- 1Laboratory of Pharmacology, School of Pharmaceutical Sciences, São Paulo State University (UNESP), Araraquara, Brazil
- 2Joint Federal University of São Carlos (UFSCar) - São Paulo State University (UNESP) Graduate Program in Physiological Sciences, São Carlos, Brazil
- 3Institute of Biological Sciences, Federal University of Goiás, Goiânia, Brazil
The prelimbic (PL) and infralimbic (IL) subareas of the medial prefrontal cortex (mPFC) have been implicated in physiological and behavioral responses during aversive threats. The previous studies reported the noradrenaline release within the mPFC during stressful events, and the lesions of catecholaminergic terminals in this cortical structure affected stress-evoked local neuronal activation. Nevertheless, the role of mPFC adrenoceptors on cardiovascular responses during emotional stress is unknown. Thus, we investigated the role of adrenoceptors present within the PL and IL on the increase in both arterial pressure and heart rate (HR) and on the sympathetically mediated cutaneous vasoconstriction evoked by acute restraint stress. For this, bilateral guide cannulas were implanted into either the PL or IL of male rats. All animals were also subjected to catheter implantation into the femoral artery for cardiovascular recording. The increase in both arterial pressure and HR and the decrease in the tail skin temperature as an indirect measurement of sympathetically mediated cutaneous vasoconstriction were recorded during the restraint session. We observed that the microinjection of the selective α2-adrenoceptor antagonist RX821002 into either the PL or IL decreased the pressor response during restraint stress. Treatment of the PL or IL with either the α1-adrenoceptor antagonist WB4101 or the α2-adrenoceptor antagonist reduced the restraint-evoked tachycardia. The drop in the tail skin temperature was decreased by PL treatment with the β-adrenoceptor antagonist propranolol and with the α1- or α2-adrenoceptor antagonists. The α2-adrenoceptor antagonist into the IL also decreased the skin temperature response. Our results suggest that the noradrenergic neurotransmission in both PL and IL mediates the cardiovascular responses to aversive threats.
Introduction
The survival and adaptation of all species to aversive threats depend on a tightly controlled set of physiological responses (Sterling, 2012; McEwen, 2017). The autonomic nervous system evokes the immediate adjustments during stress that includes; for instance, the increase in arterial pressure and heart rate (HR) and the sympathetically mediated cutaneous vasoconstriction (Dampney, 2015; Crestani, 2016). The vasoconstriction of the cutaneous beds decreases the skin temperature (Vianna and Carrive, 2005; Busnardo et al., 2019). Although the relevance of the physiological responses during aversive situations, the neurobiological mechanisms involved are not completely understood.
The medial prefrontal cortex (mPFC) is activated by several aversive stimuli, such as restraint stress (Cullinan et al., 1995; Yokoyama and Sasaki, 1999; Figueiredo et al., 2002). Accordingly, the prelimbic (PL) and infralimbic (IL) subregions of the mPFC have been reported as the prominent regulators of the physiological stress responses (Ulrich-Lai and Herman, 2009; McKlveen et al., 2015, 2019; Myers, 2017). In this sense, some studies have reported that these mPFC subregions display opposite roles in the control of the responses during stressful events. For instance, studies comparing these regions identified that the PL ablation enhanced the activation of the hypothalamic–pituitary–adrenal (HPA) axis and the cardiovascular reactivity evoked by acute aversive stimuli, while the inhibition of the IL decreased these responses (Frysztak and Neafsey, 1994; Radley et al., 2006a; Tavares et al., 2009). A similar opposite role was reported for behavioral responses to stress (Vidal-Gonzalez et al., 2006). Taken together, these results indicated a region-specific regulation of responses to stress within the mPFC. Despite these fragments of evidence, the neurochemical mechanisms related to the control of stress responses by the PL and IL are poorly understood.
Noradrenergic neurotransmission is a prominent brain neurochemical mechanism involved in the regulation of stress responses (Morilak et al., 2005; Joëls and Baram, 2009; Wood and Valentino, 2017; Herman, 2018). In this sense, all adrenoceptors were identified within the mPFC (Wang et al., 2007; Ji et al., 2008; Liu et al., 2014; Santana and Artigas, 2017). Besides, the previous studies documented that the noradrenergic terminals within the mPFC arise mainly from the locus coeruleus (LC) (Sara, 2009; Chandler et al., 2013), and stress activates the LC neurons projecting to the mPFC (Borodovitsyna et al., 2020). Accordingly, aversive stimuli increased the local extracellular levels of noradrenaline within the mPFC (Nakane et al., 1994; Finlay et al., 1995; Kawahara et al., 1999; Gresch et al., 2002). The idea that the noradrenergic neurotransmission of the mPFC is a part of the central network in regulating stress responses is further supported by evidence that the lesion of catecholaminergic terminals in the mPFC inhibited the neuronal activation in other limbic structures induced by stressful stimuli (Spencer and Day, 2004). The catecholaminergic lesion within the mPFC also inhibited the activation of the HPA axis induced by restraint stress (Radley et al., 2008). Despite these fragments of evidence, the role of the noradrenergic neurotransmission of mPFC in regulating the cardiovascular responses to aversive stimuli has never been reported. Hence, this study aimed to evaluate the specific role of adrenoceptors present within the PL and IL subregions of the mPFC in cardiovascular reactivity to an acute session of restraint stress in male rats.
Materials and Methods
Animals
Male Wistar rats were supplied by the breeding facility of the São Paulo State University (UNESP; Botucatu, São Paulo, Brazil) and were housed in collective plastic cages (e.g., four animals/cage) in a temperature-controlled room at 24°C in the Animal Facility of the Laboratory of Pharmacology, School of Pharmaceutical Sciences, UNESP. They were kept under a 12:12-h light–dark cycle (lights on between 7:00 a.m. and 7:00 p.m.) with free access to water and standard rat chow. Housing conditions and experimental procedures were approved by the local Ethical Committee for Use of Animals (School of Pharmaceutical Sciences, UNESP), which complies with the Brazilian and international guidelines for animal use and welfare.
Drugs and Solutions
WB4101 (selective α1-adrenoceptor antagonist; Tocris, Westwoods Business Park, Ellisville, MO, USA; cat. # 0946), RX821002 (selective α2-adrenoceptor antagonist; Tocris, cat. # 1324), propranolol (β-adrenoceptor antagonist; Sigma–Aldrich, St. Louis, Missouri, USA; cat. # P0884), tribromoethanol (Sigma–Aldrich, cat. # T48402), and urethane (Sigma–Aldrich; cat. # U2500) were dissolved in saline (NaCl 0.9%). Flunixin meglumine (Banamine, Schering Plough, Cotia, São Paulo, Brazil) and the polyantibiotic preparation of streptomycins and penicillins (Pentabiotico, Fort Dodge, Campinas, São Paulo, Brazil) were used as provided.
Surgical Preparation
Rats underwent stereotaxic surgery at least 1 week after they arrived in the Animal Facility of the Laboratory of Pharmacology, UNESP. For this, animals were anesthetized with tribromoethanol [250 mg/kg, intraperitoneal (i.p.)], the scalp was anesthetized with 2% lidocaine, and the skull was exposed. Then, by using a stereotaxic apparatus (Stoelting, Wood Dale, IL, USA), stainless steel cannulas (i.e., 26 G, 10-mm long) directed to either the PL or IL were bilaterally implanted. The stereotaxic coordinates for the PL were as follows: anteroposterior = +3.3 mm from bregma, lateral = +1.9 mm from medial suture, and ventral = −2.4 mm from the skull. The stereotaxic coordinates for the IL were as follows: anteroposterior = +3.3 mm from bregma, lateral = +2.7 mm from medial suture, and ventral = −3.2 mm from the skull. For both structures, cannulas were implanted with an inclination of 24°. Dental cement was used to fix cannulas to the skull. After surgery, the rats were treated with a polyantibiotic preparation containing streptomycins and penicillins to prevent infection [560 mg/ml/kg, intramuscular (i.m.)] and the non-steroidal anti-inflammatory flunixin meglumine for post-operative analgesia [0.5 mg/ml/kg, subcutaneous (s.c.)].
One day before the trial, rats were again anesthetized with tribromoethanol (250 mg/kg, i.p.), and a polyethylene cannula (a 4-cm segment of PE-10 bound to a 13-cm segment of PE-50) (Clay Adams, Parsippany, NJ, USA) was implanted into the abdominal aorta through the femoral artery for cardiovascular recording. The catheter was tunneled under the skin and exteriorized on the dorsum of the animal. After the surgery, the non-steroidal anti-inflammatory flunixin meglumine was administered for post-operative analgesia (0.5 mg/ml/kg, s.c.). The animals were kept in individual cages during the post-operative period and the cardiovascular recording.
