- 1Institute for Human Movement and Medical Sciences, Niigata University of Health and Welfare, Niigata, Japan
- 2Department of Physical Therapy, Niigata University of Health and Welfare, Niigata, Japan
- 3Institute of Human Movement Science, Sport and Health, University of Graz, Graz, Austria
- 4Department of Sports Medicine and Exercise Physiology, Institute of Occupational, Social and Environmental Medicine, Goethe University Frankfurt, Frankfurt, Germany
In sports and clinical settings, roller massage (RM) interventions are used to acutely increase range of motion (ROM); however, the underlying mechanisms are unclear. Apart from changes in soft tissue properties (i.e., reduced passive stiffness), neurophysiological alterations such as decreased spinal excitability have been described. However, to date, no study has investigated both jointly. The purpose of this trial was to examine RM’s effects on neurophysiological markers and passive tissue properties of the plantar flexors in the treated (ROLL) and non-treated (NO-ROLL) leg. Fifteen healthy individuals (23 ± 3 years, eight females) performed three unilateral 60-s bouts of calf RM. This procedure was repeated four times on separate days to allow independent assessments of the following outcomes without reciprocal interactions: dorsiflexion ROM, passive torque during passive dorsiflexion, shear elastic modulus of the medial gastrocnemius muscle, and spinal excitability. Following RM, dorsiflexion ROM increased in both ROLL (+19.7%) and NO-ROLL (+13.9%). Similarly, also passive torque at dorsiflexion ROM increased in ROLL (+15.0%) and NO-ROLL (+15.2%). However, there were no significant changes in shear elastic modulus and spinal excitability (p > 0.05). Moreover, significant correlations were observed between the changes in DF ROM and passive torque at DF ROM in both ROLL and NO-ROLL. Changes in ROM after RM appear to be the result of sensory changes (e.g., passive torque at DF ROM), affecting both rolled and non-rolled body regions. Thus, therapists and exercise professionals may consider applying remote treatments if local loading is contraindicated.
Introduction
Improving flexibility is a frequent goal in sports. Besides representing a potential hallmark of athletic performance, it may also be related to injury risk. Previous studies reported an association of low flexibility (Witvrouw et al., 2001), high muscle stiffness (Pickering Rodriguez et al., 2017), and the occurrence of musculoskeletal complaints. Besides stretching and mobility exercise, rolling massage (RM) is another popular self-treatment to increase flexibility. In essence, it uses various tools such as polypropylene rollers (then called foam rolling), massagers, balls, and other similar devices to apply a compressive force to the soft tissue of the targeted body region.
Previous studies (Krause et al., 2019; Wilke et al., 2019, 2020; Behm et al., 2020) have not only shown an immediate increase in range of motion (ROM) following foam rolling but also revealed the absence of changes in athletic performance (Macdonald et al., 2013; Behara and Jacobson, 2017). This is relevant because static stretching for more than 60 s can likely decrease muscle strength and athletic performance if performed isometrically (Kay and Blazevich, 2012; Behm et al., 2016, 2021). Although foam rolling appears to be a useful technique for athletes, its mechanisms remain a matter of debate. Initially, foam rolling was assumed to modify local tissue characteristics and properties. Hotfiel et al. (2017) found an increase in tissue perfusion, which in turn could cause a decrease in mechanical stiffness. Moreover, Morales-Artacho et al. (2017) and Wilke et al. (2019) showed decreased mechanical stiffness immediately after foam rolling. However, previous studies investigated the immediate changes in muscle hardness or muscle stiffness after foam rolling, showing increased ROM but no significant differences in the aforementioned morphological outcomes (Yoshimura et al., 2020; Nakamura et al., 2021a). As a consequence, the initial assumptions that RM may primarily cause alterations of the mechanical soft tissue properties have been questioned (Behm and Wilke, 2019).
Considering the mixed evidence regarding local foam rolling effects, recent research has increasingly focused on neurophysiological changes. For instance, a previous study investigated the effect of rolling intervention on spinal excitability (Young et al., 2018) and corticospinal excitability (Aboodarda et al., 2018). With regard to functional outcomes, it was shown that foam rolling also induces changes in non-treated areas such as an increased ROM of the contralateral leg, which potentially reflects systemic changes in stretch sensation (i.e., stretch tolerance) (Kelly and Beardsley, 2016; Killen et al., 2019). Yet, contrarily, another previous study showed no significant increase in ROM of the contralateral leg (Grabow et al., 2017). Besides ROM, other studies examined the pressure pain threshold (PPT). Interestingly, some reported a non-local increase in PPT, again suggesting that neural changes could be responsible for flexibility changes after foam rolling (Aboodarda et al., 2015; Cavanaugh et al., 2017).
Summarizing the evidence regarding foam rolling mechanisms in a topical review, Behm and Wilke (2019) pointed out that the relative contributions of structural (e.g., passive stiffness) and neural effects (e.g., spinal excitability; i.e., H/M ratio) are yet to be elucidated. However, to our knowledge, no other study has examined the surrogates of both in one study. Examining structural and neural markers in the same participants and both the treated and non-treated body regions could help to understand better how foam rolling increases ROM.
Against this background, this study was geared to (1) investigate the effects of roller massage (RM) on passive tissue properties and neurological changes as well as their relationship with ROM in the treated leg and (2) investigate the possible cross-education effect of the passive properties and neurological parameters.
Materials and Methods
Experimental Design
A randomized, repeated-measures experimental design was used to investigate the neural and tissue-specific effects of RM. The participants, in random order, visited the laboratory five times (Figure 1). The first day was a familiarization session, including a demonstration of the RM intervention and the measurement protocols. The remaining four sessions included four measurement trials with an identical intervention (3 × 60-s RM on the calf). This approach with repeated interventions was chosen in order to prevent reciprocal interactions between the various measurements. The participants have been applied RM intervention in the dominant leg (prefer to kick the ball), and we defined the treated leg as ROLL, and the non-treated leg as NO-ROLL. The outcome variables consisted of two measurement trials, e.g., one trial was composed of passive tissue property measurements (DF ROM, passive torque, and shear elastic modulus) and PPT, and the other trial was composed of H/M recruitment curve (spinal excitability) measurement. Four measurements (2 trials [passive property and spinal excitability] × 2 sides [ROLL and NO-ROLL]) were conducted in random order with more than a 48-h interval.
