- 1Laboratory of Comparative Physiology of Pigmentation, Department of Physiology, Institute of Biosciences, University of São Paulo, São Paulo, Brazil
- 2Laboratory of Neurobiology, Department of Physiology and Biophysics, Institute of Biomedical Sciences, University of São Paulo, São Paulo, Brazil
- 3Center of Brain, Behavior and Metabolism, Institute of Neurobiology, Lübeck University, Lübeck, Germany
- 4Department of Biology, University of Virginia, Charlottesville, VA, United States
The control of the biological rhythms begins with the activation of photo- and thermosensitive cells located in various organs of the fish such as brain, eye, and skin, but a central clock is still to be identified in teleosts. Thermal changes are stressors which increase cortisol and affect the rhythm of other hormones such as melatonin and growth hormone (GH), in both endo- and ectothermic organisms. Our aim was to investigate how temperature (23°C for 6 days) lower than the optimal (28°C) modulates expression of several gene pathways including growth hormone (gh1) and its receptors (ghra, ghrb), insulin-like growth factor1 (igf1a, igf1b) and its receptors (igf1ra, igf1rb), cortisol and its receptor (gr), the limiting enzyme of melatonin synthesis (arylalkylamine N-acetyltransferase, aanat) and melatonin receptors (mtnr1aa, mtnr1bb), as well as their relationship with clock genes in Danio rerio in early light and early dark phases of the day. Lower temperature reduced the expression of the hormone gene gh1, and of the related receptors ghra, ghrb, igf1ra, and igf1rb. Cortisol levels were higher at the lower temperature, with a decrease of its receptor (gr) transcripts in the liver. Interestingly, we found higher levels of aanat transcripts in the brain at 23°C. Overall, lower temperature downregulated the transcription of hormone related genes and clock genes. The results suggest a strong correlation of temperature challenge with the clock molecular mechanism and the endocrine systems analyzed, especially the growth hormone and melatonin axes, in D. rerio tissues.
Introduction
A variety of physiological and biochemical processes displays a daily rhythmic profile which is adjusted by environmental factors such as temperature and light, major temporal cues and first order zeitgebers (time-giver in German) (Aschoff, 1965; West and Bechtold, 2015). In the natural environment, temperature has a close relationship with the light-dark cycles, as the thermo-phase (high temperature) usually coincides with the photo-phase, and the cryo-phase (low temperature) coincides with the scoto-phase (López-Olmeda and Sánchez-Vázquez, 2011).
Early studies in Danio rerio showed that oscillations in the clock components were very similar to the mouse, and occurred in all tissues examined, both in vivo and in vitro (Lahiri et al., 2005). However, it’s important to notice that the peak of clock genes occur in antiphase in these species. In addition to the canonical light effects, temperature variation also promotes changes in the clock molecular core of D. rerio tissues and cells (Lahiri et al., 2005; Jerônimo et al., 2017).
In D. rerio, the protein products of clock and bmal1 genes (peak at dusk) activate another group of clock genes and clock-controlled genes, through the binding of Clock:Bmal heterodimers to E-box elements in the target genes, including period (per) and cryptochrome (cry). The genes per and cry are transcribed (peak at dawn), translated and transported into the nucleus as Per:Cry heterodimers which inhibit the transcription of Clock:Bmal complex. This complex is reactivated after degradation of Per:Cry. This cycle is adjusted to a 24-h period by the light-dark cycle and represents the molecular mechanism by which the organism keeps track of time (Lahiri et al., 2005; Vatine et al., 2011). The rhythm amplitude of some clock gene expression in D. rerio is strongly influenced by the temperature (Kaneko and Cahill, 2005; Vallone et al., 2005; López-Olmeda and Sánchez-Vázquez, 2011; Jerônimo et al., 2017). In developing larvae as well as zebrafish cell lines, temperature cycles of as little as 2°C difference, or temperature pulses, are able to entrain the circadian rhythms of clock genes (Vallone et al., 2005) and increase per1, per2 and cry1, cry2 expression (Jerônimo et al., 2017). Endocrine factors are a mainstay of circadian biology. Their synthesis, secretion and also the sensitivity of the target organs to these signals are subject to a rigorous and often predictable control dependent on the time of day. Among the best-studied examples of endocrine factors that fluctuate over the course of the day are those whose production is governed by hypothalamic-pituitary axes, such as growth hormone (GH). In fish, Gh production is mainly stimulated by pituitary adenylate cyclase activating polypeptide (PACAP), which belongs to the same superfamily of neuropeptides as mammal GH-releasing hormone (GHRH) (Cowan et al., 2017). The current knowledge of fish physiology shows that their growth is dependent on photoperiod and temperature (Boeuf and Falcón, 2001). Growth-promoting effects are mostly mediated by IGFs (insulin-like growth factors), mainly IGF-1, which favor proliferation, differentiation, survival and cell migration (Butler and LeRoith, 2001; Reindl et al., 2011).
In addition, other hormones like glucocorticoids, vasopressin, adrenocorticotropic hormone, and thyrotropin exhibit pronounced circadian rhythms (Hastings et al., 2007). The endocrine and nervous systems respond together to a diversity of internal and external stimuli in fish. One of them is stress. It is worth mentioning that the stress caused by anthropological causes and environmental conditions (as temperature, food availability, climate seasonality, and photoperiod) affect the metabolism of the animal and generate adverse results such as development delay (Faught and Vijayan, 2016). In teleost fish, the major neuroendocrine circuit involved in stress response is the hypothalamic-pituitary-interrenal (HPI) axis. The cortisol is synthesized in the interrenal cells, and its release is part of the endocrine response evoked by stress, together with catecholamine and pro-opiomelanocortin secretion. Cortisol rhythms are endogenously driven by a circadian pacemaker, because they persist under constant environmental conditions (Auró de Ocampo and Ocampo, 1999; Cowan et al., 2017). Once in the cells, cortisol binds to the glucocorticoid receptor (GR) and ultimately modulates gene expression (Faught et al., 2016). The fish cortisol upregulates pathways involved in energy substrate mobilization, including gluconeogenesis, while downregulating energy demanding pathways, including growth and immune function (Faught and Vijayan, 2016). A glucocorticoid response element (GRE) was identified in the promoter of Per1 gene suggesting a direct genomic action of glucocorticoids on the molecular clock components (Yamamoto et al., 2005).
In D. rerio and other teleost fish, besides the retina and peripheral tissues, the pineal gland cells are also capable of sensing the light (Davies et al., 2015) and are core components of the circadian system (Doyle and Menaker, 2007; Falcón et al., 2007). The pineal gland contains an intrinsic circadian clock that favors the rhythmic synthesis of melatonin. In teleosts, as well as other vertebrates, melatonin participates in the control of physiological process such as growth, food intake and digestion, reproduction, in addition to antioxidant activity and regulation of the immune system (Ngasainao and Lukram, 2016). Melatonin levels are higher in the dark due to light inhibition of the transcription and the stability of arylalkylamine N-acetyltransferase (aanat), an enzyme required for the hormone synthesis (Vatine et al., 2011). It is well established that melatonin counteracts stress-induced elevations of glucocorticoids directly on adrenal tissue, as reported in mammals and also suggested in fish, where peripheral (intraperitoneal) but not central (intracerebroventricular) melatonin injection is able to reduce cortisol secretion (Torres-Farfan et al., 2003; Azpeleta et al., 2010). Melatonin ability to reduce stress in teleosts has been usually associated with simultaneous changes in brain serotoninergic activity (Gesto et al., 2016; Sánchez-Vázquez et al., 2019). In addition, melatonin seems to modulate the GH production directly (on the pituitary gland) or indirectly (on the hypothalamus) (Boeuf and Falcón, 2001). After the teleost-specific whole-genome duplication, D. rerio has two distinct genes, aanat1 and aanat2, respectively more expressed in the retina and the pineal gland (Vatine et al., 2011). Melatonin receptors also display a circadian rhythm (Quera and Hartley, 2012), and were identified as mtnr1aa, mtnr1bb, mtnr1ab, mtnr1al, and mtnr1c in D. rerio. It is well known that changes in temperature significantly affect the melatonin rhythm in ectothermic organisms (López-Olmeda and Sánchez-Vázquez, 2011).
Considering the effects of temperature in fish physiology, our aim was to investigate how temperature (23°C for 6 days), lower than the optimal (28°C), modulates expression of several gene pathways including growth hormone (gh1) and its receptors (ghra, ghrb), insulin-like growth factor 1 (igf1a, igf1b) and its receptors (igf1ra, igf1rb), cortisol and its receptor (gr), the limiting enzyme of melatonin synthesis (arylalkylamine N-acetyltransferase, aanat) and melatonin receptors (mtnr1aa, mtnr1bb), as well as their relationship with clock genes in D. rerio in early light and early dark phases of the day.
Materials and Methods
Animals
Danio rerio AB strain was bred in the zebrafish facility of the Department of Genetics and Evolutionary Biology in the Institute of Biosciences, University of São Paulo IB-USP, and donated to the Department of Physiology, IB-USP. Animals were maintained in glass tanks at 28 ± 1°C under photoperiod regimen of 14 h light and 10 hours dark (14:10 LD, lights on at 8:00 AM, ∼1000 lux) until reached ∼3 months of age. The fish were randomly fed three times a day with Artemia sp. (Artemia cyst, INVE, Salt Lake City, UT, United States, raised according to protocol established in the laboratory), in addition to commercial adult food (Zeigler® Larval AP100, Gardners, PA, United States). The Central Vivarium of the Faculty of Medicine FM-USP (protocol 4172) carried out the sanitary control. The nomenclature used in this article follows the conventions established in: https://zfin.atlassian.net/wiki/spaces/general/pages/1818394635/ZFIN+Zebrafish+Nomenclature+Conventions.
Experimental Protocol
This was a two-factor experimental design aiming to compare the responses to two temperatures (28 and 23oC) at two times of day [early light phase EL, 2 h after lights on, 10 AM, and early dark phase ED, 2 h after lights off, 12 AM (Figure 1)]. Adult males (n = 144, ∼0.150 g and ∼90 days old) were placed in a water recirculating system in two BOD (Bio-Oxygen Demand) incubators (Jobilnet, São Paulo, Brazil). Each incubator had a capacity for six 7 L-tanks (three tanks per temporal point), and a total water flow of 60 L. Physical (grid) and biological (Bio Filter Quartz Ceramic Ring, China) filters were used, and two thermostats were placed to ensure constant temperature. Each tank had a blue background according to Oliveira et al. (2015) and received light intensity of ∼1000 lux (white light, 420–750 nm) measured with the aid of a lux meter (LX-102, Lutron Electronic Enterprise, Taipei, Taiwan). The pH of the water was evaluated every 3 days using the LabconTest-pH-Tropical and adjusted, when necessary, with Labcon-Acid and or Labcon-Alcali (Alcon®, Camboriú, Santa Catarina, Brazil).
