- Department of Pharmacology and Toxicology, College of Osteopathic Medicine, Michigan State University, East Lansing, MI, United States
Resistance arteries and downstream arterioles in the peripheral microcirculation contribute substantially to peripheral vascular resistance, control of blood pressure, the distribution of blood flow to and within tissues, capillary pressure, and microvascular fluid exchange. A hall-mark feature of these vessels is myogenic tone. This pressure-induced, steady-state level of vascular smooth muscle activity maintains arteriolar and resistance artery internal diameter at 50–80% of their maximum passive diameter providing these vessels with the ability to dilate, reducing vascular resistance, and increasing blood flow, or constrict to produce the opposite effect. Despite the central importance of resistance artery and arteriolar myogenic tone in cardiovascular physiology and pathophysiology, our understanding of signaling pathways underlying this key microvascular property remains incomplete. This brief review will present our current understanding of the multiple mechanisms that appear to underlie myogenic tone, including the roles played by G-protein-coupled receptors, a variety of ion channels, and several kinases that have been linked to pressure-induced, steady-state activity of vascular smooth muscle cells (VSMCs) in the wall of resistance arteries and arterioles. Emphasis will be placed on the portions of the signaling pathways underlying myogenic tone for which there is lack of consensus in the literature and areas where our understanding is clearly incomplete.
Introduction
Myogenic tone is a hall-mark feature of resistance arteries and their downstream arterioles. This pressure-induced contractile activity of vascular smooth muscle contributes substantially to all functions of the resistance vasculature, including maintenance of peripheral vascular resistance, control of blood pressure, distribution of blood flow to and within tissues, and regulation of capillary pressure and microvascular fluid exchange. However, our understanding of the molecular mechanisms responsible for myogenic tone remains incomplete. This review will outline the multiple mechanisms that appear to underlie this key microvascular process, including important roles for G-protein-coupled receptors, multiple ion channels, and several protein kinases emphasizing the portions of the signaling pathways for which there is a lack of consensus.
Setting the Stage
What Are Resistance Arteries and Arterioles?
Resistance arteries are arterial vessels that feed blood flow to the microcirculation and contribute to peripheral vascular resistance (Zweifach and Lipowsky, 1984; Segal, 2000). These small arteries have internal, maximal diameters ranging from 500 to 100 μm and have two or more layers of vascular smooth muscle in their walls. Arterioles are downstream from resistance arteries, usually have internal, maximal diameters less than 100 μm, and importantly, have only a single layer of vascular smooth muscle wrapped circumferentially around the endothelial cell tube that forms the lumen of these microvessels. Another distinguishing characteristic of arterioles is that they are usually embedded within the parenchyma to which they supply blood flow. Arterioles form a branching network of vessels that ultimately provide blood flow to the capillary bed, with 3–5 levels of branching, dependent on the tissue/organ being perfused. The last arterial microvessels with vascular smooth muscle cells (VSMCs) in their walls are termed terminal arterioles which then branch into 1–20 capillaries. As with resistance arteries, arterioles contribute substantially to determination and control of vascular resistance and blood pressure (Renkin, 1984; Zweifach and Lipowsky, 1984; Pries and Secomb, 2011).
In skeletal muscle, for example, resistance arteries contribute about 30–40% of total skeletal muscle vascular resistance (Segal and Duling, 1986; Segal, 2000), with downstream arterioles contributing 50% and capillary and venules contributing the remainder of the hydraulic resistance (Fronek and Zweifach, 1975). Thus, for example, during skeletal muscle contraction, when blood flow can increase 100-fold (Saltin et al., 1998; Mortensen and Saltin, 2014), coordinated vasodilation of arterioles in the microcirculation and upstream resistance arteries is essential for attainment of these massive increases in blood flow (Segal and Duling, 1986; Segal, 2000).
What Are the Myogenic Response and Myogenic Tone?
A step-wise increase in the blood pressure within a resistance artery or arteriole leads to a rapid, pressure-induced increase in vessel diameter as shown in Figure 1A. If the pressure is maintained, the smooth muscle in the wall of the vessel will respond, contracting and returning the internal diameter of the vessel to or below its initial diameter (Figure 1A). This is the classic myogenic response (Johnson, 1980; Davis and Hill, 1999; Hill et al., 2001; Davis et al., 2011) that was originally described by Bayliss over a century ago (Bayliss, 1902). The steady-state level of contractile activity of the vascular smooth muscle in a pressurized blood vessel is myogenic tone (Figure 1B). It should be noted that myogenic tone not only encompasses the steady-state activity of the smooth muscle contractile machinery (actin-myosin cross bridge cycling), but also remodeling of the actin cytoskeleton (Gunst and Zhang, 2008) and alterations in interactions of smooth muscle cells with the extracellular matrix that accompanies maintained vasoconstriction (Martinez-Lemus et al., 2004) or vasodilation (Clifford et al., 2018). An important point is that the time scale for these latter events (remodeling of the actin cytoskeleton and interactions and remodeling of the extracellular matrix) may occur on much longer time scales than simple Ca2+-dependent cross-bridge cycling during maintained levels of myogenic tone.