Blood Pressure and HR Recording
The cannula inserted into the femoral artery was connected to a pressure transducer (DPT100, Utah Medical Products Inc., Midvale, UT, USA). The pulsatile arterial pressure (PAP) was recorded using an amplifier (Bridge Amp, ML224, ADInstruments, Australia) and an acquisition board (PowerLab 4/30, ML866/P, ADInstruments, Australia) that is connected to a personal computer. Mean arterial pressure (MAP) and HR values were obtained from the PAP recording.
Tail Skin Temperature Recording
The tail skin temperature was recorded using an IR digital thermographic camera (IRI4010, InfraRed Integrated Systems Ltd., Northampton, UK). The thermographic analysis was performed using a software, and the temperature was represented by color intensity variations (Vianna and Carrive, 2005; Busnardo et al., 2019). For image analysis, the temperature was measured at five points along the tail of the animal, and the mean was calculated for each recording (Oliveira et al., 2015, 2021).
Restraint Stress
Restraint is a commonly used stressor to investigate the behavioral and physiological responses evoked by aversive stimuli in laboratory animals (Buynitsky and Mostofsky, 2009; Campos et al., 2013; Bali and Jaggi, 2015). In this study, restraint stress consisted of introducing the animals into plastic cylindrical tubes (diameter = 6.5 cm and length = 15 cm), which were ventilated by half-inch holes that comprised ~20% of the tube. The animals were maintained for 30 min into the restraint tube (Gouveia et al., 2016; Barretto-de-Souza et al., 2018b). Each animal was submitted to a single session of stress to avoid habituation (Benini et al., 2019, 2020; Santos et al., 2020).
Drug Microinjection
The needles (33 G, Small Parts, Miami Lakes, FL, USA) used for microinjection into the PL and IL were 1 mm longer than the guide cannulas and were connected to a 2-μL syringe (7002-KH, Hamilton Co., Reno, NV, USA) through a PE-10 tubing (Clay Adams, Parsippany, NJ, USA). The intracerebral microinjections were performed within a 5-s period, and the needle was left in the guide cannula for 1 min after the microinjection before being removed. The microinjection was performed without restraining the animals, and the drugs were administrated in a final volume of 100 nl/side (Tavares et al., 2009; Fassini et al., 2016; Brasil et al., 2018).
Histological Determination of Microinjection Sites
At the end of each experiment, animals were anesthetized with urethane (250 mg/ml per 200-g body weight, i.p.), and 1% Evans blue dye was microinjected into the brain at the same volume of drug injection (i.e., 100 nl/side) as a marker of the microinjection site. Then, the brains were removed and post-fixed in 10% formalin solution for at least 48 h at 4°C. Later, the 40-μm-thick serial sections of the mPFC region were cut using a cryostat (CM1900, Leica, Wetzlar, Germany). The sites of injection were analyzed from the serial sections according to the study of Paxinos and Watson (1997) using a light microscope (Zeiss Axioskop 2).
Experimental Protocol
Rats were brought to the recording room in their own cage. The recording room was temperature-controlled (24°C) and acoustically isolated. Before starting the experiment, animals were allowed at least 60 min to adapt to the room conditions, such as sound and illumination.
Each animal was connected to the cardiovascular recording system and subjected to a 30-min period of basal cardiovascular recording. Then, an independent set of rats received the bilateral microinjection into either the PL or IL of vehicle (saline, 100 nl), the selective α1-adrenoceptor antagonist WB4101 (10 nmol/100 nl), the selective α2-adrenoceptor antagonist RX821002 (10 nmol/100 nl), or the β-adrenoceptor antagonist propranolol (10 nmol/100 nl). The doses were used based on the previous studies (Fernandes et al., 2003; Resstel et al., 2005; Crestani et al., 2008). Ten minutes after the treatment, rats underwent the 30-min restraint stress session. The cardiovascular parameters (i.e., MAP and HR) were continuously recorded throughout the restraint session. The tail skin temperature was measured 10, 5, and 0 min before the restraint and at every 5 min during restraint stress.
Data Analysis
The data were expressed as mean ± SEM. The basal values (i.e., pre-stress values) of MAP, HR, and tail skin temperature after PL or IL treatment were compared using the one-way ANOVA followed by the Bonferroni post-hoc test. The effects of restraint stress on the cardiovascular parameters were presented as the changes from the pre-stress values. The restraint-evoked changes were obtained by subtracting the measurements recorded during stress to the mean of values recorded for 10 min before restraint onset. The two-way ANOVA, with treatment as the main factor and time as the repeated measure, was used for the analysis of the time-course curves of the restraint-evoked changes in MAP, HR, and tail skin temperature. The results of the statistical tests with P < 0.05 were considered statistically significant.
Results
The diagrammatic representations showing the bilateral sites of microinjection into the PL and IL of all animals that were used in this study are presented in Figure 1.
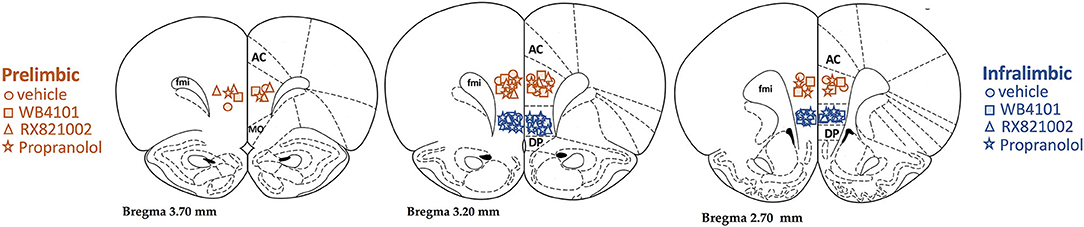
Figure 1. Diagrammatic representation based on the rat brain atlas of Paxinos and Watson (1997) indicating prelimbic (PL; orange symbols) and infralimbic (IL; blue symbols) microinjection sites of the selective α1-adrenoceptor antagonist WB4101 (squares), the selective α2-adrenoceptor antagonist RX821002 (triangles), the β-adrenoceptor antagonist propranolol (stars), and vehicle (circles). AC, anterior cingulate; DP, dorsal peduncular; fmi, forceps minor of the corpus callosum.
Effect of Pharmacological Treatment of PL With Adrenoceptor Antagonists in Cardiovascular and Tail Skin Temperature Changes to Acute Restraint Stress
α1-adrenoceptor: The bilateral microinjection of the selective α1-adrenoceptor antagonist WB4101 (10 nmol/100 nl, n = 6) into the PL did not affect the basal values of either MAP, HR, or tail skin temperature (Table 1). Restraint stress increased MAP [F(20,220) = 16.6, P < 0.0001] and HR [F(20,220) = 9.72, P < 0.0001] and decreased the tail skin temperature [F(8,88) = 15.76, P < 0.0001] (Figure 2). Furthermore, the microinjection of WB4101 into the PL reduced the restraint-evoked tachycardia [F(1,11) = 5.52, P = 0.0406] and the drop in the tail skin temperature [F(1,11) = 4.89, P = 0.0478] but without affecting the MAP response [F(1,11) = 2.77, P = 0.1242] (Figure 2). The analysis also indicated a treatment–time interaction for MAP [F(20,220) = 2.58, P = 0.0004] and HR [F(20,220) = 2.35, P = 0.0015] but not for the tail skin temperature [F(8,88) = 2.01, P = 0.0547] values.
