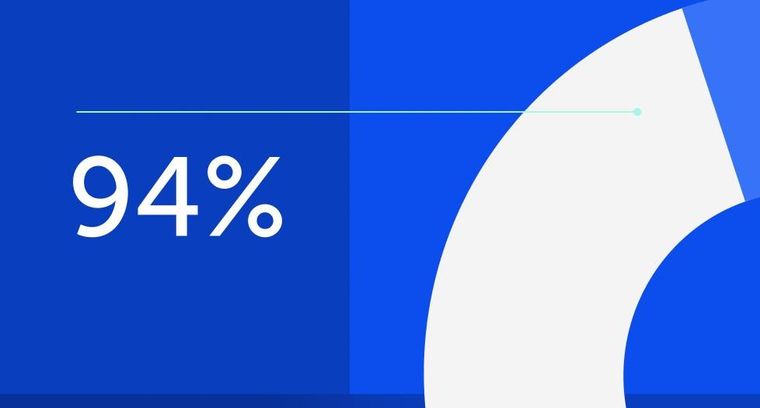
94% of researchers rate our articles as excellent or good
Learn more about the work of our research integrity team to safeguard the quality of each article we publish.
Find out more
ORIGINAL RESEARCH article
Front. Physiol., 26 November 2021
Sec. Membrane Physiology and Membrane Biophysics
Volume 12 - 2021 | https://doi.org/10.3389/fphys.2021.728625
This article is part of the Research TopicIon Channels and Transporters in Ca2+ -dependent Functions of LymphocytesView all 10 articles
Ca2+ is an important intracellular second messenger known to regulate several cellular functions. This research aimed to investigate the mechanisms of exercise-induced immunosuppression by measuring intracellular calcium levels, Ca2+-regulating gene expression, and agonist-evoked proliferation of murine splenic T lymphocytes. Mice were randomly assigned to the control, sedentary group (C), and three experimental groups, which performed a single bout of intensive and exhaustive treadmill exercise. Murine splenic lymphocytes were separated by density-gradient centrifugation immediately (E0), 3h (E3), and 24h after exercise (E24). Fura-2/AM was used to monitor cytoplasmic free Ca2+ concentration in living cells. The combined method of carboxyfluorescein diacetate succinimidyl ester (CFSE) labeling and flow cytometry was used for the detection of T cell proliferation. The transcriptional level of Ca2+-regulating genes was quantified by using qPCR. Both basal intracellular Ca2+ levels and agonist (ConA, OKT3, or thapsigargin)-induced Ca2+ transients were significantly elevated at E3 group (p<0.05 vs. control). However, mitogen-induced cell proliferation was significantly decreased at E3 group (p<0.05 vs. control). In parallel, the transcriptional level of plasma membrane Ca2+-ATPases (PMCA), sarco/endoplasmic reticulum Ca2+-ATPases (SERCA), TRPC1, and P2X7 was significantly downregulated, and the transcriptional level of IP3R2 and RyR2 was significantly upregulated in E3 (p<0.01 vs. control). In summary, this study demonstrated that acute exercise affected intracellular calcium homeostasis, most likely by enhancing transmembrane Ca2+ influx into cells and by reducing expression of Ca2+-ATPases such as PMCA and SERCA. However, altered Ca2+ signals were not transduced into an enhanced T cell proliferation suggesting other pathways to be responsible for the transient exercise-associated immunosuppression.
Exercise can enhance marked transient physiological changes and has a profound influence on the human immune function. There are a number of studies showing that the effects of acute exhaustive exercise seem to be detrimental, while regular, moderate-intensity physical activity can improve immune defense functions. There is evidence that the transient “open-window” of immunosuppression might exist in the recovery from strenuous exercise as indicated by an impaired immunity around 3–72h after exercise when athletes seem to be more susceptible to infections. However, this consensus meaning has been disagreed recently due to novel findings demonstrating that exercise stimulates T cell redistribution within organs and tissues, enhances mobilization of hematopoietic stem cells as a result of apoptotic T cells, and reverses T cell immunosenescence (Simpson et al., 2020). Therefore, the effect of a single bout of intensive exercise on immune function remains a controversial topic, and the investigation of underlying molecular mechanisms seems to be a helpful approach to enhance our current understanding.
Ca2+ is a key second messenger in the network of cellular signal transduction, which participates in many physiological and pathological processes. The stress of exercise may influence the intracellular Ca2+ dynamics of lymphocytes. By using Fura-2 AM dual-wavelength detection technology, our previous work reported that long-term physical training had a significant effect on intracellular calcium signal transduction of lymphocytes in mice (Liu et al., 2017). Since alterations of calcium metabolism have been shown to be involved in some abnormal immune responses, we hypothesized that the mechanisms of acute exercise-induced immunosuppression involve abnormalities in intracellular calcium handling. In order to test this hypothesis, this research examined the effects of acute exercise on intracellular free calcium ion concentrations under basal and agonist-stimulated conditions, the expression of Ca2+-handling factors, and changes of T cell function.
In this study, we used a total of 60, 12-week-old male CD1 Swiss mice, weighing (28.0±3.2g), which were fed in the animal facilities at the Department of Sports Medicine of University of Münster and Justus-Liebig University (Germany), and kept under controlled conditions of temperature and humidity. This specie and model were chosen for being a homogeneous line of mice. Mice were housed collectively (4–6 per cage) and fed tap standard rodent diet and water at will, with a standard 12h day-night cycle. All procedures were performed following the approval of the local Animal Care and Use Committee. We exert our effort to minimize animal pain and discomfort, and this experiment was performed in accordance with the ARRIVE guidelines.
Animals were adapted to exercise for 1week before the exercise test, aiming to minimize the stress induced by the equipment. Each animal underwent an incremental exercise test to exhaustion to measure individual maximal oxygen uptake (VO2max) and the fastest speed as our group previously described (Kruger et al., 2009). After a 5-min warm-up at 0.20m/s, the running speed was elevated by 0.05m/s every 3min until exhaustion. The animals were randomly divided into in four groups, five in each group. The animals in the control group had exposure to the noise of treadmill and allowed to freely run on a treadmill without effort, while the animals of the exercise groups were submitted a single bout of exercise of 80% VO2max workload until exhaustion, which were anesthetized and killed immediately (E0), 3h (E3), and 24h (E24) after exercise for spleen removal.
