- 1Military Performance Division, United States Army Research Institute of Environmental Medicine, Natick, MA, United States
- 2Military Nutrition Division, United States Army Research Institute of Environmental Medicine, Natick, MA, United States
- 3Oak Ridge Institute for Science and Education, Oak Ridge, TN, United States
Muscle quality (MQ), defined as the amount of strength and/or power per unit of muscle mass, is a novel index of functional capacity that is increasingly relied upon as a critical biomarker of muscle health in low functioning aging and pathophysiological adult populations. Understanding the phenotypical attributes of MQ and how to use it as an assessment tool to explore the efficacy of resistance exercise training interventions that prioritize functional enhancement over increases in muscle size may have implications for populations beyond compromised adults, including healthy young adults who routinely perform physically demanding tasks for competitive or occupational purposes. However, MQ has received far less attention in healthy young populations than it has in compromised adults. Researchers and practitioners continue to rely upon static measures of lean mass or isolated measures of strength and power, rather than using MQ, to assess integrated functional responses to resistance exercise training and physical stress. Therefore, this review will critically examine MQ and the evidence base to establish this metric as a practical and important biomarker for functional capacity and performance in healthy, young populations. Interventions that enhance MQ, such as high-intensity stretch shortening contraction resistance exercise training, will be highlighted. Finally, we will explore the potential to leverage MQ as a practical assessment tool to evaluate function and enhance performance in young populations in non-traditional research settings.
Introduction
The ability of skeletal muscle to produce substantial force in relatively short periods of time is crucial to success in many sporting events, tactical operations, and activities of daily living. While the focus of improving muscular force generation traditionally centers on increasing muscle quantity (i.e., muscle hypertrophy), recent efforts have focused on improving muscle quality (MQ) for enhancing muscle function. MQ is an umbrella term referring to the ability of skeletal muscle to perform several functions effectively, including force production, contraction and relaxation, metabolism, substrate turnover and storage, heat generation, myokine production, and electrical conduction (Fragala et al., 2015; Correa-de-Araujo et al., 2017). Although no consensus definition of MQ exists as of yet, the ambiguity of the term has allowed investigators to examine several dimensions of MQ that relate to muscle function. As the most prominent function of skeletal muscle is force production, the present review will focus on MQ in this context; however, the role of skeletal muscle in other biological functions should not be overlooked.
In applied and clinical research settings, MQ is often quantified as muscle strength or power per unit of muscle mass (Barbat-Artigas et al., 2012; McGregor et al., 2014), commonly reported as peak force or maximal power production relative to a measure of muscle size, such as muscle volume or cross-sectional area (CSA). This definition accounts for the functional significance of the architecture, the overall tissue characteristics of the skeletal muscle, and ultimately, force production capabilities (Mangine et al., 2020). Increased force output as a product of increased muscle mass is a well-established physiological concept (Bamman et al., 2000); however, research demonstrates that force generation is largely a product of skeletal MQ aside from muscle quantity (Ivey et al., 2000; Brooks et al., 2006; Goodpaster et al., 2006; Fukumoto et al., 2012). The distinction between MQ and muscle quantity is an important aspect of functional assessment, as two individuals with similar muscle mass may not be capable of producing equivalent amounts of force. Similarly and counterintuitively, individuals with smaller amounts of muscle mass may be more effective at producing force than those with greater amounts. Differences in MQ between individuals may help explain why certain muscles are stronger than others, despite similar sizes. However, MQ may also vary between limbs, generating potential for injury if not properly corrected.
Muscle quality has largely been studied in aging and adult populations with pathophysiological conditions, and in those studies, higher MQ is related to increased muscle strength, function, and physical performance, and vice versa (Fragala et al., 2015; Brown et al., 2016). As posited in this review, understanding the phenotypic attributes of MQ and using MQ as an assessment tool to explore the efficacy of exercise training that prioritizes functional enhancement over increases in muscle size may have implications for populations beyond compromised adults, including healthy young adults who routinely perform physically demanding tasks for competitive or occupational purposes. Accordingly, this review will critically examine MQ and the evidence base that supports MQ as a practical and important biomarker for functional capacity and performance in healthy, young populations. Interventions that augment MQ, such as high-intensity stretch shortening contraction resistance exercise training (RET), will be highlighted. Finally, we will explore the potential to leverage MQ as a practical assessment tool to evaluate function and performance enhancement in young populations in non-traditional research settings.
Discerning MQ From Muscle Size Alone
One of the most important features of muscle is that it is dynamically regulated by changes in physical demands, as well as environmental challenges, and is remarkably plastic in response to various forms of stimuli (Caldow et al., 2015). One of the defining characteristics of exercise is its ability to initiate a complex assortment of highly coordinated molecular and cellular signaling events, as well as transcriptional regulators, which in turn have profound positive effects on muscle tissue (Bamman et al., 2014). Upon exposure to physical activity demands, muscle responds in a coordinated fashion by initiating various signaling events that drive changes in gene expression of intracellular processes, such as metabolism, alterations in hormone signaling, defense mechanisms against oxidative stress, DNA damage and repair, protein turnover, and muscle remodeling (Phillips et al., 2013; Camera et al., 2016). Thus, customary stimuli by appropriate activity or exercise prompt changes in gene expression, which ultimately results in an integrated physiological and functional adaptation in skeletal muscle and the surrounding soft tissue. Therefore, by solely examining changes in muscle size with exercise, the true exquisiteness of skeletal muscle functional adaptation is lost.
While the relationship between increased muscle size and increased force output is well-established, force generation is a result of several factors aside from exclusively mass (Ivey et al., 2000; Goodpaster et al., 2006; Fukumoto et al., 2012). The relationship between size and strength is not linear, as strength can be improved independently of significant increases in muscle growth (Parente et al., 2008; Dankel et al., 2017; Mattocks et al., 2017), and likewise, increases in size can occur without concomitant increases in force production (Ahtiainen et al., 2016; Jakobsgaard et al., 2018). Thus, beyond muscle size, other factors such as tissue composition (e.g., contractile and non-contractile components), architecture, metabolism, and neural activation contribute to the overall force production capabilities (McGregor et al., 2014). For example, lower-body CSA and volume are associated with greater adiposity (Varanoske et al., 2017), indicating that increased intramuscular fat may account for greater muscle volume. However, in this case, greater muscle size will likely not translate to greater force production. Factors contributing to muscle strength and size are listed in Table 1.
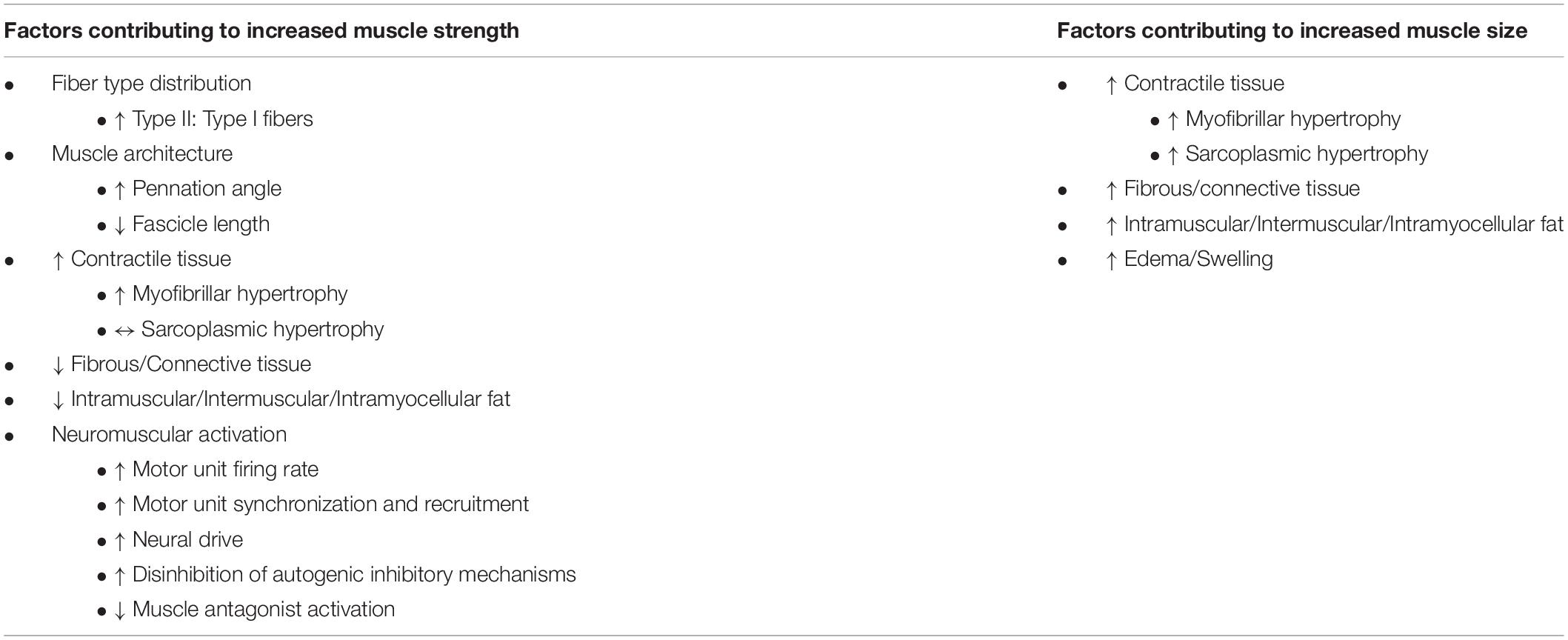
Table 1. Factors contributing to increased muscle strength and size, which are important determinants of MQ.
In this regard, muscle architectural characteristics that affect force production may change following RET, leading to improved muscle strength, power, and subsequently overall MQ. For instance, increases in fascicle length and pennation angle have been observed following RET (Kawakami et al., 1995; Aagaard et al., 2001; Blazevich et al., 2007; Seynnes et al., 2007; Baroni et al., 2013; Ema et al., 2013; Franchi et al., 2014, 2016; McMahon et al., 2014), with RET-induced increases in pennation angle implied to be associated with increased force production attributable to the degree of muscle hypertrophy experienced (Azizi and Brainerd, 2007; Ema et al., 2013). Interestingly, increases in fascicle length do not always accompany significant muscle hypertrophy (Fukutani and Kurihara, 2015), demonstrating that muscle force production is greater than just a factor of its size. Thus, measures of size alone, such as muscle CSA, inadequately estimate force production. This is why in recent years, sarcopenia has evolved from being defined exclusively as the age-related loss of muscle mass to more contemporary definitions that account for loss of strength or physical performance (Cruz-Jentoft et al., 2019). However, this same line of thinking has yet to be applied to younger populations, including research, clinical, and standard occupational assessment settings, as measures of body composition, muscle mass, and/or static strength continue to be prevalent in these environments.