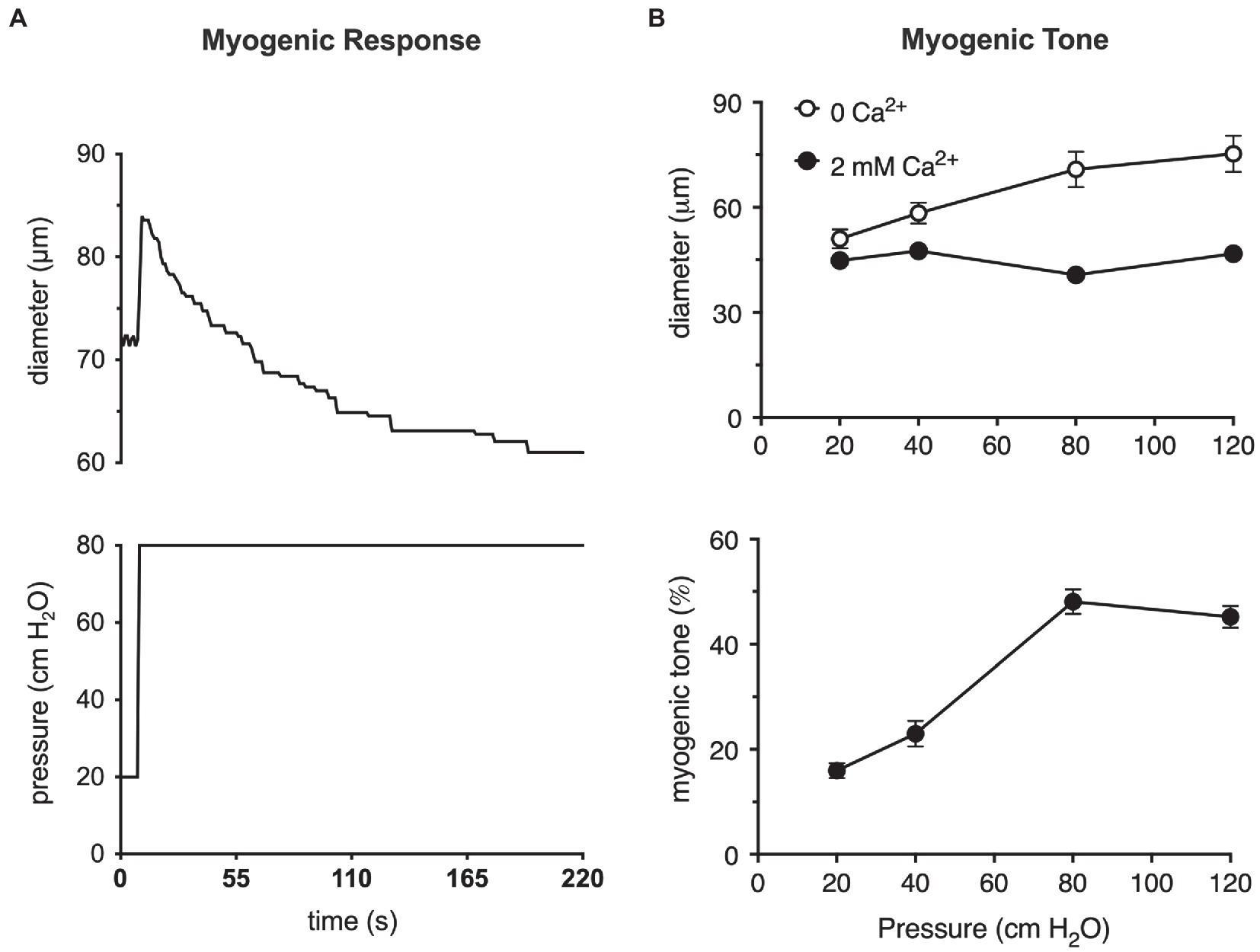
Figure 1. The myogenic response and myogenic tone in arterioles. (A) Myogenic response in a cannulated hamster cremaster arteriole, ex vivo, prepared as described (Westcott and Jackson, 2011). Shown in the upper panel is a digitized diameter record of the response of a second-order cremaster arteriole to a step-increase in luminal pressure from 20 to 80 cm H2O as depicted in the lower panel. At the onset of the pressure step, arteriolar diameter increases due to passive distention of the vessel. As the smooth muscle responds and begins to contract, diameter recovers to a new steady-state diameter that is slightly less than the diameter at 20 cm H2O. This behavior is the myogenic response. (B) Myogenic tone in cannulated hamster cremaster arterioles. Top panel shows the steady-state diameters of arterioles at different pressures in the absence of extracellular Ca2+ (passive) and presence of 2 mM Ca2+ (active). Bottom panel shows the % myogenic tone at the given pressures computed from the data in the top panel as myogenic tone = (passive diameter–active diameter)/passive diameter × 100%. At pressures greater than 20 cm H2O, arterioles develop significant myogenic tone (i.e., steady-state pressure-induced constriction). Data in (A) are replotted from Jackson (2012), with permission. Data in (B) are replotted from Westcott and Jackson, (2011) and Jackson, (2012), with permission.
These two processes, the myogenic response and myogenic tone (Figure 1), participate in the blood flow autoregulation in organs, such as the brain (Tuma, 2011), heart (Laughlin et al., 1996; Zhang et al., 2011), kidney (Navar et al., 2011), eye (Riva and Schmetterer, 2011), intestine (Granger et al., 2011), and skeletal muscle (Shepherd, 1983), buffering organ blood flow and capillary pressure in the face of changes in blood pressure (Johnson, 1980; Davis et al., 2011). The myogenic response and myogenic tone also appear to contribute to blood pressure regulation by amplifying vasoconstrictor-induced vasoconstriction (Meininger and Trzeciakowski, 1988, 1990). Myogenic tone offers a resting level of smooth muscle contractile activity such that resistance arteries and arterioles can both dilate and constrict around their resting diameters, maintaining cardiovascular homeostasis (Renkin, 1984; Davis et al., 2011).
Steps Involved in the Myogenic Response Leading to Myogenic Tone
Two questions need to be answered to understand the mechanisms responsible for the myogenic response and myogenic tone: (1) How are changes in luminal pressure sensed by smooth muscle cells in the wall of resistance arteries and arterioles? and (2) How are changes in luminal pressure transduced into vascular smooth muscle contraction and maintained tone in these vessels?
How is Pressure Sensed in Resistance Arteries and Arterioles?
In a resistance artery or arteriole, increased luminal pressure results in a radial force that increases tangential wall stress (tension) as described by the Law of Laplace: tangential wall stress = Pr/Δ, where P is the pressure in the lumen of the vessel, r is the lumen radius, and Δ is the thickness of the vessel wall. The increased wall stress passively dilates the vessel and “stretches” (induces strain in) the smooth muscle cells in the vessel wall. The passive dilation will continue until either, the passive wall tension (due to collagen, elastin, cytoskeletal elements, etc.) matches the pressure-induced tangential wall stress, or the VSMCs actively contract and generate enough active wall stress to overcome the pressure-driven tangential wall stress.