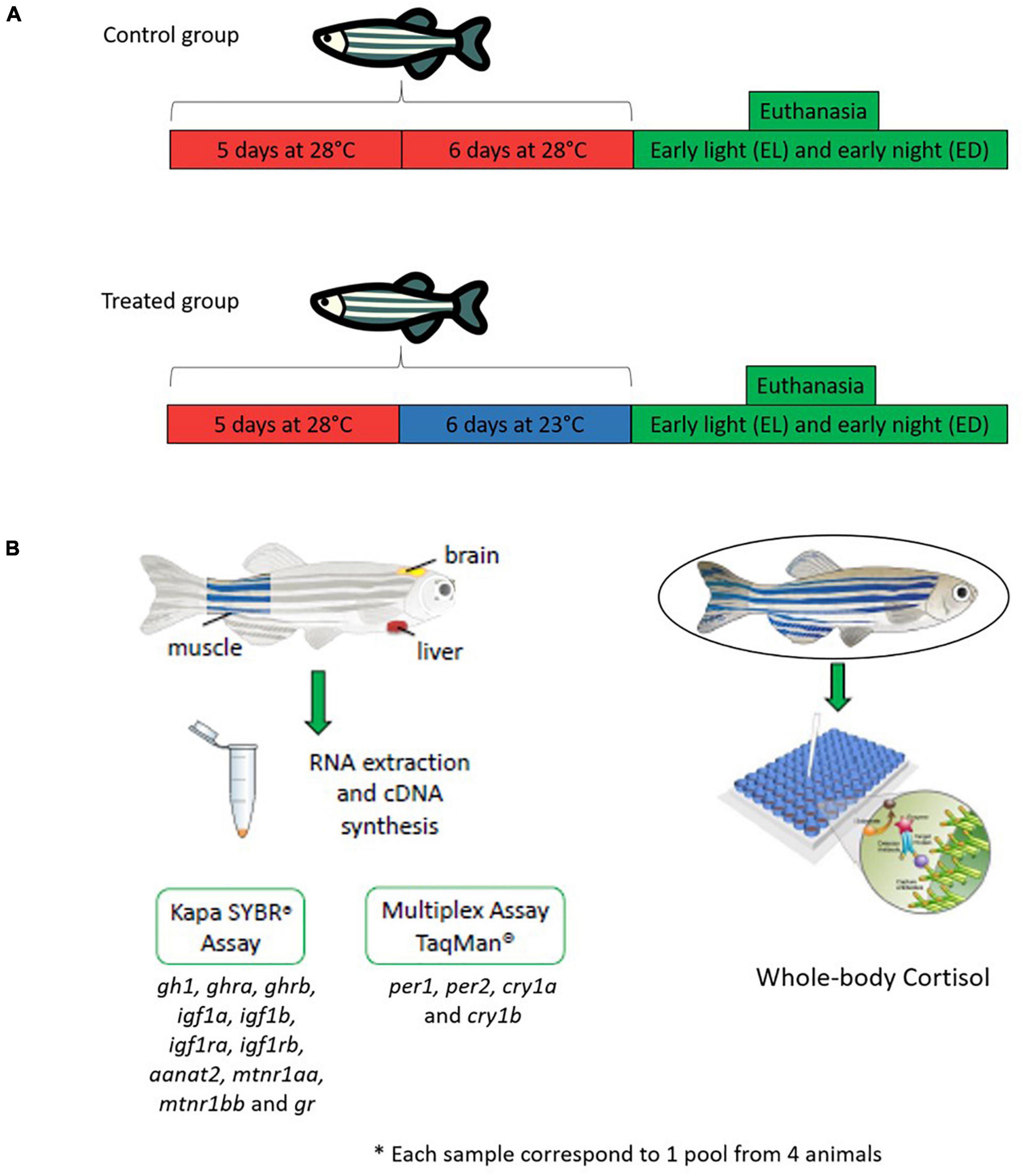
Figure 1. Experimental design. (A) Danio rerio male adults (n = 144) were divided in two large groups: a control temperature group (animals maintained at 28oC during 5 days and challenged 6 days at the same temperature) and a lower temperature group (animals maintained at 28oC during 5 days and challenged 6 days at lower temperature 23oC). After stimulation the animals were euthanized at early light (EL, 10 AM) and early dark (ED, 12 AM). (B) For the analysis, fish were separated into another two groups: a qPCR (96 animals) and a cortisol (48 animals) group. For the qPCR we evaluated gh1, aanat2, mtnr1aa, mtnr1bb, opn4m1, opn4m2 in the brain, ghra, ghrb, igf1a, igf1b, igf1ra, igf1rb, gr in the liver and muscle. Also, in all tissues analyzed we performed a qPCR for clock genes (per and cry). Each experimental n comprised pooled tissues from four fish. The total number of samples used in each temperature and time point was n = 3–6.
For the control group we placed and maintained 4 animals per tank for 11 days at 28°C (5 days of acclimation and 6 days of experimental period). For the treated group we also placed and maintained four animals per tank for 5 days (acclimation at 28°C) and then all the animals were transferred to an incubator at 23°C for 6 days (Figure 1A). At the end, the animals were euthanized by cryo-anesthesia (5 parts ice/1 part water, 0–4°C, during circa 30 s until the fish stopped its swimming movements), at EL (10 AM) and ED (12 AM). In order to avoid the postprandial peak of cortisol, the fish were not fed on the day of sampling. To obtain the brain, the head of the fish was removed and a dorsal cut was made from cranial to caudal, exposing the organ which was collected whole. To excise the liver, a ventral lengthwise cut exposed the abdominal organs, and the liver was then separated from the gastrointestinal tract. The muscle was excised from the caudal region of the fish by crosswise slicing, after isolating the skin and discarding the spine. The number of fish used for each experiment is described below and detailed in Supplementary Table S1. Each sample was a pool of four organs from four animals kept at each temperature and euthanized at two time points. Each experiment was independently repeated twice.
All procedures were performed according to the Ethics Committee on Use of Animals (CEUA) of the Institute of Biosciences, University of São Paulo, protocol N° 331/2018.
Total RNA Extraction and Reverse Transcriptase Reaction
The quantitative polymerase chain reaction (qPCR) group consisted of 96 animals (n = 48 for each temperature). To obtain the samples, the brain, liver and muscle were removed from four fish and pooled. The final number of pooled samples was n = 3–6 for each temperature and temporal point (Figure 1B). The samples were stored at –80°C until processing, and then homogenized with sterile pestles in a final volume of 1 mL of Trizol (Ambion, Foster City, CA, United States). RNA extraction and purification were performed according to the manufacturer’s instruction. To prevent DNA contamination, each sample was treated with DNase I (turbo DNA-free™, Ambion, Foster City, CA, United States). The RNA concentration and quality were determined using a spectrophotometer (ND-1000 Spectrophotometer, NanoDrop, Wilmington, DE, United States). The cDNA synthesis was performed using 1 μg RNA, Superscript III, random hexamer primer and the reagents recommended by the manufacturer (ThermoFisher, Waltham, CA, United States). The samples containing the cDNA were stored at 4°C.
Quantitative Polymerase Chain Reaction
As a reference gene for all organs 18S ribosomal RNA was chosen, tested, and validated for the stability of its expression in the various experimental conditions (data not shown). Housekeeping gene values were accepted as stables when the standard deviation (SD) < 1 across time points and temperature groups. The final concentrations of primers and probes were, respectively, 300 and 200 nM for the genes of interest, and 50 nM for primers and probe of 18S rRNA. In all assays, 50 ng of cDNA were used, and depending on the gene of interest, the samples were subject to multiplex reaction in two groups: per1-cry1b genes and per2-cry1a genes. In these reactions, KAPA PROBE FAST 2 mix (Kapa Biosystems, Wilmington, MA, United States) and the respective primers and probes were used. The remaining genes (Table 1) were individually evaluated in duplicates, using KAPA SYBR® Fast qPCR Mix for iCycler® 2x (Kapa Biosystems, United States). For the qPCR we evaluated gh1, aanat2, mtnr1aa, mtnr1bb, opn4m1, opn4m2 in the brain, ghra, ghrb, igf1a, igf1b, igf1ra, igf1rb, gr in the liver and muscle. Also, in all tissues analyzed we performed a qPCR for clock genes (per and cry).
Primers and probes were designed with the PrimerBlast program or Integrated DNA Technologies (IDT, Coralville, IA, United States), according to GenBank1 sequences (Table 1), and synthesized by ThermoFisher (Waltham, CA, United States) (hormone receptor genes) or IDT (clock and gh1 gene). Primers’ efficiencies were determined using serial dilutions (1, 1:2, 1:4, 1:8, and 1:16) of single cDNA samples. The efficiency for each primer pair was calculated according to the equation: 10∧ (−1/x)−1(100), in which x corresponds to the slope of the linear regression curve. Values between 90 and 110% were considered as indicators of appropriate efficiency.
All assays were performed in a thermocycler iQ5 (BioRad Laboratories, Hercules, CA, United States) under the following conditions: SYBR® GreenER™ – 10 min at 95°C, followed by 45 cycles of 15 s at 95°C, 1 min at 60°C and 80 cycles of 10 s at 55°C, with gradual increase of 0.5°C; for the multiplex reaction – 3 min at 95°C followed by 45 cycles of 15 s at 95°C and 60 s at 60°C.
Gene expression was quantified according to the 2–Δ Δ CT method (Livak and Schmittgen, 2001). Cycle threshold (CT) for each reaction was determined as the number of cycles of the geometric portions of the amplification curves crossed by the threshold line. The ΔCT was determined by subtracting the 18S RNA CT from the CT of the gene of interest at the same time point and temperature, both corresponding to the average of replicates of the same cDNA sample. The mean value obtained from control temperature (28°C) at EL was subtracted from all other values, obtaining the ΔΔCT, which was used as the negative exponential of base 2 (2–Δ Δ CT). Results were expressed as mean ± standard error of the mean (SEM), and fold change was calculated as transcripts of A/transcripts of B between experimental conditions.
Quantitative Determination of Total Cortisol (ELISA Kit)
The procedure of cortisol extraction was adapted from Alderman and Bernier (2009) and Egan et al. (2009). The cortisol group consisted of 48 animals (n = 24 for each temperature). To obtain the samples, the animals were euthanized at EL and ED, weighed, and placed in 2 mL plastic tubes. Each sample corresponded to a pool of four animals. The final number of pooled samples was n = 3 for each temperature and temporal point (Figure 1B). The samples were homogenized in 500 μL of ice-cold 1X PBS buffer using an X1000 Homogenizer Drive (CAT Scientific, Paso Robles, CA, United States), and processed according to the kit instructions (Salivary Cortisol ELISA kit, Salimetrics, Carlsbad, CA, United States). The optical densities (OD) for the duplicate wells were averaged, the non-specific binding OD was subtracted from all wells, and the percent bound was calculated dividing the mean OD of each duplicate (B) by the mean of zero (Bo). The whole-body cortisol concentrations were interpolated in the standard curve using a 4-parameter non-linear regression curve fit (if r > 0.99), normalized with the weight of each sample (each one a pool of four animals) and reported as cortisol concentrations (ng/g body weight) (according to Egan et al., 2009). Results were expressed as mean ± standard error of the mean (SEM).
Statistical Analysis
The log values of qPCR and the hormone concentrations, in the control temperature (28oC) and treated (23oC) groups, in both time points, were tested for normality and compared to each other by two-way ANOVA. To evaluate the interaction between factors (time point and temperature) the F value was analyzed [F(DFn, DFd), p < 0.05], followed by a Bonferroni’s test to compare the temporal points or temperatures factor, considering significant differences when p < 0.05.
For the correlation analysis, the log values of qPCR (2–Δ Δ CT) were analyzed by Pearson correlation coefficients, with a multiple comparison test, and significance set for p < 0.05. The correlation coefficient, r, ranges from –1 to +1, assuming + 1 as perfect correlation, 0–0.99 the two variables tend to increase or decrease together, 0 the two variables do not vary together at all, 0 to –0.99 one variable increases as the other decreases and –1 as perfect negative or inverse correlation. All analyses were carried out in GraphPad Prism Version 7.0 (La Jolla, CA, United States).
Results
Growth Hormone Axis
Considering that fish growth is affected by the photoperiod and temperature (Boeuf and Falcón, 2001), we initially investigated the effect of lower temperature as a cold stressor on the GH axis. At molecular level we evaluated the expression of the growth hormone gene (gh1) in the brain, its receptors (ghra and ghrb) and the insulin-like growth factor 1 (ig1a and igf1b) and its receptors (ig1ra and igf1rb) in the liver and muscle.
We found a significant interaction between time and temperature of gh1 [F(1,14) = 5.068, p = 0.04] in the brain. It should be noticed that the gh1 gene expression was higher (fourfold change, p = 0.009) at early dark phase (ED) in comparison to early light phase (EL) in fish subjected to 28°C (Figure 2A). A reduction of expression (fivefold change, p = 0.018) was found in the lower temperature-treated animals at ED as compared to EL (Figure 2A). Due to this decrease of mRNA level, the difference seen between the analyzed time points was abolished, due to dampening of the amplitude or shift of the expression peak (Figure 2A).