Relying exclusively on measures of strength (e.g., handgrip), size (e.g., CSA), or performance alone likely limits our ability to draw meaningful conclusions about the effectiveness of a training intervention because they do not tell a complete story. For instance, military body fat standards, which emphasize abdominal circumference measures and/or bioelectrical impedance analysis of body composition, not only have their own flaws when it comes to accuracy and reliability, but are also outdated because recruits today tend to have more body fat and more lean mass compared to previous generations, which aids in their ability to perform lifting and carrying tasks (Foulis et al., 2020). Moreover, there is an increased prevalence of those soldiers who are metabolically obese but normal weight, with low muscle mass and thus overall poor function (Foulis et al., 2020). Absolute handgrip strength is often used in clinical and research settings as a measure of muscle strength and function, but research has demonstrated that this is not the best indicator of function and that forearm MQ is a stronger predictor of physical performance (Abe et al., 2016). Because the majority of physically demanding tasks performed by younger individuals (e.g., load carriage during military operations) requires a combination of muscle strength, power, and size in order to maximize the potential of these individuals to perform their duties (Szivak, 2020), MQ should be viewed as a critically important metric for evaluating the capabilities of these individuals to maintain effective performance and reduce the likelihood of injury prior to deployment.
Assessment of MQ
Measures of muscle size and function are often integrated into a single measure of MQ, termed relative strength (Correa-de-Araujo et al., 2017), which is more informative for laboratory or field studies when findings demonstrate improvements or declines in performance in response to a given stressor, or changes in muscle mass without associated changes in function. Relative strength can be calculated by measuring the strength or power of a muscle/muscle group divided by muscle mass. However, several methodologies of measuring muscle size and strength exist, resulting in limited consensus on the most appropriate way of assessing MQ. For instance, physical movement is often the result of several muscles working in synchrony to produce a desired effect, and thus, it becomes difficult to isolate the strength of an individual muscle. Additionally, differences in the type of exercise assessment administered (e.g., isometric, isokinetic, isotonic, dynamic, single-joint, and multi-joint, etc.) will result in different strength values, diminishing the predictive ability of relative strength measures. In this regard, Wheeler et al. (2014) used a specialized statistical approach and applied it to studies previously conducted in young and old rats that were subjected to a previous animal RET protocol (Cutlip et al., 2006; Murlasits et al., 2006; Baker et al., 2010) and demonstrated that dynamic forces were more robust and specific for representing the occurrence of skeletal muscle adaptation compared to isometric values.
The examination of muscle composition may provide additional insight into MQ which can circumvent some of the limitations associated with muscle strength measures. The Bergstrom needle biopsy technique (Bergstrom, 1975) provides useful information about fiber type distribution, muscle capillarization, intramuscular fat content, and muscle metabolism, which aid in predicting muscle function; however, invasive muscle biopsies may not be appropriate for routine MQ assessment. Advancements in fine needle biopsies techniques allow for a less-invasive immunohistological and morphological analysis of muscle tissue (Townsend et al., 2016), but this method still requires repeated muscle sample collection, which may not be practical for most populations. In contrast, muscle imaging techniques, such as computed tomography (CT), peripheral quantitative computed tomography (pQCT) magnetic resonance imaging (MRI), and ultrasonography (US) serve as non-invasive approaches for assessing muscle size and tissue composition, allowing for surrogate measures of MQ that can be derived independently of muscle strength measures. Previous studies have reported skeletal muscle-based outcome measures using dual x-ray absorptiometry (DXA) in various healthy populations following RET despite the notion that DXA cannot distinguish between muscle mass and MQ (Heymsfield et al., 2015; Phillips et al., 2017). Anthropometric measurements that are commonly used to represent skeletal muscle health, such as skinfolds and circumferences, not only have their own inherent limitations such as poor precision/reproducibility, they provide no information pertaining to the underlying contractile and non-contractile tissue components that influence functional outcomes and account for disconnects between size and force production (Heymsfield et al., 2015). Therefore, muscle imaging may be preferred for MQ assessment when muscle strength measures cannot be obtained or when muscle composition estimates are also desired, as relative strength measures do not provide any information on muscle composition. However, the reliability of MQ measures are dependent on the method of examination. For example, the reliability of echo intensity measured via ultrasonography will be different from that of radiological density measured by MRI, which will be different from that of relative strength. Nevertheless, all methods of assessing MQ mentioned herein have been reported to be reliable across different populations and are widely cited throughout the literature. Advantages and disadvantages of these imaging techniques used to measure MQ are presented in Table 2.
CT produces high-resolution, cross-sectional images of body “slices,” which can be combined to estimate muscle volumes and whole body muscle mass (Heymsfield et al., 2015; Correa-de-Araujo et al., 2020). However, CT is unable to distinguish fibrotic tissue from normal muscle tissue, limiting the ability of CT to provide measures of MQ that exclude impacts of fat infiltration. CT is also unable to isolate individual muscle groups or distinguish the location of intramuscular fat. (Goodpaster, 2002; Machann et al., 2003). Advancements in CT technology have resulted in the development of pQCT machines, which enable 3-dimensional CT scans of the peripheral skeleton. Advantages of pQCT over CT include its lower cost, increased portability and smaller size, shorter scan time, and lower emittance of radiation (Erlandson et al., 2016, 2017). However, pQCT shares some of the same limitations in that it is incapable of isolating individual muscle groups and cannot determine the location of intramuscular fat. MRI is often considered the gold standard method for measuring muscle size and CSA (Ahtiainen et al., 2010) and provides high-resolution images of body tissues, making visualization easy (Pillen and van Alfen, 2011).
MRI is also more sensitive than CT for detecting muscle fat infiltration and visualizing anatomical features (Gloor et al., 2011). Furthermore, MRI assesses the detailed structure and composition of individual muscles and differentiates between edema, fatty infiltration, and fibrosis (Erlandson et al., 2016).
While there is currently no gold standard imaging device to assess MQ, the use of US has become increasingly prevalent in recent research due to the low cost, safety, portability, and reliability as an imaging device. US has recently emerged as one of the most popular ways to assess muscle morphology; like MRI, US does not emit radiation, making it attractive for repeated use. US-derived measures of muscle CSA have demonstrated high reliability and accuracy in comparison to other imaging techniques (Ahtiainen et al., 2010; Noorkoiv et al., 2010; Scott et al., 2012). MQ measures are based off of echo intensity (EI), or brightness, of the tissue in the US image. Thus, muscle with a greater proportion of non-contractile tissue appears brighter in color, accompanied by a higher EI value, and ultimately lower MQ. Several studies report that EI is related to muscle strength, power, and function across the population spectrum, demonstrating that EI may be a proxy measure of MQ (Jajtner et al., 2013; Watanabe et al., 2013; Fragala et al., 2014b; Mangine et al., 2015a; Stock and Thompson, 2020; Wong et al., 2020).
Exercise and MQ
In general, exercise training elicits positive adaptations to MQ. However, as outlined in Table 3, in comparison to other exercise training regimens such as aerobic exercise training or hypertrophy-based moderate intensity resistance exercise, high-intensity RET appears to be the primary intervention for improving force production, power development, and subsequently augmenting MQ as well as overall muscle function, especially when consisting of stretch-shortening contractions (SSCs) (Fry, 2004; Rader and Baker, 2017; Rader et al., 2018a; Szivak, 2020; Duchateau et al., 2021). SSCs consist of sequential isometric, eccentric, and concentric contractions, such as the squat, during which the muscle is activated prior to (isometric - this represents the stabilization phase when holding the bar prior to the descent) as well as during the initial stretch/lengthening (eccentric- the descending portion of the squat) and subsequent shortening (concentric- the ascending portion of the squat) (Cutlip et al., 2006; Rader and Baker, 2017; Rader et al., 2017b). SSCs provide a significant advantage over isolated isometric-, concentric-, or eccentric-only contractions because they are more physiologically relevant as the typical contraction sequence utilized by mammals that occur during activities of daily living as well as the majority of exercise movements (e.g., walking, sprinting, resistance training, and plyometrics) (Vaczi et al., 2014; Rader and Baker, 2017, 2020; Rader et al., 2018a). As most traditional free-weight RET paradigms incorporate SSCs as part of the typical routine (i.e., most training programs do not isolate a single type of contraction), we will refer to RET using SSCs as “RET” for the remainder of the paper. Common physiological adaptations promoted specifically by a high-intensity RET stimulus (≥70% one-repetition maximum, 1RM) include an augmentation of muscle capillarization, reduced muscle lipid content, increased motor unit recruitment, and a selective increase in the number of type II muscle fibers that are activated during training, which are able to generate the greatest force output and have the highest capability for growth (Frontera et al., 1988; Hepple et al., 1997; Hagerman et al., 2000; Vaczi et al., 2014; Rader and Baker, 2017; Szivak, 2020). While increases in muscle mass have been reported to occur over a broader range of intensities (American College of Sports Medicine, 2009; Shoepe et al., 2020), preferential increases in type II muscle fiber CSA have been shown specifically through high-intensity training protocols (Fry, 2004; Vann et al., 2020).
MQ and High-Intensity RET in Young Populations
Despite the critical importance of MQ in meeting the requirements of physically demanding tasks, a comprehensive summary of the effects of enhanced MQ associated with specific exercise regimens has not focused on younger populations in highly physically demanding occupations, such as the military. Synthesizing this information is highly relevant for making evidence-based recommendations for improving MQ in younger populations who regularly engage in physical tasks where optimizing muscle function is of high importance. Before providing a review of studies, it is important to note that the classification of what is appropriately labeled “high-intensity” appears to be quite ambiguous and often differs between researchers (Steele, 2014; Steele et al., 2017). However, there is a general consensus that, when relating intensity to load, ≥70% of 1RM is the minimum threshold for being considered high-intensity (Kristensen and Franklyn-Miller, 2012; Steele, 2014). Therefore, we considered and discussed studies that directly measured MQ where intensity as it relates to load was exclusively or predominantly at ≥ 70% or greater 1RM, while recognizing the limitation of the lack of clarity on a consensus definition. For this review, only studies that utilized SSCs were considered, while those employing isometric, concentric-, or eccentric-only training were excluded given the advantages of this contraction sequence. In addition to these standards, other criteria for inclusion were training studies with a minimum duration of 4 weeks, a minimum training frequency of two times per week, and the requirement that there was at least one metric of skeletal muscle performance/strength and/or muscle mass reported in the study.