After the animals were anaesthetized and killed by cervical dislocation, their spleens were separated under aseptic conditions and placed on a 100-μm-pore size nylon mesh (BD Falcon™ Cell Strainer, BD Biosciences, Heidelberg, Germany). And the mesh was put into a culture dish, which PBS was poured into. The spleen was gently squeezed with a 2-ml syringe plunger to generate single cell suspensions. Biocoll separating solution (Biochrom, Berlin, Germany) was used, and lymphocytes are stratified after density gradient centrifugation as our group described before (Liu et al., 2017). The white band of lymphocytes was removed after centrifugation and washed two times by centrifugation in Hanks’ Balance Salt Solution (HBSS) containing 5% heat-inactivated fetal calf serum (FCS; Gibco, Darmstadt, Germany). The cell suspension in RPMI1640 containing 10% FCS was prepared to be measured. Cell viability (98%) was quantified by the Trypan blue exclusion assay, whereas purity (95%) was verified by a flow cytometry (EPICS XL Beckman Coulter, Fullerton, CA, United States) in the forward/sideward scatter mode. Moreover, the percentage of T cells in the remaining mixed lymphocyte population was determined to be about 85% by labeling with anti-CD3 antibody as described recently (Krüger et al., 2008). The number of cells was then counted by using a semiautomated hematology analyzer (F-820, Sysmex, Norderstedt, Germany).
Intracellular Ca2+ level was assessed by the fluorescence intensity ratio of the calcium probe Fura-2 AM (Molecular Probes Inc., Eugene, OR, United States) as described our previous study (Liu et al., 2017). Briefly, the cells were loaded with 5μl/ml Fura-2 AM stock (1mM in DMSO) in 0.8mM Ca2+-containing solution (140mM NaCl, 3mM KCl, 0.4mM Na2HPO4, 10mM HEPES, 5mM glucose, and 1mM MgCl2, and 0.8mM CaCl2 with pH 7.4). The fluorescence intensities were measured in the dual wavelength ratio mode in a spectrofluorometer (Deltascan; PTI, Canada) at 340 and 380nm (excitation filters) and 510nm (emission filter). According to the equation of Grynkiewicz et al. (1985), [Ca2+]i was calculated as follows: [Ca2+]i=(R-Rmin)/(Rmax-R)×Kd×F, with a Kd of Fura-2 for calcium of 220nM, and where R is the ratio of fluorescence of the sample at 340 and 380nm. Rmax and Rmin are the ratios for Fura-2 at these wavelengths in the presence of saturating Ca2+ (after application of 10mM digitonin) and during Ca2+-free conditions (after addition of EGTA, 10mM final concentration), respectively, and F is the ratio of fluorescence intensity at 380nm during Ca2+-free conditions to the fluorescence intensity at 380nm during Ca2+-saturating conditions. To remove the free fura-2AM, cells loaded with the dye were washed two times with PBS solution. The maximum peak and plateau level of the agonist-induced [Ca2+]i transient was quantified after the addition of mitogen, concanavalin A (Con A; Sigma Aldrich, St. Louis, United States), the anti-CD3 antibodies (OKT3; Janssen-Cilag, Neuss, Germany), or thapsigargin (TG; Sigma Aldrich, St. Louis, United States). To estimate intracellular Ca2+ release and extracellular Ca2+ influx, experiments were performed either in absence or in presence of 0.8mmol/l Ca2+ in the measurement medium. For experiments performed in the absence of extracellular calcium, CaCl2 was replaced by 1mM EGTA in the buffer. The addition of Ca2+ allowed measuring transmembrane Ca2+ influx.
Bivalent cation, Mn2+ and Ca2+, can share common entry channels in cell membrane (Sage et al., 1989), and the former can bind to intracellular Fura-2 (Fura-2-acetoxymethyl ester), with a stronger chemical attraction than the latter and to quench the fluorescence. The decrease in fluorescence of Fura-2 can reflect extracellular Mn2+ influx into cells. The determination of Ca2+ entry can be quantified the Mn2+-quenching technique (Ferreira et al., 2009). The Mn2+-quenching experiments were carried out by using the same equipment as that for intracellular Ca2+ measurements as described above. In the experiments, we used 4mM Mn2+ in the measurement buffer which corresponds to more than twice extracellular Ca2+ concentration. The fluorescence was excited at the isosbestic point at 360nm, and emission was monitored at 510nm. The rate of Mn2+ entry can be obtained from the slope of the fluorescence intensity curve with time. The rate of Mn2+-quenching is shown by the slope of the tangent against a quenching curve after the addition of stimulant. The basal rate of Mn2+-quenching can be determined by measuring “slopes 1” of the initial Fura-2 fluorescence decline, while the rate of fluorescence intensity decreases after the addition of TG (=“slope 2”). Therefore, ∆slope was regarded as an index to access the rate of fluorescence quenching by extracellular Mn2+ influx into cells.
Lymphocytes were prepared as described above, and cell proliferation was monitored using the cell tracker dye carboxyfluorescein diacetate succinimidyl ester (CFSE, Molecular Probes, Eugene, OR, United States) according to the standard procedure provided by Quah and Parish (2010). Briefly, cells were stained with CFSE dye at 5μM concentrations and loaded at 37°C for 10min, and the reaction was terminated by using PBS with 10% (v/v) heat-inactivated FCS. After washing, cells were resuspended in Dulbecco’s modified eagle medium (DMEM, PAA Laboratories, Pasching, Austria) with 10% (v/v) heat-inactivated FCS, 50mg/ml streptomycin, and 50U/ml penicillin. And the cells were seeded at a density of 2×106 cells/per culture dish and then left to incubate at 37°C in a humidified 5% CO2 atmosphere with additional doses of 2.5μg/ml Con A or PHA (Sigma-Aldrich, St.Louis, MO, United States). After 72h of incubation, cells were harvested, stained with anti-CD3-PE antibody (Immunotools, Friesoythe, Germany). Data analysis was performed using a flow cytometer with FlowJo software (Version X; TreeStar, Ashland, OR, United States). AUC is defined as the area under the curve enclosed by the coordinate axis, of which the x-axis indicates cellular generations, and the y-axis shows the percentage of cells in each generation. △AUC as an index was used to evaluate cell proliferative ability.