It has been argued that wall strain (change in length) is unlikely to be the main variable sensed in the myogenic response because, for example, arterioles, often constrict to diameters below their starting point with step increases in pressure (Johnson, 1980; Carlson and Secomb, 2005). However, cytoskeletal remodeling appears to occur coincident and in parallel with, smooth muscle contraction and relaxation such that cell “length” is not a constant (Gunst and Zhang, 2008). Thus, whether it is stress or strain that is sensed in the vessel wall remains to be clarified (Hill and Meininger, 1994; Davis and Hill, 1999; Hill et al., 2001; Davis et al., 2011). Nonetheless, several mechanisms have been proposed to act as sensors during the myogenic response leading up to steady-state myogenic tone including: several G-protein coupled receptors (Brayden et al., 2013; Schleifenbaum et al., 2014; Storch et al., 2015; Kauffenstein et al., 2016; Mederos et al., 2016; Hong et al., 2017; Pires et al., 2017; Chennupati et al., 2019), several cation channels (Welsh et al., 2002; Jernigan and Drummond, 2005; Gannon et al., 2008; VanLandingham et al., 2009; Nemeth et al., 2020), integrins (Davis et al., 2001; Martinez-Lemus et al., 2005; Colinas et al., 2015), matrix metalloproteinases (MMPs), and epidermal growth factor receptors (EGFR; Lucchesi et al., 2004; Amin et al., 2011); and membrane-bound tumor necrosis factor α (mTNFα), TNFα receptor (TNFR), and downstream sphingosine-1-phosphate (S1P) signaling (Kroetsch et al., 2017; Figure 2). What remains unclear is what determines which of these putative mechanosensitive elements are expressed in a particular blood vessel and how this expression is controlled under different physiological and pathological conditions. It is also not clear whether the different potential mechanosensitive elements represent independent “sensors” or whether some are linked together. For example, while there is evidence that TRPC6 channels are mechanosensitive and could serve as independent sensors of pressure-induced stress/strain in vascular smooth muscle (Spassova et al., 2006), it is also quite clear that these channels lie downstream from mechanosensitive G-protein coupled receptors, like the angiotensin II type 1 receptors (AT1R) that appear to mediate myogenic reactivity in rodent cerebral resistance arteries (Gonzales et al., 2014). Another example is the potential link between G-protein coupled receptors, such as the AT1R and the EGFR. There is considerable evidence for transactivation of the EGFR and its downstream targets upon activation of AT1R by angiotensin II (Forrester et al., 2016). Thus, it seems likely that mechanical activation of AT1R would do the same and may reconcile studies identifying EGFR as a key component of myogenic signaling in coronary artery vascular smooth muscle (Lucchesi et al., 2004; Amin et al., 2011) with studies identifying AT1R as the key mechanosensor in myogenic tone (Mederos y Schnitzler et al., 2008; Yasuda et al., 2008; Storch et al., 2012; Gonzales et al., 2014; Mederos et al., 2016; Hong et al., 2017; Pires et al., 2017). However, it is not clear how signaling downstream from the EGFR involving extracellular signal-related kinases 1 and 2 (ERK1/2), janus kinase (JAK), and signal transducer and activator of transcription 3 (STAT3; Amin et al., 2011) fits into the overall scheme of myogenic tone. Similarly, while a role for mTNFα, its receptor and downstream S1P have been proposed to mediate myogenic tone (Peter et al., 2008; Lidington et al., 2009; Yang et al., 2012; Hui et al., 2015; Yagi et al., 2015; Sauve et al., 2016; Kroetsch et al., 2017), it is not clear how this mechanism “fits” with the bulk of data supporting membrane depolarization and activation of voltage-gated Ca2+ channels (VGCCs) as the fundamental basis for myogenic tone. Certainly, activation of PLCγ is a potential downstream signal in the S1P-pathway feeding into the same signaling pathway that has been proposed for AT1R, for example Gonzales et al. (2014). In addition, S1P signaling reportedly can lie downstream from the AT1R (Wilson et al., 2015). Unfortunately, few investigators have tried to perform critical tests of alternative hypotheses for all the proposed mechanisms underlying myogenic tone to try and sort out which mechanisms are functional in a particular blood vessel. In addition, most investigators tend to focus on single or at most a few blood vessels such that the generality of proposed mechanisms remains unclear and will require additional research. Investigators are encouraged to explore multiple mechanisms in arteries/arterioles from different vascular beds so that patterns can be better assessed.
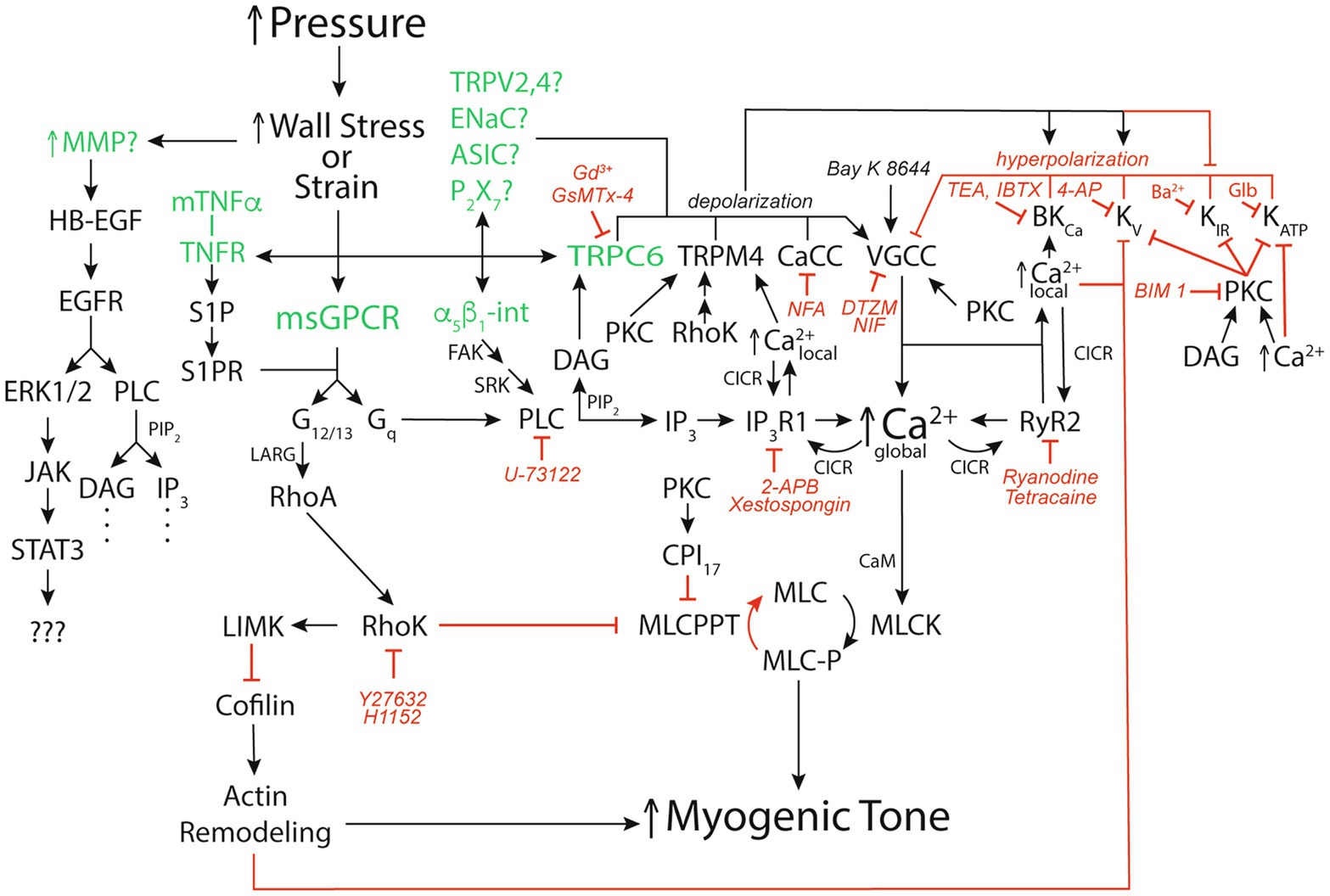
Figure 2. Signaling pathways for pressure-induced myogenic tone. Schematic diagram of reported signaling pathways involved in myogenic tone in resistance arteries and arterioles. Green font color depicts putative mechanosensors in pressure-induced myogenic tone. Black arrows show stimulation, increases or activation of signaling molecules, ion channels, or enzymes that participate in myogenic tone. Red capped lines indicate inhibition, decreases or deactivation of signaling molecules, ion channels, or enzymes involved in myogenic tone. Also shown are pharmacological agents that we have used to interrogate the ion channels and signaling pathways in arteriolar myogenic tone. MMP, matrix metalloproteinase; HB-EGF, heparin-bound epidermal growth factor; EGFR, epidermal growth factor receptor; ERK1/2, extracellular-signal-related kinases 1 or 2; JAK, janus kinase; STAT3, signal transducer and activator of transcription 3; mTNFα, membrane-bound tumor necrosis factor α; TNFR, TNFα