Animal-based rodent models have provided an important and fundamental approach to studying MQ responses in young muscle because of the capability to tightly control high-intensity RET paradigms and precisely assess components of MQ. As shown in Table 4, young rats have consistently and robustly responded to an in vivo dynamometer model of SSC-loading with a training-induced adaptation in the tibialis anterior (TA), a muscle predominantly comprised of type II fibers (>90%) (Delp and Pette, 1994). This adaptive response is reflected by a ∼15–100% increase in isometric and dynamic skeletal muscle performance as well as a roughly ∼10–25% increase in TA muscle wet-weight, depending on the specific metric utilized and selected method of quantification, ultimately resulting in a robust enhancement of MQ. Additionally, young mice subjected to the same model and training protocol to the plantar flexor muscles responded with a ∼20% increase in dynamic skeletal muscle performance, but with no change in plantaris muscle mass. The other primary model included in Table 4 was a recently developed custom-built voluntary weightlifting apparatus designed for mice to perform squat-like activities against an adjustable load during feeding (Cui et al., 2020). Using this model, substantial increases in plantar flexor contractile force, muscle CSA, and MQ (plantar flexor torque forces normalized to muscle mass) were observed.
Besides the summary of the results for MQ provided in Table 4, a key observation regarding fiber type distribution has been that young rats (3 month old) have demonstrated a transition to a more oxidative phenotype with high-intensity RET, either from type IIb to IIx (Rader et al., 2016, 2017a) or type IIx to IIa (Aguiar et al., 2013). Also, with respect to adult skeletal muscle, results from a study (Rader et al., 2016) indicated there was an altered phenotype occurring prior to complete biological development of rodents, which generally occurs by 6 months of age, or young adulthood. This was highlighted by impairments in the adaptive capacity due to a blunted MQ response following the same high-intensity RET protocol in comparison to 3-month-old rats who had markedly increased MQ (Cooper et al., 2015; Rader et al., 2016). These results indicate that skeletal muscle can potentially experience declines in its ability to adapt with age, including at the onset of young adulthood. These outcomes, should they hold true for humans, have important implications for young adult military personnel who are still expected to perform at high levels of function during physically demanding tasks.
The effects of high-intensity RET (>70% 1RM) on MQ, as defined by muscle strength relative to mass, has been investigated in young, healthy human populations (Table 5). Collectively, following high-intensity RET, all but one of these studies showed significant improvements in muscle strength/performance (∼4–75%), and all seven studies showed increases in muscle mass (∼4–35%). Ultimately, these studies had an overall ∼8.5–35.0% increase in MQ following RET. Of these studies, four used untrained participants (Cureton et al., 1988; Chestnut and Docherty, 1999; Ivey et al., 2000) and in two others, training status was not reported (Dons et al., 1979; Welle et al., 1996). It is important to acknowledge that untrained individuals respond differently to RET than trained individuals. In the reviewed studies, untrained individuals respond positively to the vast majority of RET programs, and thus specific nuances to the exercise prescription are less critical (Aagaard and Andersen, 1998). Castro et al. (1995) found trained participants had significantly greater mean torque per unit of muscle and bone CSA for the upper arm (28.9%) and thigh (18.8%) compared to untrained participants following RET (Castro et al., 1995), indicating that trained participants potentially have greater capabilities to augment MQ in response to high-intensity RET. Two of the aforementioned studies made direct comparisons between different RET intensities. One study found MQ to be 30% higher at post-training in a group that trained at 80% 1RM, whereas there was only a 5% increase in post-training MQ in a 50% 1RM group (Dons et al., 1979). In the other study, there were no changes in MQ between 4RM vs. 10RM group (Cureton et al., 1988). However, since both groups trained at relatively high intensities of 85% and 70% 1RM, respectively, along with the lack of other available direct comparisons, it is difficult to draw any conclusions on the effects of specific intensities on MQ in young populations following high-intensity RET.
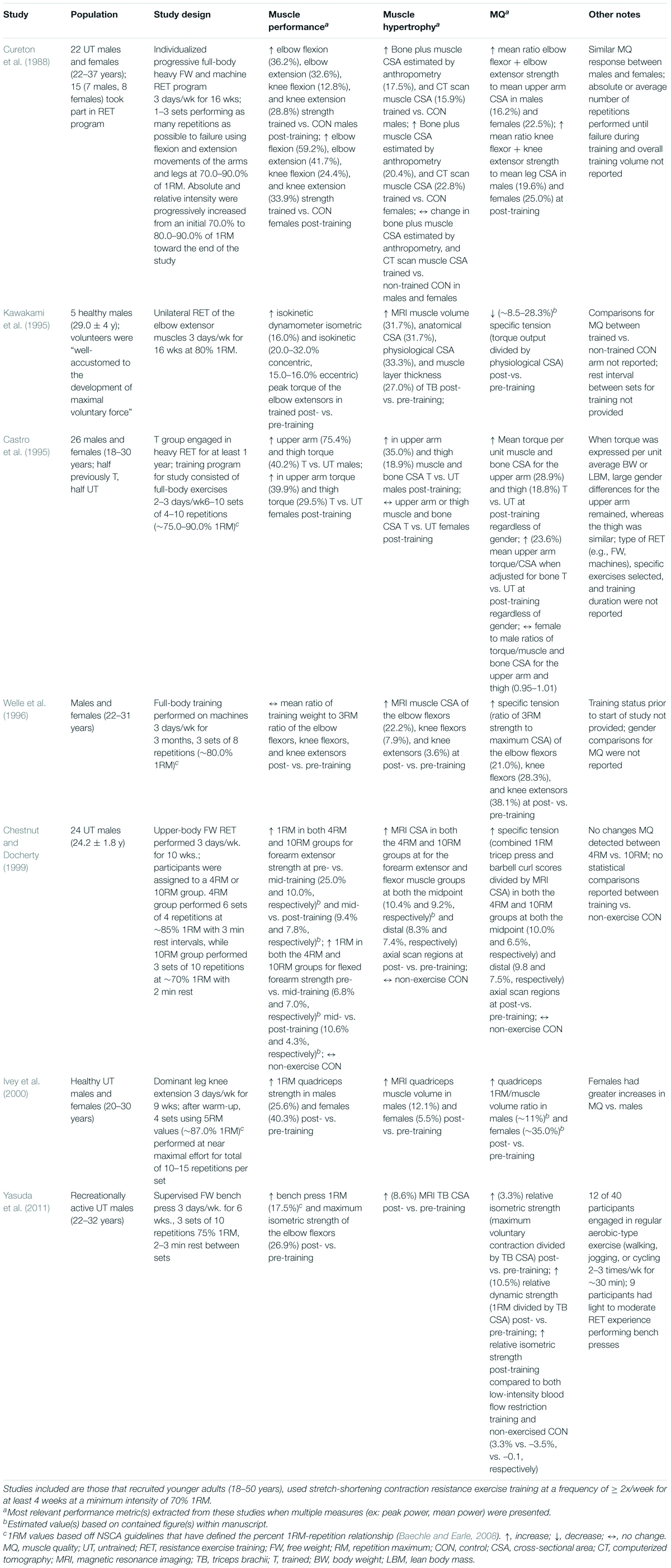
Table 5. Effects of high-intensity RET on MQ, as measured by relative strength, in young (18–50 years), healthy humans.
Given that published research provides support that high-intensity RET improves muscle strength, mass, and composition in young individuals, proxy measures of MQ assessed with imaging techniques such as EI measured via US would also be expected to demonstrate this MQ improvement. However, a majority of the research demonstrating that exercise interventions increase MQ as reflected by a decrease in muscle EI have been conducted in older adults or diseased populations (Radaelli et al., 2013, 2014; Cadore et al., 2014; Wilhelm et al., 2014; Yoshiko et al., 2017, 2018; Yamada et al., 2019). Few investigations have examined the impact of RET on EI in young individuals, making it difficult to make definitive conclusions. Nevertheless, studies examining the effects of high-intensity RET on EI in younger populations listed in Table 6 demonstrate comparable training-induced improvements in muscle performance and/or mass to those in Table 5. Furthermore, the majority of these studies showed enhanced MQ (i.e., decreased EI of ∼5–16%) following high-intensity RET. However, two of the aforementioned studies, despite showing increased muscle performance (∼4–22%) and size (∼3–7%), did not report changes in MQ (Wells et al., 2014; Jenkins et al., 2016). A number of factors related to US measurement could have affected the results, such as probe placement, muscle depth, subcutaneous adipose tissue thickness, and patient positioning (Young et al., 2015; Dankel et al., 2018; Varanoske et al., 2019). Of these studies, three performed direct comparisons between high and low intensity training and showed that high-intensity training resulted in greater post-training relative increases in muscle performance and hypertrophy, with two of the three also reporting greater decreases in EI (higher improvements in MQ) in the high-intensity group (Jenkins et al., 2017; Ikezoe et al., 2020). Taken together, these findings demonstrate that RET in young individuals may improve MQ as assessed via ultrasonography.
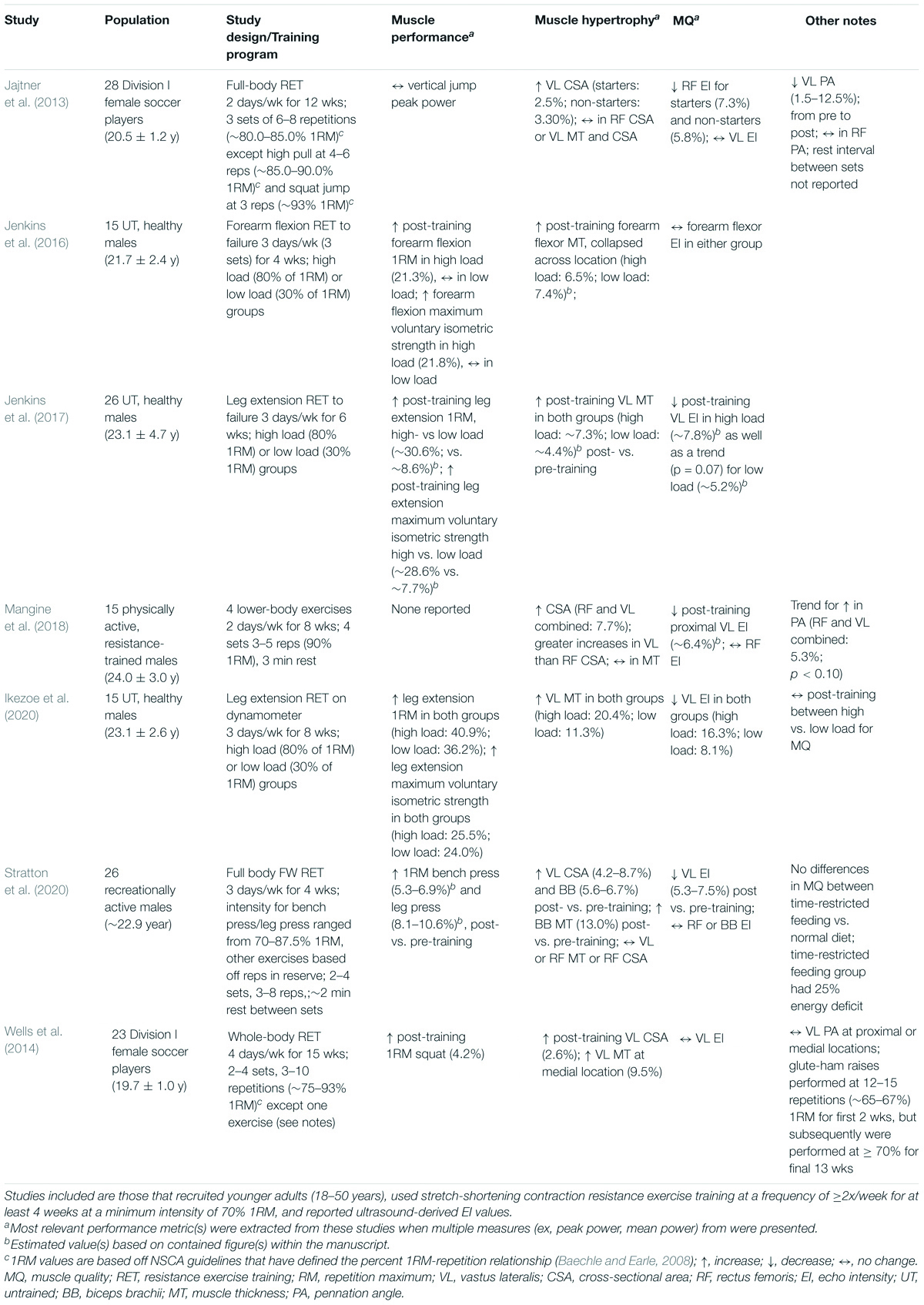
Table 6. Effects of high-intensity RET on MQ measured via ultrasound-derived EI in young (18–50 years) humans.