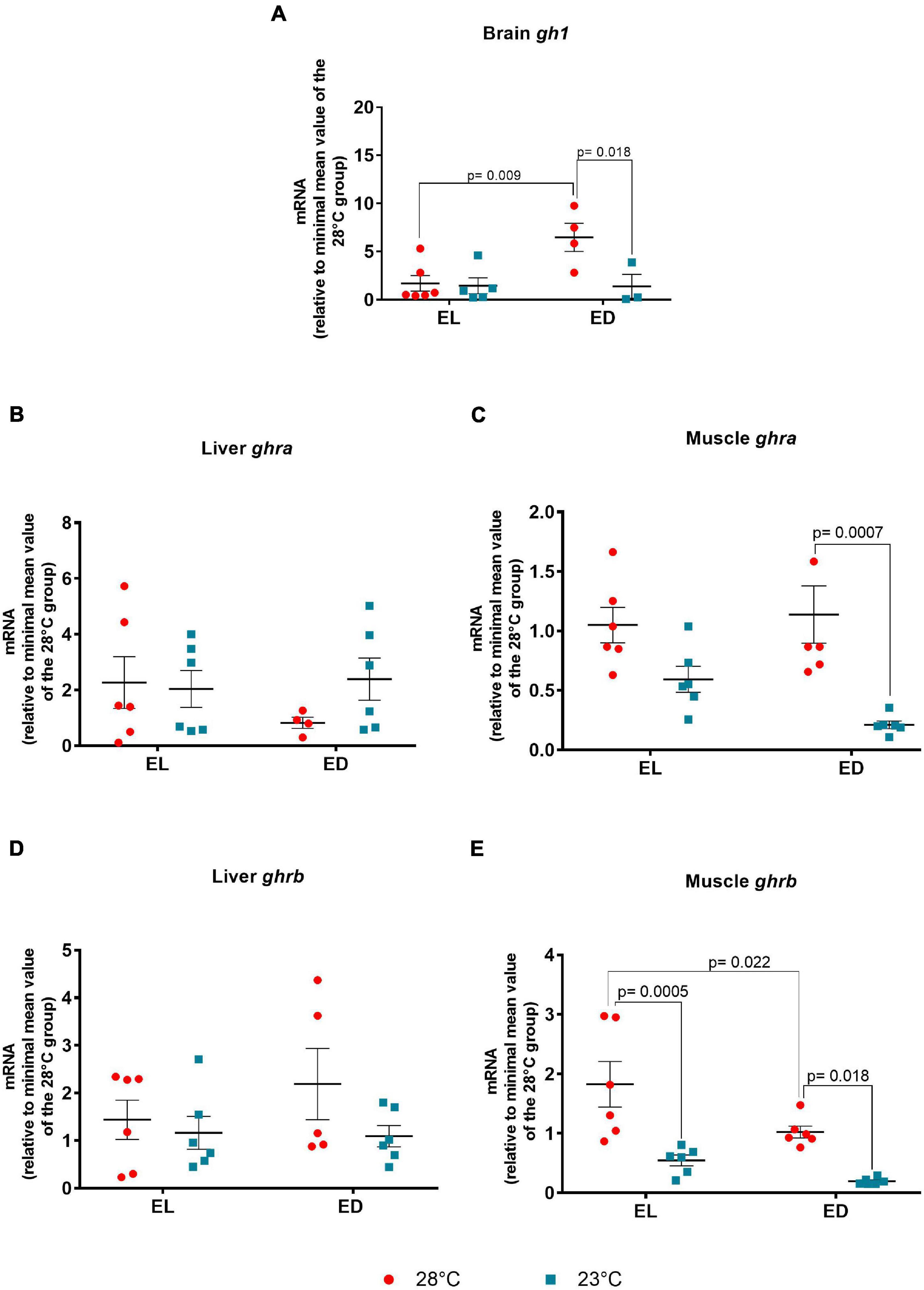
Figure 2. Early light (EL) and early dark (ED) gene expression of growth hormone (gh1) and growth hormone receptors (ghra and ghrb) in adult Danio rerio. (A) Brain gh1; (B) liver ghra; (C) muscle ghra; (D) liver ghrb; (E) muscle ghrb. The gene expression was normalized by the 18S rRNA and expressed relative to the lowest mean of the control temperature group. The values are expressed as mean ± SEM. n = 3–6 (pools of four animals each). Significant differences are shown when p < 0.05. Red symbol corresponds to 28oC and blue symbol corresponds to 23oC.
In the liver, the GH receptor ghra did not vary between EL and ED at 28 or 23oC (Figure 2B). In the muscle, ghra (Figure 2C) did not vary between time points at both temperatures, but its transcript exhibited a marked reduction (fivefold change, p = 0.0007) in 23°C-exposed animals at ED compared to the control temperature group at the same time of day. The ghrb expression showed no variation in the liver of animals from both temperatures (Figure 2D). On the other hand, the F value of muscle ghrb indicated a significant impact for each stimulus (mostly temperature) [time point F(1,20) = 8.078, p = 0.01], [temperature F(1,20) = 26.91, p < 0.0001] but no interaction between them. The ghrb mRNA level in the muscle displayed a decrease (twofold change, p = 0.022) at ED compared to EL in the animals at 28oC and a reduction at the lower temperature at EL (threefold change, p = 0.0005) and ED (fivefold change, p = 0.018), as compared to the control temperature group (Figure 2E).
Next, we evaluated other components of the GH pathway such as the insulin-like growth factors igf1a and igf1b and their receptors, igf1ra and igf1rb. The expression of igf1a in the liver showed no statistical differences between temperatures or times of day (F value not significant) (Figure 3A). However, the ANOVA analysis for the muscle igf1a showed significant interaction between the two factors [F(1,10) = 23.23, p = 0.0007]. Also in the muscle, there was no difference between the expression at EL and ED at 28oC, but a reduction at ED compared to EL at 23oC was observed (sixfold change, p = 0.0005) (Figure 3B). Still in the muscle, an increase in igf1a mRNA was observed at 23oC in comparison to 28oC at EL (fourfold change, p = 0.002). In the liver, the F value was not significant for the interaction between time and temperature. However, the igf1b transcripts showed the highest expression at ED compared to EL at 28°C suggesting a nocturnal peak (fourfold change, p = 0.032); no statistical differences were found between EL and ED at 23oC (Figure 3C). The F value for the muscle igf1b indicated significant interaction between time and temperature [F(1,10) = 19.48, p = 0.001]. The muscle igf1b transcripts were found to decrease at ED compared to EL at 23oC (sevenfold change, p = 0.0003) and to increase at 23oC compared to 28oC at EL (14-fold change, p = 0.0003) (Figure 3D).
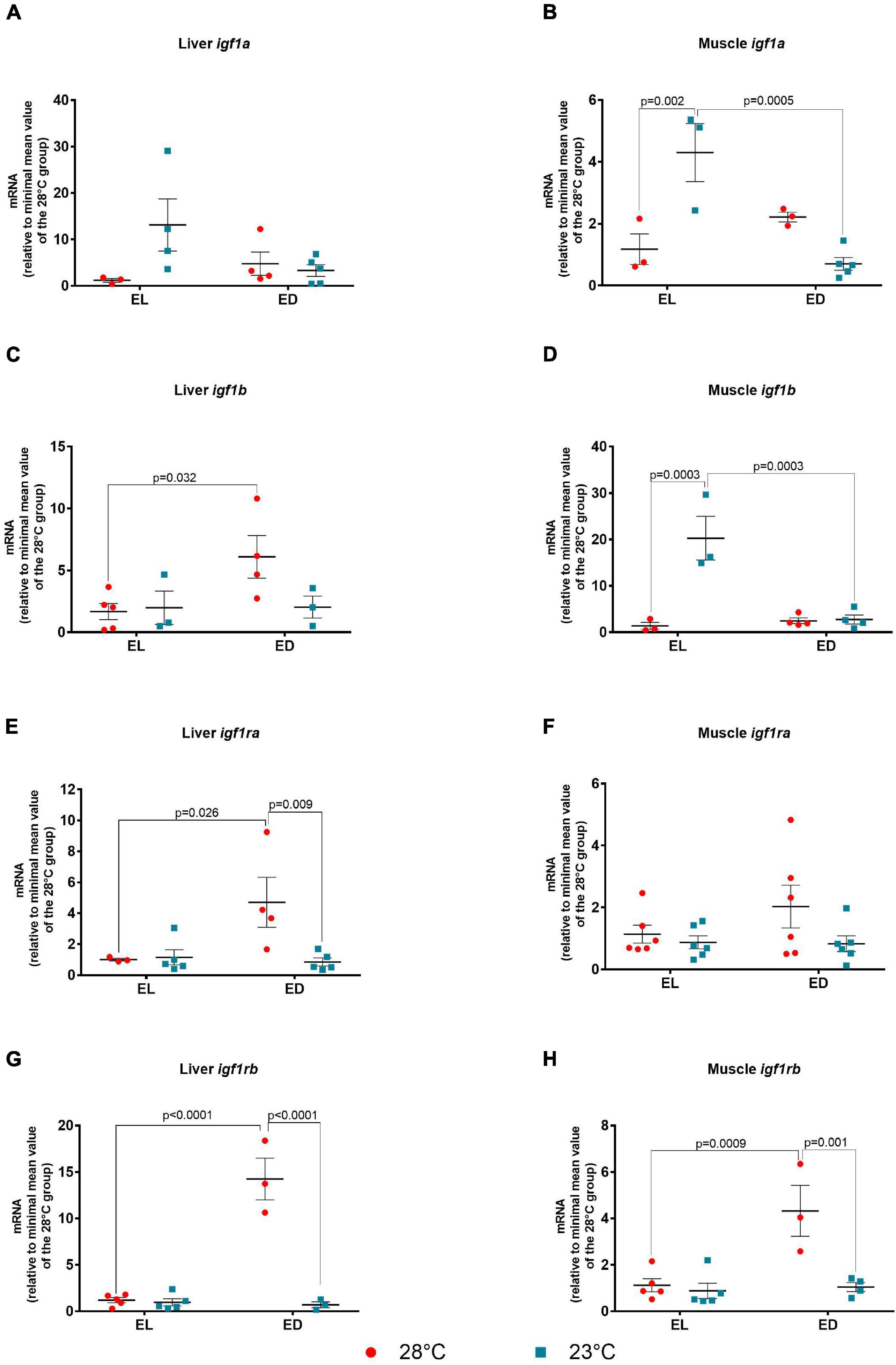
Figure 3. Early light (EL) and early dark (ED) gene expression of insulin-like factors (igf1a and igf1b), and their receptors (igf1ra and igf1rb) in adult Danio rerio. (A) Liver igf1a; (B) muscle igf1a; (C) liver igf1b; (D) muscle igf1b; (E) liver igf1ra; (F) muscle igf1ra; (G) liver igf1rb; (H) muscle igf1rb. The gene expression was normalized by the 18S rRNA and expressed relative to the lowest mean of the control temperature group. The values are expressed as mean ± SEM. n = 3–6 (pools of four animals each). Significant differences are shown when p < 0.05. Red symbol corresponds to 28oC and blue symbol corresponds to 23oC.
As to Igf receptors, the ANOVA analysis for the hepatic igf1ra showed significant interaction between the factors [F(1,13) = 5.683, p = 0.03]. In this organ, the igf1ra showed the highest expression at ED compared to EL at 28°C (fivefold change, p = 0.026), and a decrease at 23oC at ED when compared to 28°C (fivefold change, p = 0.009) (Figure 3E). There were no differences in the muscle igf1ra between time points or temperatures (F value not significant) (Figure 3F).
The F value of hepatic igf1rb showed a significant interaction between time and temperature [F(1,12) = 56.37, p < 0.0001]. The igf1rb transcripts in the liver exhibited similar profile to igf1ra. An increase at 28oC at ED compared to EL (12-fold change, p < 0.0001) and a marked decrease at 23oC compared to 28oC at ED (20-fold change, p < 0.0001) (Figure 3G). In the muscle, similar differences between time points (fourfold change, p = 0.0009) and temperatures (fourfold change, p = 0.001) were observed (Figure 3H). The F value showed a significant interaction between both factors [F(1,13) = 10.67, p = 0.006].
Cortisol Axis
Another hormone involved in teleost growth is cortisol which decreases muscle protein and hepatic glycogen levels and induces hyperglycemia (Auró de Ocampo and Ocampo, 1999; Baltzegar et al., 2015).
Since temperature changes (–5oC) are cold stressful factor, we analyzed cortisol concentration in the whole-body extract (animals’ weights are shown in Supplementary Table S2). The ANOVA analysis showed a significant interaction between the two factors [F(1,8) = 140.9, p < 0.0001]. We found an increase in the cortisol levels at ED compared to EL in the control temperature group (p = 0.011) and the opposite effect at 23oC (p < 0.0001) (Figure 4A). Unexpectedly, the control temperature group did not show higher levels in the light phase possibly because its peak preceded the time of sample collection. However, we observed an increase in cortisol (μg/g body weight) in animals at 23°C in the EL (p < 0.0001) and ED (p = 0.040) as compared to the control temperature group at the same time points (Figure 4A).