Receptor; S1P, sphingosine-1-phosphate; S1PR, S1P receptor; α5β1-int, α5β1 integrin: FAK, focal adhesion kinase; SRK, Src-related kinases; CaCC, Ca2+-activated Cl− channel; TRPV2,4, transient receptor potential vanilloid-family 2 or 4 channels; ENaC, epithelial Na+ channel; ASIC, acid sensing ion channel; P2X7, P2X7 purinergic receptor; VGCC, voltage-gated Ca2+ channel; BKCa, large-conductance Ca2+-activated K+ channel; KV, voltage-gated K+ channel; KIR, inwardly-rectifying K+ channel; KATP, ATP-sensitive K+ channel; msGPCR, mechanosensitive G-protein-coupled receptor; DAG, diacylglycerol; PKC, protein kinase C; NFA, niflumic acid; DTZM, diltiazem; NIF, nifedipine; TEA, tetraethylammonium; IBTX, iberiotoxin; 4-AP, 4-aminopyridine; GLIB, glibenclamide; BIM I, bisindolylmaleimide I; PLC, phospholipase C; PIP2, phosphatidylinositol bisphosphate; IP3, inositol, 1,4,5 trisphosphate; IP3R1, IP3 receptor 1; RyR, ryanodine receptor; CICR, Ca2+-induced-Ca2+ release; LARG, guanine nucleotide exchange factor LARG; RhoA, small G-protein Rho; 2-APB, 2-Aminoethoxydiphenyl borate; RhoK. Rho kinase; LIMK, LIM kinase; CPI17, C-kinase potentiated protein phosphatase-1 Inhibitor; MLCPPT, myosin light-chain phosphatase; MCL, myosin light-chain; MLCK, myosin light-chain kinase; See text for more details and references.
Finally, it is not yet known exactly how membrane proteins are activated by increases in membrane stress or strain. Based on studies in other systems, membrane-bound proteins may be activated or altered by forces transmitted via connections with the extracellular matrix and/or the cytoskeleton, by changes in membrane curvature and/or by membrane thinning-induced protein conformational changes (Leiphart et al., 2019).
How Are Changes in Luminal Pressure Transduced Into Myogenic Tone?
Regardless of the precipitating mechanisms, in vitro, pressure myography studies have repeatedly shown that pressure-induced myogenic tone involves vascular smooth muscle membrane depolarization, activation of VGCCs, and an increase in intracellular Ca2+ (Harder, 1984; Knot and Nelson, 1998; Kotecha and Hill, 2005; Figure 2). As will be discussed in a subsequent section, there also may be activation of the small G-protein RhoA and the Rho kinase pathway which induces Ca2+ sensitization (Lagaud et al., 2002; Moreno-Dominguez et al., 2013) and Rho-kinase (Moreno-Dominguez et al., 2013) or protein kinase C (PKC)-dependent remodeling of the actin cytoskeleton (Moreno-Dominguez et al., 2013; Hong et al., 2016) that also can contribute to myogenic tone (Figure 2).
Which Ion Channels Contribute to Pressure-Induced Membrane Depolarization?
A number of ion channels have been proposed to contribute to pressure-induced depolarization of VSMCs (Harder, 1984; Knot and Nelson, 1998; Kotecha and Hill, 2005) including: members of the transient receptor potential (TRP)-family of cation channels, such as TRPC6 (Welsh et al., 2002; Gonzales et al., 2014), TRPM4 (Earley et al., 2004; Gonzales et al., 2014), TRPV2 (McGahon et al., 2016), and TRPV4 (Soni et al., 2017); members of the degenerin family of channels including the epithelial Na+ channel (ENaC) family (Jernigan and Drummond, 2005, 2006; VanLandingham et al., 2009; Drummond and Stec, 2015), the acid-sensitive ion channel (ASIC) family of channels (Gannon et al., 2015), and purinergic P2X7 Purinergic Receptor (P2X7) channels (Kauffenstein et al., 2016; Figure 2). In addition, Ca2+-activated Cl− channels (CaCC; ANO1/TMEM16A) may be activated by Ca2+ influx through TRPC6 channels (Bulley et al., 2012; Wang et al., 2016) and also contribute to pressure-induced depolarization of VSMCs (Figure 2). Finally, the activity of several K+ channels may be inhibited by either the membrane depolarization [inwardly-rectifying K+ channel (KIR) channels], increases in intracellular Ca2+ [voltage-gated K+ channel (KV) and ATP-sensitive K+ channel (KATP) channels], or increased activity of kinases such as protein kinase C (KV and KATP channels) and Rho kinase (KV channels) and indirectly contribute to pressure-induced depolarization (Tykocki et al., 2017; Figure 2). As for other aspects of myogenic tone, there appears to be vascular bed- and, perhaps, species-dependent differences in the ion channels that participate in pressure-induced depolarization making generalizations difficult. Our understanding of the mechanisms responsible for this heterogeneity is lacking.
What Activates the Ion Channels Responsible for Pressure-Induced Depolarization?
The answer to this question remains incomplete. In cerebral resistance arteries, TRPC6 and TRPM4 appear to be activated indirectly (Gonzales et al., 2014), likely downstream from pressure-induced activation of AT1R and phospholipase Cγ (PLCγ; Gonzales et al., 2014) with diacylglycerol (DAG) from hydrolysis of membrane phosphatidyl-inositol-bis-phosphate (PIP2) activating TRPC6 and Ca2+ from Ca2+- and IP3-induced release of Ca2+ through IP3 receptor (IP3R) activating TRPM4 (Gonzales et al., 2014; Figure 2). In cerebral penetrating arterioles, Rho kinase may be involved in the activation of TRPM4 by setting its Ca2+ sensitivity (Li and Brayden, 2017; Figure 2). However, not all blood vessels appear to require PLC for pressure-induced myogenic tone. For example, we have shown that myogenic tone and global Ca2+ levels in hamster cheek pouch arterioles are unaffected by inhibition of PLC and IP3R, and yet are inhibited by nanomolar concentrations of Gd3+ and micromolar concentrations of GsMTX-4, established inhibitors of mechanosensitive ion channels (Jackson and Boerman, 2017). Thus, it is possible that, in cheek pouch arterioles, pressure-induced mechanical activation of TRPC6, for example, directly contributes to membrane depolarization and myogenic tone (Figure 2).