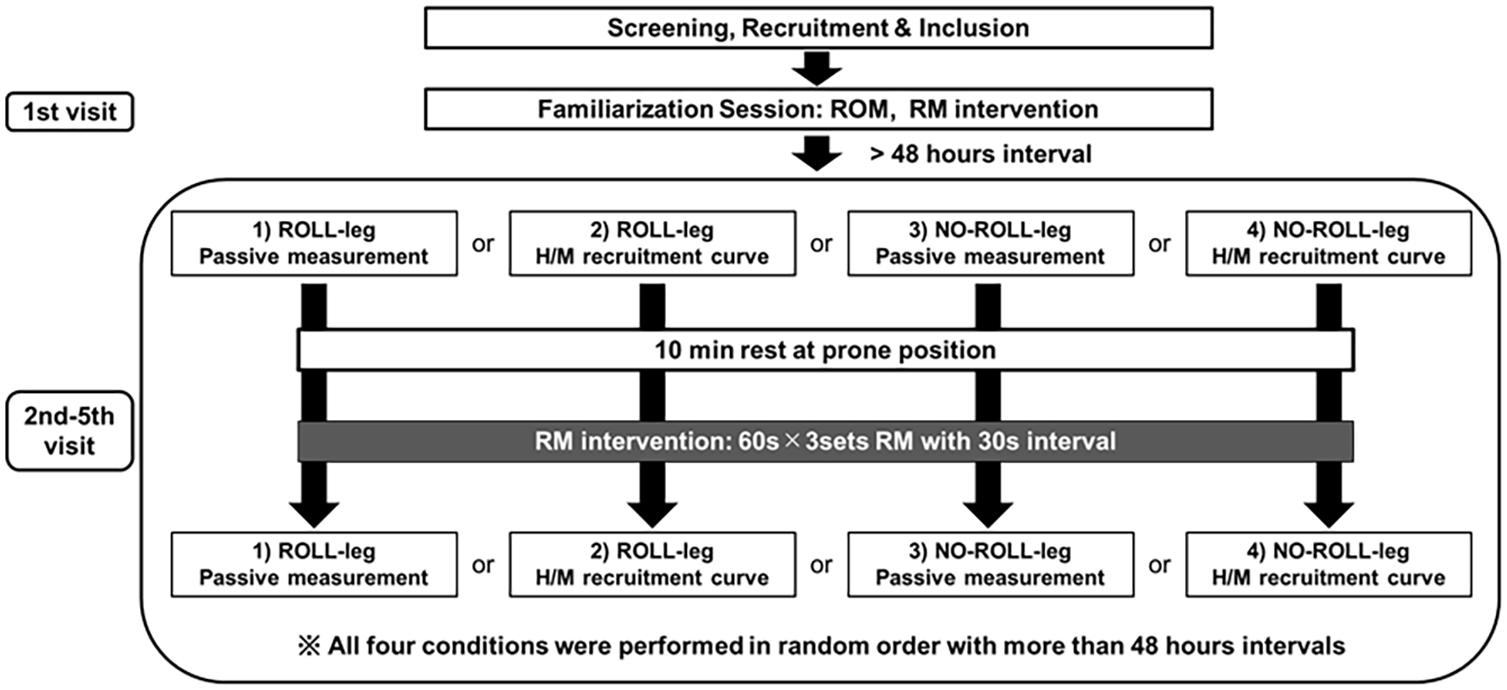
Figure 1. Flowchart of the study. The participants were applied roller massage (RM) intervention in the dominant leg, and we defined the treated leg as ROLL, and the non-treated leg as NO-ROLL, respectively. The outcome variables consisted of two trials, and one trial was composed of passive tissue property measurements (dorsiflexion range of motion [DF ROM], passive torque, and shear elastic modulus) and pressure pain threshold, and the other trial was composed of spinal excitability (H/M recruitment curve) measurement. Four measurements (2 sections × 2 sides [ROLL and NO-ROLL]) were conducted in random order with more than a 48-h interval.
The protocol for the four measurement days was identical and based on a previous study (Young et al., 2018). After the baseline measurement preceding the RM intervention (PRE), a 10-min break was given, and after the break, three sets of RM intervention for 60 s were performed. At 2 min after RM intervention, either assessment of the passive properties or spinal excitability (POST) measurement was performed.
Participants
Fifteen healthy adults volunteered to participate in this crossover study (mean ± SD: age, 22.8 ± 3.0 years; height, 165.2 ± 7.4 cm; weight, 58.7 ± 8.0 kg; male: female, 7: 8) Individuals with a history of neuromuscular disease and musculoskeletal injury involving the lower extremities were excluded. They were fully informed about the procedures and purpose of the study and provided written informed consent. The Ethics Committee of the Niigata University of Health and Welfare, Niigata, Japan (Procedure #18304), approved the study, which complied with the Declaration of Helsinki.
G∗Power (v 3.0.10; Dr. Franz Faul, Kiel University, Kiel, Germany) was used to perform an a priori sample-size calculation (primary outcome: ROM) for a repeated-measure analysis of variance (ANOVA) (effect size = 0.4, α = 0.05, and power = 0.80) based on a previous study (Phillips et al., 2018). It yielded a minimum of n = 15 individuals to be recruited for this study.
RM Intervention
Roller massage was applied using a roller massager (TheraBand, Akron, OH, United States). Participants were in a prone position on a treatment bed. An examiner moved the roller massager forward and backward, rolling on the muscle belly of the gastrocnemius. Speed was controlled with a metronome set to 60 bpm (Smart Metronome; Tomohiro Ihara, Japan). At each beep, the examiner foam moved the massager one stroke up or down. The intensity was steered based on the participants’ feedback, targeting a 7/10 in discomfort on a numerical rating scale (0, no discomfort; 10, maximal discomfort) (Smith et al., 2019; Kiyono et al., 2020a). Based on previous studies, participants performed three sets of RM for 60 s with a 30-s break between sets (Smith et al., 2019; Behm et al., 2020; Kiyono et al., 2020a).
Assessment of the DF ROM and Passive Torque
The participants were in the prone position with a 0° knee angle (i.e., the anatomical position), having the foot of the measured leg attached to the footplate of a dynamometer. Using the passive mode, the device moved the ankle into passive dorsiflexion at a speed of 5°/s, starting from 30° plantar flexion (i.e., anatomical neutral position was 0°) (Kiyono et al., 2020a; Nakamura et al., 2021b). Measurements were ended when the participants experienced discomfort and stopped the dynamometer by activating the safety trigger (Kiyono et al., 2020a; Nakamura et al., 2021b). The achieved angle at this point was documented as the DF ROM. The data collected during the ROM measurement was also used to calculate the passive–resistive torque of the ankle joint, which represents a measure of dynamic stiffness. In this study, dynamic stiffness was calculated as the change in the passive torque from the neutral ankle position (0°) to the least dorsiflexion angle before and after RM intervention divided by the change of the joint angle (Konrad et al., 2017). Additionally, passive torque at DF ROM was defined as the sensory perception index (Morse et al., 2008; Nakamura et al., 2018). DF ROM and passive torque were measured twice, utilizing its average value for further analysis.