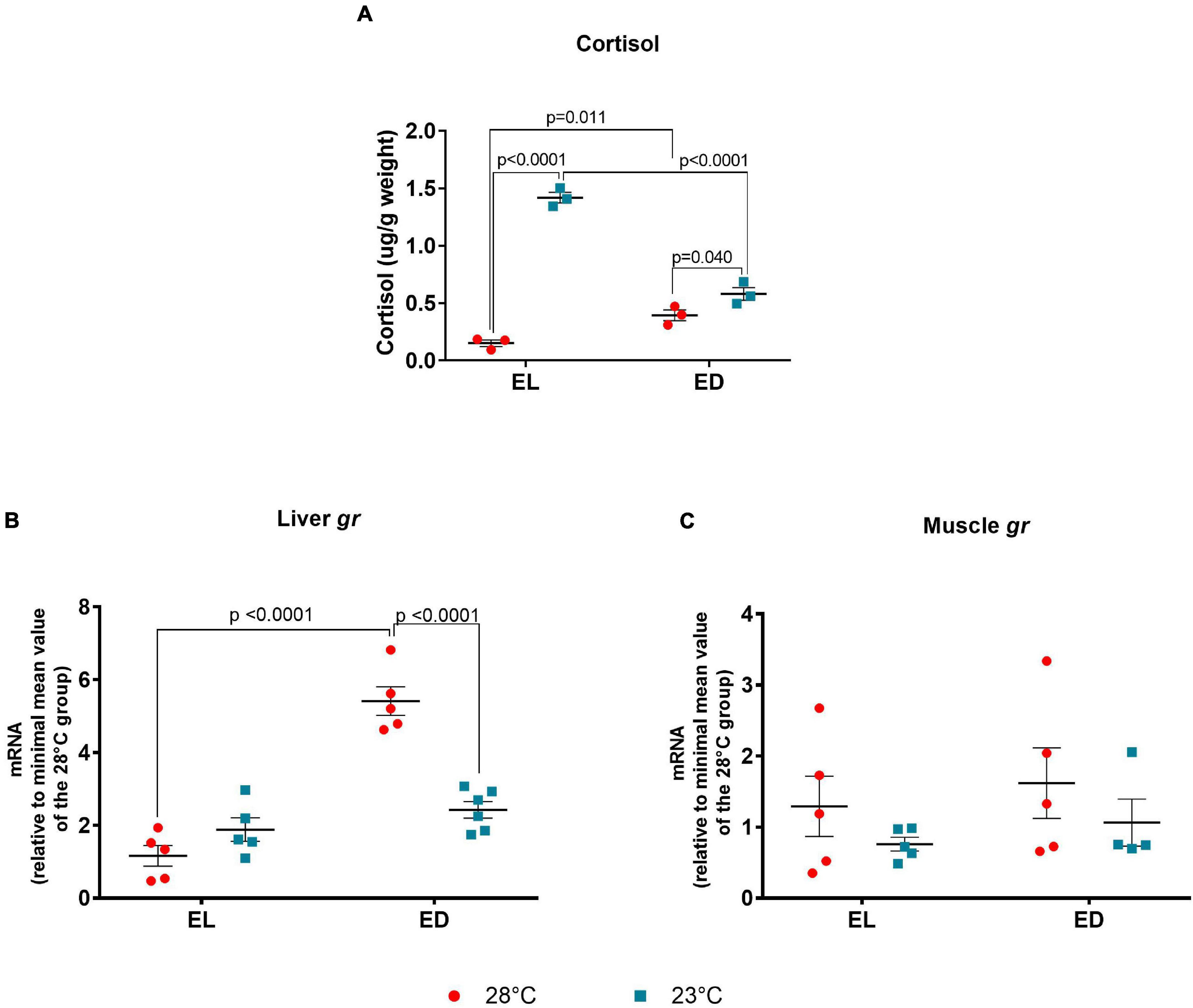
Figure 4. Early light (EL) and early dark (ED) whole body cortisol and gene expression of glucocorticoid receptor (gr) in adult Danio rerio. (A) Cortisol levels; (B) liver gr; (C) muscle gr. The hormone concentration (μg) was normalized by sample weight (g, Supplementary Table S2). The gene expression was normalized by the 18S rRNA and expressed relative to the lowest mean of the control temperature group. The values are expressed as mean ± SEM. n = 3 (pools of four animals each). Significant differences are shown when p < 0.05. Red symbol corresponds to 28oC and blue symbol corresponds to 23oC.
We next analyzed the glucocorticoid receptor (gr) expression in metabolic tissues as liver and muscle. In the liver, the ANOVA analysis indicated significant interaction between time of day and temperature [F(1,17) = 36.64, p < 0.0001]. In addition, we found a significant peak in gr expression at ED in animals at 28oC (Figure 4B) (fivefold change, p < 0.0001); when subject to 23oC, there were no differences between EL and ED. At ED, the transcripts of gr decreased at 23oC (twofold change, p < 0.0001) as compared to 28oC (Figure 4B). In the muscle, the gr expression showed no significant differences between day and night at the same temperature or between temperatures at the same time point (Figure 4C).
Melatonin Axis
Based on the interactions of melatonin with the previously evaluated hormone axis, we decided to investigate whether this pathway (at the molecular level) was affected by temperature variation, analyzing the gene expression of the enzyme arylalkylamine N-acetyltransferase and melatonin receptors.
In the brain, aanat2 expression was evaluated. The ANOVA analysis showed significant interaction between time and temperature [F(1,10) = 39.75, p < 0.0001]. This gene transcripts showed no difference between time points at 28°C but showed an increase at ED compared to EL at 23oC (21-fold change, p < 0.0001) (Figure 5A). On the other hand, a remarkable increase of aanat2 mRNA was induced in animals exposed to 23°C when compared with the control temperature animals at 28°C, at ED (214-fold change, p < 0.0001) (Figure 5A). As expected, aanat2 expression peaked in the dark at 23oC which may suggest an increase of melatonin synthesis in lower temperature-exposed animals.
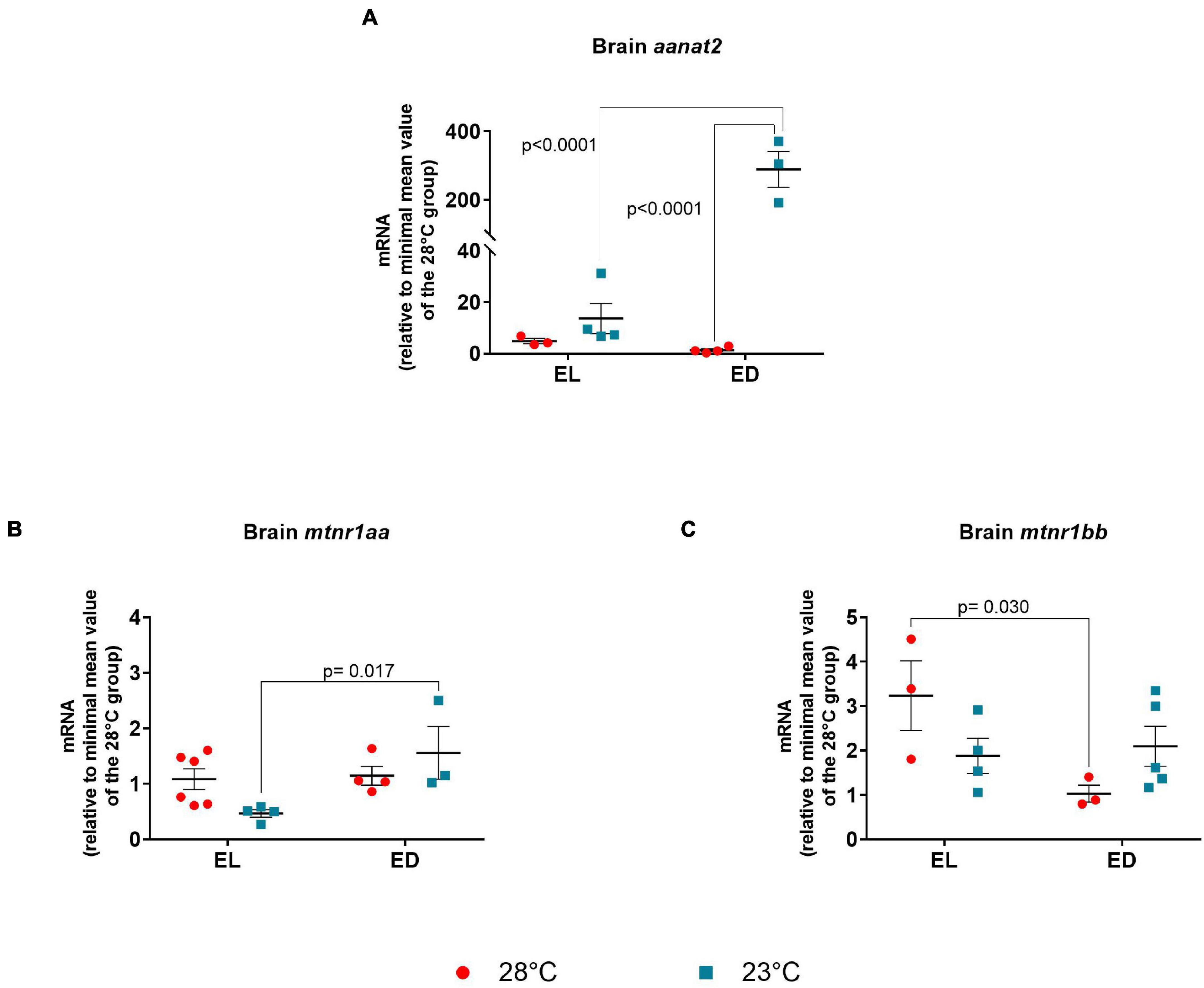
Figure 5. Early light (EL) and early dark (ED) gene expression of arylalkylamine N-acetyltransferase (aanat2) and melatonin receptors (mtnr1aa and mtnr1bb) in adult Danio rerio. (A) Brain aanat2; (B) Brain mtnr1aa; (C) brain mtnr1bb. The gene expression was normalized by the 18S rRNA and expressed relative to the lowest mean of the control temperature group. The values are expressed as mean ± SEM. n = 3–6 (pools of four animals each). Significant differences are shown when p < 0.05. Red symbol corresponds to 28oC and blue symbol corresponds to 23oC.
Melatonin plays a key role in several tissues, but we focused on the hormone central sources and its local effects. In the brain, the melatonin receptors mtnr1aa and mtnr1bb were evaluated. The ANOVA analysis showed a significant interaction between the two factors for both genes [mtnr1aa F(1,13) = 4.896, p = 0.04] and [mtnr1bb F(1,11) = 5.923, p = 0.03]. Temporal variation was seen for mtnr1aa mRNA in the brain at 23oC, with peak in the ED phase (threefold increase, p = 0.017) (Figure 5B). The transcripts of mtnr1bb showed a decrease at ED compared to EL in the control temperature group (threefold decrease, p = 0.030) (Figure 5C).
Clock Genes
Finally, we investigated if a change of 5°C would be sufficient to affect the molecular clock in adult D. rerio. Previous results from literature and from our laboratory indicated that clock genes such as clock (in D. rerio larvae at 20 and 30oC) and bmal (in D. rerio ZEM-2S cells at 28 and 33oC) are not affected by temperature changes (Lahiri et al., 2005; Jerônimo et al., 2017), so we chose to evaluate the negative clock genes, per and cry in all tissues evaluated.
The ANOVA analysis for per1 indicated a significant interaction between the two factors only in the brain [F(1,14) = 13.17, p = 0.002]. In the liver and muscle an increase of per1 expression was observed in EL animals as compared to ED fish [liver F(1,10) = 10.33, p = 0.009], [muscle F(1,17) = 59.92, p < 0.0001]. At 28°C, per1 had higher expression at EL compared to ED in the brain (13-fold change, p < 0.0001) and muscle (sevenfold change, p < 0.0001), evidencing that the gene peaks in the light phase (Figures 6A–C). In the brain, there was a decrease in per1 expression at 23°C at EL as compared to the control temperature animals (threefold decrease, p = 0.001), which demonstrate that low temperatures disrupt (reduced or phase shifted) per1 expression (Figure 6A). At 23°C, we also observed a decrease in per1 expression at ED compared to EL in the liver (17-fold decrease, p = 0.012) and the muscle (fivefold decrease, p < 0.0001) (Figures 6B,C).
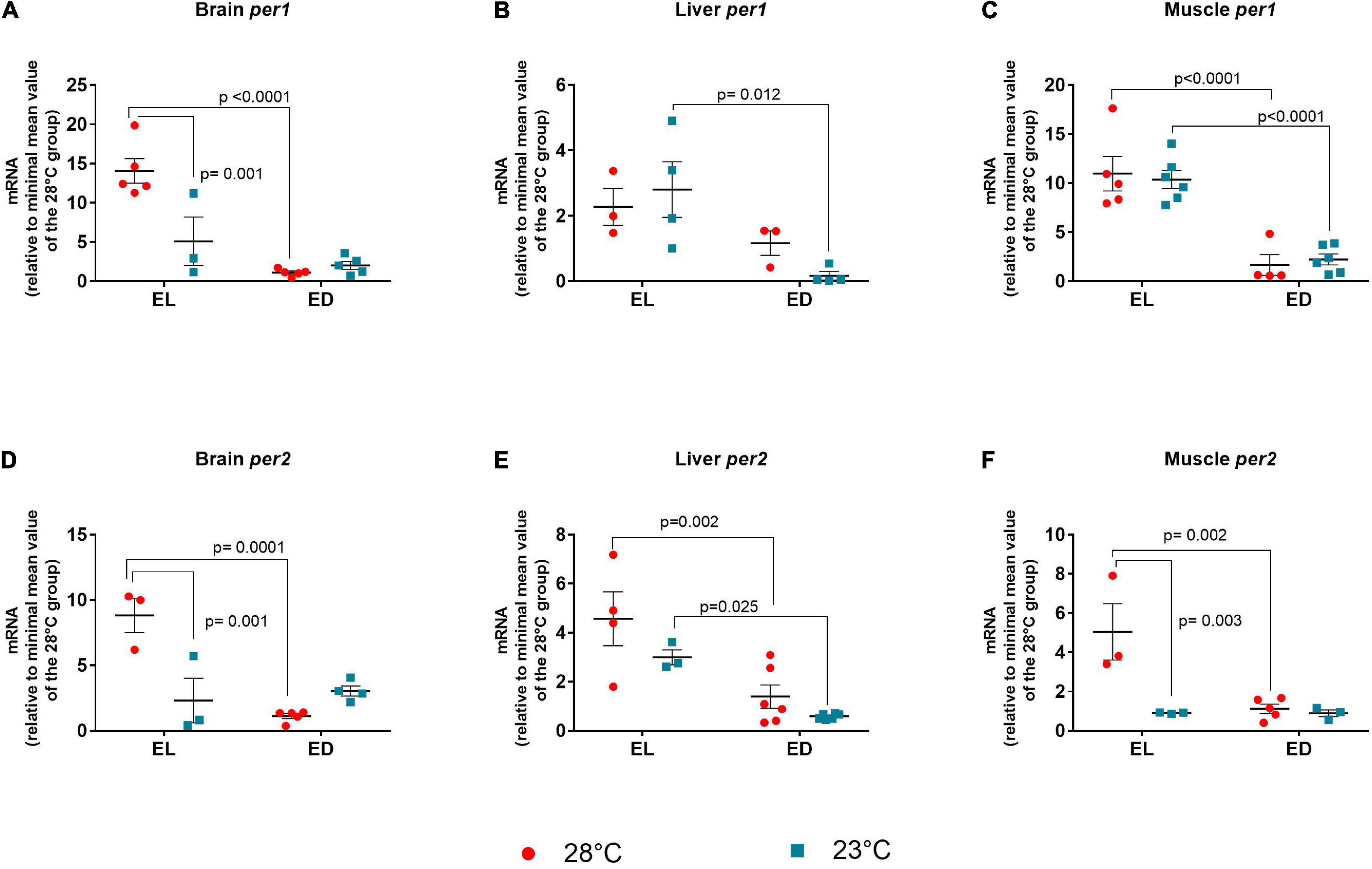
Figure 6. Early light (EL) and early dark (ED) expression of period (per1 and per2) genes in adult Danio rerio. (A) Brain per1; (B) liver per1; (C) muscle per1; (D) brain per2; (E) liver per2; (F) muscle per2. The gene expression was normalized by the 18S rRNA and expressed relative to the lowest mean of the control temperature group. The values are expressed as mean ± SEM. n = 3–6 (pools of four animals each). Significant differences are shown when p < 0.05. Red symbol corresponds to 28oC and blue symbol corresponds to 23oC.