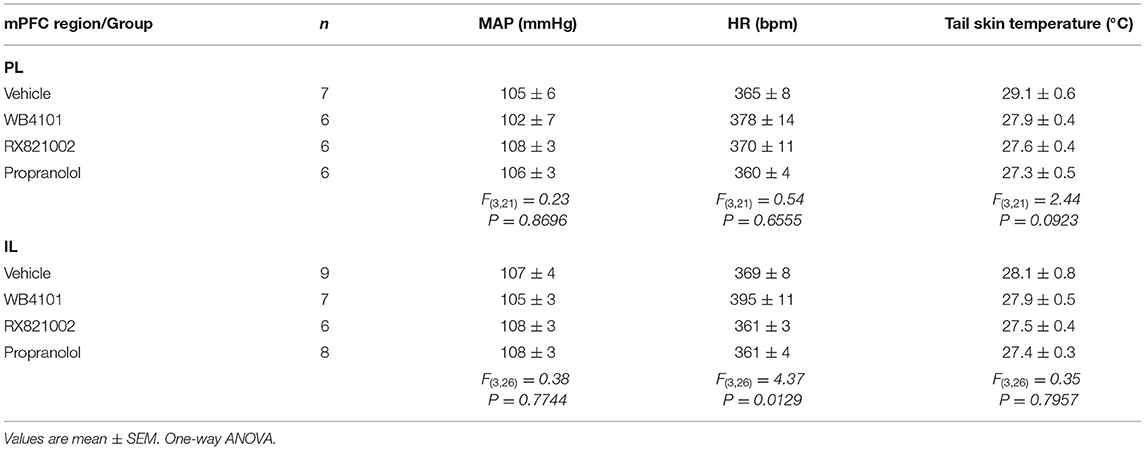
Table 1. Basal parameters (i.e., pre-stress values) of the mean arterial pressure (MAP), heart rate (HR), and the tail skin temperature after pharmacological treatment of either the prelimbic (PL) or infralimbic (IL) cortex with the selective α1-adrenoceptor antagonist WB4101 (10 nmol/100 nl), the selective α2-adrenoceptor antagonist RX821002 (10 nmol/100 nl), the β-adrenoceptor antagonist propranolol (10 nmol/100 nl), or vehicle (10 nmol/100 nl).
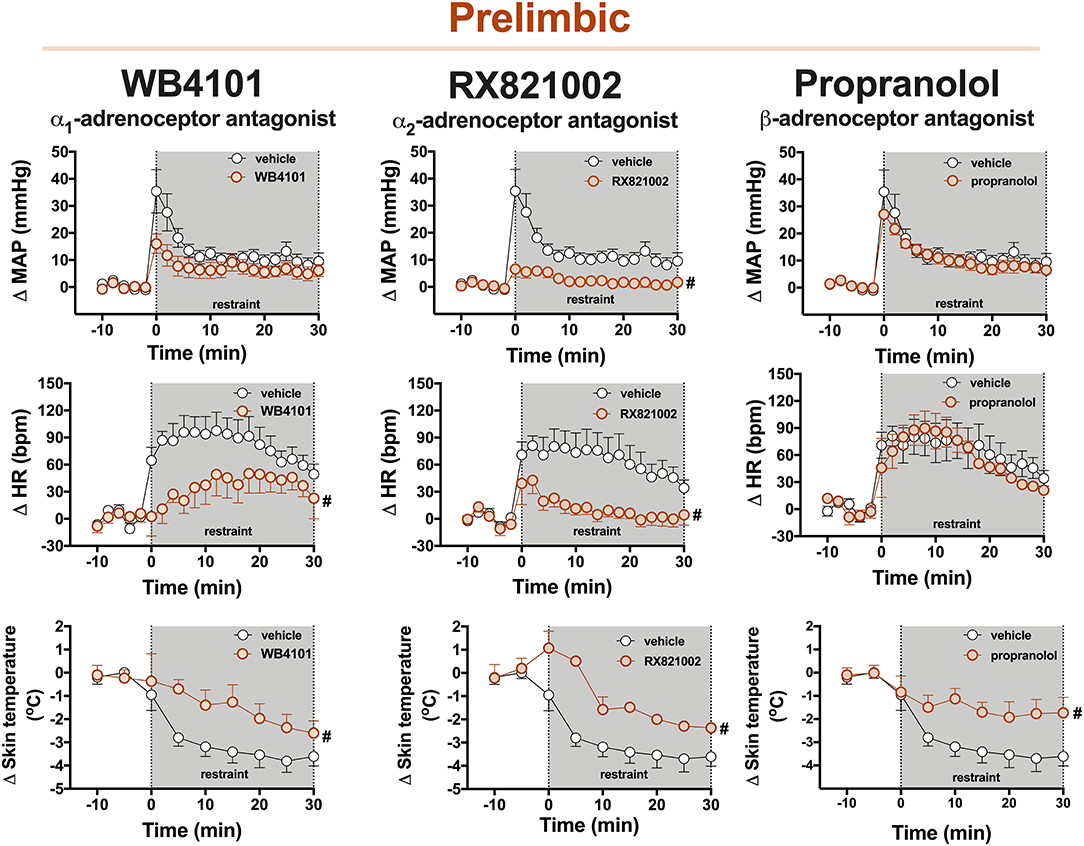
Figure 2. Time-course curves of the mean arterial pressure (ΔMAP), heart rate (ΔHR), and tail skin temperature (Δskin temperature) changes evoked by an acute session of restraint stress in animals treated with the selective α1-adrenoceptor antagonist WB4101 (10 nmol/100 nl, n = 6), the selective α2-adrenoceptor antagonist RX821002 (10 nmol/100 nl, n = 6), the β-adrenoceptor antagonist propranolol (10 nmol/100 nl, n = 6), or vehicle (100 nl, n = 7) into the PL cortex. Circles represent the mean ± SEM. #P < 0.05 over the entire restraint stress period compared with the control group (vehicle), two-way ANOVA.
α2-adrenoceptor: The bilateral microinjection of the selective α2-adrenoceptor antagonist RX821002 (10 nmol/100 nl, n = 6) into the PL did not affect the basal parameters of either MAP, HR, or tail skin temperature (Table 1). Restraint stress increased MAP [F(20,220) = 12.86, P < 0.0001] and HR [F(20,220) = 7.12, P < 0.0001] and decreased the tail skin temperature [F(8,88) = 24.17, P < 0.0001] (Figure 2). The microinjection of RX821002 into the PL decreased the pressor [F(1,11) = 11.93, P = 0.0054] and tachycardiac [F(1,11) = 9.89, P = 0.0093] responses and the drop in the tail skin temperature [F(1,11) = 16.68, P = 0.0018] evoked by restraint stress (Figure 2). The analysis also identified a treatment–time interaction for MAP [F(20,220) = 5.89, P < 0.0001], HR [F(20,220) = 2.72, P = 0.0002], and the tail skin temperature [F(8,88) = 3.32, P = 0.0023].
β-adrenoceptor: The bilateral microinjection of the PL with the β-adrenoceptor antagonist propranolol (10 nmol/100 nl, n = 6) did not affect the baseline values of either MAP, HR, or tail skin temperature (Table 1). Restraint stress increased MAP [F(20,220) = 24.63, P < 0.0001] and HR [F(20,220) = 12.90, P < 0.0001] and decreased the tail skin temperature [F(8,88) = 18.90, P < 0.0001] (Figure 2). Treatment of PL with propranolol decreased the drop in the tail skin temperature evoked by restraint [F(1,11) = 5.30, P = 0.0420] but without affecting the pressor [F(1,11) = 0.36, P = 0.5595] and tachycardiac [F(1,11) = 0.12, P = 0.7293] responses (Figure 2). The analysis also indicated a treatment–time interaction for skin temperature [F(8,88) = 2.86, P = 0.0072] but not for MAP [F(20,220) = 0.66, P = 0.8694] and HR [F(20,220) = 0.54, P = 0.9458] measurements.
Effect of Pharmacological Treatment of IL With Adrenoceptor Antagonists in Cardiovascular and Tail Skin Temperature Changes to Acute Restraint Stress
α1-adrenoceptor: The bilateral microinjection of the selective α1-adrenoceptor antagonist WB4101 (10 nmol/100 nl, n = 7) into the IL did not affect the baseline parameters of either MAP or tail skin temperature (Table 1). The one-way ANOVA indicated a significant effect of pharmacological treatments of IL for the HR values, but the post-hoc analysis did not reveal the differences between WB4101 and vehicle groups (Table 1). Restraint stress increased MAP [F(20,280) = 19.68, P < 0.0001] and HR [F(20,280) = 8.60, P < 0.0001] and reduced the tail skin temperature [F(8,112) = 24.39, P < 0.0001] (Figure 3). The microinjection of WB4101 into the IL decreased the tachycardiac response evoked by restraint [F(1,14) = 4.84, P = 0.0466] but without affecting the increase in MAP [F(1,14) = 0.01, P = 0.9391] and the drop in the tail skin temperature [F(1,14) = 0.03, P = 0.8533] (Figure 3). The analysis also indicated a treatment–time interaction for the HR values [F(20,280) = 2.08, P = 0.0052] but not for MAP [F(20,280) = 1.28, P = 0.1978] and the tail skin temperature [F(8,112) = 0.53, P = 0.8270] measurements.