Murine CD3+ T cells were purified directly from splenic cells using an EasySep™ Mouse T Cell Isolation Kit (StemCell Technologies, Vancouver, CA, United States) based on magnetic bead separation technique according to manufactures’ instructions. Total RNA was extracted from T lymphocytes using the RNA isolation kit (RNeasy Mini Kit, Qiagen, Hilden, Germany), and a UV-Vis spectrophotometer (ND-1000, Nano-Drop Technologies, Rockland, United States) was used to determine the quantity and purity of the extracted RNA. To remove possible DNA contamination, on-column DNase digestion was applied by using the RNase-free DNase set (Qiagen, Hilden, Germany) in the context of RNA isolation. cDNA was subsequently synthesized by using a cDNA synthesis kit (high-capacity cDNA reverse transcription kit, Applied Biosystems) from RNA samples according to the manufacturer’s protocol. cDNA was obtained by on a PCR thermal cycler (T100, Bio-Rad Laboratories, Munich, Germany), and the product was used for qPCR. To determine mRNA expression in T cells, PCR was performed by using the iQ SYBR Green Supermix (Bio-Rad Laboratories, Munich, Germany), and an iCycler (Bio-Rad Laboratories, Munich, Germany) was applied to quantify the amplification products. The PCR primers used are listed in Table 1. The reaction conditions were as follows: 1cycle at 95°C for 3min, 42cycles at 95°C for 15s (denaturation), 61°C for 30s (annealing), and 72°C for 30s (elongation). The transcriptional level was normalized to the mRNA expression of housekeeping gene, β-actin. The results were calculated using the 2−Δ(ΔCt) method and expressed as fold change in comparison with controls.
Data are expressed as means±SEM. Shapiro–Wilkʼs test revealed that data were normally distributed; thus, statistical analysis was performed using one-way ANOVA for comparison between groups. p<0.05 was set as statistical significance. SPSS 20.0 software was used throughout.
In the control group, basal [Ca2+]i of T cells was determined to be 45.5±5.1nM. Immediately after exercise, the resting [Ca2+]i was 49.7±6.2nM, and no significant change was observed (p>0.01 vs. control, n=5). After 3h of post-exercise recovery, the resting [Ca2+]i was highly significantly increased to 101.0±30.9nM (p<0.01 vs. control, n=5), and the resting intracellular calcium level 24h after exercise (E24) was 46.0±15.2nM and unchanged to pre-exercise conditions (p>0.01 vs. control, n=5; Figure 1).
Figure 1. The change of basal [Ca2+]i of murine splenic T lymphocytes in the control group (C), immediately after (E0), 3h after (E3), and 24h (E24) after acute exercise. Data are presented as means±SEM. **p<0.01 vs. control.
Next, cells were stimulated with different agonists known to affect intracellular Ca2+ concentrations such as Con A and anti-CD3 antibody (OKT3). Representative tracings of intracellular Ca2+ signal after the administration of Con A in calcium containing or Ca2+-free buffer are shown in Figures 2A,B, respectively. The stimulant induced a biphasic intracellular Ca2+ transient with a peak and a plateau phase in calcium containing buffer. After addition of Con A, a significant absolute and net increase (=Δ[Ca2+]I) of intracellular Ca2+ concentration ([Ca2+]i) in the E3 group could be observed during both peak (Figures 2C,D) and plateau phase (Figures 2E,F; p<0.05 vs. control, n=5). In Figures 2G,H, the measurement was performed in the presence of Ca2+-free buffer. After an initial resting phase of 100s (=time 1), Con A was added (=time 2) followed by administration of CaCl2 (=time 3). By subtracting mean concentrations at time 1 from time 2 and at time 2 from time 3, the resulting differences should indicate Ca2+ release from intracellular stores (stage 1) and transmembrane Ca2+ influx (stage 2), respectively. After the administration of Con A, intracellular Ca2+ release wasnot affected in the E3 group (p>0.05 vs. control, n=5), while an improved transmembrane Ca2+ influx into cells could be observed (p<0.05 vs. control, n=5). Further stimulations were performed only up to 3h after exercise.
Figure 2. The effect of acute exercise on Con A-induced change of [Ca2+] of murine splenic lymphocytes at the different time points after exercise. (A,B) The representative tracings of Con A-induced change of intracellular Ca2+ signal in the calcium buffer and the Ca2+-free buffer, respectively; arrow shows when Con A (at 100s) or Ca2+ (at 350s) were applied; tracing C, the control group; tracings E0, E3, and E24 indicate the exercise groups with cell isolation at time points after, 3h, and 24h after exercise. (C–H) Bar chart diagrams summarizing the results of the entire groups. (C–F) The experiments were performed in the Ca2+ buffer; [Ca2+]i and Δ[Ca2+]i were referred to as absolute [Ca2+]i and net [Ca2+]i after the addition of Con A, respectively, and were presented separately for peak and plateau phase. (G,H) The measurements were performed in Ca2+-free buffer; Time 1: initial resting period, Time 2: addition of Con A, and Time 3: addition of CaCl2; the calculated Δ[Ca2+]i levels of stage 1 and stage 2 indicate Ca2+ release from ER and transmembrane Ca2+ influx from extracellular space, respectively. Data are presented as means±SEM (n=5). * means p<0.05 vs. control; # means p<0.05 vs. E0.
After stimulation with the anti-CD3 antibody (OKT-3), the representative tracings of intracellular Ca2+ signals in calcium containing or Ca2+-free buffer are shown in Figures 3A,B, respectively. In contrast to stimulation with Con A, a monophasic response after OKT-3 was found making an analysis of peak and plateau values quite impossible. The pattern of exercise effects on anti-CD3 induced Ca2+ transients, however, was similar to Con A. In Figures 3C,D, using 20μg/ml OKT-3, a significant increase of absolute and net [Ca2+]i was found in the E3 group (p<0.05 vs. control, n=5). When cells were suspended first in Ca2+-free solution followed by addition of OKT3, the release of intracellular Ca2+ wasnot changed significantly in the E3 group (p>0.05 vs. control, n=5). Upon addition of Ca2+, an improved transmembrane Ca2+ influx was shown after exercise (p<0.01 vs. control, n=5; Figures 3E,F).