The ANOVA analysis of per2 showed significant interaction between factors in the brain [F(1,11) = 23.16, p = 0.0005] and the muscle [F(1,10) = 9.258, p = 0.01]. In the liver a higher expression of per2 was seen in EL [F(1,15) = 23.26, p = 0.0002]. Like per1, per2 mRNA in the brain (Figure 6D), liver (Figure 6E), and muscle (Figure 6F) of animals kept at 28°C exhibited higher expression at EL than at ED (p = 0.0010; p = 0.002; p = 0.002, respectively), peaking in the early light phase. This peak was also observed only in the liver at 23oC (p = 0.025) (Figure 6E).
In the brain and muscle, there was a significant decrease in gene expression of per2 in EL in animals kept at 23°C compared to the control temperature group (brain, fourfold decrease, p = 0.001), (muscle, fivefold decrease, p = 0.003) (Figures 6D,F).
For cry1a, a significant interaction between the two factors was found in the liver [F(1,13) = 83.54, p < 0.0001] and the muscle [F(1,17) = 17.63, p = 0.0006)]. In the brain a higher transcript level was demonstrated in the EL group [F(1,9) = 19.4, p = 0.0017]. In all three organs, the expression of cry1a at 28°C at EL was significantly higher than at ED (brain p = 0.003; liver p < 0.0001; muscle p < 0.0001, respectively) (Figures 7A–C). This light phase peak was evidenced also in the liver (p = 0.013) and muscle (p = 0.002) at 23oC. In the liver (Figure 7B) and muscle (Figure 7C), although the expression peak of crya was still maintained at the lower temperature, its amplitude was significantly decreased (liver, fivefold decrease, p < 0.0001), (muscle, twofold decrease, p = 0.002).
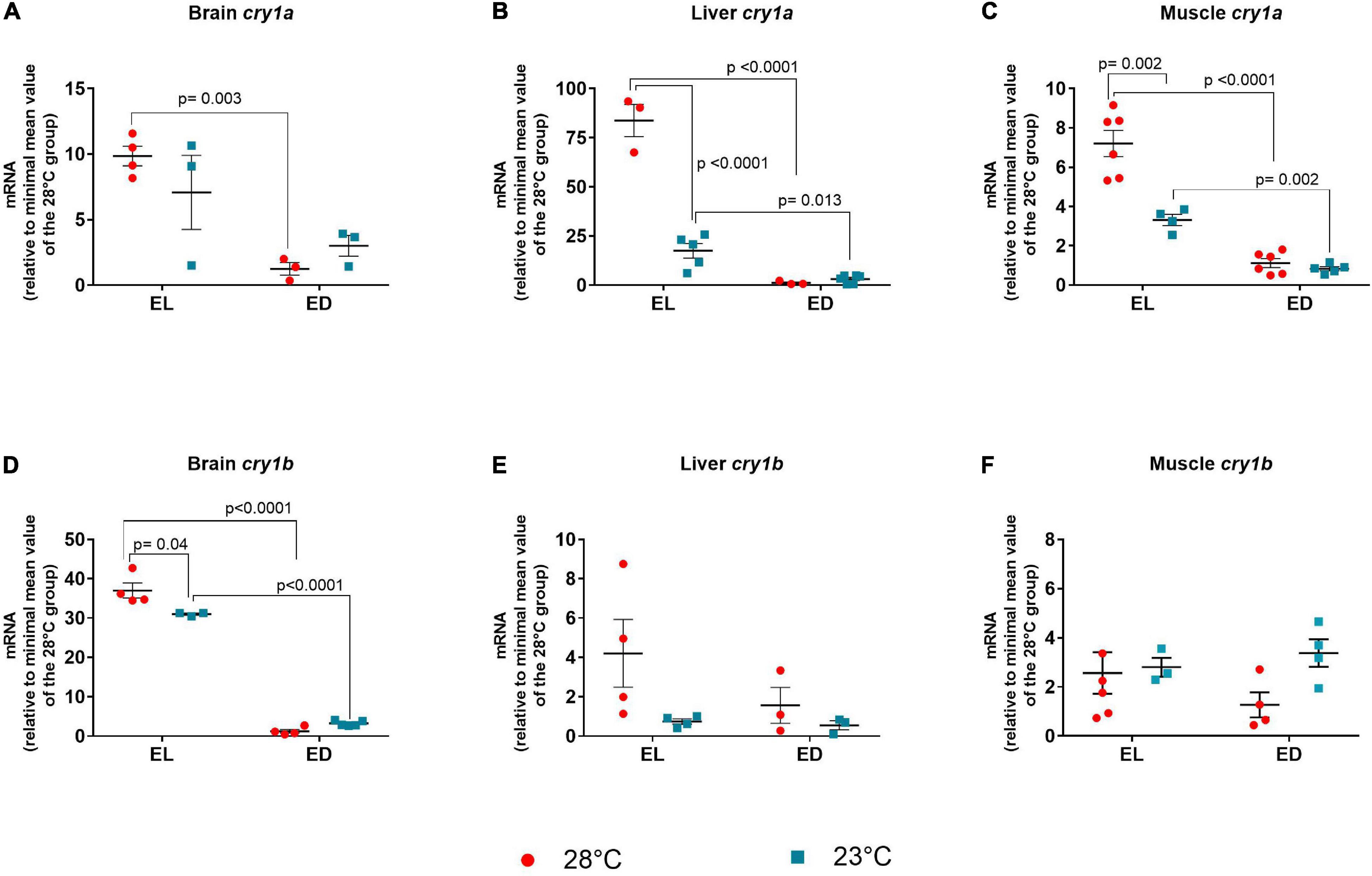
Figure 7. Early light (EL) and early dark (ED) expression of cryptochrome (cry1a and cry1b) genes in adult Danio rerio. (A) Brain cry1a; (B) liver cry1a; (C) muscle cry1a; (D) brain cry1b; (E) liver cry1b; (F) muscle cry1b. The gene expression was normalized by the 18S rRNA and expressed relative to the lowest mean of the control temperature group. The values are expressed as mean ± SEM. n = 3–6 (pools of four animals each). Significant differences are shown when p < 0.05. Red symbol corresponds to 28oC and blue symbol corresponds to 23oC.
When the F value of cry1b was analyzed, a significant interaction between the two factors was seen only in the brain [F(1,12) = 14.72, p = 0.002]. The expression in the brain of animals kept at 28°C or 23oC peaked in the EL with a considerable reduction at ED (30-fold decrease at 28oC and ninefold decrease at 23oC, p < 0.0001) (Figure 7D). Also, the brain showed decreased cry1b expression in the EL at lower temperature (1.2-fold decrease, p = 0.004) as compared to the control temperature group, but the peak was still observed in EL. Liver and muscle cry1b transcripts showed no statistical differences between time points or temperatures (Figures 7E,F).
In summary, regardless of the temperature a general pattern of transcripts was seen in the organs evaluated, which exhibited higher clock gene expression during the day (Table 2).
Correlation Analysis
Once the gene expression values were obtained, we performed a Pearson correlation analysis between the expression of clock genes and every other gene in the different tissues. It should be noted that this type of analysis offers a hypothetical relationship that cannot necessarily be evidenced in the physiology of the animal. A heat map was built to present the results: samples correspond to the same day time (EL or ED) and the temperature conditions (28 or 23°C) (p < 0.05) (Figure 8).
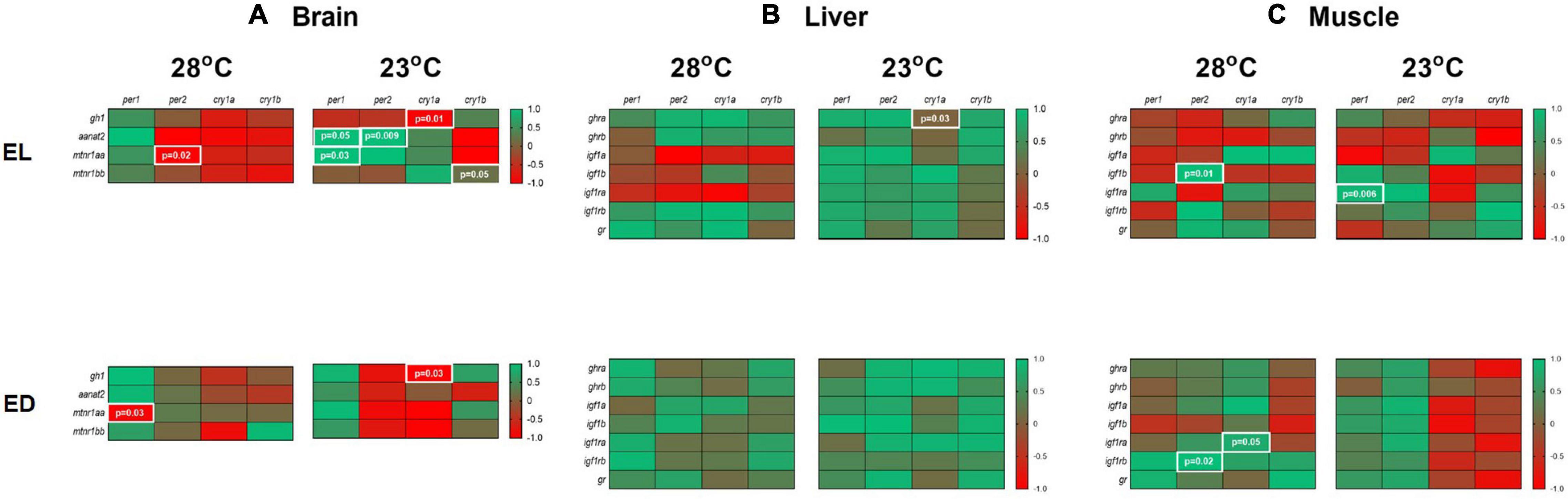
Figure 8. Correlation of gene expression in the brain, liver and muscle of adult Danio rerio. (A) Brain. Four positive and four negative correlations; (B) liver. One positive correlation; (C) muscle. Four positive correlations. Data from the same sample, temperature and time point with a Gaussian distribution were analyzed by Pearson correlation coefficients. The values are expressed as coefficient r. Values: 1 perfect correlation; 0 to 0.99 the two variables tend to increase or decrease together; 0 the two variables do not vary together at all; 0 to –0.99 one variable increases as the other decreases and –1 as perfect negative or inverse correlation. Significance was set for p < 0.05, shown in white letters inside white boxes.
In the brain, at 28oC, we observed negative correlations between per1/mtnr1aa and per2/mtnr1aa. At 23°C, we found positive correlations for per1/aanat2, per1/mtnr1aa, per2/aanat2 and cry1b/mtnr1bb. A negative correlation was found in the cold-exposed group between cry1a/gh1 (p < 0.05) (Figure 8A).
In the liver, only a positive correlation was observed between cry1a/ghra at 23°C group (p < 0.05) (Figure 8B). In the muscle, at 28°C, positive correlations were observed between per2/igf1b, per2/igf1rb and cry1a/igf1ra (p < 0.05) (Figure 8C). At 23°C, a positive correlation between per1/igf1ra (p < 0.05) was seen (Figure 8C).