Assessment of the Shear Elastic Modulus
Shear elastic modulus of MG was measured by means of an ultrasonic shear wave elastography (SWE) imaging device (Aixplorer SuperSonic Imagine, Aix-en-Provence, France) with a linear probe (4–15 MHz, SuperLinear 15-4, Vermon, Tours, France) and using the SWE mode (musculoskeletal preset, penetration mode, smoothing level 5, persistence medium). SWE provides a two-dimensional map of the shear elastic modulus on the B-mode image of the target tissue at 1 Hz with a spatial resolution of 1 × 1 mm (Lacourpaille et al., 2012; Umehara et al., 2021). Measurements were obtained at a neutral ankle position and 20° plantar flexion, which is similar to the positions of DF ROM measurements. We measured the shear elastic modulus at these angles to evaluate the changes in the passive mechanical property of the muscle under tension (neutral position) and without tension (20° plantar flexion). Also, the H and M waves were measured at 20° plantar flexion (described below), and we investigated the changes in the passive mechanical properties at that angle. The shear elastic modulus of MG was measured at 30% of the lower leg length from the popliteal crease to the lateral malleolus near the point of the lower leg maximal cross-sectional area (Nakamura et al., 2019; Kiyono et al., 2020a,b; Sato et al., 2020). The size of the region of interest was 10 × 20 mm2 near the center of MG, with a 5-mm-diameter-circle analysis area at the center of the region (Saeki et al., 2019). Elastographic images of the MG long axis were obtained twice. Based on previous studies (Nakamura et al., 2019; Fukaya et al., 2020; Sato et al., 2020), the shear elastic modulus was calculated by dividing the obtained Young’s modulus by 3, whereas the ultrasound measurements were measured twice in each ankle position. The average shear elastic modulus value was used for analysis.
Pressure Pain Threshold
For PPT measurements, we employed an algometer (NEUTONE TAM-22 (BT10); TRY ALL, Chiba, Japan). Participants adopted a prone position on the treatment bed similar to that for the DF ROM measurements. The joint position was 20° at the plantar flexion position. With continuously increasing pressure, the metal rod of the algometer was used to compress the soft tissue in the measurement area. Participants were instructed to immediately press a trigger when pain was experienced rather than just pressure. The value read from the device at this time point (kilograms per square centimeter) corresponded to the PPT. Based on previous studies (Kim and Lee, 2018; Naderi et al., 2020), the mean value (kilograms per square centimeter) of three repeated measurements was taken with a 30-s interval for data analysis.
Electromyography
Electromyographic activity of the soleus muscle was recorded using self-adhesive electrodes with recording surface electrodes (Ambu, Blue Sensor N). Before measurements, the skin was cleaned with alcohol to improve conductivity. Electrodes were placed on the soleus according to SENIAN (Hermens et al., 2000), with the ground electrode between the electrical stimulation and surface electromyogram electrodes. Electromyographic activity was filtered with a 10–1,000-Hz band-pass filter (Fa-DL-720-140, 4Assist, Tokyo, Japan) and digitally stored (10 kHz sampling rate) on a personal computer for offline analysis, which was performed using PowerLab 8/30 (AD Instruments, Colorado Springs, CO, United States) and LabChart 7 (AD Instruments). In the passive dorsiflexion test, the soleus muscle activity was monitored to confirm whether the participant was relaxed, ensuring no high EMG activity occurrence.
H-Reflex, M-Wave
In the spinal cord mononeuron excitability assessment, participants were instructed to lie in a prone position on the treatment bed with the knee at a 0° knee angle and the ankle in a 20° plantar flexion position. The participants were requested to relax completely, which we ensured by controlling EMG activity. In this study, electrical stimulations were delivered using a constant current stimulator (Isolator SS-104 J: Nihon Kohden Corporation) targeting the tibial nerve using 1-ms pulses. Based on previous researches (Hirabayashi et al., 2018, 2020), the tibial nerve was selectively stimulated in a monopolar manner, inducing soleus H-reflex and M waves. The anode was placed on the patella and the cathode on the popliteal fossa overlying the nerve at a position using the electrode (Blue Sensor N, size: 30 × 22 mm), providing the greatest H wave amplitude at the smallest stimulus intensity, which was identified by stimulating the different skin surface sites with relatively low currents. Electrodes were attached to the skin using surgical tape to prevent unwanted movement during testing.
H/M recruitment curves were assessed before and after sessions. At the beginning of each, H-reflex and M wave recruitment curves were measured under resting conditions to determine the H-reflex (Hmax) and M wave (Mmax) maximum amplitudes. Stimulations were increased by 0.5 mA every 10 s until Mmax was reached, with the corresponding intensity for all stimulations retained for analysis. The three stable Hmax and Mmax are measured, and the average value of each is used for analysis. The H/M ratio, an index of spinal excitability, was calculated from the measured Hmax and Mmax (Blazevich et al., 2012, 2014; Stutzig and Siebert, 2017).
Pilot Measurements of Test–Retest Reliability
The test–retest reliability of the measurements (all variables) was determined by the coefficient variation (CV) and intraclass correlation coefficient (ICC) using eight healthy students (four men, four women, 21.9 ± 1.1 years, 162.9 ± 7.3 cm, 55.2 ± 9.0 kg), with a 1-week washout between the two measures. The CV of and passive torque at DF ROM, PPT, shear elastic modulus at 0° and 20°, and H/M ratio were 9.9 ± 13.3, 6.4 ± 3.9, 4.0 ± 3.3, 2.0 ± 1.4, 3.6 ± 2.4, and 9.7 ± 8.7%, respectively, and the ICCs for the measurements were 0.96, 0.96, 0.97, 0.92, 0.91, 0.88, and 0.96, respectively.
Statistical Analysis
Normal distribution of data was assessed with the Shapiro–Wilk test. Examinations showed that no violations of the testing assumption were present. Comparisons of the parameters’ PRE values were made between the ROLL and NO-ROLL legs using the paired t-test. If a significant difference was noted between PRE values, we used a paired t-test with Bonferroni corrections to compare the significant differences between the PRE and POST values of each leg, and the Wilcoxon signed-rank test was used to compare the pre-post changes (in percentage) between the ROLL and NO-ROLL leg. Conversely, if no significant difference was noted between PRE values, a two-way repeated-measure ANOVA (time [PRE vs. POST] × legs [ROLL leg vs. NO-ROLL leg]) was used to investigate the interactions and main effects in the experiment. If a main effect for time (without interaction) was noted, significant differences between the PRE and POST values were determined using a paired t-test. The effect size was calculated as a difference in the mean value between PRE and POST divided by the pooled standard deviation (SD) (Cohen, 1988) with effect sizes of 0.00–0.19, 0.20–0.49, 0.50–0.79, and ≥0.80 being considered trivial, small, moderate, and large, respectively. Finally, the correlations between changes in ROM and each variable using Spearman’s rank correlation coefficient were investigated. A 5% significance level was used. All results are shown as mean ± SD. The Statistical Package for the Social Sciences, version 24.0 (IBM Corp., Armonk, NY, United States) was used for statistical analyses.
Results
Comparison of Preintervention Values
All values are shown in Tables 1, 2. Paired t-tests showed a significant difference in PPT between the PRE values of the ROLL and NO-ROLL legs (p = 0.018; Table 2). No significant baseline differences were found concerning the other parameters (DF ROM, dynamic stiffness, passive torque at DF ROM, shear elastic modulus, and H/M ratio).