Which Ion Channels Contribute to the Negative-Feedback Regulation of Myogenic Tone?
Membrane depolarization in cells that express VGCCs is inherently a positive feedback process that would lead to depolarization approaching the Nernst equilibrium potential for Ca2+ (approximately +60 mV) and maximal vasoconstriction if it were not for negative feedback mechanisms that limit membrane depolarization and the activity of VGCCs. In VSMCs, this negative feedback is provided by large-conductance Ca2+-activated K+ (BKCa) channels and several voltage-gated K+ (KV) channel family members including KV1.5, 2.1, and 7.X channels (Tykocki et al., 2017; Figure 2). The membrane depolarization induced by TRPC6, TRPM4, etc. activates both BKCa and KV channels, limiting membrane depolarization (Tykocki et al., 2017; Figure 2). The BKCa channels are also activated by increased Ca2+ (Tykocki et al., 2017). In resistance arteries, the source of Ca2+ responsible for activation of BKCa channels is Ca2+ sparks released through ryanodine receptors (RyR; Nelson et al., 1995; Westcott and Jackson, 2011; Westcott et al., 2012), whereas in downstream arterioles, BKCa channels appear to be activated by Ca2+ entry through VGCCs and other membrane channels (Guia et al., 1999; Westcott and Jackson, 2011; Westcott et al., 2012; Suzuki et al., 2013; Figure 2).
Which Ion Channels Contribute to Pressure-Induced Increases in Global Intracellular Ca2+?
L-type VGCCs composed of CaV1.2 α-pore-forming subunits contribute substantially to pressure-induced myogenic tone that is observed in pressurized resistance arteries and arterioles studied ex vivo (Tykocki et al., 2017; Figure 2). L-type CaV1.2 VGCCs appear essential for the initiation of the myogenic response because block of these channels prevents the development of myogenic tone (Knot and Nelson, 1998; Kotecha and Hill, 2005). In rat middle cerebral arteries, the voltage dependence of intracellular Ca2+ and myogenic tone is the same as that for currents through L-type VGCCs, and both depolarization-induced increases in intracellular Ca2+ and myogenic tone are prevented or completely reversed by L-type VGCC blockers (Knot and Nelson, 1998). In first-order rat cremaster muscle arterioles, the relationship between membrane potential and tone is steeper than observed in cerebral arteries, and a significant portion of pressure-induced tone remains after block of L-type VGCCs (Kotecha and Hill, 2005). Block of L-type VGCCs also only inhibits a portion of Ca2+-dependent myogenic tone in second-order hamster cremaster (86%) and cheek pouch (54%) arterioles (Jackson and Boerman, 2017). These data suggest that Ca2+ entry through additional ion channels, such as T-type VGCCs (CaV3.X) or mechano-sensitive cation channels such as TRPC6, for example, also contribute to elevated [Ca2+]in and activation of contraction, particularly in the microcirculation (Tykocki et al., 2017). While it has been shown that smooth muscle-specific knockout of CaV1.2 abolishes myogenic reactivity in murine tibialis arteries (Moosmang et al., 2003), these data are difficult to interpret because CaV1.2 appears essential for the initiation of the myogenic response and hence, myogenic tone (Knot and Nelson, 1998; Kotecha and Hill, 2005).
The role played by SMC CaV1.2 channels in myogenic tone in arterioles, in situ, is not as clear. While there are a number of in situ studies supporting a role for CaV1.2 channels in various vascular beds (see Tykocki et al., 2017 for refs.), several intravital microscopy studies of arterioles in rat (Hill and Meininger, 1994), hamster (Jackson, 2012) and mouse (Pemberton et al., 1996; Ngo et al., 2013) cremaster muscles, and hamster cheek pouch (Boric et al., 1990; Welsh et al., 1998) have shown that topical application of L-type channel blockers has little effect on resting myogenic tone. Importantly, the efficacy the Ca2+ channel blockers was verified because they abolished vasomotion (Hill and Meininger, 1994; Welsh et al., 1998; Jackson, 2012; Ngo et al., 2013) and prevented O2-induced vasoconstriction (Welsh et al., 1998; Jackson, 2012). The resting tone in these studies appeared to be voltage-dependent because KATP channel agonists such as pinacidil (Jackson, 1993; Hill and Meininger, 1994) and cromakalim (Jackson, 1993) cause near maximal dilation of arterioles in these preparations. These data suggest that some other voltage-dependent channel, such as T-type CaV3.X channels for example, can determine resting tone in these microvascular beds under the conditions studied, that is simply not recapitulated in the ex vivo study of isolated resistance arteries and arterioles where CaV1.2 channels appear essential.
In some resistance arteries and arterioles, Ca2+ influx through VGCCs also appears to be amplified by Ca2+ release from intracellular stores (Figure 2). In cremaster arterioles, for example, Ca2+ influx through L-type VGCCs activates Ca2+ release through IP3Rs in the form of Ca2+ waves that contribute to myogenic tone (Westcott and Jackson, 2011; Westcott et al., 2012; Jackson and Boerman, 2018). In resistance arteries upstream from cremaster arterioles, Ca2+ waves also contribute to myogenic tone, but appear to involve both IP3R and RyR (Westcott and Jackson, 2011; Westcott et al., 2012).
How Is the Pressure-Dependent Ca2+ Signal Translated Into Tone?