Additional evidence demonstrates that the components of muscle adaptation that MQ is derived from (i.e., force to size ratio) is noticeably improved by high-intensity RET. In a recent review by Abe et al. (2020), the authors found from RET studies using heavy exercise loads (>70% 1RM) increased vastus lateralis muscle CSA (∼11%), pennation angle (∼6%), and fascicle length (5%). Aube et al. (2020) used young well-trained male participants to investigate the effects of three different 8-week high-intensity lower-body progressive RET interventions (loads equivalent to ∼80–85% 1RM) differing in the number of total sets performed. All three groups showed significant improvements in back squat 1RM muscle strength ranging from ∼5.4 to 16.2%; additionally, all three groups demonstrated a significant increase in thigh muscle thickness (∼6.1–7.7%). In another study by Lasevicius and colleagues (Lasevicius et al., 2018), the authors found following 12 weeks RET that the highest intensity group (80% 1RM) demonstrated superior increases in muscle strength and CSA in comparison to low intensities. Mangine et al. (2015b) found in response to their 8 week moderate or high-intensity RET program, both groups responded with significant increases in bench press and back squat 1RM strength; however, the high-intensity group that trained at 90% of 1RM also had significantly higher bench press 1RM compared to the moderate group.
However, not all studies have shown positive effects of high-intensity training on MQ. For example, a previous investigation used data questionnaires in college-aged males and females, and based on answers about previous training history, assigned individuals to either a low- or high-intensity resistance training group and subsequently performed a battery of tests looking at muscle function (Shoepe et al., 2020). The high-intensity group had significantly greater handgrip strength but both groups had lower MQ compared to individuals with no history of RET. However, there were several limitations; the study design was retrospective in nature as the authors relied on participant questionnaire responses for group assignments, so an inherent self-bias could have led to inaccurate answers and group placement. Additionally, due to the retrospective design, the exercise intervention conducted was not under the supervision of the investigators; previous research has shown larger effects in strength when RET is supervised (Mazzetti et al., 2000; Lacroix et al., 2017). Finally, the authors chose handgrip strength as a surrogate measure of total body strength. Previous research has shown that handgrip strength is not the best indicator of physical performance (Abe et al., 2016) and that applying 1RM would have been a more representative outcome metric in calculating MQ. In another study by Nóbrega and colleagues (Nobrega et al., 2018), young men (average age: 23.0 years old) participated in two days per week of unilateral leg extension RET for 12 weeks at 30% and 80% 1RM. Following both 6 (midpoint) and 12 weeks (post-training) of exercise, similar improvements in 1RM muscle strength, muscle CSA, and pennation angle were observed. However, training was done using a unilateral leg extension machine (3 sets total per training session), so perhaps the magnitude of the results in the high-intensity groups were hampered by the fact that participants were not performing full body RET with free weights and with overall low session training volume. As noted earlier, a benefit of high-intensity RET is the ability to maximally recruit the majority or all the fibers of a targeted muscle group (Rader et al., 2017b), which is somewhat offset using machines. Although some previous research has demonstrated that short-term (8-week) RET training with free weights or machines induces similar increases in muscle mass and strength (Schwanbeck et al., 2020), free weight usage has resulted in greater electromyography muscle activation (McCaw and Friday, 1994; Schwanbeck et al., 2009), and greater increases in circulating testosterone concentrations (Schwanbeck et al., 2020) than RET using machines. As most improvements in muscle strength during the first phases of training are due to neural adaptations rather than muscle hypertrophy (Moritani and deVries, 1979; Sale, 1988), it is not surprising that increases in muscle mass during short-term RET are similar with free weights and machines (Schwanbeck et al., 2020). However, the greater anabolic response and neural activation observed with free weight RET provide promising evidence that increases in strength and/or hypertrophy may be greater when using free weight RET than machines during longer-term training regimens. Collectively, these studies provide evidence strongly supporting the notion that high-intensity RET, in comparison to other exercise-based interventions, is the most effective exercise strategy for the augmentation of force production, relevant muscle mass, power development, and, ultimately, MQ.
Conclusion
While most practical measurement techniques will not capture all of the complex adaptations of muscle to an exercise training stimulus, measurements that account for MQ ultimately reflect the functional summation of complex physiologic changes in response to adaptations to training. MQ has historically been evaluated in older adult and/or clinical populations. However, the paradigm shift observed in the field of aging, to include a focus on MQ rather than a mere loss of muscle mass, has not been replicated to the same degree in young populations. Thus, we reviewed the importance of this measurement in young, healthy adults and highlighted studies that have emphasized the importance of MQ in this cohort.
The collective results from the literature in animals and humans that have utilized physiologically relevant exercise models indicate that high-intensity RET, compared to other exercise training regimens, has the most robust positive effects on augmenting MQ. Younger individuals engaged in intense physical tasks do not simply need to acquire large amounts of muscle mass, but would benefit from increased functional capacity in order to successfully complete their duties. Hence, we put forth the assertion that MQ should be recognized as one of the most important biomarkers for performance enhancement in young individuals. Accordingly, focusing on evidence-based exercise interventions that serve to further enhance MQ in various young populations, such as high-intensity RET, will ultimately improve the capabilities of these individuals to optimally perform the critical responsibilities related to their physically demanding occupational tasks.
Author Contributions
All authors listed have made a substantial, direct and intellectual contribution to the work, and approved it for publication.
Funding
This research was funded by the Military Operational Medicine Research Program, U.S. Army Medical Research and Development Command. This work was supported in part by an appointment to the Postgraduate Research Participation Program (ANV) funded by the U.S. Army Research Institute of Environmental Medicine and administered by the Oak Ridge Institute for Science and Engineering through an interagency agreement between the U.S. Department of Energy and the United States Army Medical Research and Development Command.
Author Disclaimer
The opinions or assertions contained herein are the private views of the authors and are not to be construed as official or as reflecting the views of the Army or the Department of Defense.
Conflict of Interest
The authors declare that the research was conducted in the absence of any commercial or financial relationships that could be construed as a potential conflict of interest.
Publisher’s Note
All claims expressed in this article are solely those of the authors and do not necessarily represent those of their affiliated organizations, or those of the publisher, the editors and the reviewers. Any product that may be evaluated in this article, or claim that may be made by its manufacturer, is not guaranteed or endorsed by the publisher.
Acknowledgments
We gratefully acknowledge the efforts of Susan P. Proctor, DSc, and CPT Brandon M. Roberts, Ph.D., for their careful review of the manuscript.
References
Aagaard, P., and Andersen, J. L. (1998). Correlation between contractile strength and myosin heavy chain isoform composition in human skeletal muscle. Med. Sci. Sports Exerc. 30, 1217–1222. doi: 10.1097/00005768-199808000-00006
Aagaard, P., Andersen, J. L., Dyhre-Poulsen, P., Leffers, A. M., Wagner, A., Magnusson, S. P., et al. (2001). A mechanism for increased contractile strength of human pennate muscle in response to strength training: changes in muscle architecture. J. Physiol. 534(Pt. 2), 613–623. doi: 10.1111/j.1469-7793.2001.t01-1-00613.x
Abe, T., Dankel, S., Spitz, R. W., Buckner, S. L., Wong, V., Viana, R. B., et al. (2020). Does resistance training increase aponeurosis width? The current results and future tasks. Eur. J. Appl. Physiol. 120, 1489–1494. doi: 10.1007/s00421-020-04400-x
Abe, T., Thiebaud, R. S., and Loenneke, J. P. (2016). Forearm muscle quality as a better indicator of physical performance than handgrip strength in older male ground golf players aged 70 to 89. J. Musculoskelet Neuronal. Interact. 16, 296–301.
Aguiar, A. F., Vechetti-Junior, I. J., Alves, de Souza, R. W., Castan, E. P., Milanezi-Aguiar, R. C., et al. (2013). Myogenin, MyoD and IGF-I regulate muscle mass but not fiber-type conversion during resistance training in rats. Int. J. Sports Med. 34, 293–301. doi: 10.1055/s-0032-1321895
Ahtiainen, J. P., Hoffren, M., Hulmi, J. J., Pietikainen, M., Mero, A. A., Avela, J., et al. (2010). Panoramic ultrasonography is a valid method to measure changes in skeletal muscle cross-sectional area. Eur. J. Appl. Physiol. 108, 273–279. doi: 10.1007/s00421-009-1211-6
Ahtiainen, J. P., Walker, S., Peltonen, H., Holviala, J., Sillanpaa, E., Karavirta, L., et al. (2016). Heterogeneity in resistance training-induced muscle strength and mass responses in men and women of different ages. Age 38:10. doi: 10.1007/s11357-015-9870-1
American College of Sports Medicine (2009). American College of Sports Medicine position stand. Progression models in resistance training for healthy adults. Med. Sci. Sports Exerc. 41, 687–708. doi: 10.1249/MSS.0b013e3181915670
Arroyo, E., Stout, J. R., Beyer, K. S., Church, D. D., Varanoske, A. N., Fukuda, D. H., et al. (2018). Effects of supine rest duration on ultrasound measures of the vastus lateralis. Clin. Physiol. Funct. Imaging 38, 155–157. doi: 10.1111/cpf.12403
Aube, D., Wadhi, T., Rauch, J., Anand, A., Barakat, C., Pearson, J., et al. (2020). Progressive resistance training volume: Effects on muscle thickness, mass, and strength adaptations in resistance-trained individuals. J. Strength Cond. Res. 2020:3524. doi: 10.1519/JSC.0000000000003524
Azizi, E., and Brainerd, E. L. (2007). Architectural gear ratio and muscle fiber strain homogeneity in segmented musculature. J. Exp. Zool. A Ecol. Genet. Physiol. 307, 145–155. doi: 10.1002/jez.a.358
Baechle, T. R., and Earle, R. W. (2008). Essentials of Strength Training and Conditioning. Champaign, IL: Human Kinetics.