Considering these data, we suggest an interaction (positive or negative) between clock genes and a few genes of the hormonal pathways studied including those of the growth and melatonin pathways (Figure 9).
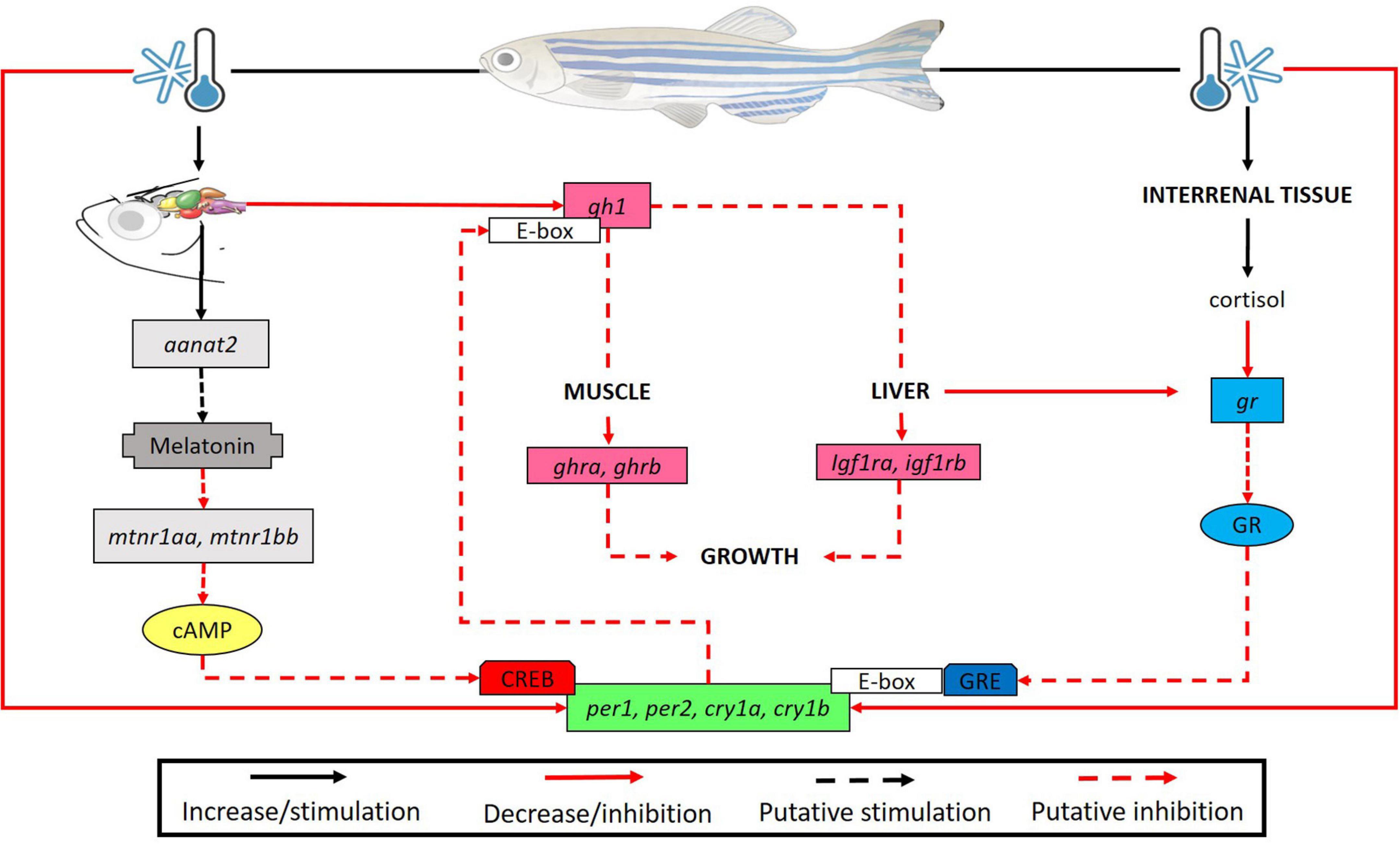
Figure 9. Brain of D. rerio submitted to low temperatures show high levels of the aanat2 transcripts causing a putative increase in melatonin synthesis. We suggested that expression of melatonin receptors (mtnr1aa and mtnr1bb) may decrease to ensure homeostasis. In turn, it is known that mtnr1aa, mtnr1bb (Gi protein-coupled receptors) inhibit adenylate cyclase activity which ultimately leads to the inhibition of CREB phosphorylation, a transcription factor required for clock gene expression, resulting in a reduction of their transcripts. Also, in the brain we found a reduction in the gh1 transcripts at lower temperature, leading to a decrease of ghra and ghrb in the muscle, and igf1ra, igf1rb in the liver, suggesting a long-term inhibition of the animal growth. In turn, clock genes are capable to bind to the promoter E-box region of genes as gh1 thus modulating its expression (a putative inhibition in our study). On the other hand, there was an increase in the cortisol concentration and a compensatory reduction of its receptor transcripts. The glucocorticoid (Gc)/glucocorticoid receptor complex modulates the expression of clock genes by binding to glucocorticoid response elements (GRE) in their promoters. Consequently, we evidenced a decrease in the expression of clock genes in all organs analyzed. This may be associated to cold-modulation by melatonin and cortisol pathways or a direct effect of low temperature on clock gene transcripts.
Discussion
In several teleost species, GH secretion is increased at higher temperatures suggesting accelerated growth during spring/summer and reduced GH levels in the winter (Deane and Woo, 2009). The otoliths commonly known as “ear stones” are hard, calcium carbonate structures, located directly behind the teleost brain. Its measurement has frequently been used as a proxy for somatic growth in marine and freshwater fish species (Rudy and Forsberg, 2016). In D. rerio and other teleosts, the environmental temperature modulates the growth of the otoliths due to an up- downregulation of the GH axis (Wang et al., 2015; Grønkjær, 2016; Loeppky et al., 2021). When gh1 in the brain was evaluated, we showed that gene expression peaked in the early dark in control temperature animals with a notable reduction in animals kept at lower temperature, demonstrating the nocturnal pattern of this hormone. Also, according to Cowan et al. (2017) the highest GH production usually coincides with the highest water temperature in several fish species. In fish, the cAMP signaling pathway leads to increased gh1 mRNA transcription and hormone release through the activation of transcription factors such as c-fos (Klein and Sheridan, 2008). In mammals, the cryptochrome (Cry) genes are known to inhibit the accumulation of cAMP by interacting with Gs protein (Zhang et al., 2010). According to the correlation analyses, we demonstrate this modulation by the clock genes (specifically per1 and cry1a) on gh1 transcripts. In this way, the gh1 is down-regulated not only by the lower temperature as by the time of the day in D. rerio adults.
Due to their ectothermic physiology, tropical fish in cold waters decrease their metabolism, resulting in a reduction of growth, probably due to a decrease of both GH production and the affinity and/or number of its receptors (White et al., 2012). No difference in size and weight was found in animals from the two temperatures, probably due to the short (6 days) exposure to 23oC (data not shown). Molecular alterations probably occur before those changes reflect as actual growth. Our results agree with what was reported by Gabillard et al. (2006) who kept rainbow trout (Oncorhynchus mykiss) at two temperatures (12 and 4°C) and obtained an increase for ghra transcripts when the water temperature was raised and a remarkable decrease at low temperature.
The liver is the major source of circulating IGF1 in fish (Leroith et al., 2001). In our study, we evidenced high levels specifically of the hepatic igf1b in the temperature control group at ED, corresponding with the gh1 peak at the same time of the day. Curiously, the muscle presented an increase of igf1a and igf1b in the lower temperature group during EL, so we hypothesized that in this organ the protein is acting locally to compensate the cold-induced down-regulation of the GH axis. In agreement with our study, in zebrafish larvae exposed to low temperatures the igfbp1a (or igf1a) expression was up-regulated (Long et al., 2013).
The igf1ra/igf1rb in the liver showed a similar pattern of expression of the igf1, demonstrated an exocrine/paracrine effect. Mohammed et al. (2015) described the gilt-head bream (Sparus aurata) muscle as one of the organs with highest expression igf1r followed by the pituitary gland. This is explained by the fact that fish, unlike mammals, possess a more active Igf-1 muscle binding, replacing the active insulin binding in mammalian muscles (Dai et al., 2015).
Peterson et al. (2005) demonstrated that GH injection did not have an effect on IGF expression in catfish (Ictalurus punctatus) muscle. Studies from Gabillard et al. (2003) and Reindl and Sheridan (2012) have shown that the GH-IGF system is modulated by adjusting peripheral sensitivity to both hormones as well as their availability at the target cells. In addition, nutritional state, insulin, somatostatin, cortisol and thyroid hormones, light and temperature exert effects on the expression and activity of the elements of the GH/IGF system. The interactions described above may explain the increase of igf1a and igf1b in the muscle even when the animals were kept at low temperatures since IGF mRNA expression is only partly regulated by GH in extra-hepatic tissues.
In general, changes in water temperature causes stress in fish, which includes physiological and behavioral adaptive changes and even death (Gordon, 2005). Our data for whole body cortisol showed remarkably higher amounts in animals kept at 23°C, with a noticeable reduction at early dark phase. The study conducted by Ramsay et al. (2009) in adult D. rerio demonstrated high levels of cortisol in animals stressed by handling, with a recovery of baseline levels 24 h post-stimulation. Unexpectedly, animals kept at 28°C had low hormonal levels at early morning and at night, not showing the well-known anticipatory peak in the early morning. Because of the time points chosen in our study, we suggest that the peak release of cortisol in animals kept at 28°C may have occurred prior to EL and that the high levels in animals at lower temperatures correspond to a sustained stress-induced response, with a phase shift of the peak.
Several studies using corticosteroids demonstrated a remarkable transcriptional modulation of clock genes (Zhao et al., 2016, 2018; Sousa et al., 2017). The fludrocortisone acetate at concentration of 6 and 42 μg/L downregulates the expression of per1 per2 and nr1d1 genes in peripheral tissues of adult zebrafish (Danio rerio) (Zhao et al., 2016). On the other hand, an increase in per1 and cry1b transcripts was found in D. rerio ZEM-2S cells treated with 3 nM dexamethasone Sousa et al., 2017). In addition, in D. rerio eleuthero-embryos, progesterone, dexamethasone and 7α-ethinylestradiol at 1 μM up-regulate the expression of per1, cry1a, rora, nr1d1, and nr1d2a genes (Zhao et al., 2018). In contrast, our in vivo data suggest that the increase of the cortisol level (to 1.5 μg/g body weight) in animals at lower temperatures did not affect the expression of clock genes. We hypothesize that the interaction between lower temperature and the subsequent increase of cortisol may have resulted in a different response than when glucocorticoid is applied in a controlled temperature condition.
As to the expression of gr, there was a reduction in the mRNA of this receptor in the liver, but not in the muscle, of animals kept in colder temperature at early dark phase. Reports have shown that the activation of the hypothalamus-hypophysis-adrenal axis in heat stress-induced responses was accompanied by a peripheral adaptation in the amount and affinity of glucocorticoid receptors (Molijn et al., 1995). In our protocol (6 days at 23°C), it would be energy consuming to the organism to generate and keep high levels of gr mRNA and/or protein, as it would trigger an exacerbated response to cortisol which was elevated, and consequently could lead to homeostasis disruption.
In particular, temperature can have a strong impact on melatonin biosynthesis. The maximum activity of Aanat2 in a number of species correlated with the species-specific preferred water temperature and it is proposed as a mechanism of evolutionary adaptation (Falcón et al., 2009; Cowan et al., 2017). Furthermore, recent work in salmonids has suggested that pineal photoreceptors may also be thermoreceptors, since they express thermotransient receptor potential (TRP) channels (Nisembaum et al., 2015). In tropical fish such as the Senegalese sole (Solea senegalensis), the highest values of melatonin were observed at 25oC when the animals were kept in water ranging from 11.5oC to at 25°C compared to temperature ranging between 17 and 20°C (Vera et al., 2007). In our study, lower temperature led to a considerable increase on brain aanat levels in ED, suggesting possible increased melatonin levels. Studies demonstrated the rhythmic secretion of melatonin in D. rerio and its maximal release at the second third of the dark phase (Appelbaum et al., 2009; Ben-Moshe et al., 2016). Considering that at 28°C EL and ED, aanat expression exhibited no difference, we suggest that the highest aanat level and consequently the melatonin peak may have occurred at a nighttime point subsequent to the one evaluated. If this hypothesis holds true, there was an advance phase shift in the expression of aanat2 in the brain at 23°C.
Loganathan et al. (2018) found that fish challenged by high temperatures had an increase in mtnr1aa and mtnr1bb mRNA levels in the brain. Interestingly, we found that the expression of mtnr1aa depends on the time point but not the temperature. As for mtnr1bb, we found a decrease of transcripts related to time point in the temperature control group, and an interaction between time and temperature shown by the ANOVA analysis. Likewise, our data suggest expression peaks of brain mtnr1aa in the dark, like the results of Yumnamcha et al. (2017) in Danio rerio females.