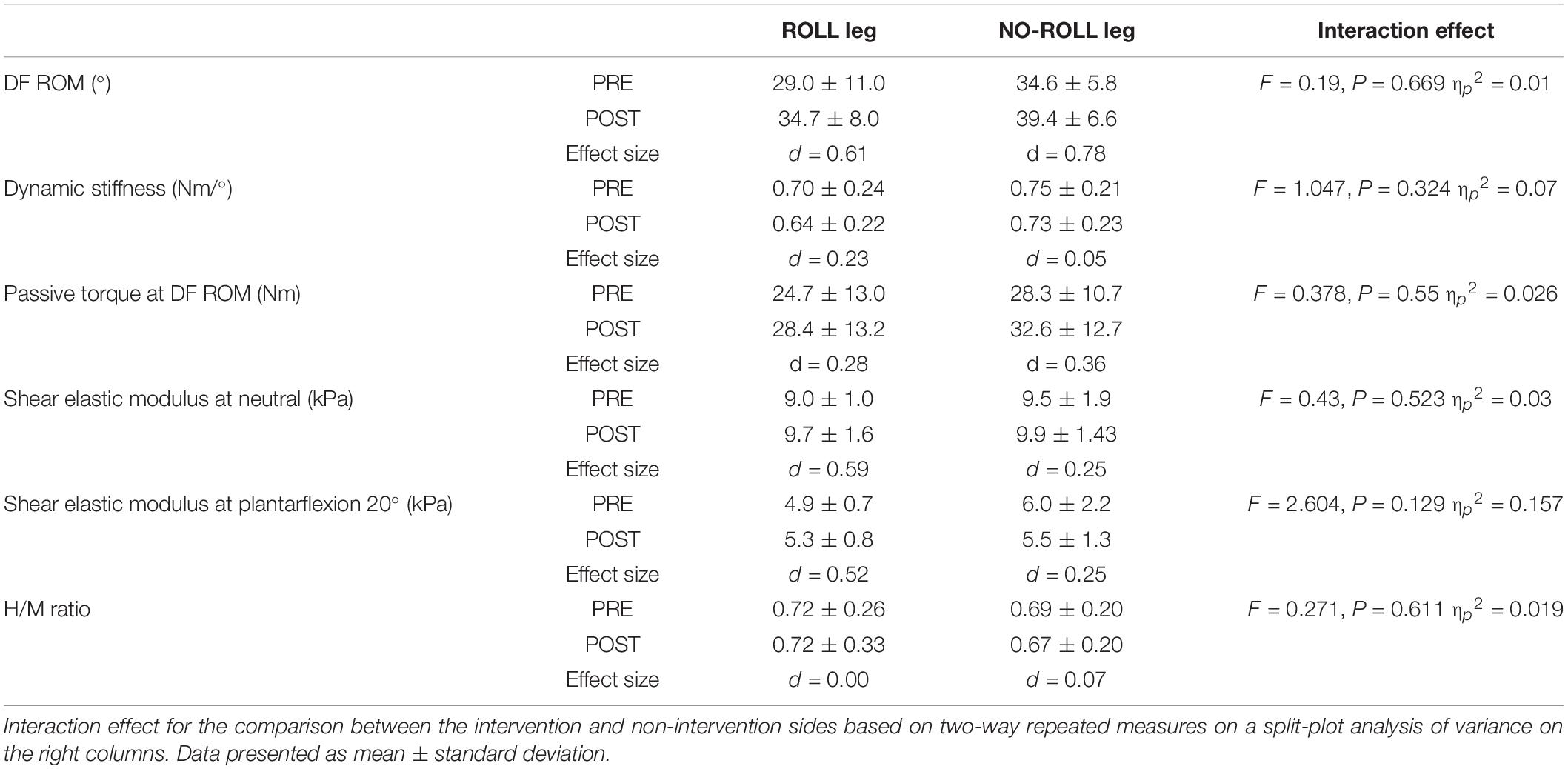
Table 1. Change in dorsiflexion range of motion (DF ROM), dynamic stiffness, passive torque at DF ROM, shear elastic modulus, and H/M ratio before (PRE) and after (POST) the roller massage intervention for treated side (ROLL) and non-treated side (NO-ROLL) legs.
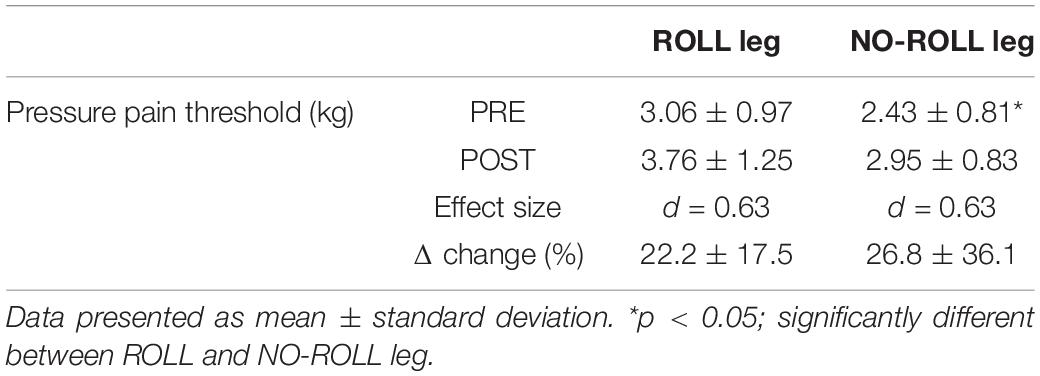
Table 2. Change in pressure pain threshold before (PRE) and after (POST) the roller massage intervention for treated side (ROLL) and non-treated side (NO-ROLL) legs.
Pre–Post Changes in the ROLL and NO-ROLL Legs
The two-way repeated-measure analysis of variance did not show interaction effects (ROLL/NO-ROLL leg) in any other outcome (p > 0.05; Table 1). Main effects for time were found in DF ROM and passive torque at DF ROM (p < 0.05) but not for dynamic stiffness, shear elastic modulus, and H/M ratio (p > 0.05). According to post hoc testing with paired t-tests, DF ROM and PPT increased after RM (p < 0.01). In addition, regarding PPT, paired t-tests with Bonferroni corrections showed a significant increase after RM intervention in both ROLL and NO-ROLL sides, but the Wilcoxon signed-rank test revealed no significant difference in the relative pre–post changes between the ROLL and NO-ROLL leg (p = 0.609; Table 2).
Relationship Between the Changes in DF ROM and All Variables
Significant correlations were observed between the changes in DF ROM and passive torque at DF ROM in both ROLL and NO-ROLL legs (Figure 2A: rs = 0.761, p < 0.01; Figure 2B: rs = 0.660, p < 0.01, respectively), whereas no significant associations occurred between changes in DF ROM and PPT (rs = 0.311, p = 0.26; rs = 0.048, p = 0.864), dynamic stiffness (rs = 0.129, p = 0.65; rs = –0.176, p = 0.53), shear elastic modulus at neutral (rs = 0.418, p = 0.121; rs = −0.021, p = 0.94), 20° plantarflexion (rs = 0.325, p = 0.237; rs = −0.15, p = 0.594), and H/M ratio (rs = 0.136, p = 0.63; rs = 0.239, p = 0.39) at both legs.