Figure 3. The effect of acute exercise on OKT3-induced change of [Ca2+]i of murine splenic lymphocytes at the different time points after exercise. (A,B) The representative tracings of OKT3-induced change of intracellular Ca2+ signal in the calcium buffer and the Ca2+-free buffer, respectively; arrow shows when OKT3 (at 100s) and Ca2+ were applied (at 350s); tracing C, the control group; tracing E0, and E3 indicate the exercise groups with cell isolation at time points after and 3h after exercise. (C–F) Bar chart diagrams summarize the results of the entire group. (C,D) The measurement was performed in the Ca2+ buffer; [Ca2+]i plateau and Δ[Ca2+]i plateau were referred to as absolute [Ca2+]i and net [Ca2+]i during the plateau phase after stimulation with OKT3, respectively. (E,F) The measurement was performed in the Ca2+-free buffer; after the initiative scanning for time 1, OKT3 was added into the buffer for time 2. Next, calcium was administrated for time 3; the calculated Δ[Ca2+]i levels of stage 1 and stage 2 indicate Ca2+ release from ER and transmembrane Ca2+ influx from extracellular space, respectively. Data are presented as means±SEM (n=5). *p<0.05 or **p<0.01 vs. control, #p<0.05 or ##p<0.01 vs. E0 (n=5).
Finally, thapsigargin (TG), an inhibitor of sarco-endoplasmic Ca2+-ATPase, was used. After application of TG in the Ca2+-free buffer, a similar release of Ca2+ from intracellular stores could be observed suggesting an identical load of intracellular calcium stores. However, after addition of Ca2+ into the extracellular medium, an enhanced Ca2+ influx signal was found in the E3 group suggesting an improved entry of extracellular calcium (p<0.05 vs. control, n=5; in Figures 4A,B). The calcium transients after TG stimulation were monophasic in nature (not biphasic as after Con A stimulation) and were analyzed therefore as single values.
Figure 4. The effect of acute exercise on TG-induced change of [Ca2+]i and Mn2+ influx in murine splenic lymphocytes. C: the control group; E3: the exercise group with cell isolation 3h after exercise. (A) Measurement of [Ca2+]i initially under Ca2+-free buffer (=time 1), application of TG (=time 2), and addition of CaCl2 (=time 3); (B) Ca2+ release was quantified by the differences of levels between time 2 and time 1, whereas Ca2+ influx was quantified by the differences of levels between time 3 and time 2. (C) Representative tracings of the manganese influx measurements of the E3 group (heavy dotted line) and C group (light continuous line). (D) The bar chart diagrams show that transmembrane Mn2+ influx into cells after the addition of TG was statistically elevated in E3. Data are presented as means±SEM (n=5). *p<0.05 vs. control (n=5).
In order to confirm an exercise-associated enhanced Ca2+ entry, we used the Mn2+-quenching technique. As shown schematically in Figure 4C, the fluorescence intensity at a wavelength of 360nM continuously decreased after addition of Mn2+. After the application of TG, we observed a larger deflection of the Fura-2 fluorescence quenching in the E3 group (bold line) compared to control group (light line), which indicates an enhanced transmembrane Mn2+ influx into cells after exercise (p<0.05 vs. control, n=5), as shown in Figure 4D.
As shown in Figure 5, the transcription levels of cellular calcium ATPase (PMCA1, SERCA3) and ion channels (TRPC1, P2X7) were statistically reduced, while the transcription levels of internal Ca2+ release channels (IP3R2, RYR2) were significantly enhanced in the E3 group compared to control group (p<0.05/p<0.01 vs. control, n=5). The transcription level of ion channel, TRPM5, was significantly reduced, while transcription levels of intracellular Ca2+-regulating factors, IP3R2, ATP2C1, Cav2.3, and Kcnk5, were significantly increased in the E24 group compared to control group (p<0.05/p<0.01/p<0.001 vs. control, n=5). The transcription levels of TRPC1 and Cav2.3 were significantly increased, while the transcription levels of intracellular Ca2+-regulating genes, TRPV6 and Hspa1a, were statistically reduced in the E24 group compared to E3 group (p<0.05/p<0.01 vs. the E3 group, n=5).
Figure 5. The effects of acute exercise in transcriptional level of Ca2+-regulating genes of murine splenic T lymphocytes. (A) mRNA expression of the E3 group vs. the control. (B) mRNA expression of the E24 group vs. the control. (C) mRNA expression of the E24 group vs. the E3 group. The transcription level of Ca2+-regulating genes was normalized to the housekeeping gene, β-actin mRNA. Note that columns and error bars represent (mRNA in % to respective control ±SEM); *p<0.05, **p<0.01, ***p<0.001 (n=5).
A single acute bout of exhaustive exercise significantly reduced the mitogen-induced cell proliferation of T cells in the E3 group compared to control group (p<0.05/p<0.001 vs. control, n=5). The effect could be observed for both PHA and ConA, as shown in Figure 6.
Figure 6. Effects of acute exercise on proliferative capacity of lymphocytes. (A,B) CFSE proliferation profile; (C) statistical analysis of cell proliferation. E, the exercise mice, which were killed 3h after exercise; C, the control group; Con A: concanavalin A; PHA: phytohemagglutinin. Data are presented as mean±SEM, *p<0.05, ***p<0.001 vs. control (n=5).
The major findings of this study were that a single bout of intensive exercise caused reversible increases of both basal and agonist-induced intracellular Ca2+ concentrations during the early post-exercise recovery period. These changes could be related to an enhanced transmembrane Ca2+ influx but not to an altered load of intracellular calcium stores. However, enhanced Ca2+ transients were not transduced into an improved T cell function as indicated by an impaired cell proliferation suggesting the involvement of other intracellular signaling pathways.