Melatonin is known to affect the rhythm of three clock genes in vivo, specifically per1, per2 and cry1 in the Japanese flounder (Paralichthys olivaceus) (Mogi et al., 2017). The activation of the melatonin receptor 1 inhibits cAMP formation, protein kinase A (PKA) activity, and phosphorylation of the cAMP-responsive element binding protein (CREB), required for the transcription of clock genes such as per1 (Masana and Dubocovich, 2001).
Danio rerio cells exhibited decreased per1 and cry1-3 expression at high temperature and increased mRNA at low temperature (Lahiri et al., 2005). Curiously, previous studies in our laboratory have shown increased expression of per1, per2, cry1a, and cry1b clock genes after heat shock (33oC) in cells of D. rerio maintained in dark-light cycle; in this study the authors suggested that the transient receptor potential TRPV1 played a role in heat-mediated responses since per2 increase was partially inhibited by TRPV1 blocker, demonstrating the channel participation in clock gene regulation by heat shock (Jerônimo et al., 2017).
Overall, we found higher expression of clock genes at EL with a marked decrease at ED and/or lower temperature. Several authors described a higher expression of the clock genes per1, per2, cry1a, and cry1b in D. rerio and other teleosts during the light phase or in the dark-light transition. In the specific case of per2 and cry1a, the light stimulus is sufficient to trigger their expression, generally at ZT0 (night-day transition) (Delaunay et al., 2003; Wang, 2008). Our data corroborate the previous results: independently of the organ, the peak expression was at early light phase, assuming that per and cry transcription as well as the protein heterodimer formation (Per:Cry) were achieved; during early dark phase, smaller expressions were obtained, corresponding to the decrease of the transcripts in the dark phase. These findings demonstrate that the molecular clock seems to show phase shift or a reduction of transcript amplitude in cold-exposed fish. To confirm this, a 24 h long gene expression analysis every 3 or 4 h is needed. It should be noticed that lower temperature decreases the clock genes transcripts even in the EL in most studies. In ectothermic vertebrates, low temperature dampens circadian rhythms of melatonin secretion and clock gene expression (Rensing and Ruoff, 2002; Vallone et al., 2007). Also, Prokkola et al. (2018) reported that a genome-wide decrease in transcriptional rhythms is due to the combination of decreasing day length and cold temperature in fish. Furthermore, these and our results contrast with those obtained in Danio rerio larvae exposed to low temperatures (16 and 12°C). The previous study demonstrated up-regulation of genes like per1, per2, cry1a, and cry1b in animals kept at reduced temperatures, suggesting the involvement of circadian clocks in the cold adaptation (Long et al., 2012, 2013). These controversial reports indicate that cold responses evolve during ontogeny and may have been lost in adults. Further studies should be carried out to demonstrate this point.
Our in vivo data at 23°C are similar to those in vitro assays previously obtained by our group. In embryonic cells of Danio rerio (ZEM-2S) challenged by a cold pulse (25.5°C) in constant dark, there was a decrease in per1 and cry1b mRNAs six hours after the end of the pulse (unpublished data). We can reconcile the above data with the presence/absence of light, since in the natural environment, the thermophase (high temperature) usually coincides with the photophase, and the cryophase (low temperatures) coincides with the scotophase (López-Olmeda and Sánchez-Vázquez, 2011).
As the gene transcripts may not reflect the dynamics of the protein expression and only two time points have been addressed, further studies are necessary to investigate the endpoint physiological responses at other time points.
Conclusion
We demonstrated that lower temperature negatively affects the transcripts of several genes including clock genes and genes of the melatonin, GH and cortisol pathways in zebrafish. This downregulation possibly impairs the physiological parameters of the fish. We also showed that, in animals exposed to lower temperatures, there is a physiological compensation for high levels of hormones such as cortisol, with reduction of the respective receptors. In addition, the clock molecular machinery possibly correlates with the other analyzed systems including the GH axis and the melatonin pathway. In the Figure 9 we raised some hypotheses of effects of low temperatures on the endocrine axis and molecular system. For example, we hypothesize an increase in melatonin synthesis when animals are submitted at reduced temperatures modifying its receptors (Gi protein-coupled receptors). Thus, it may lead to an inhibition of adenylate cyclase activity which ultimately may results in the inhibition of CREB phosphorylation (transcription factor required for clock gene expression). We also suggest that if low temperature period was longer maybe a possible decrease of growth were observed, since the GH axis were inhibited. On the other hand, the reduction of clock transcripts can lead to a putative inhibition of genes as gh1. Consequently, this reduction in all organs analyzed can be associated to cold-modulation by melatonin and cortisol pathways or a direct effect of low temperature on clock gene transcripts. To prove these hypotheses more studies involving longer treatment and other experimental techniques are necessary.
Data Availability Statement
The raw data supporting the conclusions of this article will be made available by the authors, without undue reservation.
Ethics Statement
The animal study was reviewed and approved by Ethics Committee on Use of Animals (CEUA) of the Institute of Biosciences, University of São Paulo, protocol N° 331/2018.
Author Contributions
CDSC, MNM, LVMA, and AMLC conceptualized the research. CDSC collected and analyzed the experimental data with the help of DDD, JASN, and OGL. CDSC wrote the manuscript, which was critically revised by AMLC, MNM, and LVMA. All the authors have approved the definitive version of the manuscript and agreed to be accountable for all aspects of the study in ensuring its accuracy and integrity.
Funding
This work was supported by the Coordination for the Improvement of Higher Education Personnel (CAPES, 88882.377378/2019-01 to CDSC and 88882.377368/2019-01 to JASN), the São Paulo Research Foundation (FAPESP, grants 2017/24615-5 and 2018/14728-0 to AMLC; scholarships 2018/23915-8 to OGL and 2018/23043-0 to DDD), and the National Council of Technological and Scientific Development (CNPq grants 303078/2019-7 to AMLC and 428754/2018-0 to MNM). MNM is a Young Investigator of FAPESP (2017/26651-9). LVMA was a fellow of FAPESP (2018/16511-8). Funding sources had no involvement in the study design, in the analysis and interpretation of data, in the writing of the report, and in the decision to submit the article for publication.
Conflict of Interest
The authors declare that the research was conducted in the absence of any commercial or financial relationships that could be construed as a potential conflict of interest.
Publisher’s Note
All claims expressed in this article are solely those of the authors and do not necessarily represent those of their affiliated organizations, or those of the publisher, the editors and the reviewers. Any product that may be evaluated in this article, or claim that may be made by its manufacturer, is not guaranteed or endorsed by the publisher.
Acknowledgments
We are thankful to the Department of Genetics and Evolutionary Biology of the Institute of Biosciences, especially to Professor Maria Rita dos Santos e Passos Bueno, and the Central Vivarium of the Faculty of Medicine, especially to Professor Bianca Helena Ventura Fernandes, facilities of the University of São Paulo, which provided the breeding stocks. To my advising committee, Professors Carla Denise Bonan (Catholic University of Rio Grande do Sul, PUCRS, Brazil) and Yohana Maria Velasco Santamaria (BioTox Laboratory, School of Animal Science, Universidad de los Llanos, Colombia) for the scientific discussions.
Supplementary Material
The Supplementary Material for this article can be found online at: https://www.frontiersin.org/articles/10.3389/fphys.2021.707067/full#supplementary-material
Footnotes
References
Alderman, S., and Bernier, N. (2009). Ontogeny of the corticotropin-releasing factor system in zebrafish. Gen. Comp. Endocrinol. 164, 61–69. doi: 10.1016/j.ygcen.2009.04.007
Appelbaum, L., Wang, G., Maro, G., Mori, R., Tovin, A., Marin, W., et al. (2009). Sleep-wake regulation and hypocretin-melatonin interaction in zebrafish. Proc. Natl. Acad. Sci. U.S.A. 106, 21942–21947. doi: 10.1073/pnas.906637106
Aschoff, J. (1965). Circadian rhythms in man. Science 148, 1427–1432. doi: 10.1126/science.148.3676.1427
Azpeleta, C., Martínez-Alvarez, R., Delgado, M., Isorna, E., and De Pedro, N. (2010). Melatonin reduces locomotor activity and circulating cortisol in goldfish. Horm. Behav. 57, 323–329. doi: 10.1016/j.yhbeh.2010.01.001
Baltzegar, D., Reading, B., Douros, J., and Borski, R. (2015). Role for leptin in promoting glucose mobilization during acute hyperosmotic stress in teleost fishes. J. Endocrinol. 220, 61–72. doi: 10.1530/JOE-13-0292
Ben-Moshe, Z., Alon, S., Vallone, D., Bayleyen, Y., Tovin, A., Shainer, I., et al. (2016). Genetically blocking the zebrafish pineal clock affects circadian behavior. PLoS Genet. 12:e1006445. doi: 10.1371/journal.pgen.1006445
Butler, A., and LeRoith, D. (2001). Control of growth by the somatotrophic axis: growth hormone and the insulin-like growth factors have related and independent roles. Annu. Rev. Physiol. 63, 141–164. doi: 10.1146/annurev.physiol.63.1.141
Cowan, M., Azpeleta, C., and López-Olmeda, J. (2017). Rhythms in the endocrine system of fish: a review. J. Comp. Physiol. B 187, 1057–1089. doi: 10.1007/s00360-017-1094-5
Dai, X., Zhang, W., Zhuo, Z., He, J., and Yin, Z. (2015). Neuroendocrine regulation of somatic growth in fishes. Sci. China Life. Sci. 58, 137–147. doi: 10.1007/s11427-015-4805-8
Davies, W., Tamai, K., Zheng, L., Fu, J., Rihel, J., Foster, R., et al. (2015). An extended family of novel vertebrate photopigments is widely expressed and displays a diversity of function. Gen. Res. 25, 1666–1679. doi: 10.1101/gr.189886.115
Deane, E., and Woo, N. (2009). Modulation of fish growth hormone levels by salinity, temperature, pollutants and aquaculture related stress: a review. Rev. Fish. Biol. Fish. 19, 97–120. doi: 10.1007/s11160-008-9091-0
Delaunay, F., Thisse, C., Thisse, B., and Laudet, V. (2003). Differential regulation of period2 and period3 expression during development on the zebrafish circadian clock. Gene Expr. Patterns 3, 319–324. doi: 10.1016/S1567-133X(03)00050-4
Doyle, S., and Menaker, M. (2007). Circadian photoreception in vertebrates. Cold Spring Harb. Symp. Quant. Biol. 72, 499–508. doi: 10.1101/sqb.2007.72.003
Egan, R., Bergner, C., Hart, P., Cachat, J., Canavello, P., Elegante, M., et al. (2009). Understanding behavioral and physiological phenotypes of stress and anxiety in zebrafish. Behav. Brain Res. 205, 38–44. doi: 10.1016/j.bbr.2009.06.022
Falcón, J., Besseau, L., Fuentès, M., Sauzet, S., Magnanou, E., and Boeuf, G. (2009). Structural and functional evolution of the pineal melatonin system in vertebrates. Ann. N. Y. Acad. Sci. 1163, 101–111. doi: 10.1111/j.1749-6632.2009.04435.x
Falcón, J., Besseau, L., Sauzet, S., and Boeuf, G. (2007). Melatonin effects on the hypothalamo-pituitary axis in fish. Trends Endocrinol. Metab. 18, 81–88. doi: 10.1016/j.tem.2007.01.002
Faught, E., Aluru, N., and Vijayan, M. (2016). The molecular stress response. Fish Physiol. 35, 113–166. doi: 10.1016/B978-0-12-802728-8.00004-7
Faught, E., and Vijayan, M. (2016). Mechanisms of cortisol action in fish hepatocytes. Comp. Biochem. Physiol. B Biochem. Mol. Biol. 199, 136–145. doi: 10.1016/j.cbpb.2016.06.012
Gabillard, J., Rescan, P., Weil, C., Fauconneau, B., and Le Bail, P. (2003). Effects of temperature on GH/IGF system gene expression during embryonic development of rainbow trout (Oncorhynchus mykiss). J. Exp. Zool. 298, 134–142. doi: 10.1002/jez.a.10280
Gabillard, J., Yao, K., Vandeputte, M., Guitierrez, J., and Le Bail, P. (2006). Differential expression of two GH receptor mRNA following temperature change in rainbow trout (Oncorhynchus mykiss). J. Endocrinol. 190, 29–37. doi: 10.1677/joe.1.06695
Gesto, M., Álvarez-Otero, R., Conde-Sieira, M., Otero-Rodi-o, C., Usandizaga, S., Soengas, J., et al. (2016). A simple melatonin treatment protocol attenuates the response to acute stress in the sole Solea senegalensis. Aquaculture 452, 272–282. doi: 10.1016/j.aquaculture.2015.11.006
Gordon, J. (2005). Temperature and Toxicology: And Integrative, Comparative, and Environmental Approach, 1st Edn. Boca Raton, FL: CRC Press.