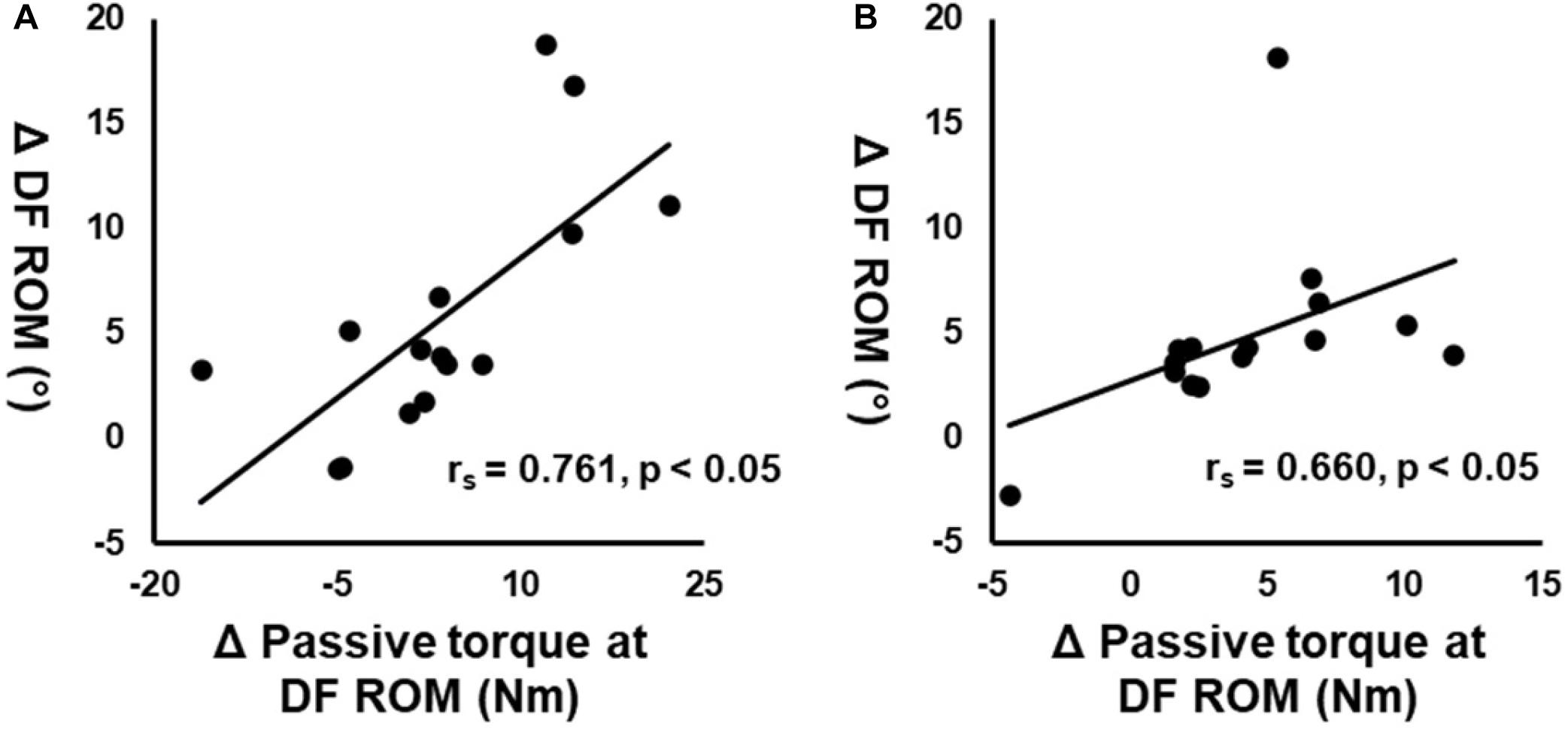
Figure 2. Correlation between the changes in dorsiflexion range of motion (DF ROM) before (PRE) and after roller massage (POST) and changes in passive torque at DF ROM on the treated (A) and non-treated (B) legs.
Discussion
To the best of our knowledge, our study was the first to jointly investigate the acute effects of RM on structural and neuronal outcomes. On both sides, the results showed a significant increase in DF ROM and PPT but no significant changes in dynamic stiffness, shear elastic modulus, and spinal excitability. Since the changes in DF ROM correlated with changes in passive torque at DF ROM, the increasing ROM after the RM intervention could be associated with stretch tolerance changes on both treated and non-treated sides.
The ROM increases following RM in ROLL and NO-ROLL are generally in accordance with the findings of previous trials (Kelly and Beardsley, 2016; Killen et al., 2019). Beyond this, we found no significant interaction effect and no significant difference in the changes between legs. Similar to our findings, Kelly and Beardsley (2016) and Killen et al. (2019) reported no significant difference in the effects of foam rolling on ROM in the two legs. In sum, it appears that ROM increases after foam rolling locally and remotely at a comparable magnitude. This may mean that foam rolling-induced ROM changes largely stem from neural mechanisms, i.e., stretch tolerance, and to a minor degree by local/structural alterations, i.e., passive stiffness of the muscle–tendon unit or the skeletal muscles.
In addition to increased ROM, the present study found significant changes in PPT on both sides. Previous studies (Cheatham and Baker, 2017; Cheatham and Stull, 2019) showed similar results, which strengthens the assumption that foam rolling modifies sensory perception. Behm and Wilke (2019) proposed that the gate control theory, parasympathetic hyperactivity, and diffuse noxious inhibitory control could be involved. Previous studies suggested the involvement of the central pain modulatory system in contralateral PPT increase (Aboodarda et al., 2015; Cavanaugh et al., 2017). Although the exact mechanism in the present study is unknown, the aforementioned processes may also have caused the changes in PPT in our case.
Foam rolling and RM intervention have been shown to modify myofascial viscoelastic properties by mechanisms such as thixotropy (reduced viscosity), myofascial restriction reduction, fluid changes, and cellular responses (Kelly and Beardsley, 2016; Cheatham and Stull, 2019). No significant changes were found in dynamic stiffness and shear elastic modulus in both ROLL and NO-ROLL legs, respectively. This is of interest because Morales-Artacho et al. (2017) reported a significant decrease in hamstring muscle stiffness immediately after foam rolling. Contrarily, Yoshimura et al. (2020) showed no significant decrease in muscle hardness of the gastrocnemius after a 300-s foam rolling intervention. In addition, Nakamura et al. (2021a) showed that different duration foam rolling intervention (30, 90, and 300 s) could not cause changes in shear elastic modulus of the same muscle, which is consistent with the results of the present study (Nakamura et al., 2021a). Consequently, the different target muscles may respond individually to foam rolling intervention, and the mechanical effects of foam rolling seem to require further investigation.