The enhanced resting [Ca2+]i after acute exercise might be the results of various underlying mechanisms. Exercise stress might damage cell membranes directly by mechanical stress as shown for foot-strike hemolysis during long distance running (Fazal et al., 2017). Changes in cell membrane integrity might result also from enhanced lipid peroxidation due to enhanced exercise-associated oxidative stress. Likewise, previous data from our group have proved that intracellular Ca2+ of lymphocytes was affected transiently by acute exercise in humans (Mooren et al., 2001). However, membrane damage seems to be unlikely in the actual case as it should occur very early during and after exercise. The delayed alteration of resting [Ca2+]i (=3h post-exercise) in the current study points to another, more likely regulatory processes. The determination of Ca2+-handling proteins mRNA showed a significant reduced expression of both the plasma membrane Ca2+-ATPases (PMCA) and sarco/endoplasmic reticulum Ca2+-ATPases (SERCA). Ca2+-ATPase maintains the global basal free Ca2+ levels actively on the expense of ATP in order to transport free calcium ion against its electrochemical gradient either out of cells or into intracellular Ca2+ stores. The decreased expression of Ca2+-ATPases could mean a decreased enzyme activity and an impaired ability to regulate intracellular Ca2+ levels. The reduced Ca2+-ATPase expression and function should therefore result in a cytoplasmic Ca2+ buildup as a result of a new balance between Ca2+ increasing and decreasing processes. Previous studies showed also that the impairment in Ca2+ homeostasis just due to one of the Ca2+ extruding pathway is enough to interfere with calcium homeostasis and cell function. Therefore, defective Ca2+ extrusion due to decreased PMCA expression was responsible for an enhanced cell apoptosis (Pellegrini et al., 2007).
Azenabor and Hoffman-Goetz (2000) found that exhaustive exercise caused the increase of intracellular Ca2+ levels in murine thymocytes, and there was a continuous influx of Ca2+ after exercise when cells were monitored in Ca2+ rich medium. Intracellular Ca2+ level actually is the result of a mutual balance among Ca2+-increasing, Ca2+-decreasing, and Ca2+-buffering mechanisms (Mooren and Kinne, 1998). Exercise is obviously able to transiently disturb the balance among the Ca2+-regulating mechanisms in resting cells, which was fully reversible 24h after exercise. Beside the calcium handling machinery, however, other signaling pathways might be responsible for the dysregulation of basal [Ca2+]i after exercise. There is evidence that an activation of the transcription factor nuclear factor of activated T cells (NFAT), a key regulator of T cell activation, is followed by increased basal Ca2+ levels (Martinez et al., 2015).
Next, acute exercise was followed also by enhanced agonist-induced intracellular Ca2+ dynamics independent of the stimulant used (Con A, OKT3, and TG). In order to discriminate between the contribution of Ca2+ release from intracellular pools such as endoplasmic reticulum and Ca2+ influx via transmembrane Ca2+ channels such as store-operated calcium entry (SOCE), a unique plasma membrane Ca2+ entry mechanism, experiments were performed under Ca2+-free conditions. CRAC channels, which are formed by ORAI1-3, are the major SOCE channels in T cells; other channels, such as transient receptor potential (TRP) and voltage-dependent calcium (Cav) channels, also can participate in SOCE (Hoth, 2016). However, at least the voltage-gated Ca2+ (Cav) channels are less characterized in T cells. The results suggest that after exercise the transmembrane Ca2+ influx into T cells is the major factor for the altered Ca2+ signals. Likewise, thapsigargin (TG) by inhibiting the SERCA pumps releases similar amounts of Ca2+ from intracellular Ca2+ pools suggesting that their load wasnot affected by exercise. Furthermore, the Fura-2-quenching experiments using Mn2+ as a surrogate permeable bivalent cation for Ca2+ after application of TG support that intensive exercise could improve the transmembrane Ca2+ entry during the early (3h) post-exercise recovery period. The capacity of extracellular Ca2+ influx is thereby directly proportional to the ER store depletion (Zweifach and Lewis, 1996). The linearity of the capacity to deplete ER Ca2+ stores to determine the subsequent degree and timing of influx could offer an explanation for that the experiments performed in the calcium medium evoked a lower Ca2+ entry than those in the Ca2+-free medium. Another explanation might be the nonlinear response of the fluorescent dye especially during/after Ca2+-depleted conditions.
A challenging question is how alterations of Ca2+-handling factors fit with the changed Ca2+ transients. The downregulated expression of Ca2+-extruding pumps should delay Ca2+ extrusion resulting in enhanced Ca2+ transients as observed. The individual contribution of the Ca2+-ATPase is exquisitely coordinated in T cells in time and space in order to effectively modulate intracellular Ca2+ dynamics upon activation. PMCA has previously been shown to functionally associate with SOCE channels (Bautista et al., 2002). Thus, Ca2+ entry pathways such as TRPC1 and P2X7 have been downregulated as well. The role of purinergic ionotropic P2X7R in T cells has been studied in mice; the activation requires millimolar concentrations of its ligand ATP, which can induce the influx of Ca2+/Na+ and the efflux of K+ (Foster et al., 2013). The expression of the TRPV4, a Ca2+-permeable nonselective ion channel that is sensitive to variations of body temperature has been shown to be affected by chronic exercise in a tissue dependent manner (Chen et al., 2015). However, acute exercise as used in the actual study did not affect its expression in T cells. It might be speculated that the decreased expression of some transmembrane Ca2+ channels serves as a protective mechanism to prevent a further intracellular Ca2+ overload. In contrast, the enhanced expression of both IP3R2 and RYR2 receptors had no effects on the calcium load of intracellular stores, but it may have an amplifying effect during agonist induced Ca2+ transients. In T cells, Ca2+ release from the ER is mediated by binding of IP3 to IP3R and is further modulated by RyR (Dadsetan et al., 2008). For a proper function, SOCE requires the assembly of ER-located STIM proteins with the plasma membrane channels which occurs within distinct regions in the cell that have been termed as endoplasmic reticulum (ER)–plasma membrane (PM) junctions. The PM and ER are in close proximity to each other within this region, which allows STIM1 in the ER to interact with and activate either ORAI1 or TRPC1 in the plasma membrane (Subedi et al., 2017). Higher expression of intracellular Ca2+ release channels might therefore modulate Ca2+ permeability and enhance transmembrane Ca2+ influx despite lower expression of Ca2+ entry pathways. Compared to our recent publication about the effects of regular long-term exercise training on T cell and Ca2+ signaling, similarities and dichotomies were found. Exercise training had no effect on the expression of Ca2+ extruding pumps from both cell membrane and intracellular stores. Ca2+ channel proteins from cell membrane and intracellular stores, such as ORAI1, IP3R2, TRPM5, TRPC1, and TRPV4 Ca2.3 were broadly downregulated (Liu et al., 2017). In contrast, acute exercise induced upregulation of some Ca2+ channels such as the intracellular channels IP3R2 and RYR2 and some transmembrane Ca2+ channels like Cav2.3 and TRPC1 suggesting a differential regulation of Ca2+ signaling in T cells by acute and chronic exercise. The enhanced expression of Cav2.3 occurred delayed 24h after exercise without any influence on cellular Ca2+ signals. Recently, the Cav2.3 gene was shown to be upregulated in pregnancy and type 1 diabetes indicating a shift in immunological status under these conditions (Bhandage et al., 2018).