Grønkjær, P. (2016). Otoliths as individual indicators: a reappraisal of the link between fish physiology and otolith characteristics. Mar. Freshw. Res. 67, 881–888. doi: 10.1071/MF15155
Hastings, M., O’Neill, J., and Maywood, E. (2007). Circadian clocks: regulators of endocrine and metabolic rhythms. J. Endocrinol. 195, 187–198. doi: 10.1677/JOE-07-0378
Jerônimo, R., Moraes, M., de Assis, L., Ramos, B., Rocha, T., and Castrucci, A. (2017). Thermal stress in Danio rerio: a link between temperature, light, thermo-TRP channels, and clock genes. J. Therm. Biol. 68, 128–138. doi: 10.1016/j.jtherbio.2017.02.009
Kaneko, M., and Cahill, G. (2005). Light-dependent development of circadian gene expression in transgenic zebrafish. PLoS Biol. 3:e34. doi: 10.1371/journal.pbio.0030034
Klein, S., and Sheridan, M. (2008). Somatostatin signaling and the regulation of growth and metabolism in fish. Mol. Cell. Endocrinol. 286, 148–154. doi: 10.1016/j.mce.2007.08.010
Lahiri, K., Vallone, D., Gondi, S., Santoriello, C., Dickmeis, T., and Foulkes, N. (2005). Temperature regulates transcription in the zebrafish circadian clock. PLoS Biol. 3:e351. doi: 10.1371/journal.pbio.0030351
Leroith, D., Bondy, C., Yakar, S., Liu, J., and Buter, A. (2001). The somatomedin hypothesis: 2001. Endocr. Rev. 22, 53–74. doi: 10.1210/edrv.22.1.0419
Livak, K., and Schmittgen, T. (2001). Analysis of relative gene expression data using real-time quantitative PCR and the 2(-delta delta C(T)) method. Methods 25, 402–408. doi: 10.1006/meth.2001.1262
Loeppky, A., Belding, L., Quijada-Rodriguez, A., Morgan, J., Pracheil, B., Chakoumakos, B., et al. (2021). Influence of ontogenetic development, temperature, and pCO2 on otolith calcium carbonate polymorph composition in sturgeons. Sci. Rep. 11:13878. doi: 10.1038/s41598-021-93197-6
Loganathan, K., Moriya, S., and Parhar, I. (2018). High melatonin conditions by constant darkness and high temperature differently affect melatonin receptor mt1 and TREK channel trek2a in the brain of Zebrafish. Zebrafish 5, 473–483. doi: 10.1089/zeb.2018.1594
Long, Y., Li, L., Li, Q., He, X., and Cui, Z. (2012). Transcriptomic characterization of temperature stress responses in larval Zebrafish. PLoS One 7:e37209. doi: 10.1371/journal.pone.0037209
Long, Y., Song, G., Yan, J., He, X., Li, Q., and Cui, Z. (2013). Transcriptomic characterization of cold acclimation in larval Zebrafish. BMC Genomics 14:612. doi: 10.1186/1471-2164-14-612
López-Olmeda, J., and Sánchez-Vázquez, F. (2011). Thermal biology of zebrafish (Danio rerio). J. Therm. Biol. 36, 91–104. doi: 10.1016/j.jtherbio.2010.12.005
Masana, M., and Dubocovich, M. (2001). Melatonin receptor signaling: finding the path through the dark. Sci. STKE 107:pe39. doi: 10.1126/stke.2001.107.pe39
Mogi, M., Yokoi, H., and Suzuki, T. (2017). Analyses of the cellular clock gene expression in peripheral tissue, caudal fin, in the Japanese flounder, Paralichthys olivaceus. Gen. Comp. Endocrinol. 248, 97–105. doi: 10.1016/j.ygcen.2017.02.009
Mohammed, G., Martos, J., Galal, A., Mancera, J., and Martínez, G. (2015). Insulin-like growth factor 1 (IGF-1) regulates prolactin, growth hormone, and IGF-1 receptor expression in the pituitary gland of the gilthead sea bream Sparus aurata. Fish Physiol. Biochem. 42, 365–377. doi: 10.1007/s10695-015-0144-8
Molijn, G., Koper, J., van Uffelen, C., de Jong, F., Brinkmann, A., Bruinlng, H., et al. (1995). Temperature-induced down-regulation of the glucocorticoid receptor in peripheral blood mononuclear leucocyte in patients with sepsis or septic shock. Clin. Endocrinol. 43, 197–203. doi: 10.1111/j.1365-2265.1995.tb01915.x
Ngasainao, M., and Lukram, I. (2016). A review on melatonin and its prospects in fish aquaculture. J. Zool. Sci. 4, 34–41.
Nisembaum, L., Besseau, L., Paulin, C., Charpantier, A., Martin, P., Magnanou, E., et al. (2015). In the heat of the night: thermo-TRPV channels in the salmonid pineal photoreceptors and modulation of melatonin secretion. Endocrinology 156, 4629–4638. doi: 10.1210/en.2015-1684
Oliveira, J., Silveira, M., Chacon, D., and Luchiari, A. (2015). The zebrafish world of colors and shapes: preference and discrimination. Zebrafish 12, 166–173. doi: 10.1089/zeb.2014.1019
Peterson, B., Waldbieser, G., and Bilodeau, L. (2005). Effects of recombinant bovine somatotropin on growth and abundance of mRNA for IGF-I and IGF-II in channel catfish (Ictalurus punctatus). J. Anim. Sci. 83, 816–824. doi: 10.2527/2005.834816x
Prokkola, J., Nikinmaa, M., Lewis, M., Anttila, K., Kanerva, M., Ikkala, K., et al. (2018). Cold temperature represses daily rhythms in the liver transcriptome of a stenothermal teleost under decreasing day length. J. Exp. Biol. 221:jeb170670. doi: 10.1242/jeb.170670
Quera, S., and Hartley, S. (2012). Mood disorders, circadian rhythms, melatonin and melatonin agonists. J. Cent. Nerv. Syst. Dis. 4, 15–26. doi: 10.4137/JCNSD.S4103
Ramsay, J., Feist, G., Varga, Z., Westerfield, M., Kent, M., and Schreck, C. (2009). Whole-body cortisol response of zebrafish to acute net handling stress. Aquaculture 297, 157–162. doi: 10.1016/j.aquaculture.2009.08.035
Reindl, K. M., and Sheridan, M. A. (2012). Peripheral regulation of the growth hormone-insulin-like growth factor system in fish and other vertebrates. Comp. Biochem. Physiol. A Mol. Integr. Physiol. 163, 231–245. doi: 10.1016/j.cbpa.2012.08.003
Reindl, K., Kittilson, J., Bergan, H., and Sheridan, M. (2011). Growth hormone-stimulated insulin-like growth factor-1 expression in rainbow trout (Oncorhynchus mykiss) hepatocytes is mediated by ERK, PI3K-AKT, and JAK-STAT. Am. J. Physiol. Regul. Integr. Comp. Physiol. 301, 236–243. doi: 10.1152/ajpregu.00414.2010
Rensing, L., and Ruoff, P. (2002). Temperature effect on entrainment, phase shifting, and amplitude of circadian clocks and its molecular bases. Chronobiol. Int. 19, 807–864. doi: 10.1081/CBI-120014569
Rudy, D., and Forsberg, J. (2016). “Chapter 5: Biology and ecosystem research. Chapter 5.2: An examination of otolith growth increments in relation to somatic growth for Pacific halibut (Hippoglossus stenolepis),” in Report Assessment and Research Activities. (Seattle, WA: International Pacific Halibut Commission).
Sánchez-Vázquez, F., López-Olmeda, J., Vera, L., Migaud, H., López-Patiño, M., and Míguez, J. (2019). Environmental cycles, melatonin, and circadian control of stress response in fish. Front. Endocrinol. 10:279. doi: 10.3389/fendo.2019.00279
Sousa, J., Magalhaes, K., Da Silveira Cruz Machado, S., Moraes, M., and Castrucci, A. (2017). Dexamethasone modulates non-visual opsins, glucocorticoid receptor and clock genes in Danio rerio ZEM-2S cells. Biomed Res. Int. 2017:8459385. doi: 10.1155/2017/8459385
Torres-Farfan, C., Richter, H., Rojas, P., Vergara, M., Forcelledo, M., Valladares, L., et al. (2003). mt1 Melatonin receptor in the primate adrenal gland: inhibition of adrenocorticotropin-stimulated cortisol production by melatonin. J. Clin. Endocrinol. Metab. 88, 450–458. doi: 10.1210/jc.2002-021048
Vallone, D., Frigato, E., Vernesi, C., Foá, A., Foulkes, N., and Bertolucci, C. (2007). Hypothermia modulates circadian clock gene expression in lizard peripheral tissues. Am. J. Physiol. Regul. Integr. Comp. Pysiol. 292, R160–R166. doi: 10.1152/ajpregu.00370.2006
Vallone, D., Lahiri, K., Dickmeis, T., and Foulkes, N. (2005). Zebrafish cell clocks feel the heat and see the light! Zebrafish 2, 171–187. doi: 10.1089/zeb.2005.2.171
Vatine, G., Vallone, D., Gothilf, Y., and Foulkes, N. (2011). It’s time to swim! Zebrafish and the circadian clock. FEBS Lett. 585, 1485–1494. doi: 10.1016/j.febslet.2011.04.007
Vera, L., De Oliveira, C., López-Olmeda, J., Ramos, J., Mananos, E., Madrid, J., et al. (2007). Seasonal and daily plasma melatonin rhythms and reproduction in Senegal sole kept under natural photoperiod and natural or controlled water temperature. J. Pineal Res. 43, 50–55. doi: 10.1111/j.1600-079X.2007.00442.x
Wang, H. (2008). Comparative analysis of period genes in teleost fish genomes. J. Mol. Evol. 67, 29–40. doi: 10.1007/s00239-008-9121-5
Wang, J., Song, Q., Yu, D., Yang, G., Xia, L., Su, K., et al. (2015). Ontogenetic development of the auditory sensory organ in zebrafish (Danio rerio): changes in hearing sensitivity and related morphology. Sci. Rep. 5:15943. doi: 10.1038/srep15943
West, A., and Bechtold, D. (2015). The cost of circadian desynchrony: evidence, insights and open questions. Bioessays 37, 777–788. doi: 10.1002/bies.201400173
White, C., Alton, L., and Frappell, P. (2012). Metabolic cold adaptation in fishes occurs at the level of whole animal, mitochondria and enzyme. Proc. Biol. Sci. 279, 1740–1747. doi: 10.1098/rspb.2011.2060
Yamamoto, T., Nakahata, Y., Tanaka, M., Yoshida, M., Soma, H., Shinohara, K., et al. (2005). Acute physical stress elevates mouse period1 mRNA expression in mouse peripheral tissues via a glucocorticoid-responsive element. J. Biol. Chem. 280, 42036–42043. doi: 10.1074/jbc.M509600200
Yumnamcha, T., Ahmad, Z., Rajiv, C., Dharmajyoti, D., Mondal, G., Sanjita, H., et al. (2017). Interaction of melatonin and gonadotropin-inhibitory hormone on the Zebrafish brain-pituitary axis. Mol. Reprod. Dev. 84, 389–400. doi: 10.1002/mrd.22795
Zhang, E., Liu, Y., Dentin, R., Pongsawakul, P., Liu, A., Hirota, T., et al. (2010). Cryptochrome mediates circadian regulation of cAMP signaling and hepatic gluconeogenesis. Nat. Med. 16, 1152–1156. doi: 10.1038/nm.2214
Zhao, Y., Zhang, K., and Fent, K. (2016). Corticosteroid fludrocortisone acetate targets multiple end points in zebrafish (Danio rerio) at low concentrations. Environ. Sci. Technol. 50, 10245–10254. doi: 10.1021/acs.est.6b03436
Keywords: Danio rerio, temperature, hormones, clock genes, hormone receptors
Citation: Sua-Cespedes CD, David DD, Souto-Neto JA, Lima OG, Moraes MN, de Assis LVM and Castrucci AML (2021) Low Temperature Effect on the Endocrine and Circadian Systems of Adult Danio rerio. Front. Physiol. 12:707067. doi: 10.3389/fphys.2021.707067
Received: 08 May 2021; Accepted: 19 October 2021;
Published: 24 November 2021.
Edited by:
Cristiano Bertolucci, University of Ferrara, ItalyReviewed by:
Mattia Toni, Sapienza University of Rome, ItalyJose Fernando Lopez-Olmeda, University of Murcia, Spain
Copyright © 2021 Sua-Cespedes, David, Souto-Neto, Lima, Moraes, de Assis and Castrucci. This is an open-access article distributed under the terms of the Creative Commons Attribution License (CC BY). The use, distribution or reproduction in other forums is permitted, provided the original author(s) and the copyright owner(s) are credited and that the original publication in this journal is cited, in accordance with accepted academic practice. No use, distribution or reproduction is permitted which does not comply with these terms.
*Correspondence: Ana Maria de Lauro Castrucci, YW1kbGNhc3RAaWIudXNwLmJy