For the ROM change noted after the RM intervention, Grabow et al. (2018) suggested that ROM increases are related to a spinal excitability change. A previous study (Young et al., 2018) investigated RM effects on the H-reflex and reported a decrease during RM, with transient effects and an immediate return to baseline after the intervention. However, our study showed no significant change in the H/M ratio. The difference between the trial of Young and colleagues and ours’ may be explained by the timing of measurements. The previous study (Young et al., 2018) measured the H-reflex during and immediately after the RM intervention, whereas the current study examined it at 2 min post-intervention. Therefore, RM-induced changes of spinal excitability may occur during the intervention but with effects that return immediately to baseline after the intervention.
Our correlation analysis showed a significant association between the changes in DF ROM and the passive torque at DF ROM in both ROLL and NO-ROLL (Figures 2A,B) but not in other variables. These results suggested that the DF ROM increase on both sides after RM was related to changes in stretch perception, i.e., stretch tolerance. According to previous studies, moderate-to-strong correlations between ROM and passive torque changes at ROM were indicated as altered type III or IV afferent activity, influencing pain perception and the magnitude of ROM changes (Magnusson et al., 1996; Weppler and Magnusson, 2010). In addition, this association could be good evidence for the importance of neurological adaptation (i.e., change in stretch tolerance) for the acute increase in ROM (Kay et al., 2015). Killen et al. (2019) found that foam rolling intervention increased ROM on the non-intervention side, suggesting that stretch tolerance was involved in the increase in ROM. Moreover, Aboodarda et al. (2015) showed that PPT was increased after a foam rolling intervention on both the intervention side and the non-intervention side. Thus, this suggested that the central nervous system changes; for example, the noxious counter-irritating mechanism is responsible for increasing PPT on the non-intervention side after the foam rolling intervention (Aboodarda et al., 2015). The details of the mechanism of stretch tolerance change in the non-intervention side (NO-ROLL) are unclear in this study, but it is possible that the central nervous system could be responsible for stretch tolerance change and the increase in ROM on the non-intervention side. However, Behm and Wilke (2019) suggested that PPT change is related to the increase in ROM after foam rolling intervention. Although the present study results showed increased PPT on both legs, no significant correlations were observed between the changes in DF ROM and PPT. The difference between the changes in PPT and stretch tolerance is unclear. Also, PPT measurement assessed not deep tissue but superficial tissue, and therefore might not assess RM-induced muscle changes. The present study suggested that PPT changes are not related to ROM changes.
Other factors involved in the ROM change could be changes in muscle stiffness and/or spinal excitability. However, the current study showed that RM intervention increased ROM, with no changes in shear elastic modulus and H/M ratio, i.e., mechanical property and neurological change, suggesting that muscle stiffness and H/M ratio changes were not related to DF ROM increase in both legs after the RM intervention. Young et al. (2018) showed decreased H-reflex during RM intervention, which returned to baseline after RM intervention. In addition, the results of this study showed no significant changes in H/M ratio and no significant correlations between the changes in DF ROM and H/M ratio on both legs. Thus, the present study suggested that muscle stiffness and H/M ratio changes were not related to the increase in ROM after RM intervention.
This study had several limitations. In the previous study (Young et al., 2018), H-reflex was measured during the RM intervention. In contrast, in this study, as indicated, the H/M ratio was measured only 2 min after the RM intervention. Future studies may consider investigating the effects of RM interventions on H/M ratio and muscle stiffness during RM application. Also, we did not measure the control condition (without RM intervention). In addition, because the different measurements may mutually influence each other, we had to repeat the treatment four times on separate days. Thus, although we used the exact same protocol in the same participants in one study, the different outcomes, technically, have not been assessed simultaneously.
Conclusion
This study investigated the effect of RM on DF ROM, passive torque, shear elastic modulus, PPT, and H/M ratio on both the intervention and non-intervention sides. The results showed that DF ROM and PPT increased after treatment in both legs. The increases in ROM were related to the changes in stretch tolerance but not muscle stiffness, PPT, and spinal excitability. Interestingly, this study showed the same degree of RM intervention effect on DF ROM and the intervention and non-intervention sides. These results could be a treatment option in clinical and sports settings. Specifically, unilateral RM intervention on the healthy side may maintain or increase the ROM of the disabled side even if RM intervention could not be adaptable owing to injury or pain.
Data Availability Statement
The raw data supporting the conclusions of this article will be made available by the authors, without undue reservation.
Ethics Statement
The studies involving human participants were reviewed and approved by this study was approved by the Ethics Committee of the Niigata University of Health and Welfare, Niigata, Japan (Procedure #18304), and has complied with the Declaration of Helsinki requirements. The patients/participants provided their written informed consent to participate in this study.
Author Contributions
MN contributed to the study design and data collection, and drafted and critical revision to the manuscript. RK, SS, KaY, RY, KoY, YM, and FS contributed to the data collection and made critical revisions to the manuscript. AK and JW contributed to the study design and data analysis and made critical revisions to the manuscript. All the authors approved the final version of the manuscript and agreed to be accountable for all aspects of the work.
Funding
This work was supported by JSPS KAKENHI with grant number 19K19890 (MN), and the Austrian Science Fund (FWF) project P 32078-B (AK).
Conflict of Interest
The authors declare that the research was conducted in the absence of any commercial or financial relationships that could be construed as a potential conflict of interest.
Acknowledgments
The authors gratefully acknowledge all participants involved in this study. The authors would like to thank Enago (http://www.enago.jp/) for editorial assistance with the manuscript.