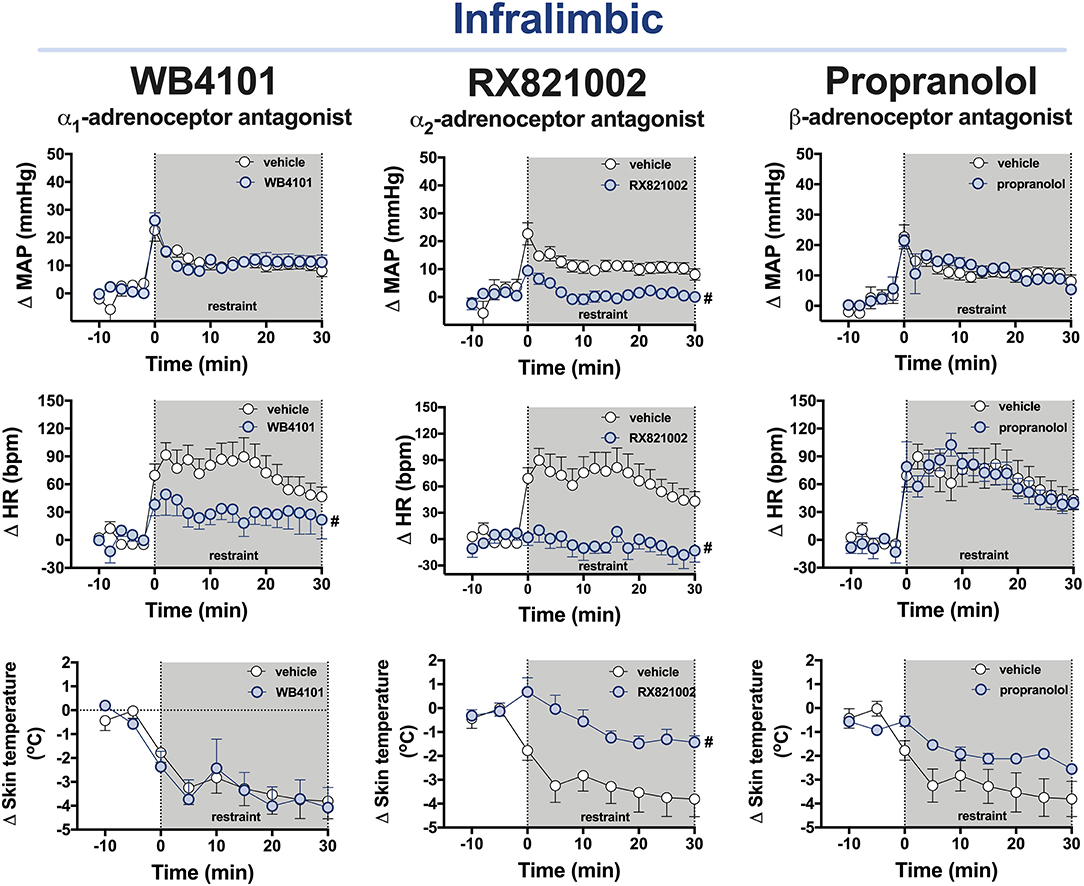
Figure 3. Time-course curves of the mean arterial pressure (ΔMAP), heart rate (ΔHR), and tail skin temperature (Δskin temperature) changes evoked by an acute session of restraint stress in animals treated with the selective α1-adrenoceptor antagonist WB4101 (10 nmol/100 nl, n = 7), the selective α2-adrenoceptor antagonist RX821002 (10 nmol/100 nl, n = 6), the β-adrenoceptor antagonist propranolol (10 nmol/100 nl, n = 8), or vehicle (100 nl, n = 9) into the PL cortex. Circles represent the mean ± SEM. #P < 0.05 over the entire restraint stress period compared with the control group (vehicle), two-way ANOVA.
α2-adrenoceptor: The bilateral microinjection of the selective α2-adrenoceptor antagonist RX821002 (10 nmol/100 nl, n = 6) into the IL did not affect the basal values of either MAP or tail skin temperature (Table 1). The one-way ANOVA indicated a significant effect of pharmacological treatments of IL for the HR values, but the post-hoc analysis did not reveal the differences between RX821002 and vehicle groups (Table 1). Restraint enhanced MAP [F(20,260) = 8.47, P < 0.0001] and HR [F(20,260) = 4.08, P < 0.0001] and decreased the tail skin temperature [F(8,104) = 13.15, P < 0.0001] (Figure 3). Treatment of the IL with RX821002 reduced the pressor [F(1,13) = 19.14, P = 0.0008] and tachycardiac [F(1,13) = 14.94, P = 0.0020] responses and the drop in the tail skin temperature [F(1,13) = 7.52, P = 0.0168] (Figure 3). The analysis also indicated a treatment–time interaction for MAP [F(20,260) = 3.27, P < 0.0001] and HR [F(20,260) = 4.32, P < 0.0001] but not for the tail skin temperature [F(8,104) = 4.03, P = 0.0003].
β-adrenoceptor: The bilateral microinjection of the β-adrenoceptor antagonist (10 nmol/100 nl, n = 8) into the IL did not affect the basal values of either MAP or the tail skin temperature (Table 1). The one-way ANOVA indicated a significant effect of pharmacological treatments of IL for the HR values, but the post-hoc analysis did not reveal the differences between propranolol and vehicle groups (Table 1). Restraint stress increased MAP [F(20,300) = 17.61, P < 0.0001] and HR [F(20,300) = 19.50, P < 0.0001] and decreased the tail skin temperature [F(8,120) = 17.84, P < 0.0001] (Figure 3). The administration of propranolol into the IL did not affect the restraint-evoked pressor [F(1,15) = 0.06, P = 0.8193] and tachycardiac [F(1,15) = 0.04, P = 0.8441] responses and the drop in the tail skin temperature [F(1,15) = 2.49, P = 0.1364] (Figure 3). The analysis indicated a treatment–time interaction for the values of tail skin temperature [F(8,120) = 3.3, P = 0.0017] but not for MAP [F(20,300) = 0.77, P = 0.7581] and HR [F(20,300) = 0.88, P = 0.6156].
Discussion
Our results provide evidence that the noradrenergic neurotransmission in the mPFC is involved in cardiovascular adjustments during aversive threats. Despite some specific differences in the adrenoceptors involved, the data reported in this study indicate a similar facilitatory influence of the noradrenergic neurotransmissions within the PL and IL subregions of the mPFC in the restraint-evoked cardiovascular changes. Figure 4 summarizes the specific role of each adrenoceptor in the PL and IL in regulating the arterial pressure, HR, and tail skin temperature responses to acute restraint stress.
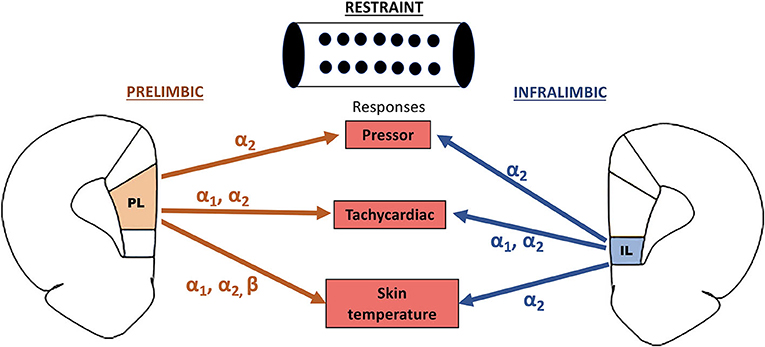
Figure 4. Summary of the facilitatory control of restraint-evoked pressor, tachycardiac, and skin temperature responses by α1-, α2-, and β-adrenoceptors in the PL and IL subregions of the medial prefrontal cortex.
Tavares et al. (2009) reported that the non-selective synaptic inhibition caused by the local microinjection of CoCl2 into the PL enhanced the restraint-evoked tachycardia, whereas the decreased HR response was observed in animals subjected to the same treatment in the IL. These results indicated opposite roles of PL (inhibitory) and IL (facilitatory) in cardiac responses to restraint stress (Tavares et al., 2009). In this sense, the results reported in this study provide the first evidence that the local noradrenergic neurotransmission is involved in the facilitatory influence of the IL in restraint-evoked tachycardia. The idea that the noradrenergic neurotransmission is involved in the IL control of stress responses is supported by the previous evidence that the lesion of catecholaminergic terminals within the mPFC, including the IL region, decreased the local neuronal activation caused by stress (Spencer and Day, 2004). Nevertheless, a previous study identified that IL treatment with the excitatory amino acid, i.e., N-methyl-d-aspartate, into the IL decreased the cardiovascular responses to air-jet stress (Müller-Ribeiro et al., 2012). These results were surprising once the activation of an excitatory receptor mimicked the changes observed following the local non-selective synaptic blockade within the IL (Tavares et al., 2009). Therefore, further studies evaluating the effect of IL treatment with glutamate receptor antagonists are necessary for a full characterization of the role of glutamatergic neurotransmission in the IL control of the cardiovascular responses to stress.
