventilatory stimulus during breath holding. J. Appl. Physiol. 14, 701–705. doi: 10.1152/jappl.1959.14.5.701
Moore, T. O., Lin, Y. C., Lally, D. A., and Hong, S. K. (1972). Effects of temperature, immersion, and ambient pressure on human apneic bradycardia. J. Appl. Physiol. 33, 36–41. doi: 10.1152/jappl.1972.33.1.36
Muxworthy, J. F. J. (1951). Breath holding studies: Relationship to lung volume. Air Force Techn. Rep. 6528, 452–456.
Oldridge, N. B., Heigenhauser, G. J., Sutton, J. R., and Jones, N. L. (1978). Resting and exercise heart rate with apnea and facial immersion in female swimmers. J. Appl. Physiol. 45, 875–879. doi: 10.1152/jappl.1978.45.6.875
Palada, I., Bakovic, D., Valic, Z., Obad, A., Ivancev, V., Eterovic, D., et al. (2008). Restoration of hemodynamics in apnea struggle phase in association with involuntary breathing movements. Respir. Physiol. Neurobiol. 161, 174–181. doi: 10.1016/j.resp.2008.01.008
Parkes, M. (2006). Breath-holding and its breakpoint. Exp. Physiol. 91, 1–15. doi: 10.1113/expphysiol.2005.031625
Sant’Ambrogio, G. (1982). Information arising from the tracheobronchial tree of mammals. Physiol. Rev. 62, 531–569. doi: 10.1152/physrev.1982.62.2.531
Sant’Ambrogio, G., and Sant’Ambrogio, F. B. (1991). “Reflexes from the airway, lung, chest wall, and limbs,” in The Lung: Scientific Foundations, eds R. G. Crystal, J. B. West, P. J. Barnes, N. S. Cherniak, and E. R. Weibel (New York: Raven Press), 1383–1395.
Schagatay, E., and Andersson, J. (1998). Diving Response and Apneic Time in Humans. Unders. Hyperb. Med. 25, 13–19.
Schagatay, E., Van Kampen, M., and Andersson, J. (1999). Effects of repeated apneas on apneic time and diving response in non-divers. Unders. Hyperb. Med. 26, 143–149.
Schagatay, E., Van Kampen, M., Emanuelsson, S., and Holm, B. (2000). Effects of physical and apnea training on apneic time and the diving response in humans. Eur. J. Appl. Physiol. 82, 161–169. doi: 10.1007/s004210050668
Schelegle, E. S., and Green, J. F. (2001). An overview of the anatomy and physiology of slowly adapting pulmonary stretch receptors. Respir. Physiol. 125, 17–31.
St. John, W. M., and Zhou, D. (1990). Discharge of vagal pulmonary receptors differentially alters neural activities during various stages of expiration in the cat. J. Physiol. 424, 1–12. doi: 10.1113/jphysiol.1990.sp018051
Sterba, J. A., and Lundgren, C. E. (1988). Breath-hold duration in man and the diving response induced by face immersion. Unders. Biomed. Res. 15, 361–375.
Keywords: face immersion, human diving response, lung volume, physiological breaking point, chemoreceptor stimulation
Citation: McCulloch PF, Gebhart BW and Schroer JA (2021) Large Lung Volumes Delay the Onset of the Physiological Breaking Point During Simulated Diving. Front. Physiol. 12:731633. doi: 10.3389/fphys.2021.731633
Received: 28 June 2021; Accepted: 10 September 2021;
Published: 29 September 2021.
Edited by:
Fabrice Joulia, Université de Toulon, FranceReviewed by:
Alessandro Marroni, DAN Europe Foundation, MaltaCopyright © 2021 McCulloch, Gebhart and Schroer. This is an open-access article distributed under the terms of the Creative Commons Attribution License (CC BY). The use, distribution or reproduction in other forums is permitted, provided the original author(s) and the copyright owner(s) are credited and that the original publication in this journal is cited, in accordance with accepted academic practice. No use, distribution or reproduction is permitted which does not comply with these terms.
*Correspondence: Paul F. McCulloch, cG1jY3VsQG1pZHdlc3Rlcm4uZWR1
†Deceased
Disclaimer: All claims expressed in this article are solely those of the authors and do not necessarily represent those of their affiliated organizations, or those of the publisher, the editors and the reviewers. Any product that may be evaluated in this article or claim that may be made by its manufacturer is not guaranteed or endorsed by the publisher.
Research integrity at Frontiers
Learn more about the work of our research integrity team to safeguard the quality of each article we publish.