Baker, B. A., Hollander, M. S., Kashon, M. L., and Cutlip, R. G. (2010). Effects of glutathione depletion and age on skeletal muscle performance and morphology following chronic stretch-shortening contraction exposure. Eur. J. Appl. Physiol. 108, 619–630. doi: 10.1007/s00421-009-1258-4
Bamman, M. M., Cooper, D. M., Booth, F. W., Chin, E. R., Neufer, P. D., Trappe, S., et al. (2014). Exercise biology and medicine: innovative research to improve global health. Mayo Clin. Proc. 89, 148–153. doi: 10.1016/j.mayocp.2013.11.013
Bamman, M. M., Newcomer, B. R., Larson-Meyer, D. E., Weinsier, R. L., and Hunter, G. R. (2000). Evaluation of the strength-size relationship in vivo using various muscle size indices. Med. Sci. Sports Exerc. 32, 1307–1313. doi: 10.1097/00005768-200007000-00019
Barbat-Artigas, S., Rolland, Y., Zamboni, M., and Aubertin-Leheudre, M. (2012). How to assess functional status: a new muscle quality index. J. Nutr. Health Aging 16, 67–77. doi: 10.1007/s12603-012-0004-5
Baroni, B. M., Geremia, J. M., Rodrigues, R., De Azevedo Franke, R., Karamanidis, K., and Vaz, M. A. (2013). Muscle architecture adaptations to knee extensor eccentric training: rectus femoris vs. vastus lateralis. Muscle Nerve 48, 498–506. doi: 10.1002/mus.23785
Benito, P. J., Cupeiro, R., Ramos-Campo, D. J., Alcaraz, P. E., and Rubio-Arias, J. A. (2020). A Systematic Review with Meta-Analysis of the Effect of Resistance Training on Whole-Body Muscle Growth in Healthy Adult Males. Int. J. Environ. Res. Public Health 17:ijerh17041285. doi: 10.3390/ijerph17041285
Bergstrom, J. (1975). Percutaneous needle biopsy of skeletal muscle in physiological and clinical research. Scand. J. Clin. Lab. Invest. 35, 609–616. doi: 10.3109/00365517509095787
Blazevich, A. J., Cannavan, D., Coleman, D. R., and Horne, S. (2007). Influence of concentric and eccentric resistance training on architectural adaptation in human quadriceps muscles. J. Appl. Physiol. 103, 1565–1575. doi: 10.1152/japplphysiol.00578.2007
Brooks, N., Layne, J. E., Gordon, P. L., Roubenoff, R., Nelson, M. E., and Castaneda-Sceppa, C. (2006). Strength training improves muscle quality and insulin sensitivity in Hispanic older adults with type 2 diabetes. Int. J. Med. Sci. 4, 19–27. doi: 10.7150/ijms.4.19
Brown, J. C., Harhay, M. O., and Harhay, M. N. (2016). The muscle quality index and mortality among males and females. Ann. Epidemiol. 26, 648–653. doi: 10.1016/j.annepidem.2016.07.006
Cadore, E. L., Gonzalez-Izal, M., Pallares, J. G., Rodriguez-Falces, J., Hakkinen, K., Kraemer, W. J., et al. (2014). Muscle conduction velocity, strength, neural activity, and morphological changes after eccentric and concentric training. Scand. J. Med. Sci. Sports 24, e343–e352. doi: 10.1111/sms.12186
Cadore, E. L., Izquierdo, M., Conceicao, M., Radaelli, R., Pinto, R. S., Baroni, B. M., et al. (2012). Echo intensity is associated with skeletal muscle power and cardiovascular performance in elderly men. Exp. Gerontol. 47, 473–478. doi: 10.1016/j.exger.2012.04.002
Caldow, M. K., Thomas, E. E., Dale, M. J., Tomkinson, G. R., Buckley, J. D., and Cameron-Smith, D. (2015). Early myogenic responses to acute exercise before and after resistance training in young men. Physiol. Rep. 3:12511. doi: 10.14814/phy2.12511
Camera, D. M., Smiles, W. J., and Hawley, J. A. (2016). Exercise-induced skeletal muscle signaling pathways and human athletic performance. Free Radic. Biol. Med. 98, 131–143. doi: 10.1016/j.freeradbiomed.2016.02.007
Castro, M. J., McCann, D. J., Shaffrath, J. D., and Adams, W. C. (1995). Peak torque per unit cross-sectional area differs between strength-trained and untrained young adults. Med. Sci. Sports Exerc. 27, 397–403.
Chestnut, J. L., and Docherty, D. (1999). The Effects of 4 and 10 Repetition Maximum Weight-Training Protocols on Neuromuscular Adaptations in Untrained Men. J. Strength Condition. Res. 13, 353–359. doi: 10.1519/00124278-199911000-00009
Cooper, R., Bann, D., Wloch, E. G., Adams, J. E., and Kuh, D. (2015). “Skeletal muscle function deficit” in a nationally representative British birth cohort in early old age. J. Gerontol. A Biol. Sci. Med. Sci. 70, 604–607. doi: 10.1093/gerona/glu214
Correa-de-Araujo, R., Addison, O., Miljkovic, I., Goodpaster, B. H., Bergman, B. C., Clark, R. V., et al. (2020). Myosteatosis in the Context of Skeletal Muscle Function Deficit: An Interdisciplinary Workshop at the National Institute on Aging. Front. Physiol. 11:963. doi: 10.3389/fphys.2020.00963
Correa-de-Araujo, R., Harris-Love, M. O., Miljkovic, I., Fragala, M. S., Anthony, B. W., and Manini, T. M. (2017). The Need for Standardized Assessment of Muscle Quality in Skeletal Muscle Function Deficit and Other Aging-Related Muscle Dysfunctions: A Symposium Report. Front. Physiol. 8:87. doi: 10.3389/fphys.2017.00087
Cruz-Jentoft, A. J., Bahat, G., Bauer, J., Boirie, Y., Bruyere, O., Cederholm, T., et al. (2019). Sarcopenia: revised European consensus on definition and diagnosis. Age Ageing 48:601. doi: 10.1093/ageing/afz046
Cui, D., Drake, J. C., Wilson, R. J., Shute, R. J., Lewellen, B., Zhang, M., et al. (2020). A novel voluntary weightlifting model in mice promotes muscle adaptation and insulin sensitivity with simultaneous enhancement of autophagy and mTOR pathway. FASEB J. 34, 7330–7344. doi: 10.1096/fj.201903055R
Cureton, K. J., Collins, M. A., Hill, D. W., and McElhannon, F. M. Jr. (1988). Muscle hypertrophy in men and women. Med. Sci. Sports Exerc. 20, 338–344. doi: 10.1249/00005768-198808000-00003
Cutlip, R. G., Baker, B. A., Geronilla, K. B., Mercer, R. R., Kashon, M. L., Miller, G. R., et al. (2006). Chronic exposure to stretch-shortening contractions results in skeletal muscle adaptation in young rats and maladaptation in old rats. Appl. Physiol. Nutr. Metab. 31, 573–587. doi: 10.1139/h06-033
Dankel, S. J., Abe, T., Bell, Z. W., Jessee, M. B., Buckner, S. L., Mattocks, K. T., et al. (2018). The Impact of Ultrasound Probe Tilt on Muscle Thickness and Echo-Intensity: A Cross-Sectional Study. J. Clin. Densitom. 2018:003. doi: 10.1016/j.jocd.2018.10.003
Dankel, S. J., Counts, B. R., Barnett, B. E., Buckner, S. L., Abe, T., and Loenneke, J. P. (2017). Muscle adaptations following 21 consecutive days of strength test familiarization compared with traditional training. Muscle Nerve 56, 307–314. doi: 10.1002/mus.25488
Delp, M. D., and Pette, D. (1994). Morphological changes during fiber type transitions in low-frequency-stimulated rat fast-twitch muscle. Cell Tissue Res. 277, 363–371. doi: 10.1007/BF00327784
Dons, B., Bollerup, K., Bonde-Petersen, F., and Hancke, S. (1979). The effect of weight-lifting exercise related to muscle fiber composition and muscle cross-sectional area in humans. Eur. J. Appl. Physiol. Occup. Physiol. 40, 95–106. doi: 10.1007/BF00421155
Drakonaki, E. E., Allen, G. M., and Wilson, D. J. (2012). Ultrasound elastography for musculoskeletal applications. Br. J. Radiol. 85, 1435–1445. doi: 10.1259/bjr/93042867
Duchateau, J., Stragier, S., Baudry, S., and Carpentier, A. (2021). Strength training: In search of optimal strategies to maximize neuromuscular performance. Exerc. Sport Sci. Rev. 49, 2–14. doi: 10.1249/JES.0000000000000234
Ema, R., Wakahara, T., Miyamoto, N., Kanehisa, H., and Kawakami, Y. (2013). Inhomogeneous architectural changes of the quadriceps femoris induced by resistance training. Eur. J. Appl. Physiol. 113, 2691–2703. doi: 10.1007/s00421-013-2700-1
Erlandson, M. C., Lorbergs, A. L., Mathur, S., and Cheung, A. M. (2016). Muscle analysis using pQCT, DXA and MRI. Eur. J. Radiol. 85, 1505–1511. doi: 10.1016/j.ejrad.2016.03.001
Erlandson, M. C., Wong, A. K. O., Szabo, E., Vilayphiou, N., Zulliger, M. A., Adachi, J. D., et al. (2017). Muscle and Myotendinous Tissue Properties at the Distal Tibia as Assessed by High-Resolution Peripheral Quantitative Computed Tomography. J. Clin. Densitom. 20, 226–232. doi: 10.1016/j.jocd.2016.10.005
Fisher, J., Steele, J., and Smith, D. (2017). High- and Low-Load Resistance Training: Interpretation and Practical Application of Current Research Findings. Sports Med. 47, 393–400. doi: 10.1007/s40279-016-0602-1
Foulis, S. A., Hughes, J. M., and Friedl, K. E. (2020). New concerns about military recruits with metabolic obesity but normal weight (”skinny fat”). Obesity 28:223. doi: 10.1002/oby.22724
Fragala, M. S., Fukuda, D. H., Stout, J. R., Townsend, J. R., Emerson, N. S., Boone, C. H., et al. (2014a). Muscle quality index improves with resistance exercise training in older adults. Exp. Gerontol. 53, 1–6. doi: 10.1016/j.exger.2014.01.027
Fragala, M. S., Jajtner, A. R., Beyer, K. S., Townsend, J. R., Emerson, N. S., Scanlon, T. C., et al. (2014b). Biomarkers of muscle quality: N-terminal propeptide of type III procollagen and C-terminal agrin fragment responses to resistance exercise training in older adults. J. Cachexia Sarcopenia Muscle 5, 139–148. doi: 10.1007/s13539-013-0120-z