What might be the exercise-associated condition which modulates transiently intracellular calcium? Previous work has demonstrated that levels of oxidative stress are increased after intensive bouts of exercise (Kruger et al., 2009). Moreover, it has been well documented that free radicals are able to modulate the activity of many Ca2+-handling proteins (Gorlach et al., 2015), which may result in changed capacitative Ca2+ entry and finally in intracellular Ca2+ overload. The function of PMCA can be altered by an enhanced lipid peroxidation (Kako et al., 1988; Ohta et al., 1989), and SERCA is highly susceptible to oxidative damage, which can lead to a decrease of SERCA activity and then result in elevation of intracellular Ca2+ level (Antipenko and Kirchberger, 1997; Squier and Bigelow, 2000). On the other hand, the elevation of cytosolic Ca2+ level can activate the mitochondrial Ca2+ uniporter (MCU) that induces mitochondrial Ca2+ uptake, an event that drives mitochondrial respiration thereby causing the generation of further free radicals, which may amplify the cellular oxidative stress. A Ca2+ overload in mitochondrial matrix causes the opening of the permeability transition pore (PTP) followed by a collapse of mitochondrial potential, mitochondrial swelling and release of cell death inducing agents (Gherardi et al., 2020). Such sequence finally leads to apoptotic cell death, which recently was described to be induced after exhaustive exercise (Kruger et al., 2009).
Finally, acute exhaustive exercise can modulate the ability of T cell proliferation. Many studies have shown that an acute bout of strenuous exercise is followed by reduced immune cells functions in humans and mice (Randall Simpson et al., 1989; Mazzeo et al., 1998; Potteiger et al., 2001). The results of this study confirmed these results in the murine model by demonstrating a reduced proliferative response of splenic lymphocytes to mitogens. However, after acute exercise, the increased intracellular Ca2+ signals were not transferred into an enhanced cellular function contrary to our recent findings after chronic exercise training. Chronic moderate exercise elevated basal Ca2+ level and agonist-induced Ca2+ influx, which was followed by an improved lymphocyte proliferation (Liu et al., 2017). These contrary findings suggest that after acute exercise the usual stimulus–response relationship is broken at least for cell proliferation as one of several T cell functions. One reason might be that exercise induces alterations of spatial and temporal Ca2+ patterns such as calcium oscillations, which could not be recognized using the actual technical approach. The increasing [Ca2+]i per se is not enough to trigger cell proliferation (Silberman et al., 2005). The Ca2+ signaling promotes cellular proliferation depending on the amplitude of increase, frequency of oscillations, the duration and nature of change, and the location of cytosolic Ca2+ responses (Monteith et al., 2007; Brini and Carafoli, 2009). On the other hand, cellular proliferation ability is controlled also by other signaling pathways than [Ca2+]i, such as transcription factors like cAMP response element-binding protein (CREB), AP-1, and NFAT (Dolmetsch et al., 1998; Martinez et al., 2015; Shin et al., 2019). T cells show hyporesponsive states during conditions of persistent stimulation, referred to as cellular exhaustion. There is evidence that such a hypo-response does not affect all cellular functions to the same degree. Moreover, impaired cellular proliferation occurs despite preserved intracellular Ca2+ signaling (Agnellini et al., 2007). NFAT could be identified as a major factor responsible for promoting exhaustion of activated T cells (Martinez et al., 2015). Further studies should therefore focus on the exercise-dependent regulation of cellular transcription factors. Another limitation of the current study includes the investigation of cell suspensions and not single cells. The latter approach would enable the detection of individual calcium signals and patterns and the use of the patch clamp technique for electrophysiological measurements. With the development of molecular biology cloning technology, we have gradually learned that there are great similarities of molecular signatures between humans and animals, but there are also certain differences. Although it is tempting to extrapolate results from one species to another, doing such without assessing the differences of the various ion channels, such as calcium and potassium channels, in different species brings risks, as translation is not always that simple (Tanner and Beeton, 2018).
Thus, taken together, our findings demonstrated that acute exercise affects intracellular calcium homeostasis during resting and stimulated conditions in murine T cells. The changed calcium levels result from an enhanced transmembrane Ca2+ influx into cells and an altered expression of cellular Ca2+ ATPases such as PMCA and SERCA as well as intracellular Ca2+ release channels such as IP3R and RyR. However, the alterations of Ca2+ signaling could not be related to the inhibition of cell proliferation in T cells making further studies on the role of additional signaling pathways such as transcription factor activation necessary.
The original contributions presented in the study are included in the article/supplementary material, further inquiries can be directed to the corresponding author.
The animal study was reviewed and approved by the Animal Care and Use Committee of Giessen University.
All authors have made substantial contributions to the conception or design of the work or the acquisition, analysis, or interpretation of data for the work, drafted the work or revised it critically for important intellectual content, approved the final version to be published, and agreed to be accountable for all aspects of the work in ensuring that questions related to the accuracy or integrity of any part of the work are appropriately investigated and resolved. All authors contributed to the article and approved the submitted version.
The authors declare that the research was conducted in the absence of any commercial or financial relationships that could be construed as a potential conflict of interest.