As identified in this study, IL treatment with opioid receptor antagonists also decreased the blood pressure and HR responses to restraint stress (Fassini et al., 2015), thus suggesting that the noradrenergic neurotransmission might act in modulating the action of other local neurochemical mechanisms to control the cardiovascular responses during aversive threats. Although a direct interaction between noradrenergic and opioid neurotransmissions has never been reported in the mPFC, this idea is supported by evidence that α1- and α2-adrenoceptors presynaptically modulate the local release of glutamate and acetylcholine within the mPFC, respectively (Tellez et al., 1999; Luo et al., 2014). Besides, the expression of α1-adrenoceptor was identified in GABAergic interneurons within the IL (Santana et al., 2013). However, we cannot exclude the possibility of the direct action in pyramidal neurons, once α1- and α2-adrenoceptors were identified in these neurons and their activation increases the excitability of IL pyramidal neurons (Carr et al., 2007; Luo et al., 2014).
We identified that PL treatment with the adrenoceptor antagonists caused opposite effects in relation to that reported previously in animals treated with the non-selective synaptic blocker CoCl2 (Tavares et al., 2009). Similar findings were reported in relation to the control of the HPA axis response by the PL. In fact, the non-selective inhibition of the PL evoked by the local ibotenic acid lesion enhanced the restraint-evoked activation of the HPA axis (Radley et al., 2006b), whereas the ablation of noradrenergic terminals centered on the PL attenuated this neuroendocrine response (Radley et al., 2008). The attenuation of the HPA axis response to restraint evoked by the lesion of noradrenergic terminals was followed by a marked increase in the restraint-evoked activation of neurons within the PL (Radley et al., 2008). Therefore, the control of stress responses by the noradrenergic neurotransmission within the PL seems to be mediated by an inhibitory influence in the activity of local neurons. In this sense, the results reported in this study provide new evidence that this inhibitory mechanism of the noradrenergic neurotransmission within the PL is involved in the modulation of cardiovascular responses in addition to the activation of the HPA axis during aversive threats. This idea is further supported by a previous report that the microinjection of a GABAA receptor antagonist within the PL decreased the restraint-evoked pressor and tachycardiac responses (Fassini et al., 2016). Therefore, the results reported in this study indicate similar roles of the noradrenergic neurotransmission (i.e., facilitatory) in the PL and IL in control of the cardiovascular responses to stress.
Evidence that α1-, α2-, and β-adrenoceptors acting post-synaptically increase the excitability of pyramidal neurons in the mPFC precludes the idea that the inhibition of pyramidal neurons by the local noradrenergic neurotransmission during aversive threats is mediated by the direct action in these cells (Carr et al., 2007; Mueller et al., 2008; Luo et al., 2014). Therefore, the control of stress responses by the noradrenergic neurotransmission in the PL seems to occur primarily via the modulation of other local neurochemical mechanisms. In this sense, it was reported a facilitatory influence of local GABAergic, angiotensinergic, and opioid neurotransmissions in the restraint-evoked cardiovascular responses (Fassini et al., 2014, 2016; Brasil et al., 2018). The control by α2-adrenoceptors might also be mediated by autoreceptors inhibiting the local noradrenaline release (Dwyer et al., 2010; Devoto et al., 2015).
Both PL and IL are connected with other limbic structures involved in the cardiovascular control during aversive threats (Hurley et al., 1991; Vertes, 2004; Myers et al., 2014; Wood et al., 2019). In this sense, a previous study identified that the lesion of catecholaminergic inputs within the mPFC decreased the stress-induced neuronal activation in the bed nucleus of the stria terminalis (BNST) (Spencer and Day, 2004). The BNST is a limbic structure that has been implicated in physiological and behavioral responses to stress (Crestani et al., 2013; Gouveia et al., 2016; Gomes-de-Souza et al., 2021). Glutamatergic pyramidal neurons of the mPFC project to the BNST (Hurley et al., 1991; Vertes, 2004; Wood et al., 2019), and recent studies indicate a facilitatory influence of glutamatergic neurotransmission within the BNST in the cardiovascular responses to restraint stress (Adami et al., 2017; Barretto-de-Souza et al., 2018a). Therefore, the facilitatory influence of the IL noradrenergic neurotransmission is possibly mediated by the activation of pyramidal neurons projecting to the BNST. Accordingly, the previous studies have reported that the IL is the main mPFC subregion projecting to the BNST (Vertes, 2004; Massi et al., 2008).
Although evidence that PL also innervates the BNST (Radley et al., 2009; Myers, 2017), the aim that the facilitatory influence of the PL noradrenergic neurotransmission in the restraint-evoked cardiovascular changes is mediated by the inhibition of local pyramidal neurons (refer to the earlier discussions) precludes the idea of an involvement of glutamatergic neurotransmission within the BNST. In this sense, the PL densely innervates other structures involved in stress responses, such as midbrain raphe nuclei (i.e., dorsal and median) and central (CeA) and basolateral (BLA) nuclei of the amygdala (Vertes, 2004; Ulrich-Lai and Herman, 2009). The previous studies indicated that the lesion of the CeA of borderline hypertensive rats reduced the increase in arterial pressure evoked by the intermittent foot shock (Sanders et al., 1994). Regarding the BLA, in addition to a role of local angiotensin-converting enzyme 2 (ACE2)/Ang-(1–7)/Mas receptor axis pathway (Oscar et al., 2015; Silva et al., 2020), the previous studies using the chemical blockade with muscimol in non-human primates and rodents have indicated a facilitatory role of this amygdaloid nucleus in the cardiovascular responses to stress (Salomé et al., 2007; Elorette et al., 2020). As for the projections to the BNST, the results indicating a facilitatory role of the CeA and BLA in the cardiovascular responses to stress do not support the hypothesis of an involvement of these amygdaloid nuclei in the control of the cardiovascular responses to stress by the PL noradrenergic neurotransmission. Regarding the raphe nuclei, although evidence of a facilitatory influence of medullary raphe nuclei in the stress-evoked cardiovascular responses (Nalivaiko et al., 2005; Vianna et al., 2008; Pham-Le et al., 2011; Ikoma et al., 2018), to the best of our knowledge, the role of dorsal and median raphe nuclei in the cardiovascular changes has never been reported. Therefore, further studies will be necessary for a discussion of the possible neural circuitry related to the control of the cardiovascular responses to stress by the PL noradrenergic neurotransmission.
Conclusions
Our data indicate that the noradrenergic neurotransmission within the mPFC is a part of the central network in regulating the cardiovascular responses during aversive threats. Despite some differences in the specific adrenoceptor in the PL and IL in controlling each response evaluated in this study (Figure 4), the results reported in this study indicate that the noradrenergic neurotransmission in both mPFC subregions plays a facilitatory role in cardiovascular and autonomic responses during restraint stress.
Data Availability Statement
The original contributions presented in the study are included in the article/supplementary material, further inquiries can be directed to the corresponding author/s.
Ethics Statement
The animal study was reviewed and approved by Ethical Committee for Use of Animals (School of Pharmaceutical Sciences/UNESP).
Author Contributions
LO: conceptualization, methodology, writing the original draft preparation, and visualization. TP and ER: conceptualization, methodology, formal analysis, investigation, and writing the review and editing. JD and CX: methodology, formal analysis, investigation, and writing the review and editing. CC: conceptualization, methodology, resources, data curation, writing the review and editing, visualization, supervision, project administration, and funding acquisition. All authors contributed to the article and approved the submitted version.
Funding
This study was supported by grants from the São Paulo Research Foundation (FAPESP) (Grant No. 2012/14376-0 and 2019/24478-3), the National Council for Scientific and Technological Development (CNPq) (Grant No. 456405/2014-3 and 431339/2018-0), and the Scientific Support and Development Program of School of Pharmaceutical Sciences (UNESP). CC is a CNPq research fellow (Process No. 304108/2018-9).