Fragala, M. S., Kenny, A. M., and Kuchel, G. A. (2015). Muscle quality in aging: a multi-dimensional approach to muscle functioning with applications for treatment. Sports Med. 45, 641–658. doi: 10.1007/s40279-015-0305-z
Franchi, M. V., Atherton, P. J., Maganaris, C. N., and Narici, M. V. (2016). Fascicle length does increase in response to longitudinal resistance training and in a contraction-mode specific manner. Springerplus 5:94. doi: 10.1186/s40064-015-1548-8
Franchi, M. V., Atherton, P. J., Reeves, N. D., Fluck, M., Williams, J., Mitchell, W. K., et al. (2014). Architectural, functional and molecular responses to concentric and eccentric loading in human skeletal muscle. Acta Physiol. 210, 642–654. doi: 10.1111/apha.12225
Frontera, W. R., Meredith, C. N., O’Reilly, K. P., Knuttgen, H. G., and Evans, W. J. (1988). Strength conditioning in older men: skeletal muscle hypertrophy and improved function. J. Appl. Physiol. 64, 1038–1044. doi: 10.1152/jappl.1988.64.3.1038
Fry, A. C. (2004). The role of resistance exercise intensity on muscle fibre adaptations. Sports Med. 34, 663–679. doi: 10.2165/00007256-200434100-00004
Fukumoto, Y., Ikezoe, T., Yamada, Y., Tsukagoshi, R., Nakamura, M., Mori, N., et al. (2012). Skeletal muscle quality assessed from echo intensity is associated with muscle strength of middle-aged and elderly persons. Eur. J. Appl. Physiol. 112, 1519–1525. doi: 10.1007/s00421-011-2099-5
Fukutani, A., and Kurihara, T. (2015). Tendon Cross-Sectional Area is Not Associated With Muscle Volume. J. Appl. Biomech. 31, 176–180. doi: 10.1123/jab.2014-0183
Garber, C. E., Blissmer, B., Deschenes, M. R., Franklin, B. A., Lamonte, M. J., Lee, I. M., et al. (2011). American College of Sports Medicine position stand. Quantity and quality of exercise for developing and maintaining cardiorespiratory, musculoskeletal, and neuromotor fitness in apparently healthy adults: guidance for prescribing exercise. Med. Sci. Sports Exerc. 43, 1334–1359. doi: 10.1249/MSS.0b013e318213fefb
Gloor, M., Fasler, S., Fischmann, A., Haas, T., Bieri, O., Heinimann, K., et al. (2011). Quantification of fat infiltration in oculopharyngeal muscular dystrophy: comparison of three MR imaging methods. J. Magn. Reson. Imaging 33, 203–210. doi: 10.1002/jmri.22431
Goodpaster, B. H. (2002). Measuring body fat distribution and content in humans. Curr. Opin. Clin. Nutr. Metab. Care 5, 481–487. doi: 10.1097/00075197-200209000-00005
Goodpaster, B. H., Park, S. W., Harris, T. B., Kritchevsky, S. B., Nevitt, M., Schwartz, A. V., et al. (2006). The loss of skeletal muscle strength, mass, and quality in older adults: the health, aging and body composition study. J. Gerontol. A Biol. Sci. Med. Sci. 61, 1059–1064. doi: 10.1093/gerona/61.10.1059
Hacker, E. D., Peters, T., and Garkova, M. (2016). Ultrasound Assessment of the Rectus Femoris Cross-Sectional Area: Subject Position Implications. West J. Nurs. Res. 38, 1221–1230. doi: 10.1177/0193945916644751
Hagerman, F. C., Walsh, S. J., Staron, R. S., Hikida, R. S., Gilders, R. M., Murray, T. F., et al. (2000). Effects of high-intensity resistance training on untrained older men. I. Strength, cardiovascular, and metabolic responses. J. Gerontol. A Biol. Sci. Med. Sci. 55, B336–B346.
Hellsten, Y., and Nyberg, M. (2015). Cardiovascular Adaptations to Exercise Training. Compr. Physiol. 6, 1–32. doi: 10.1002/cphy.c140080
Hepple, R. T., Mackinnon, S. L., Thomas, S. G., Goodman, J. M., and Plyley, M. J. (1997). Quantitating the capillary supply and the response to resistance training in older men. Pflügers Archiv. Eur. J. Physiol. 433, 238–244. doi: 10.1007/s004240050273
Heymsfield, S. B., Gonzalez, M. C., Lu, J., Jia, G., and Zheng, J. (2015). Skeletal muscle mass and quality: evolution of modern measurement concepts in the context of sarcopenia. Proc. Nutr. Soc. 74, 355–366. doi: 10.1017/S0029665115000129
Hughes, D. C., Ellefsen, S., and Baar, K. (2018). Adaptations to Endurance and Strength Training. Cold Spring Harb. Perspect. Med. 8:a029769. doi: 10.1101/cshperspect.a029769
Ihnatsenka, B., and Boezaart, A. P. (2010). Ultrasound: Basic understanding and learning the language. Int. J. Shoulder Surg. 4, 55–62. doi: 10.4103/0973-6042.76960
Ikezoe, T., Kobayashi, T., Nakamura, M., and Ichihashi, N. (2020). Effects of Low-Load, Higher-Repetition vs. High-Load, Lower-Repetition Resistance Training Not Performed to Failure on Muscle Strength, Mass, and Echo Intensity in Healthy Young Men: A Time-Course Study. J. Strength Cond. Res. 34, 3439–3445. doi: 10.1519/JSC.0000000000002278
Ivey, F. M., Tracy, B. L., Lemmer, J. T., NessAiver, M., Metter, E. J., Fozard, J. L., et al. (2000). Effects of strength training and detraining on muscle quality: age and gender comparisons. J. Gerontol. A. Biol. Sci. Med. Sci. 55, B152–B157. doi: 10.1093/gerona/55.3.b152
Jajtner, A. R., Hoffman, J. R., Gonzalez, A. M., Worts, P. R., Fragala, M. S., and Stout, J. R. (2015). Comparison of the effects of electrical stimulation and cold-water immersion on muscle soreness after resistance exercise. J. Sport Rehabil. 24, 99–108. doi: 10.1123/jsr.2013-0113
Jajtner, A. R., Hoffman, J. R., Scanlon, T. C., Wells, A. J., Townsend, J. R., Beyer, K. S., et al. (2013). Performance and muscle architecture comparisons between starters and nonstarters in National Collegiate Athletic Association Division I women’s soccer. J. Strength Cond. Res. 27, 2355–2365. doi: 10.1519/JSC.0b013e31829bd7c5
Jakobsgaard, J. E., Christiansen, M., Sieljacks, P., Wang, J., Groennebaek, T., de Paoli, F., et al. (2018). Impact of blood flow-restricted bodyweight exercise on skeletal muscle adaptations. Clin. Physiol. Funct. Imaging 2018:12509. doi: 10.1111/cpf.12509
Jenkins, N. D. M., Miramonti, A. A., Hill, E. C., Smith, C. M., Cochrane-Snyman, K. C., Housh, T. J., et al. (2017). Greater Neural Adaptations following High- vs. Low-Load Resistance Training. Front. Physiol. 8:331. doi: 10.3389/fphys.2017.00331
Jenkins, N. D., Housh, T. J., Buckner, S. L., Bergstrom, H. C., Cochrane, K. C., Hill, E. C., et al. (2016). Neuromuscular adaptations after 2 and 4 weeks of 80% versus 30% 1 repetition maximum resistance training to failure. J. Strength Cond. Res. 30, 2174–2185. doi: 10.1519/JSC.0000000000001308
Kawakami, Y., Abe, T., Kuno, S. Y., and Fukunaga, T. (1995). Training-induced changes in muscle architecture and specific tension. Eur. J. Appl. Physiol. Occup. Physiol. 72, 37–43. doi: 10.1007/bf00964112
Kristensen, J., and Franklyn-Miller, A. (2012). Resistance training in musculoskeletal rehabilitation: a systematic review. Br. J. Sports Med. 46, 719–726. doi: 10.1136/bjsm.2010.079376
Krzysztofik, M., Wilk, M., Wojdala, G., and Golas, A. (2019). Maximizing Muscle Hypertrophy: A Systematic Review of Advanced Resistance Training Techniques and Methods. Int. J. Environ. Res. Public Health 16:ijerh16244897. doi: 10.3390/ijerph16244897
Lacroix, A., Hortobagyi, T., Beurskens, R., and Granacher, U. (2017). Effects of supervised vs. unsupervised training programs on balance and muscle strength in older adults: A systematic review and meta-analysis. Sports Med. 47, 2341–2361. doi: 10.1007/s40279-017-0747-6
Larson-Meyer, D. E., Smith, S. R., Heilbronn, L. K., Kelley, D. E., Ravussin, E., Newcomer, B. R., et al. (2006). Muscle-associated triglyceride measured by computed tomography and magnetic resonance spectroscopy. Obesity 14, 73–87. doi: 10.1038/oby.2006.10
Lasevicius, T., Ugrinowitsch, C., Schoenfeld, B. J., Roschel, H., Tavares, L. D., De Souza, E. O., et al. (2018). Effects of different intensities of resistance training with equated volume load on muscle strength and hypertrophy. Eur. J. Sport Sci. 18, 772–780. doi: 10.1080/17461391.2018.1450898
Machann, J., Bachmann, O. P., Brechtel, K., Dahl, D. B., Wietek, B., Klumpp, B., et al. (2003). Lipid content in the musculature of the lower leg assessed by fat selective MRI: intra- and interindividual differences and correlation with anthropometric and metabolic data. J. Magn. Reson. Imaging 17, 350–357. doi: 10.1002/jmri.10255
MacInnis, M. J., and Gibala, M. J. (2017). Physiological adaptations to interval training and the role of exercise intensity. J. Physiol. 595, 2915–2930. doi: 10.1113/JP273196
Mangine, G. T., Fukuda, D. H., Townsend, J. R., Wells, A. J., Gonzalez, A. M., Jajtner, A. R., et al. (2015a). Sprinting performance on the Woodway Curve 3.0 is related to muscle architecture. Eur. J. Sport Sci. 15, 606–614. doi: 10.1080/17461391.2014.969322
Mangine, G. T., Hoffman, J. R., Gonzalez, A. M., Townsend, J. R., Wells, A. J., Jajtner, A. R., et al. (2015b). The effect of training volume and intensity on improvements in muscular strength and size in resistance-trained men. Physiol. Rep. 3:12472. doi: 10.14814/phy2.12472