All claims expressed in this article are solely those of the authors and do not necessarily represent those of their affiliated organizations, or those of the publisher, the editors and the reviewers. Any product that may be evaluated in this article, or claim that may be made by its manufacturer, is not guaranteed or endorsed by the publisher.
Agnellini, P., Wolint, P., Rehr, M., Cahenzli, J., Karrer, U., and Oxenius, A. (2007). Impaired NFAT nuclear translocation results in split exhaustion of virus-specific CD8+ T cell functions during chronic viral infection. Proc. Natl. Acad. Sci. U. S. A. 104, 4565–4570. doi: 10.1073/pnas.0610335104
Antipenko, A. Y., and Kirchberger, M. A. (1997). Membrane phosphorylation protects the cardiac sarcoplasmic reticulum Ca2+-ATPase against chlorinated oxidants in vitro. Cardiovasc. Res. 36, 67–77. doi: 10.1016/S0008-6363(97)00183-1
Azenabor, A. A., and Hoffman-Goetz, L. (2000). Effect of exhaustive exercise on membrane estradiol concentration, intracellular calcium, and oxidative damage in mouse thymic lymphocytes. Free Radic. Biol. Med. 28, 84–90. doi: 10.1016/S0891-5849(99)00209-9
Bautista, D. M., Hoth, M., and Lewis, R. S. (2002). Enhancement of calcium signalling dynamics and stability by delayed modulation of the plasma-membrane calcium-ATPase in human T cells. J. Physiol. 541, 877–894. doi: 10.1113/jphysiol.2001.016154
Bhandage, A. K., Jin, Z., Korol, S. V., Tafreshiha, A. S., Gohel, P., Hellgren, C., et al. (2018). Expression of calcium release-activated and voltage-gated calcium channels genes in peripheral blood mononuclear cells is altered in pregnancy and in type 1 diabetes. PLoS One 13:e0208981. doi: 10.1371/journal.pone.0208981
Brini, M., and Carafoli, E. (2009). Calcium pumps in health and disease. Physiol. Rev. 89, 1341–1378. doi: 10.1152/physrev.00032.2008
Chen, N., Cheng, J., Zhou, L., Lei, T., Chen, L., Shen, Q., et al. (2015). Effects of treadmill running and rutin on lipolytic signaling pathways and TRPV4 protein expression in the adipose tissue of diet-induced obese mice. J. Physiol. Biochem. 71, 733–742. doi: 10.1007/s13105-015-0437-5
Dadsetan, S., Zakharova, L., Molinski, T. F., and Fomina, A. F. (2008). Store-operated Ca2+ influx causes Ca2+ release from the intracellular Ca2+ channels that is required for T cell activation. J. Biol. Chem. 283, 12512–12519. doi: 10.1074/jbc.M709330200
Dolmetsch, R. E., Xu, K., and Lewis, R. S. (1998). Calcium oscillations increase the efficiency and specificity of gene expression. Nature 392, 933–936. doi: 10.1038/31960
Fazal, A. A., Whittemore, M. S., and Degeorge, K. C. (2017). Foot-strike haemolysis in an ultramarathon runner. BMJ Case Rep. 2017:bcr2017220661. doi: 10.1136/bcr-2017-220661
Ferreira, V. M., Gomes, T. S., Reis, L. A., Ferreira, A. T., Razvickas, C. V., Schor, N., et al. (2009). Receptor-induced dilatation in the systemic and intrarenal adaptation to pregnancy in rats. PLoS One 4:e4845. doi: 10.1371/journal.pone.0004845
Foster, J. G., Carter, E., Kilty, I., Mackenzie, A. B., and Ward, S. G. (2013). Mitochondrial superoxide generation enhances P2X7R-mediated loss of cell surface CD62L on naive human CD4+ T lymphocytes. J. Immunol. 190, 1551–1559. doi: 10.4049/jimmunol.1201510
Gherardi, G., Monticelli, H., Rizzuto, R., and Mammucari, C. (2020). The mitochondrial Ca2+ uptake and the fine-tuning of aerobic metabolism. Front. Physiol. 11:554904. doi: 10.3389/fphys.2020.554904
Gorlach, A., Bertram, K., Hudecova, S., and Krizanova, O. (2015). Calcium and ROS: A mutual interplay. Redox Biol. 6, 260–271. doi: 10.1016/j.redox.2015.08.010
Grynkiewicz, G., Poenie, M., and Tsien, R. Y. (1985). A new generation of Ca2+ indicators with greatly improved fluorescence properties. J. Biol. Chem. 260, 3440–3450. doi: 10.1016/S0021-9258(19)83641-4
Hoth, M. (2016). CRAC channels, calcium, and cancer in light of the driver and passenger concept. Biochim. Biophys. Acta 1863, 1408–1417. doi: 10.1016/j.bbamcr.2015.12.009
Kako, K., Kato, M., Matsuoka, T., and Mustapha, A. (1988). Depression of membrane-bound Na+-K+-ATPase activity induced by free radicals and by ischemia of kidney. Am. J. Phys. 254, C330–C337. doi: 10.1152/ajpcell.1988.254.2.C330
Kruger, K., Frost, S., Most, E., Volker, K., Pallauf, J., and Mooren, F. C. (2009). Exercise affects tissue lymphocyte apoptosis via redox-sensitive and Fas-dependent signaling pathways. Am. J. Phys. Regul. Integr. Comp. Phys. 296, R1518–R1527. doi: 10.1152/ajpregu.90994.2008
Krüger, K., Lechtermann, A., Fobker, M., Völker, K., and Mooren, F. C. (2008). Exercise-induced redistribution of T lymphocytes is regulated by adrenergic mechanisms. Brain Behav. Immun. 22, 324–338. doi: 10.1016/j.bbi.2007.08.008
Liu, R., Fan, W., Kruger, K., Xiao, Y. U., Pilat, C., Seimetz, M., et al. (2017). Exercise affects T-cell function by modifying intracellular calcium homeostasis. Med. Sci. Sports Exerc. 49, 29–39. doi: 10.1249/MSS.0000000000001080
Martinez, G. J., Pereira, R. M., Aijo, T., Kim, E. Y., Marangoni, F., Pipkin, M. E., et al. (2015). The transcription factor NFAT promotes exhaustion of activated CD8+ T cells. Immunity 42, 265–278. doi: 10.1016/j.immuni.2015.01.006