Conflict of Interest
The authors declare that the research was conducted in the absence of any commercial or financial relationships that could be construed as a potential conflict of interest.
Publisher's Note
All claims expressed in this article are solely those of the authors and do not necessarily represent those of their affiliated organizations, or those of the publisher, the editors and the reviewers. Any product that may be evaluated in this article, or claim that may be made by its manufacturer, is not guaranteed or endorsed by the publisher.
Acknowledgments
We would like to acknowledge Rosana Silva and Elisabete Lepera for technical assistance.
References
Adami, M. B., Barretto-De-Souza, L., Duarte, J. O., Almeida, J., and Crestani, C. C. (2017). Both N-methyl-D-aspartate and non-N-methyl-D-aspartate glutamate receptors in the bed nucleus of the stria terminalis modulate the cardiovascular responses to acute restraint stress in rats. J. Psychopharmacol. 31, 674–681. doi: 10.1177/0269881117691468
Bali, A., and Jaggi, A. S. (2015). Preclinical experimental stress studies: protocols, assessment and comparison. Eur. J. Pharmacol. 746, 282–292. doi: 10.1016/j.ejphar.2014.10.017
Barretto-de-Souza, L., Adami, M. B., Benini, R., and Crestani, C. C. (2018a). Dual role of nitrergic neurotransmission in the bed nucleus of the stria terminalis in controlling cardiovascular responses to emotional stress in rats. Br. J. Pharmacol. 175, 3773–3783. doi: 10.1111/bph.14447
Barretto-de-Souza, L., Adami, M. B., Oliveira, L. A., Gomes-de-Souza, L., Duarte, J. O., Almeida, J., et al. (2018b). Nitric oxide-cGMP-PKG signaling in the bed nucleus of the stria terminalis modulates the cardiovascular responses to stress in male rats. Eur. Neuropsychopharmacol. 28, 75–84. doi: 10.1016/j.euroneuro.2017.11.015
Benini, R., Oliveira, L. A., Gomes-de-Souza, L., and Crestani, C. C. (2019). Habituation of the cardiovascular responses to restraint stress in male rats: influence of length, frequency and number of aversive sessions. Stress 22, 151–161. doi: 10.1080/10253890.2018.1532992
Benini, R., Oliveira, L. A., Gomes-de-Souza, L., Rodrigues, B., and Crestani, C. C. (2020). Habituation of the cardiovascular responses to restraint stress is inhibited by exposure to other stressor stimuli and exercise training. J. Exp. Biol. 2020:jeb.219501. doi: 10.1242/jeb.219501
Borodovitsyna, O., Duffy, B. C., Pickering, A. E., and Chandler, D. J. (2020). Anatomically and functionally distinct locus coeruleus efferents mediate opposing effects on anxiety-like behavior. Neurobiol. Stress 13:100284. doi: 10.1016/j.ynstr.2020.100284
Brasil, T. F. S., Fassini, A., and Corrêa, F. M. (2018). AT1 and AT2 receptors in the prelimbic cortex modulate the cardiovascular response evoked by acute exposure to restraint stress in rats. Cell. Mol. Neurobiol. 518:9. doi: 10.1007/s10571-017-0518-9
Busnardo, C., Crestani, C. C., Scopinho, A. A., Packard, B. A., Resstel, L. B. M., Correa, F. M. A., et al. (2019). Nitrergic neurotransmission in the paraventricular nucleus of the hypothalamus modulates autonomic, neuroendocrine and behavioral responses to acute restraint stress in rats. Prog. Neuro-Psychopharmacology Biol. Psychiatry 90, 16–27. doi: 10.1016/j.pnpbp.2018.11.001
Buynitsky, T., and Mostofsky, D. I. (2009). Restraint stress in biobehavioral research: recent developments. Neurosci. Biobehav. Rev. 33, 1089–1098. doi: 10.1016/j.neubiorev.2009.05.004
Campos, A. C., Fogaça, M. V., Aguiar, D. C., and Guimarães, F. S. (2013). Animal models of anxiety disorders and stress. Rev. Bras. Psiquiatr. 35, S101–S111. doi: 10.1590/1516-4446-2013-1139
Carr, D. B., Andrews, G. D., Glen, W. B., and Lavin, A. (2007). α 2 -Noradrenergic receptors activation enhances excitability and synaptic integration in rat prefrontal cortex pyramidal neurons via inhibition of HCN currents. J. Physiol. 584, 437–450. doi: 10.1113/jphysiol.2007.141671
Chandler, D. J., Lamperski, C. S., and Waterhouse, B. D. (2013). Identification and distribution of projections from monoaminergic and cholinergic nuclei to functionally differentiated subregions of prefrontal cortex. Brain Res. 1522, 38–58. doi: 10.1016/j.brainres.2013.04.057
Crestani, C., Alves, F., Gomes, F., Resstel, L., Correa, F., and Herman, J. (2013). Mechanisms in the bed nucleus of the stria terminalis involved in control of autonomic and neuroendocrine functions: a review. Curr. Neuropharmacol. 11, 141–159. doi: 10.2174/1570159X11311020002
Crestani, C. C. (2016). Emotional stress and cardiovascular complications in animal models: a review of the influence of stress type. Front. Physiol. 7:251. doi: 10.3389/fphys.2016.00251
Crestani, C. C., Alves, F. H. F., Resstel, L. B., and Corrêa, F. M. A. (2008). Both α 1 and α 2-adrenoceptors mediate the cardiovascular responses to noradrenaline microinjected into the bed nucleus of the stria terminal of rats. Br. J. Pharmacol. 153, 583–590. doi: 10.1038/sj.bjp.0707591
Cullinan, W. E., Herman, J. P., Battaglia, D. F., Akil, H., and Watson, S. J. (1995). Pattern and time course of immediate early gene expression in rat brain following acute stress. Neuroscience 64, 477–505. doi: 10.1016/0306-4522(94)00355-9
Dampney, R. A. L. (2015). Central mechanisms regulating coordinated cardiovascular and respiratory function during stress and arousal. Am. J. Physiol. Regul. Integr. Comp. Physiol. 309, R429–R443. doi: 10.1152/ajpregu.00051.2015
Devoto, P., Flore, G., Saba, P., Frau, R., and Gessa, G. L. (2015). Selective inhibition of dopamine-beta-hydroxylase enhances dopamine release from noradrenergic terminals in the medial prefrontal cortex. Brain Behav. 5:393. doi: 10.1002/brb3.393
Dwyer, J. M., Platt, B. J., Sukoff Rizzo, S. J., Pulicicchio, C. M., Wantuch, C., Zhang, M. Y., et al. (2010). Preclinical characterization of BRL 44408: antidepressant- and analgesic-like activity through selective α2A-adrenoceptor antagonism. Int. J. Neuropsychopharmacol. 13, 1193–1205. doi: 10.1017/S1461145709991088
Elorette, C., Aguilar, B. L., Novak, V., Forcelli, P. A., and Malkova, L. (2020). Dysregulation of behavioral and autonomic responses to emotional and social stimuli following bidirectional pharmacological manipulation of the basolateral amygdala in macaques. Neuropharmacology 179:108275. doi: 10.1016/j.neuropharm.2020.108275
Fassini, A., Resstel, L. B. M., and Corrêa, F. M. A. (2016). Prelimbic cortex GABA A receptors are involved in the mediation of restraint stress-evoked cardiovascular responses. Stress 19, 576–584. doi: 10.1080/10253890.2016.1231177
Fassini, A., Scopinho, A. A., Resstel, L. B. M., and Correa, F. M. A. (2014). Opioid receptors in the prelimbic cortex modulate restraint stress-induced cardiovascular responses in the rat. Neuropharmacology 85, 367–374. doi: 10.1016/j.neuropharm.2014.04.019
Fassini, A., Scopinho, A. A., Resstel, L. B. M., and Corrêa, F. M. A. (2015). κ-Opioid receptors in the infralimbic cortex modulate the cardiovascular responses to acute stress. Exp. Physiol. 100, 377–387. doi: 10.1113/expphysiol.2014.084020