The global increase in intracellular Ca2+ that results from activation of membrane mechanoreceptive processes, depolarization, activation of VGCCs, and amplification by Ca2+ release from intracellular stores is translated into smooth muscle contraction mainly through binding of Ca2+ to the Ca2+-binding protein, calmodulin (CaM), and Ca2+-CaM-dependent activation of myosin light-chain kinase (MLCK). This results in phosphorylation of the 20 kD myosin light-chains which is the primary trigger for contraction and force production in vascular smooth muscle (Zou et al., 1995; Cole and Welsh, 2011; Figure 2). Myosin light-chain phosphorylation then allows interaction of filamentous actin with myosin, the formation of actin-myosin cross-bridges, cross-bridge cycling, and smooth muscle contraction or force generation (increased myogenic tone; Zou et al., 1995; Cole and Welsh, 2011; Figure 2). This process continues while Ca2+ remains elevated and cross-bridge cycling occurs. A reduction in intracellular Ca2+ or dephosphorylation of the myosin light-chains by myosin light-chain phosphatase (MLCPPT) turns off this process and allows smooth muscle relaxation (decreased myogenic tone; Zou et al., 1995; Cole and Welsh, 2011). The ratio of activity of MLCK/MLCPPT determines the Ca2+ sensitivity of the system (Cole and Welsh, 2011). Guanine nucleotide exchange factors (GEFs), such as LARG, couple G-proteins, such as G12/13 to activation of the small GTPase, RhoA which subsequently activates Rho Kinase (Chennupati et al., 2019; Figure 2). Active Rho Kinase has several targets that modulate myogenic tone including: phosphorylation and inhibition of MLCPPT and an increase in Ca2+ sensitivity (Cole and Welsh, 2011); activation of LIM kinase (LIMK) and subsequent inhibition of cofilin and actin-cytoskeleton remodeling (Loirand et al., 2006; Moreno-Dominguez et al., 2013); inhibition of KV channels (Luykenaar et al., 2009); activation of TRPM4 channels (Li and Brayden, 2017); and activation of VGCCs (Guan et al., 2019; Figure 2). All of these Rho Kinase-related effects promote increased myogenic tone. Myogenic tone can also be increased/sustained through G-protein-dependent activation of PKC that not only modulates ion channels, but also the Ca2+ sensitivity of the contractile machinery through phosphorylation of the protein CPI17 which inhibits MLCPPT (Cole and Welsh, 2011). As noted previously, PKC also can lead to actin cytoskeleton remodeling that contributes to myogenic tone (Moreno-Dominguez et al., 2013; Hong et al., 2016; Figure 2).
What is Responsible for the Apparent Heterogeneity in Mechanisms Underlying Myogenic Tone?
As outlined in previous sections, there are likely multiple mechanisms that resistance arteries and arterioles use to produce and modulate myogenic tone depending on their location in the body and the physiology/pathophysiology of the system. What determines the primary mechanisms that are functional in a resistance artery or arteriole under a given set of physiological or pathophysiological conditions remains to be established. In experimental diabetes and subarachnoid hemorrhage, for example, it has been shown that there is apparent upregulation of the role played by mTNFα and S1P-signaling in myogenic tone of skeletal muscle (Sauve et al., 2016) and cerebral (Yagi et al., 2015) resistance arteries. However, the mechanisms responsible for this upregulation remain to be established. Another example is the differences that we have found in the mechanisms of myogenic tone in hamster cremaster vs. cheek pouch second-order arterioles (Jackson and Boerman, 2017). In cremaster arterioles, PLC and IP3R substantially contribute to Ca2+ signals (Ca2+ waves) and pressure-induced myogenic tone, whereas cheek pouch arterioles generate a similar level of tone that is independent of PLC and IP3R signaling (Jackson and Boerman, 2017). The mechanisms responsible for this regional heterogeneity are not known. Nonetheless, these differences in mechanisms likely mean that vascular smooth muscle in resistance arteries and arterioles has a “toolbox” of mechanisms that are potentially available to support the vital process of the myogenic response and myogenic tone in health and disease. Regional heterogeneity in mechanisms of myogenic tone may also provide new drug targets to treat vascular disease in an organ or tissue specific manner. For this to become a reality, much more research will be required to: (1) identify all of the potential signaling pathways that can contribute to myogenic tone in a selection of resistance arteries and arterioles from different vascular beds around the body using high density transcriptomic and proteomic approaches, understanding that many ion channels and receptors are normally expressed at very low levels in VSMCs, despite having major contributions to vessel function; (2) gain a better understanding of the regulation of message and protein expression for all these components using sophisticated pathway and informatic analysis of the “signals” detected in the transcriptomic and proteomic screens; and (3) perform appropriate functional in situ and ex vivo studies measuring diameter (as a readout of smooth muscle contraction at given levels of pressure), membrane potential, both local and global Ca2+ signals as well as careful biochemical assessment of pathway activity (protein phosphorylation, etc., see Moreno-Dominguez et al., 2013; for example) using conditional, cell-specific knockout, knockdown and knockin strategies as well as careful pharmacology to evaluate the role of the various signaling pathways that can contribute to myogenic tone in both resistance arteries and arterioles from around the body. This is a daunting task, but one that appears essential to move this field forward.
Author Contributions
WJ conceived, wrote, and edited this manuscript and is solely responsible for its content. The content is solely the responsibility of the author and does not necessarily represent the official views of the National Institutes of Health.
Funding
This work was supported by National Heart, Lung, and Blood Institute grants HL-137694 and PO1-HL-070687.
Conflict of Interest
The author declares that the research was conducted in the absence of any commercial or financial relationships that could be construed as a potential conflict of interest.
References
Amin, A. H., Abd Elmageed, Z. Y., Partyka, M., and Matrougui, K. (2011). Mechanisms of myogenic tone of coronary arteriole: role of down stream signaling of the EGFR tyrosine kinase. Microvasc. Res. 81, 135–142. doi: 10.1016/j.mvr.2010.11.001
Bayliss, W. M. (1902). On the local reactions of the arterial wall to changes of internal pressure. J. Physiol. 28, 220–231. doi: 10.1113/jphysiol.1902.sp000911
Boric, M. P., Donoso, V., Fournier, A., St Pierre, S., and Huidobro-Toro, J. P. (1990). Endothelin reduces microvascular blood flow by acting on arterioles and venules of the hamster cheek pouch. Eur. J. Pharmacol. 190, 123–133. doi: 10.1016/0014-2999(90)94119-i
Brayden, J. E., Li, Y., and Tavares, M. J. (2013). Purinergic receptors regulate myogenic tone in cerebral parenchymal arterioles. J. Cereb. Blood Flow Metab. 33, 293–299. doi: 10.1038/jcbfm.2012.169
Bulley, S., Neeb, Z. P., Burris, S. K., Bannister, J. P., Thomas-Gatewood, C. M., Jangsangthong, W., et al. (2012). TMEM16A/ANO1 channels contribute to the myogenic response in cerebral arteries. Circ. Res. 111, 1027–1036. doi: 10.1161/CIRCRESAHA.112.277145
Carlson, B. E., and Secomb, T. W. (2005). A theoretical model for the myogenic response based on the length-tension characteristics of vascular smooth muscle. Microcirculation 12, 327–338. doi: 10.1080/10739680590934745
Chennupati, R., Wirth, A., Favre, J., Li, R., Bonnavion, R., Jin, Y. J., et al. (2019). Myogenic vasoconstriction requires G12/G13 and LARG to maintain local and systemic vascular resistance. eLife 8:e49374. doi: 10.7554/eLife.49374
Clifford, P. S., Ferguson, B. S., Jasperse, J. L., and Hill, M. A. (2018). Arteriolar vasodilation involves actin depolymerization. Am. J. Physiol. Heart Circ. Physiol. 315, H423–H428. doi: 10.1152/ajpheart.00723.2017
Cole, W. C., and Welsh, D. G. (2011). Role of myosin light chain kinase and myosin light chain phosphatase in the resistance arterial myogenic response to intravascular pressure. Arch. Biochem. Biophys. 510, 160–173. doi: 10.1016/j.abb.2011.02.024
Colinas, O., Moreno-Dominguez, A., Zhu, H. L., Walsh, E. J., Perez-Garcia, M. T., Walsh, M. P., et al. (2015). alpha5-integrin-mediated cellular signaling contributes to the myogenic response of cerebral resistance arteries. Biochem. Pharmacol. 97, 281–291. doi: 10.1016/j.bcp.2015.08.088
Davis, M. J., and Hill, M. A. (1999). Signaling mechanisms underlying the vascular myogenic response. Physiol. Rev. 79, 387–423. doi: 10.1152/physrev.1999.79.2.387
Davis, M. J., Hill, M. A., and Kuo, L. (2011). “Local regulation of microvascular perfusion,” in Comprehensive Physiology. ed. R. L. Terjung (Hoboken, NJ: John Wiley & Sons, Inc.), 161–284.