Mangine, G. T., Redd, M. J., Gonzalez, A. M., Townsend, J. R., Wells, A. J., Jajtner, A. R., et al. (2018). Resistance training does not induce uniform adaptations to quadriceps. PLoS One 13:e0198304. doi: 10.1371/journal.pone.0198304
Mangine, G. T., Stratton, M. T., Almeda, C. G., Roberts, M. D., Esmat, T. A., VanDusseldorp, T. A., et al. (2020). Physiological differences between advanced CrossFit athletes, recreational CrossFit participants, and physically-active adults. PLoS One 15:e0223548. doi: 10.1371/journal.pone.0223548
Martland, R., Mondelli, V., Gaughran, F., and Stubbs, B. (2020). Can high-intensity interval training improve physical and mental health outcomes? A meta-review of 33 systematic reviews across the lifespan. J. Sports Sci. 38, 430–469. doi: 10.1080/02640414.2019.1706829
Mattocks, K. T., Buckner, S. L., Jessee, M. B., Dankel, S. J., Mouser, J. G., and Loenneke, J. P. (2017). Practicing the test produces strength equivalent to higher volume training. Med. Sci. Sports Exerc. 49, 1945–1954. doi: 10.1249/MSS.0000000000001300
Mazzetti, S. A., Kraemer, W. J., Volek, J. S., Duncan, N. D., Ratamess, N. A., Gomez, A. L., et al. (2000). The influence of direct supervision of resistance training on strength performance. Med. Sci. Sports Exerc. 32, 1175–1184. doi: 10.1097/00005768-200006000-00023
McCaw, S. T., and Friday, A. J. (1994). A comparison of muscle activity between a free weight and machine bench press. J. Strength Cond. Res. 8, 259–264. doi: 10.1519/1533-4287(1994)008<0259:acomab>2.3.co;2
McGregor, R. A., Cameron-Smith, D., and Poppitt, S. D. (2014). It is not just muscle mass: a review of muscle quality, composition and metabolism during ageing as determinants of muscle function and mobility in later life. Longev. Healthspan 3:9. doi: 10.1186/2046-2395-3-9
McMahon, G. E., Morse, C. I., Burden, A., Winwood, K., and Onambele, G. L. (2014). Impact of range of motion during ecologically valid resistance training protocols on muscle size, subcutaneous fat, and strength. J. Strength Cond. Res. 28, 245–255. doi: 10.1519/JSC.0b013e318297143a
Miljkovic, I., and Zmuda, J. M. (2010). Epidemiology of myosteatosis. Curr. Opin. Clin. Nutr. Metab. Care 13, 260–264. doi: 10.1097/MCO.0b013e328337d826
Moritani, T., and deVries, H. A. (1979). Neural factors versus hypertrophy in the time course of muscle strength gain. Am. J. Phys. Med. 58, 115–130.
Murlasits, Z., Cutlip, R. G., Geronilla, K. B., Rao, K. M., Wonderlin, W. F., and Alway, S. E. (2006). Resistance training increases heat shock protein levels in skeletal muscle of young and old rats. Exp. Gerontol. 41, 398–406. doi: 10.1016/j.exger.2006.01.005
Nobrega, S. R., Ugrinowitsch, C., Pintanel, L., Barcelos, C., and Libardi, C. A. (2018). Effect of resistance training to muscle failure vs. volitional interruption at high- and low-intensities on muscle mass and strength. J. Strength Cond. Res. 32, 162–169. doi: 10.1519/JSC.0000000000001787
Noorkoiv, M., Nosaka, K., and Blazevich, A. J. (2010). Assessment of quadriceps muscle cross-sectional area by ultrasound extended-field-of-view imaging. Eur. J. Appl. Physiol. 109, 631–639. doi: 10.1007/s00421-010-1402-1
Parente, V., D’Antona, G., Adami, R., Miotti, D., Capodaglio, P., De Vito, G., et al. (2008). Long-term resistance training improves force and unloaded shortening velocity of single muscle fibres of elderly women. Eur. J. Appl. Physiol. 104, 885–893. doi: 10.1007/s00421-008-0845-0
Petre, H., Hemmingsson, E., Rosdahl, H., and Psilander, N. (2021). Development of Maximal Dynamic Strength During Concurrent Resistance and Endurance Training in Untrained, Moderately Trained, and Trained Individuals: A Systematic Review and Meta-analysis. Sports Med. 51, 991–1010. doi: 10.1007/s40279-021-01426-9
Phillips, B. E., Williams, J. P., Gustafsson, T., Bouchard, C., Rankinen, T., Knudsen, S., et al. (2013). Molecular networks of human muscle adaptation to exercise and age. PLoS Genet. 9:e1003389. doi: 10.1371/journal.pgen.1003389
Phillips, S. M., Aragon, A. A., Arciero, P. J., Arent, S. M., Close, G. L., Hamilton, D. L., et al. (2017). Changes in body composition and performance with supplemental HMB-FA+ATP. J. Strength Cond. Res. 31, e71–e72. doi: 10.1519/JSC.0000000000001760
Pillen, S., and van Alfen, N. (2011). Skeletal muscle ultrasound. Neurol. Res. 33, 1016–1024. doi: 10.1179/1743132811Y.0000000010
Pillen, S., Tak, R. O., Zwarts, M. J., Lammens, M. M., Verrijp, K. N., Arts, I. M., et al. (2009). Skeletal muscle ultrasound: correlation between fibrous tissue and echo intensity. Ultrasound Med. Biol. 35, 443–446. doi: 10.1016/j.ultrasmedbio.2008.09.016
Putman, C. T., Xu, X., Gillies, E., MacLean, I. M., and Bell, G. J. (2004). Effects of strength, endurance and combined training on myosin heavy chain content and fibre-type distribution in humans. Eur. J. Appl. Physiol. 92, 376–384. doi: 10.1007/s00421-004-1104-7
Radaelli, R., Bottaro, M., Wilhelm, E. N., Wagner, D. R., and Pinto, R. S. (2012). Time course of strength and echo intensity recovery after resistance exercise in women. J. Strength Cond. Res. 26, 2577–2584. doi: 10.1519/JSC.0b013e31823dae96
Radaelli, R., Botton, C. E., Wilhelm, E. N., Bottaro, M., Brown, L. E., Lacerda, F., et al. (2014). Time course of low- and high-volume strength training on neuromuscular adaptations and muscle quality in older women. Age 36, 881–892. doi: 10.1007/s11357-013-9611-2
Radaelli, R., Botton, C. E., Wilhelm, E. N., Bottaro, M., Lacerda, F., Gaya, A., et al. (2013). Low- and high-volume strength training induces similar neuromuscular improvements in muscle quality in elderly women. Exp. Gerontol. 48, 710–716. doi: 10.1016/j.exger.2013.04.003
Rader, E. P., and Baker, B. A. (2017). Inflammaging and the age-specific responsiveness to stretch-shortening contractions. Exerc. Sport Sci. Rev. 45, 195–200. doi: 10.1249/JES.0000000000000123
Rader, E. P., and Baker, B. A. (2020). Age-dependent stress response DNA demethylation and gene upregulation accompany nuclear and skeletal muscle remodeling following acute resistance-type exercise in rats. Facets 5, 455–473. doi: 10.1139/facets-2019-0060
Rader, E. P., Layner, K., Triscuit, A. M., Chetlin, R. D., Ensey, J., and Baker, B. A. (2016). Age-dependent muscle adaptation after chronic stretch-shortening contractions in rats. Aging Dis. 7, 1–13. doi: 10.14336/AD.2015.0920
Rader, E. P., Naimo, M. A., Ensey, J., and Baker, B. A. (2017a). Agonist muscle adaptation accompanied by antagonist muscle atrophy in the hindlimb of mice following stretch-shortening contraction training. BMC Musculoskelet Disord. 18:60. doi: 10.1186/s12891-017-1397-4
Rader, E. P., Naimo, M. A., Ensey, J., and Baker, B. A. (2018a). High-intensity stretch-shortening contraction training modifies responsivity of skeletal muscle in old male rats. Exp. Gerontol. 104, 118–126. doi: 10.1016/j.exger.2018.02.009
Rader, E. P., Naimo, M. A., Ensey, J., and Baker, B. A. (2018b). VCAM-1 upregulation accompanies muscle remodeling following resistance-type exercise in Snell dwarf (Pit1(dw/dw)) mice. Aging Cell 17:e12816. doi: 10.1111/acel.12816
Rader, E. P., Naimo, M. A., Layner, K. N., Triscuit, A. M., Chetlin, R. D., Ensey, J., et al. (2017b). Enhancement of skeletal muscle in aged rats following high-intensity stretch-shortening contraction training. Rejuvenation Res. 20, 93–102. doi: 10.1089/rej.2016.1816
Reimers, K., Reimers, C. D., Wagner, S., Paetzke, I., and Pongratz, D. E. (1993). Skeletal muscle sonography: a correlative study of echogenicity and morphology. J. Ultrasound Med. 12, 73–77. doi: 10.7863/jum.1993.12.2.73
Sale, D. G. (1988). Neural adaptation to resistance training. Med. Sci. Sports Exerc. 20(Suppl.), S135–S145. doi: 10.1249/00005768-198810001-00009
Schoenfeld, B. J., Grgic, J., Van Every, D. W., and Plotkin, D. L. (2021). Loading Recommendations for Muscle Strength, Hypertrophy, and Local Endurance: A Re-Examination of the Repetition Continuum. Sports 9:sorts9020032. doi: 10.3390/sports9020032
Schoenfeld, B. J., Wilson, J. M., Lowery, R. P., and Krieger, J. W. (2016). Muscular adaptations in low- versus high-load resistance training: a meta-analysis. Eur. J. Sport Sci. 16, 1–10. doi: 10.1080/17461391.2014.989922
Schwanbeck, S. R., Cornish, S. M., Barss, T., and Chilibeck, P. D. (2020). Effects of training With free weights versus machines on muscle mass, strength, free testosterone, and free cortisol levels. J. Strength Cond. Res. 34, 1851–1859. doi: 10.1519/JSC.0000000000003349
Schwanbeck, S., Chilibeck, P. D., and Binsted, G. (2009). A comparison of free weight squat to Smith machine squat using electromyography. J. Strength Cond. Res. 23, 2588–2591. doi: 10.1519/JSC.0b013e3181b1b181
Scott, J. M., Martin, D. S., Ploutz-Snyder, R., Caine, T., Matz, T., Arzeno, N. M., et al. (2012). Reliability and Validity of Panoramic Ultrasound for Muscle Quantification. Ultrasound Med. Biol. 38, 1656–1661. doi: 10.1016/j.ultrasmedbio.2012.04.018