Fernandes, K. B. P., Crippa, G. E., Tavares, R. F., Antunes-Rodrigues, J., and Corrêa, F. M. A. (2003). Mechanisms involved in the pressor response to noradrenaline injection into the cingulate cortex of unanesthetized rats. Neuropharmacology 44, 757–763. doi: 10.1016/S0028-3908(03)00067-4
Figueiredo, H. F., Dolgas, C. M., and Herman, J. P. (2002). Stress activation of cortex and hippocampus is modulated by sex and stage of estrus. Endocrinology 143, 2534–2540. doi: 10.1210/endo.143.7.8888
Finlay, J. M., Zigmond, M. J., and Abercrombie, E. D. (1995). Increased dopamine and norepinephrine release in medial prefrontal cortex induced by acute and chronic stress: effects of diazepam. Neuroscience 64, 619–628. doi: 10.1016/0306-4522(94)00331-X
Frysztak, R. J., and Neafsey, E. J. (1994). The effect of medial frontal cortex lesions on cardiovascular conditioned emotional responses in the rat. Brain Res. 643, 181–193. doi: 10.1016/0006-8993(94)90024-8
Gomes-de-Souza, L., Bianchi, P. C., Costa-Ferreira, W., Tomeo, R. A., Cruz, F. C., and Crestani, C. C. (2021). CB1 and CB2 receptors in the bed nucleus of the stria terminalis differently modulate anxiety-like behaviors in rats. Prog. Neuro Psychopharmacol. Biol. Psychiatry 110:110284. doi: 10.1016/j.pnpbp.2021.110284
Gouveia, M. K., Miguel, T. T., Busnardo, C., Scopinho, A. A., Corrêa, F. M. A., Nunes-De-Souza, R. L., et al. (2016). Dissociation in control of physiological and behavioral responses to emotional stress by cholinergic neurotransmission in the bed nucleus of the stria terminalis in rats. Neuropharmacology 101, 379–388. doi: 10.1016/j.neuropharm.2015.10.018
Gresch, P. J., Sved, A. F., Zigmond, M. J., and Finlay, J. M. (2002). Local influence of endogenous norepinephrine on extracellular dopamine in rat medial prefrontal cortex. J. Neurochem. 65, 111–116. doi: 10.1046/j.1471-4159.1995.65010111.x
Herman, J. P. (2018). Regulation of hypothalamo-pituitary-adrenocortical responses to stressors by the nucleus of the solitary tract/dorsal vagal complex. Cell. Mol. Neurobiol. 38, 25–35. doi: 10.1007/s10571-017-0543-8
Hurley, K. M., Herbert, H., Moga, M. M., and Saper, C. B. (1991). Efferent projections of the infralimbic cortex of the rat. J. Comp. Neurol. 308, 249–276. doi: 10.1002/cne.903080210
Ikoma, Y., Kusumoto-Yoshida, I., Yamanaka, A., Ootsuka, Y., and Kuwaki, T. (2018). Inactivation of serotonergic neurons in the rostral medullary raphé attenuates stress-induced tachypnea and tachycardia in mice. Front. Physiol. 9:832. doi: 10.3389/fphys.2018.00832
Ji, X. H., Cao, X. H., Zhang, C. L., Feng, Z. J., Zhang, X. H., Ma, L., et al. (2008). Pre- and postsynaptic β-adrenergic activation enhances excitatory synaptic transmission in layer V/VI pyramidal neurons of the medial prefrontal cortex of rats. Cereb. Cortex 18, 1506–1520. doi: 10.1093/cercor/bhm177
Joëls, M., and Baram, T. Z. (2009). The neuro-symphony of stress. Nat. Rev. Neurosci. 10, 459–466. doi: 10.1038/nrn2632
Kawahara, Y., Kawahara, H., and Westerink, B. H. C. (1999). Comparison of effects of hypotension and handling stress on the release of noradrenaline and dopamine in the locus coeruleus and medial prefrontal cortex of the rat. Naunyn. Schmiedebergs. Arch. Pharmacol. 360, 42–49. doi: 10.1007/s002109900042
Liu, Y., Liang, X., Ren, W. W., and Li, B. M. (2014). Expression of β1- and β2-adrenoceptors in different subtypes of interneurons in the medial prefrontal cortex of mice. Neuroscience 257, 149–157. doi: 10.1016/j.neuroscience.2013.10.078
Luo, F., Tang, H., Li, B., and Li, S. (2014). Activation of α1-adrenoceptors enhances excitatory synaptic transmission via a pre- and postsynaptic protein kinase C-dependent mechanism in the medial prefrontal cortex of rats. Eur. J. Neurosci. 39, 1281–1293. doi: 10.1111/ejn.12495
Massi, L., Elezgarai, I., Puente, N., Reguero, L., Grandes, P., Manzoni, O. J., et al. (2008). Cannabinoid receptors in the bed nucleus of the stria terminalis control cortical excitation of midbrain dopamine cells in vivo. J. Neurosci. 28, 10496–10508. doi: 10.1523/JNEUROSCI.2291-08.2008
McEwen, B. S. (2017). Neurobiological and systemic effects of chronic stress. Chronic Stress 1:247054701769232. doi: 10.1177/2470547017692328
McKlveen, J. M., Moloney, R. D., Scheimann, J. R., Myers, B., and Herman, J. P. (2019). “Braking” the prefrontal cortex: the role of glucocorticoids and interneurons in stress adaptation and pathology. Biol. Psychiatry 86, 669–681. doi: 10.1016/j.biopsych.2019.04.032
McKlveen, J. M., Myers, B., and Herman, J. P. (2015). The medial prefrontal cortex: coordinator of autonomic, neuroendocrine and behavioural responses to stress. J. Neuroendocrinol. 27, 446–456. doi: 10.1111/jne.12272
Morilak, D. A., Barrera, G., Echevarria, D. J., Garcia, A. S., Hernandez, A., Ma, S., et al. (2005). Role of brain norepinephrine in the behavioral response to stress. Prog. Neuro Psychopharmacol. Biol. Psychiatry 29, 1214–1224. doi: 10.1016/j.pnpbp.2005.08.007
Mueller, D., Porter, J. T., and Quirk, G. J. (2008). Noradrenergic signaling in infralimbic cortex increases cell excitability and strengthens memory for fear extinction. J. Neurosci. 28, 369–375. doi: 10.1523/JNEUROSCI.3248-07.2008
Müller-Ribeiro, F. C., de, F., Zaretsky, D. V., Zaretskaia, M. V., Santos, R. A. S., DiMicco, J. A., et al. (2012). Contribution of infralimbic cortex in the cardiovascular response to acute stress. Am. J. Physiol. Integr. Comp. Physiol. 303, R639–R650. doi: 10.1152/ajpregu.00573.2011
Myers, B. (2017). Corticolimbic regulation of cardiovascular responses to stress. Physiol. Behav. 172, 49–59. doi: 10.1016/j.physbeh.2016.10.015
Myers, B., Mark Dolgas, C., Kasckow, J., Cullinan, W. E., and Herman, J. P. (2014). Central stress-integrative circuits: forebrain glutamatergic and GABAergic projections to the dorsomedial hypothalamus, medial preoptic area, and bed nucleus of the stria terminalis. Brain Struct. Funct. 219, 1287–1303. doi: 10.1007/s00429-013-0566-y
Nakane, H., Shimizu, N., and Hori, T. (1994). Stress-induced norepinephrine release in the rat prefrontal cortex measured by microdialysis. Am. J. Physiol. Integr. Comp. Physiol. 267, R1559–R1566. doi: 10.1152/ajpregu.1994.267.6.R1559
Nalivaiko, E., Ootsuka, Y., and Blessing, W. W. (2005). Activation of 5-HT 1A receptors in the medullary raphe reduces cardiovascular changes elicited by acute psychological and inflammatory stresses in rabbits. Am. J. Physiol. Integr. Comp. Physiol. 289, R596–R604. doi: 10.1152/ajpregu.00845.2004
Oliveira, L. A., Almeida, J., Benini, R., and Crestani, C. C. (2015). CRF 1 and CRF 2 receptors in the bed nucleus of the stria terminalis modulate the cardiovascular responses to acute restraint stress in rats. Pharmacol. Res. 95–96, 53–62. doi: 10.1016/j.phrs.2015.03.012
Oliveira, L. A., Gomes-de-Souza, L., Benini, R., Wood, S. K., and Crestani, C. C. (2021). Both CRF1 and CRF2 receptors in the bed nucleus of stria terminalis are involved in baroreflex impairment evoked by chronic stress in rats. Prog. Neuro Psychopharmacol. Biol. Psychiatry 105:110009. doi: 10.1016/j.pnpbp.2020.110009
Oscar, C. G., Müller-Ribeiro, F. C., de, F., de Castro, L. G., Martins Lima, A., Campagnole-Santos, M. J., et al. (2015). Angiotensin-(1-7) in the basolateral amygdala attenuates the cardiovascular response evoked by acute emotional stress. Brain Res. 1594, 183–189. doi: 10.1016/j.brainres.2014.11.006