Davis, M. J., Wu, X., Nurkiewicz, T. R., Kawasaki, J., Davis, G. E., Hill, M. A., et al. (2001). Integrins and mechanotransduction of the vascular myogenic response. Am. J. Physiol. Heart Circ. Physiol. 280, H1427–H1433. doi: 10.1152/ajpheart.2001.280.4.H1427
Drummond, H. A., and Stec, D. E. (2015). betaENaC acts as a mechanosensor in renal vascular smooth muscle cells that contributes to renal myogenic blood flow regulation, protection from renal injury and hypertension. J. Nephrol. Res. 1, 1–9. doi: 10.17554/j.issn.2410-0579.2015.01.12
Earley, S., Waldron, B. J., and Brayden, J. E. (2004). Critical role for transient receptor potential channel TRPM4 in myogenic constriction of cerebral arteries. Circ. Res. 95, 922–929. doi: 10.1161/01.RES.0000147311.54833.03
Forrester, S. J., Kawai, T., O'Brien, S., Thomas, W., Harris, R. C., and Eguchi, S. (2016). Epidermal growth factor receptor transactivation: mechanisms, pathophysiology, and potential therapies in the cardiovascular system. Annu. Rev. Pharmacol. Toxicol. 56, 627–653. doi: 10.1146/annurev-pharmtox-070115-095427
Fronek, K., and Zweifach, B. W. (1975). Microvascular pressure distribution in skeletal muscle and the effect of vasodilation. Am. J. Phys. 228, 791–796.
Gannon, K. P., McKey, S. E., Stec, D. E., and Drummond, H. A. (2015). Altered myogenic vasoconstriction and regulation of whole kidney blood flow in the ASIC2 knockout mouse. Am. J. Physiol. Ren. Physiol. 308, F339–F348. doi: 10.1152/ajprenal.00572.2014
Gannon, K. P., Vanlandingham, L. G., Jernigan, N. L., Grifoni, S. C., Hamilton, G., and Drummond, H. A. (2008). Impaired pressure-induced constriction in mouse middle cerebral arteries of ASIC2 knockout mice. Am. J. Physiol. Heart Circ. Physiol. 294, H1793–H1803. doi: 10.1152/ajpheart.01380.2007
Gonzales, A. L., Yang, Y., Sullivan, M. N., Sanders, L., Dabertrand, F., Hill-Eubanks, D. C., et al. (2014). A PLCgamma1-dependent, force-sensitive signaling network in the myogenic constriction of cerebral arteries. Sci. Signal. 7:ra49. doi: 10.1126/scisignal.2004732
Granger, D. N., Kvietys, P. R., Korthuis, R. J., and Premen, A. J. (2011). “Microcirculation of the intestinal mucosa,” in Comprehensive Physiology. ed. R. L. Terjung (Hoboken, NJ: John Wiley & Sons, Inc.), 1405–1474.
Guan, Z., Baty, J. J., Zhang, S., Remedies, C. E., and Inscho, E. W. (2019). Rho kinase inhibitors reduce voltage-dependent Ca2+ channel signaling in aortic and renal microvascular smooth muscle cells. Am. J. Physiol. Ren. Physiol. 317, F1132–F1141. doi: 10.1152/ajprenal.00212.2018
Guia, A., Wan, X., Courtemanche, M., and Leblanc, N. (1999). Local Ca2+ entry through L-type Ca2+ channels activates Ca2+−dependent K+ channels in rabbit coronary myocytes. Circ. Res. 84, 1032–1042. doi: 10.1161/01.RES.84.9.1032
Gunst, S. J., and Zhang, W. (2008). Actin cytoskeletal dynamics in smooth muscle: a new paradigm for the regulation of smooth muscle contraction. Am. J. Phys. Cell Physiol. 295, C576–C587. doi: 10.1152/ajpcell.00253.2008
Harder, D. R. (1984). Pressure-dependent membrane depolarization in cat middle cerebral artery. Circ. Res. 55, 197–202. doi: 10.1161/01.RES.55.2.197
Hill, M. A., and Meininger, G. A. (1994). Calcium entry and myogenic phenomena in skeletal muscle arterioles. Am. J. Phys. 267, H1085–H1092. doi: 10.1152/ajpheart.1994.267.3.H1085
Hill, M. A., Zou, H., Potocnik, S. J., Meininger, G. A., and Davis, M. J. (2001). Invited review: arteriolar smooth muscle mechanotransduction: Ca2+ signaling pathways underlying myogenic reactivity. J. Appl. Physiol. 91, 973–983. doi: 10.1152/jappl.2001.91.2.973
Hong, K., Li, M., Nourian, Z., Meininger, G. A., and Hill, M. A. (2017). Angiotensin II type 1 receptor mechanoactivation involves RGS5 (regulator of G protein signaling 5) in skeletal muscle arteries: impaired trafficking of RGS5 in hypertension. Hypertension 70, 1264–1272. doi: 10.1161/HYPERTENSIONAHA.117.09757
Hong, K., Zhao, G., Hong, Z., Sun, Z., Yang, Y., Clifford, P. S., et al. (2016). Mechanical activation of angiotensin II type 1 receptors causes actin remodelling and myogenic responsiveness in skeletal muscle arterioles. J. Physiol. 594, 7027–7047. doi: 10.1113/JP272834
Hui, S., Levy, A. S., Slack, D. L., Burnstein, M. J., Errett, L., Bonneau, D., et al. (2015). Sphingosine-1-phosphate signaling regulates myogenic responsiveness in human resistance arteries. PLoS One 10:e0138142. doi: 10.1371/journal.pone.0138142
Jackson, W. F. (1993). Arteriolar tone is determined by activity of ATP-sensitive potassium channels. Am. J. Phys. 265, H1797–H1803.