Seynnes, O. R., de Boer, M., and Narici, M. V. (2007). Early skeletal muscle hypertrophy and architectural changes in response to high-intensity resistance training. J. Appl. Physiol. 102, 368–373. doi: 10.1152/japplphysiol.00789.2006
Shoepe, T. C., LaBrie, J. W., Mello, G. T., Leggett, A. G., and Almstedt, H. C. (2020). Intensity of resistance training via self-reported history is critical in properly characterizing musculoskeletal health. BMC Musculoskelet. Disord. 21:729. doi: 10.1186/s12891-020-03753-w
Sikdar, S., Wei, Q., and Cortes, N. (2014). Dynamic ultrasound imaging applications to quantify musculoskeletal function. Exerc. Sport Sci. Rev. 42, 126–135. doi: 10.1249/JES.0000000000000015
Steele, J. (2014). Intensity; in-ten-si-ty; noun. 1. Often used ambiguously within resistance training. 2. Is it time to drop the term altogether? Br. J. Sports Med. 48, 1586–1588. doi: 10.1136/bjsports-2012-092127
Steele, J., Fisher, J., Giessing, J., and Gentil, P. (2017). Clarity in reporting terminology and definitions of set endpoints in resistance training. Muscle Nerve 56, 368–374. doi: 10.1002/mus.25557
Stock, M. S., and Thompson, B. J. (2020). Echo intensity as an indicator of skeletal muscle quality: applications, methodology, and future directions. Eur. J. Appl. Physiol. 2020, 4556–4556. doi: 10.1007/s00421-020-04556-6
Stratton, M. T., Tinsley, G. M., Alesi, M. G., Hester, G. M., Olmos, A. A., Serafini, P. R., et al. (2020). Four Weeks of Time-Restricted Feeding Combined with Resistance Training Does Not Differentially Influence Measures of Body Composition, Muscle Performance, Resting Energy Expenditure, and Blood Biomarkers. Nutrients 12:nu12041126. doi: 10.3390/nu12041126
Tanisho, K., and Hirakawa, K. (2009). Training effects on endurance capacity in maximal intermittent exercise: comparison between continuous and interval training. J. Strength Cond. Res. 23, 2405–2410. doi: 10.1519/JSC.0b013e3181bac790
Townsend, J. R., Hoffman, J. R., Fragala, M. S., Oliveira, L. P., Jajtner, A. R., Fukuda, D. H., et al. (2016). A Microbiopsy Method for Immunohistological and Morphological Analysis: A Pilot Study. Med. Sci. Sports Exerc. 48, 331–335. doi: 10.1249/MSS.0000000000000772
Travis, S. K., Ishida, A., Taber, C. B., Fry, A. C., and Stone, M. H. (2020). Emphasizing Task-Specific Hypertrophy to Enhance Sequential Strength and Power Performance. J. Funct. Morphol. Kinesiol. 5:jfmk5040076. doi: 10.3390/jfmk5040076
Tsitkanou, S., Spengos, K., Stasinaki, A. N., Zaras, N., Bogdanis, G., Papadimas, G., et al. (2017). Effects of high-intensity interval cycling performed after resistance training on muscle strength and hypertrophy. Scand. J. Med. Sci. Sports 27, 1317–1327. doi: 10.1111/sms.12751
Vaczi, M., Nagy, S. A., Koszegi, T., Ambrus, M., Bogner, P., Perlaki, G., et al. (2014). Mechanical, hormonal, and hypertrophic adaptations to 10 weeks of eccentric and stretch-shortening cycle exercise training in old males. Exp. Gerontol. 58, 69–77. doi: 10.1016/j.exger.2014.07.013
Vann, C. G., Osburn, S. C., Mumford, P. W., Roberson, P. A., Fox, C. D., Sexton, C. L., et al. (2020). Skeletal muscle protein composition adaptations to 10 weeks of high-load resistance training in previously-trained males. Front. Physiol. 11:259. doi: 10.3389/fphys.2020.00259
Varanoske, A. N., Coker, N. A., Johnson, B. D. I., Belity, T., and Wells, A. J. (2020). Influence of muscle depth and thickness on ultrasound echo intensity of the vastus lateralis. Acta Radiol. 2020:284185120958405. doi: 10.1177/0284185120958405
Varanoske, A. N., Coker, N. A., Johnson, B. D. I., Belity, T., Mangine, G. T., Stout, J. R., et al. (2019). Effects of rest position on morphology of the vastus lateralis and its relationship with lower-body strength and power. J. Funct. Morphol. Kinesiol. 4:64. doi: 10.3390/jfmk4030064
Varanoske, A. N., Fukuda, D. H., Boone, C. H., Beyer, K. S., Stout, J. R., and Hoffman, J. R. (2017). Scanning plane comparison of ultrasound-derived morphological characteristics of the vastus lateralis. Clin. Anat. 30, 533–542. doi: 10.1002/ca.22803
Vechin, F. C., Conceicao, M. S., Telles, G. D., Libardi, C. A., and Ugrinowitsch, C. (2021). Interference Phenomenon with Concurrent Strength and High-Intensity Interval Training-Based Aerobic Training: An Updated Model. Sports Med. 51, 599–605. doi: 10.1007/s40279-020-01421-6
Watanabe, Y., Ikenaga, M., Yoshimura, E., Yamada, Y., and Kimura, M. (2018). Association between echo intensity and attenuation of skeletal muscle in young and older adults: a comparison between ultrasonography and computed tomography. Clin. Interv. Aging 13, 1871–1878. doi: 10.2147/CIA.S173372
Watanabe, Y., Yamada, Y., Fukumoto, Y., Ishihara, T., Yokoyama, K., Yoshida, T., et al. (2013). Echo intensity obtained from ultrasonography images reflecting muscle strength in elderly men. Clin. Interv. Aging 8, 993–998. doi: 10.2147/Cia.S47263
Welle, S., Totterman, S., and Thornton, C. (1996). Effect of age on muscle hypertrophy induced by resistance training. J. Gerontol. A Biol. Sci. Med. Sci. 51, M270–M275. doi: 10.1093/gerona/51a.6.m270
Wells, A. J., Fukuda, D. H., Hoffman, J. R., Gonzalez, A. M., Jajtner, A. R., Townsend, J. R., et al. (2014). Vastus lateralis exhibits non-homogenous adaptation to resistance training. Muscle Nerve 50, 785–793. doi: 10.1002/mus.24222
Wheeler, M. W., Dunson, D. B., Pandalai, S. P., Baker, B. A., and Herring, A. H. (2014). Mechanistic hierarchical gaussian processes. J. Am. Stat. Assoc. 109, 894–904. doi: 10.1080/01621459.2014.899234
Wilhelm, E. N., Rech, A., Minozzo, F., Radaelli, R., Botton, C. E., and Pinto, R. S. (2014). Relationship between quadriceps femoris echo intensity, muscle power, and functional capacity of older men. Age 36, 1113–1122. doi: 10.1007/s11357-014-9625-4
Wilson, J. M., Marin, P. J., Rhea, M. R., Wilson, S. M., Loenneke, J. P., and Anderson, J. C. (2012). Concurrent training: a meta-analysis examining interference of aerobic and resistance exercises. J. Strength Cond. Res. 26, 2293–2307. doi: 10.1519/JSC.0b013e31823a3e2d
Wong, V., Spitz, R. W., Bell, Z. W., Viana, R. B., Chatakondi, R. N., Abe, T., et al. (2020). Exercise induced changes in echo intensity within the muscle: a brief review. J. Ultrasound 23, 457–472. doi: 10.1007/s40477-019-00424-y
Yamada, M., Kimura, Y., Ishiyama, D., Nishio, N., Otobe, Y., Tanaka, T., et al. (2019). Synergistic effect of bodyweight resistance exercise and protein supplementation on skeletal muscle in sarcopenic or dynapenic older adults. Geriatr. Gerontol. Int. 19, 429–437. doi: 10.1111/ggi.13643
Yasuda, T., Ogasawara, R., Sakamaki, M., Ozaki, H., Sato, Y., and Abe, T. (2011). Combined effects of low-intensity blood flow restriction training and high-intensity resistance training on muscle strength and size. Eur. J. Appl. Physiol. 111, 2525–2533. doi: 10.1007/s00421-011-1873-8
Yoshiko, A., Kaji, T., Sugiyama, H., Koike, T., Oshida, Y., and Akima, H. (2017). Effect of 12-month resistance and endurance training on quality, quantity, and function of skeletal muscle in older adults requiring long-term care. Exp. Gerontol. 98, 230–237. doi: 10.1016/j.exger.2017.08.036
Yoshiko, A., Tomita, A., Ando, R., Ogawa, M., Kondo, S., Saito, A., et al. (2018). Effects of 10-week walking and walking with home-based resistance training on muscle quality, muscle size, and physical functional tests in healthy older individuals. Eur. Rev. Aging Phys. Act 15:13. doi: 10.1186/s11556-018-0201-2
Young, H. J., Jenkins, N. T., Zhao, Q., and McCully, K. K. (2015). Measurement of intramuscular fat by muscle echo intensity. Muscle Nerve 52, 963–971. doi: 10.1002/mus.24656
Keywords: performance, high-intensity exercise, adaptation, muscle architecture, stretch-shortening contractions, cross-sectional area, ultrasound
Citation: Naimo MA, Varanoske AN, Hughes JM and Pasiakos SM (2021) Skeletal Muscle Quality: A Biomarker for Assessing Physical Performance Capabilities in Young Populations. Front. Physiol. 12:706699. doi: 10.3389/fphys.2021.706699
Received: 07 May 2021; Accepted: 09 July 2021;
Published: 05 August 2021.
Edited by:
John Joseph McCarthy, University of Kentucky, United StatesReviewed by:
C. Brooks Mobley, University of Kentucky, United StatesJoao Luiz Quaglioti Durigan, University of Brasilia, Brazil
Copyright © 2021 Naimo, Varanoske, Hughes and Pasiakos. This is an open-access article distributed under the terms of the Creative Commons Attribution License (CC BY). The use, distribution or reproduction in other forums is permitted, provided the original author(s) and the copyright owner(s) are credited and that the original publication in this journal is cited, in accordance with accepted academic practice. No use, distribution or reproduction is permitted which does not comply with these terms.
*Correspondence: Marshall A. Naimo, bWFyc2hhbGwuYS5uYWltbzIuY2l2QG1haWwubWls