Pham-Le, N. M., Cockburn, C., Nowell, K., and Brown, J. (2011). Activation of GABAA or 5HT1A receptors in the raphé pallidus abolish the cardiovascular responses to exogenous stress in conscious rats. Brain Res. Bull. 86, 360–366. doi: 10.1016/j.brainresbull.2011.09.018
Radley, J. J., Arias, C. M., and Sawchenko, P. E. (2006a). Regional differentiation of the medial prefrontal cortex in regulating adaptive responses to acute emotional stress. J. Neurosci. 26, 12967–12976. doi: 10.1523/JNEUROSCI.4297-06.2006
Radley, J. J., Gosselink, K. L., and Sawchenko, P. E. (2009). A discrete GABAergic relay mediates medial prefrontal cortical inhibition of the neuroendocrine stress response. J. Neurosci. 29, 7330–7340. doi: 10.1523/JNEUROSCI.5924-08.2009
Radley, J. J., Rocher, A. B., Miller, M., Janssen, W. G. M., Liston, C., Hof, P. R., et al. (2006b). Repeated stress induces dendritic spine loss in the rat medial prefrontal cortex. Cereb. Cortex 16, 313–320. doi: 10.1093/cercor/bhi104
Radley, J. J., Williams, B., and Sawchenko, P. E. (2008). Noradrenergic innervation of the dorsal medial prefrontal cortex modulates hypothalamo-pituitary-adrenal responses to acute emotional stress. J. Neurosci. 28, 5806–5816. doi: 10.1523/JNEUROSCI.0552-08.2008
Resstel, L. B. M., Fernandes, K. B. P., and Corrêa, F. M. A. (2005). α-Adrenergic and muscarinic cholinergic receptors are not involved in the modulation of the parasympathetic baroreflex by the medial prefrontal cortex in rats. Life Sci. 77, 1441–1451. doi: 10.1016/j.lfs.2005.03.012
Salomé, N., Ngampramuan, S., and Nalivaiko, E. (2007). Intra-amygdala injection of GABAA agonist, muscimol, reduces tachycardia and modifies cardiac sympatho-vagal balance during restraint stress in rats. Neuroscience 148, 335–341. doi: 10.1016/j.neuroscience.2007.06.022
Sanders, B. J., Wirtz-Nole, C., DeFord, S. M., and Erling, B. F. (1994). Central amygdaloid lesions attenuate cardiovascular responses to acute stress in rats with borderline hypertension. Physiol. Behav. 1994:3. doi: 10.1016/0031-9384(94)90231-3
Santana, N., and Artigas, F. (2017). Laminar and cellular distribution of monoamine receptors in rat medial prefrontal cortex. Front. Neuroanat. 11:87. doi: 10.3389/fnana.2017.00087
Santana, N., Mengod, G., and Artigas, F. (2013). Expression of α1-adrenergic receptors in rat prefrontal cortex: cellular co-localization with 5-HT2A receptors. Int. J. Neuropsychopharmacol. 16, 1139–1151. doi: 10.1017/S1461145712001083
Santos, C. E., Benini, R., and Crestani, C. C. (2020). Spontaneous recovery, time course, and circadian influence on habituation of the cardiovascular responses to repeated restraint stress in rats. Pflügers Arch. Eur. J. Physiol. 472, 1495–1506. doi: 10.1007/s00424-020-02451-9
Sara, S. J. (2009). The locus coeruleus and noradrenergic modulation of cognition. Nat. Rev. Neurosci. 10, 211–223. doi: 10.1038/nrn2573
Silva, C. C., Correa, A. M. B., Kushmerick, C., Sharma, N. M., Patel, K. P., de Almeida, J. F. Q., et al. (2020). Angiotensin-converting enzyme 2 activator, DIZE in the basolateral amygdala attenuates the tachycardic response to acute stress by modulating glutamatergic tone. Neuropeptides. 2020:102076. doi: 10.1016/j.npep.2020.102076
Spencer, S. J., and Day, T. A. (2004). Role of catecholaminergic inputs to the medial prefrontal cortex in local and subcortical expression of Fos after psychological stress. J. Neurosci. Res. 78, 279–288. doi: 10.1002/jnr.20242
Sterling, P. (2012). Allostasis: a model of predictive regulation. Physiol. Behav. 106, 5–15. doi: 10.1016/j.physbeh.2011.06.004
Tavares, R. F., Corrêa, F. M. A., and Resstel, L. B. M. (2009). Opposite role of infralimbic and prelimbic cortex in the tachycardiac response evoked by acute restraint stress in rats. J. Neurosci. Res. 87, 2601–2607. doi: 10.1002/jnr.22070
Tellez, S., Colpaert, F., and Marien, M. (1999). α2-Adrenoceptor modulation of cortical acetylcholine release in vivo. Neuroscience 89, 1041–1050. doi: 10.1016/S0306-4522(98)00392-3
Ulrich-Lai, Y. M., and Herman, J. P. (2009). Neural regulation of endocrine and autonomic stress responses. Nat. Rev. Neurosci. 10, 397–409. doi: 10.1038/nrn2647
Vertes, R. P. (2004). Differential projections of the infralimbic and prelimbic cortex in the rat. Synapse 51, 32–58. doi: 10.1002/syn.10279
Vianna, D. M. L., Allen, C., and Carrive, P. (2008). Cardiovascular and behavioral responses to conditioned fear after medullary raphe neuronal blockade. Neuroscience 153, 1344–1353. doi: 10.1016/j.neuroscience.2008.03.033
Vianna, D. M. L., and Carrive, P. (2005). Changes in cutaneous and body temperature during and after conditioned fear to context in the rat. Eur. J. Neurosci. 21, 2505–2512. doi: 10.1111/j.1460-9568.2005.04073.x
Vidal-Gonzalez, I., Vidal-Gonzalez, B., Rauch, S. L., and Quirk, G. J. (2006). Microstimulation reveals opposing influences of prelimbic and infralimbic cortex on the expression of conditioned fear. Learn. Mem. 13, 728–733. doi: 10.1101/lm.306106
Wang, M., Ramos, B. P., Paspalas, C. D., Shu, Y., Simen, A., Duque, A., et al. (2007). α2A-adrenoceptors strengthen working memory networks by inhibiting cAMP-HCN channel signaling in prefrontal cortex. Cell 129, 397–410. doi: 10.1016/j.cell.2007.03.015
Wood, M., Adil, O., Wallace, T., Fourman, S., Wilson, S. P., Herman, J. P., et al. (2019). Infralimbic prefrontal cortex structural and functional connectivity with the limbic forebrain: a combined viral genetic and optogenetic analysis. Brain Struct. Funct. 224, 73–97. doi: 10.1007/s00429-018-1762-6
Wood, S. K., and Valentino, R. J. (2017). The brain norepinephrine system, stress and cardiovascular vulnerability. Neurosci. Biobehav. Rev. 74, 393–400. doi: 10.1016/j.neubiorev.2016.04.018
Keywords: adrenoceptor, prefrontal cortex, sympathetic activity, blood pressure, heart rate, rodents, psychological stress
Citation: Oliveira LA, Pollo TRS, Rosa EA, Duarte JO, Xavier CH and Crestani CC (2021) Both Prelimbic and Infralimbic Noradrenergic Neurotransmissions Modulate Cardiovascular Responses to Restraint Stress in Rats. Front. Physiol. 12:700540. doi: 10.3389/fphys.2021.700540
Received: 27 April 2021; Accepted: 16 July 2021;
Published: 12 August 2021.
Edited by:
Eugene Nalivaiko, The University of Newcastle, AustraliaReviewed by:
Lu Liu, University of New South Wales, AustraliaMaciej Gawlak, Medical University of Warsaw, Poland
Copyright © 2021 Oliveira, Pollo, Rosa, Duarte, Xavier and Crestani. This is an open-access article distributed under the terms of the Creative Commons Attribution License (CC BY). The use, distribution or reproduction in other forums is permitted, provided the original author(s) and the copyright owner(s) are credited and that the original publication in this journal is cited, in accordance with accepted academic practice. No use, distribution or reproduction is permitted which does not comply with these terms.
*Correspondence: Carlos C. Crestani, Y2FybG9zLmNyZXN0YW5pJiN4MDAwNDA7dW5lc3AuYnI=
†These authors have contributed equally to this work and share first authorship