References
Aboodarda, S. J., Greene, R. M., Philpott, D. T., Jaswal, R. S., Millet, G. Y., and Behm, D. G. (2018). The effect of rolling massage on the excitability of the corticospinal pathway. Appl. Physiol. Nutr. Metab. 43, 317–323. doi: 10.1139/apnm-2017-0408
Aboodarda, S. J., Spence, A. J., and Button, D. C. (2015). Pain pressure threshold of a muscle tender spot increases following local and non-local rolling massage. BMC Musculoskelet Disord. 16:265. doi: 10.1186/s12891-015-0729-5
Behara, B., and Jacobson, B. H. (2017). Acute effects of deep tissue foam rolling and dynamic stretching on muscular strength, power, and flexibility in division I Linemen. J. Strength Cond. Res. 31, 888–892. doi: 10.1519/JSC.0000000000001051
Behm, D. G., Alizadeh, S., Hadjizadeh Anvar, S., Mahmoud, M. M. I., Ramsay, E., Hanlon, C., et al. (2020). Foam rolling prescription: A mentary. J. Strength Cond. Res. 34, 3301–3308. doi: 10.1519/JSC.0000000000003765
Behm, D. G., Blazevich, A. J., Kay, A. D., and Mchugh, M. (2016). Acute effects of muscle stretching on physical performance, range of motion, and injury incidence in healthy active individuals: a systematic review. Appl. Physiol. Nutr. Metab. 41, 1–11. doi: 10.1139/apnm-2015-0235
Behm, D. G., Kay, A. D., Trajano, G. S., and Blazevich, A. J. (2021). Mechanisms underlying performance impairments following prolonged static stretching without a comprehensive warm-up. Eur. J. Appl. Physiol. 121, 67–94. doi: 10.1007/s00421-020-04538-8
Behm, D. G., and Wilke, J. (2019). Do self-myofascial release devices release Myofascia? rolling mechanisms: a narrative review. Sports Med. 49, 1173–1181. doi: 10.1007/s40279-019-01149-y
Blazevich, A. J., Cannavan, D., Waugh, C. M., Miller, S. C., Thorlund, J. B., Aagaard, P., et al. (2014). Range of motion, neuromechanical, and architectural adaptations to plantar flexor stretch training in humans. J. Appl. Physiol. 117, 452–462. doi: 10.1152/japplphysiol.00204.2014
Blazevich, A. J., Kay, A. D., Waugh, C., Fath, F., Miller, S., and Cannavan, D. (2012). Plantarflexor stretch training increases reciprocal inhibition measured during voluntary dorsiflexion. J. Neurophysiol. 107, 250–256. doi: 10.1152/jn.00407.2011
Cavanaugh, M. T., Döweling, A., Young, J. D., Quigley, P. J., Hodgson, D. D., Whitten, J. H., et al. (2017). An acute session of roller massage prolongs voluntary torque development and diminishes evoked pain. Eur. J. Appl. Physiol. 117, 109–117. doi: 10.1007/s00421-016-3503-y
Cheatham, S. W., and Baker, R. (2017). Differences in pressure pain threshold among men and women after foam rolling. J. Bodyw. Mov. Ther. 21, 978–982. doi: 10.1016/j.jbmt.2017.06.006
Cheatham, S. W., and Stull, K. R. (2019). Roller massage: Comparison of three different surface type pattern foam rollers on passive knee range of motion and pain perception. J. Bodyw. Mov. Ther. 23, 555–560. doi: 10.1016/j.jbmt.2019.05.002
Cohen, J. (ed). (1988). Statistical Power Analysis for the Behavioral Sciences. Routledge: Hillsdale.
Fukaya, T., Nakamura, M., Sato, S., Kiyono, R., Yahata, K., Inaba, K., et al. (2020). The relationship between stretching intensity and changes in passive properties of gastrocnemius muscle-tendon unit after static stretching. Sports (Basel) 8:140. doi: 10.3390/sports8110140
Grabow, L., Young, J. D., Alcock, L. R., Quigley, P. J., Byrne, J. M., Granacher, U., et al. (2018). Higher quadriceps roller massage forces do not amplify range-of-motion increases nor impair strength and jump performance. J. Strength Cond. Res. 32, 3059–3069. doi: 10.1519/JSC.0000000000001906
Grabow, L., Young, J. D., Byrne, J. M., Granacher, U., and Behm, D. G. (2017). Unilateral rolling of the foot did not affect non-local range of motion or balance. J. Sports Sci. Med. 16, 209–218.
Hermens, H. J., Freriks, B., Disselhorst-Klug, C., and Rau, G. (2000). Development of recommendations for SEMG sensors and sensor placement procedures. J. Electromyogr. Kinesiol. 10, 361–374. doi: 10.1016/S1050-6411(00)00027-4
Hotfiel, T., Swoboda, B., Krinner, S., Grim, C., Engelhardt, M., Uder, M., et al. (2017). Acute effects of lateral thigh foam rolling on arterial tissue perfusion determined by spectral doppler and power doppler ultrasound. J. Strength Cond. Res. 31, 893–900. doi: 10.1519/JSC.0000000000001641
Hirabayashi, R., Edama, M., Kojima, S., Nakamura, M., Ito, W., Nakamura, E., et al. (2018). Effects of reciprocal Ia inhibition on contraction intensity of Co-contraction. Front. Hum. Neurosci. 12:527. doi: 10.3389/fnhum.2018.00527
Hirabayashi, R., Kojima, S., Edama, M., and Onishi, H. (2020). Activation of the supplementary motor areas enhances spinal reciprocal inhibition in healthy individuals. Brain Sci. 10:587. doi: 10.3390/brainsci10090587
Kay, A. D., and Blazevich, A. J. (2012). Effect of acute static stretch on maximal muscle performance: a systematic review. Med. Sci. Sports Exerc. 44, 154–164. doi: 10.1249/MSS.0b013e318225cb27
Kay, A. D., Husbands-Beasley, J., and Blazevich, A. J. (2015). Effects of contract-relax, static stretching, and isometric contractions on muscle-tendon mechanics. Med. Sci. Sports Exerc. 47, 2181–2190. doi: 10.1249/MSS.0000000000000632
Kelly, S., and Beardsley, C. (2016). Specific and cross-over effects of foam rolling on ankle dorsiflexion range of motion. Int. J. Sports Phys. Ther. 11, 544–551.
Killen, B. S., Zelizney, K. L., and Ye, X. (2019). Crossover effects of unilateral static stretching and foam rolling on contralateral hamstring flexibility and strength. J. Sport Rehabil. 28, 533–539. doi: 10.1123/jsr.2017-0356
Kim, S. J., and Lee, J. H. (2018). Effects of sternocleidomastoid muscle and suboccipital muscle soft tissue release on muscle hardness and pressure pain of the sternocleidomastoid muscle and upper trapezius muscle in smartphone users with latent trigger points. Medicine (Baltimore) 97:e12133. doi: 10.1097/MD.0000000000012133
Kiyono, R., Onuma, R., Yasaka, K., Sato, S., Yahata, K., and Nakamura, M. (2020a). Effects of 5-week foam rolling intervention on range of motion and muscle stiffness. J. Strength Cond. Res. 2020:33044364. doi: 10.1519/JSC.0000000000003757
Kiyono, R., Sato, S., Inaba, K., Yahata, K., and Nakamura, M. (2020b). Time course of changes in range of motion, muscle shear elastic modulus, spinal excitability, and muscle temperature during superficial icing. Sport Sci. Health 17, 341–346. doi: 10.1007/s11332-020-00693-9
Konrad, A., Stafilidis, S., and Tilp, M. (2017). Effects of acute static, ballistic, and PNF stretching exercise on the muscle and tendon tissue properties. Scand. J. Med. Sci. Sports 27, 1070–1080. doi: 10.1111/sms.12725