Mazzeo, R. S., Rajkumar, C., Rolland, J., Blaher, B., Jennings, G., and Esler, M. (1998). Immune response to a single bout of exercise in young and elderly subjects. Mech. Ageing Dev. 100, 121–132. doi: 10.1016/S0047-6374(97)00130-9
Monteith, G. R., Mcandrew, D., Faddy, H. M., and Roberts-Thomson, S. J. (2007). Calcium and cancer: targeting Ca2+ transport. Nat. Rev. Cancer 7, 519–530. doi: 10.1038/nrc2171
Mooren, F. C., and Kinne, R. K. (1998). Cellular calcium in health and disease. Biochim. Biophys. Acta 1406, 127–151. doi: 10.1016/s0925-4439(98)00006-4
Mooren, F. C., Lechtermann, A., Fromme, A., Thorwesten, L., and Volker, K. (2001). Alterations in intracellular calcium signaling of lymphocytes after exhaustive exercise. Med. Sci. Sports Exerc. 33, 242–248. doi: 10.1097/00005768-200102000-00012
Ohta, A., Mohri, T., and Ohyashiki, T. (1989). Effect of lipid peroxidation on membrane-bound Ca2+-ATPase activity of the intestinal brush-border membranes. Biochim. Biophys. Acta 984, 151–157. doi: 10.1016/0005-2736(89)90210-1
Pellegrini, M., Finetti, F., Petronilli, V., Ulivieri, C., Giusti, F., Lupetti, P., et al. (2007). p66SHC promotes T cell apoptosis by inducing mitochondrial dysfunction and impaired Ca2+ homeostasis. Cell Death Differ. 14, 338–347. doi: 10.1038/sj.cdd.4401997
Potteiger, J. A., Chan, M. A., Haff, G. G., Mathew, S., Schroeder, C. A., Haub, M. D., et al. (2001). Training status influences T-cell responses in women following acute resistance exercise. J. Strength Cond. Res. 15, 185–191.
Quah, B. J., and Parish, C. R. (2010). The use of carboxyfluorescein diacetate succinimidyl ester (CFSE) to monitor lymphocyte proliferation. J. Vis. Exp. :2259. doi: 10.3791/2259
Randall Simpson, J. A., Hoffman-Goetz, L., Thorne, R., and Arumugam, Y. (1989). Exercise stress alters the percentage of splenic lymphocyte subsets in response to mitogen but not in response to interleukin-1. Brain Behav. Immun. 3, 119–128. doi: 10.1016/0889-1591(89)90012-3
Sage, S. O., Merritt, J. E., Hallam, T. J., and Rink, T. J. (1989). Receptor-mediated calcium entry in fura-2-loaded human platelets stimulated with ADP and thrombin. Dual-wavelengths studies with Mn2+. Biochem. J. 258, 923–926. doi: 10.1042/bj2580923
Shin, S. Y., Kim, M. W., Cho, K. H., and Nguyen, L. K. (2019). Coupled feedback regulation of nuclear factor of activated T-cells (NFAT) modulates activation-induced cell death of T cells. Sci. Rep. 9:10637. doi: 10.1038/s41598-019-46592-z
Silberman, D. M., Zorrilla-Zubilete, M., Cremaschi, G. A., and Genaro, A. M. (2005). Protein kinase C-dependent NF-kappaB activation is altered in T cells by chronic stress. Cell. Mol. Life Sci. 62, 1744–1754. doi: 10.1007/s00018-005-5058-7
Simpson, R. J., Campbell, J. P., Gleeson, M., Krüger, K., Nieman, D. C., Pyne, D. B., et al. (2020). Can exercise affect immune function to increase susceptibility to infection? Exerc. Immunol. Rev. 26, 8–22.
Squier, T. C., and Bigelow, D. J. (2000). Protein oxidation and age-dependent alterations in calcium homeostasis. Front. Biosci. 5, D504–D526. doi: 10.2741/squier
Subedi, K. P., Ong, H. L., and Ambudkar, I. S. (2017). Assembly of ER-PM junctions: A critical determinant in the regulation of SOCE and TRPC1. Adv. Exp. Med. Biol. 981, 253–276. doi: 10.1007/978-3-319-55858-5_11
Tanner, M. R., and Beeton, C. (2018). Differences in ion channel phenotype and function between humans and animal models. Front. Biosci. 23, 43–64. doi: 10.2741/4581
Keywords: acute exercise, Fura-2(AM), intracellular Ca2+, calcium homeostasis, cell proliferation, calcium channels
Citation: Liu R, Krüger K, Pilat C, Fan W, Xiao Y, Seimetz M, Ringseis R, Baumgart-Vogt E, Eder K, Weissmann N and Mooren FC (2021) Excessive Accumulation of Intracellular Ca2+ After Acute Exercise Potentiated Impairment of T-cell Function. Front. Physiol. 12:728625. doi: 10.3389/fphys.2021.728625
Received: 21 June 2021; Accepted: 18 October 2021;
Published: 26 November 2021.
Edited by:
Ildikò Szabò, University of Padua, ItalyReviewed by:
Ameet A. Chimote, University of Cincinnati, United StatesCopyright © 2021 Liu, Krüger, Pilat, Fan, Xiao, Seimetz, Ringseis, Baumgart-Vogt, Eder, Weissmann and Mooren. This is an open-access article distributed under the terms of the Creative Commons Attribution License (CC BY). The use, distribution or reproduction in other forums is permitted, provided the original author(s) and the copyright owner(s) are credited and that the original publication in this journal is cited, in accordance with accepted academic practice. No use, distribution or reproduction is permitted which does not comply with these terms.
*Correspondence: Frank Christoph Mooren, ZnJhbmsubW9vcmVuQHVuaS13aC5kZQ==
Disclaimer: All claims expressed in this article are solely those of the authors and do not necessarily represent those of their affiliated organizations, or those of the publisher, the editors and the reviewers. Any product that may be evaluated in this article or claim that may be made by its manufacturer is not guaranteed or endorsed by the publisher.
Research integrity at Frontiers
Learn more about the work of our research integrity team to safeguard the quality of each article we publish.