Krause, F., Wilke, J., Niederer, D., Vogt, L., and Banzer, W. (2019). Acute effects of foam rolling on passive stiffness, stretch sensation and fascial sliding: A randomized controlled trial. Hum. Mov. Sci. 67:102514. doi: 10.1016/j.humov.2019.102514
Lacourpaille, L., Hug, F., Bouillard, K., Hogrel, J. Y., and Nordez, A. (2012). Supersonic shear imaging provides a reliable measurement of resting muscle shear elastic modulus. Physiol. Meas. 33, N19–N28. doi: 10.1088/0967-3334/33/3/N19
Macdonald, G. Z., Penney, M. D., Mullaley, M. E., Cuconato, A. L., Drake, C. D., Behm, D. G., et al. (2013). An acute bout of self-myofascial release increases range of motion without a subsequent decrease in muscle activation or force. J. Strength Cond. Res. 27, 812–821. doi: 10.1519/JSC.0b013e31825c2bc1
Magnusson, S. P., Simonsen, E. B., Aagaard, P., Sørensen, H., and Kjaer, M. (1996). A mechanism for altered flexibility in human skeletal muscle. J. Physiol. 497, 291–298. doi: 10.1113/jphysiol.1996.sp021768
Morales-Artacho, A. J., Lacourpaille, L., and Guilhem, G. (2017). Effects of warm-up on hamstring muscles stiffness: cycling vs foam rolling. Scand. J. Med. Sci. Sports 27, 1959–1969. doi: 10.1111/sms.12832
Morse, C. I., Degens, H., Seynnes, O. R., Maganaris, C. N., and Jones, D. A. (2008). The acute effect of stretching on the passive stiffness of the human gastrocnemius muscle tendon unit. J. Physiol. 586, 97–106. doi: 10.1113/jphysiol.2007.140434
Naderi, A., Rezvani, M. H., and Degens, H. (2020). Foam rolling and muscle and joint proprioception after exercise-induced muscle damage. J. Athl. Train 55, 58–64. doi: 10.4085/1062-6050-459-18
Nakamura, M., Hirabayashi, R., Ohya, S., Aoki, T., Suzuki, D., Shimamoto, M., et al. (2018). Effect of static stretching with superficial cooling on muscle stiffness. Sports Med. Int. Open 2, E142–E147. doi: 10.1055/a-0684-9375
Nakamura, M., Onuma, R., Kiyono, R., Yasaka, K., Sato, S., Yahata, K., et al. (2021a). The acute and prolonged effects of different durations of foam rolling on range of motion, muscle stiffness, and muscle strength. J. Sports Sci. Med. 20, 62–68. doi: 10.52082/jssm.2021.62
Nakamura, M., Sato, S., Kiyono, R., Yahata, K., Yoshida, R., Fukaya, T., et al. (2021b). Comparison of the acute effects of hold-relax and static stretching among older adults. Biology (Basel) 10:126. doi: 10.3390/biology10020126
Nakamura, M., Sato, S., Kiyono, R., Takahashi, N., and Yoshida, T. (2019). Effect of rest duration between static stretching on passive stiffness of medial gastrocnemius muscle in vivo. J. Sport Rehabil. 29, 578–582. doi: 10.1123/jsr.2018-0376
Phillips, J., Diggin, D., King, D. L., and Sforzo, G. A. (2018). Effect of varying self-myofascial release duration on subsequent athletic performance. J. Strength Cond. Res. 35, 746–753. doi: 10.1519/JSC.0000000000002751
Pickering Rodriguez, E. C., Watsford, M. L., Bower, R. G., and Murphy, A. J. (2017). The relationship between lower body stiffness and injury incidence in female netballers. Sports Biomech. 16, 361–373. doi: 10.1080/14763141.2017.1319970
Sato, S., Kiyono, R., Takahashi, N., Yoshida, T., Takeuchi, K., and Nakamura, M. (2020). The acute and prolonged effects of 20-s static stretching on muscle strength and shear elastic modulus. PLoS One 15:e0228583. doi: 10.1371/journal.pone.0228583
Saeki, J., Ikezoe, T., Yoshimi, S., Nakamura, M., and Ichihashi, N. (2019). Menstrual cycle variation and gender difference in muscle stiffness of triceps surae. Clin. Biomech. 61, 222–226. doi: 10.1016/j.clinbiomech.2018.12.013
Smith, J. C., Washell, B. R., Aini, M. F., Brown, S., and Hall, M. C. (2019). Effects of static stretching and foam rolling on ankle dorsiflexion range of motion. Med. Sci. Sports Exerc. 51, 1752–1758. doi: 10.1249/MSS.0000000000001964
Stutzig, N., and Siebert, T. (2017). Assessment of the H-reflex at two contraction levels before and after fatigue. Scand. J. Med. Sci. Sports 27, 399–407. doi: 10.1111/sms.12663
Umehara, J., Nakamura, M., Saeki, J., Tanaka, H., Yanase, K., Fujita, K., et al. (2021). Acute and prolonged effects of stretching on shear modulus of the pectoralis minor muscle. J. Sports Sci. Med. 20, 17–25. doi: 10.52082/jssm.2021.17
Weppler, C. H., and Magnusson, S. P. (2010). Increasing muscle extensibility: a matter of increasing length or modifying sensation? Phys. Ther. 90, 438–449. doi: 10.2522/ptj.20090012
Wilke, J., Müller, A. L., Giesche, F., Power, G., Ahmedi, H., and Behm, D. G. (2020). Acute effects of foam rolling on range of motion in healthy adults: a systematic review with multilevel meta-analysis. Sports Med. 50, 387–402. doi: 10.1007/s40279-019-01205-7
Wilke, J., Niemeyer, P., Niederer, D., Schleip, R., and Banzer, W. (2019). Influence of foam rolling velocity on Knee Range of motion and tissue stiffness: a randomized, controlled crossover trial. J. Sport Rehabil. 28, 711–715. doi: 10.1123/jsr.2018-0041
Witvrouw, E., Bellemans, J., Lysens, R., Danneels, L., and Cambier, D. (2001). Intrinsic risk factors for the development of patellar tendinitis in an athletic population. A two-year prospective study. Am. J. Sports Med. 29, 190–195. doi: 10.1177/03635465010290021201
Yoshimura, A., Schleip, R., and Hirose, N. (2020). Effects of self-massage using a foam roller on ankle range of motion and gastrocnemius fascicle length and muscle hardness: A pilot study. J. Sport Rehabil. 29, 1171–1178. doi: 10.1123/jsr.2019-0281
Keywords: shear elastic modulus, dorsiflexion range of motion, roller massage, cross-transfer effect, H/M ratio
Citation: Nakamura M, Konrad A, Kiyono R, Sato S, Yahata K, Yoshida R, Yasaka K, Murakami Y, Sanuki F and Wilke J (2021) Local and Non-local Effects of Foam Rolling on Passive Soft Tissue Properties and Spinal Excitability. Front. Physiol. 12:702042. doi: 10.3389/fphys.2021.702042
Received: 28 April 2021; Accepted: 20 May 2021;
Published: 25 June 2021.
Edited by:
Trevor Chung-Ching Chen, National Taiwan Normal University, TaiwanReviewed by:
David George Behm, Memorial University of Newfoundland, CanadaXin Ye, University of Hartford, United States
Copyright © 2021 Nakamura, Konrad, Kiyono, Sato, Yahata, Yoshida, Yasaka, Murakami, Sanuki and Wilke. This is an open-access article distributed under the terms of the Creative Commons Attribution License (CC BY). The use, distribution or reproduction in other forums is permitted, provided the original author(s) and the copyright owner(s) are credited and that the original publication in this journal is cited, in accordance with accepted academic practice. No use, distribution or reproduction is permitted which does not comply with these terms.
*Correspondence: Masatoshi Nakamura, bWFzYXRvc2hpLW5ha2FtdXJhQG51aHcuYWMuanA=