Jackson, W. F. (2012). “Microcirculation,” in Muscle. eds. J. A. Olson and E. N. Hill (Boston/Waltham, MA: Academic Press), 1197–1206.
Jackson, W. F., and Boerman, E. M. (2017). Regional heterogeneity in the mechanisms of myogenic tone in hamster arterioles. Am. J. Physiol. Heart Circ. Physiol. 313, H667–H675. doi: 10.1152/ajpheart.00183.2017
Jackson, W. F., and Boerman, E. M. (2018). Voltage-gated Ca2+ channel activity modulates smooth muscle cell calcium waves in hamster cremaster arterioles. Am. J. Physiol. Heart Circ. Physiol. 315, H871–H878. doi: 10.1152/ajpheart.00292.2018
Jernigan, N. L., and Drummond, H. A. (2005). Vascular ENaC proteins are required for renal myogenic constriction. Am. J. Physiol. Ren. Physiol. 289, F891–F901. doi: 10.1152/ajprenal.00019.2005
Jernigan, N. L., and Drummond, H. A. (2006). Myogenic vasoconstriction in mouse renal interlobar arteries: role of endogenous beta and gammaENaC. Am. J. Physiol. Ren. Physiol. 291, F1184–F1191. doi: 10.1152/ajprenal.00177.2006
Johnson, P. C. (1980). “The myogenic response,” in Handbood of Physiology: Section 2, The Cardiovascular System, Volume II, Vascular Smooth Muscle. eds. D. F. Bohr, A. P. Somlyo, and H. V. Sparks (Bethesda, MD: American Physiological Society), 409–442.
Kauffenstein, G., Tamareille, S., Prunier, F., Roy, C., Ayer, A., Toutain, B., et al. (2016). Central role of P2Y6 UDP receptor in arteriolar myogenic tone. Arterioscler. Thromb. Vasc. Biol. 36, 1598–1606. doi: 10.1161/ATVBAHA.116.307739
Knot, H. J., and Nelson, M. T. (1998). Regulation of arterial diameter and wall Ca2+ in cerebral arteries of rat by membrane potential and intravascular pressure. J. Physiol. 508, 199–209. doi: 10.1111/j.1469-7793.1998.199br.x
Kotecha, N., and Hill, M. A. (2005). Myogenic contraction in rat skeletal muscle arterioles: smooth muscle membrane potential and Ca2+ signaling. Am. J. Physiol. Heart Circ. Physiol. 289, H1326–H1334. doi: 10.1152/ajpheart.00323.2005
Kroetsch, J. T., Levy, A. S., Zhang, H., Aschar-Sobbi, R., Lidington, D., Offermanns, S., et al. (2017). Constitutive smooth muscle tumour necrosis factor regulates microvascular myogenic responsiveness and systemic blood pressure. Nat. Commun. 8:14805. doi: 10.1038/ncomms14805
Lagaud, G., Gaudreault, N., Moore, E. D., Van Breemen, C., and Laher, I. (2002). Pressure-dependent myogenic constriction of cerebral arteries occurs independently of voltage-dependent activation. Am. J. Physiol. Heart Circ. Physiol. 283, H2187–H2195. doi: 10.1152/ajpheart.00554.2002
Laughlin, M. H., Korthuis, R. J., Duncker, D. J., and Bache, R. J. (1996). Control of Blood Flow to Cardiac and Skeletal Muscle During Exercise. New York: Published for the American Physiological Society by Oxford University Press.
Leiphart, R. J., Chen, D., Peredo, A. P., Loneker, A. E., and Janmey, P. A. (2019). Mechanosensing at cellular interfaces. Langmuir 35, 7509–7519. doi: 10.1021/acs.langmuir.8b02841
Li, Y., and Brayden, J. E. (2017). Rho kinase activity governs arteriolar myogenic depolarization. J. Cereb. Blood Flow Metab. 37, 140–152. doi: 10.1177/0271678X15621069
Lidington, D., Peter, B. F., Meissner, A., Kroetsch, J. T., Pitson, S. M., Pohl, U., et al. (2009). The phosphorylation motif at serine 225 governs the localization and function of sphingosine kinase 1 in resistance arteries. Arterioscler. Thromb. Vasc. Biol. 29, 1916–1922. doi: 10.1161/ATVBAHA.109.194803
Loirand, G., Guerin, P., and Pacaud, P. (2006). Rho kinases in cardiovascular physiology and pathophysiology. Circ. Res. 98, 322–334. doi: 10.1161/01.RES.0000201960.04223.3c
Lucchesi, P. A., Sabri, A., Belmadani, S., and Matrougui, K. (2004). Involvement of metalloproteinases 2/9 in epidermal growth factor receptor transactivation in pressure-induced myogenic tone in mouse mesenteric resistance arteries. Circulation 110, 3587–3593. doi: 10.1161/01.CIR.0000148780.36121.47
Luykenaar, K. D., El-Rahman, R. A., Walsh, M. P., and Welsh, D. G. (2009). Rho-kinase-mediated suppression of KDR current in cerebral arteries requires an intact actin cytoskeleton. Am. J. Physiol. Heart Circ. Physiol. 296, H917–H926. doi: 10.1152/ajpheart.01206.2008
Martinez-Lemus, L. A., Crow, T., Davis, M. J., and Meininger, G. A. (2005). alphavbeta3- and alpha5beta1-integrin blockade inhibits myogenic constriction of skeletal muscle resistance arterioles. Am. J. Physiol. Heart Circ. Physiol. 289, H322–H329. doi: 10.1152/ajpheart.00923.2003
Martinez-Lemus, L. A., Hill, M. A., Bolz, S. S., Pohl, U., and Meininger, G. A. (2004). Acute mechanoadaptation of vascular smooth muscle cells in response to continuous arteriolar vasoconstriction: implications for functional remodeling. FASEB J. 18, 708–710. doi: 10.1096/fj.03-0634fje
McGahon, M. K., Fernandez, J. A., Dash, D. P., McKee, J., Simpson, D. A., Zholos, A. V., et al. (2016). TRPV2 channels contribute to stretch-activated cation currents and myogenic constriction in retinal arterioles. Invest. Ophthalmol. Vis. Sci. 57, 5637–5647. doi: 10.1167/iovs.16-20279
Mederos, Y. S. M., Storch, U., and Gudermann, T. (2016). Mechanosensitive Gq/11 protein-coupled receptors mediate myogenic vasoconstriction. Microcirculation 23, 621–625. doi: 10.1111/micc.12293
Mederos y Schnitzler, M., Storch, U., Meibers, S., Nurwakagari, P., Breit, A., Essin, K., et al. (2008). Gq-coupled receptors as mechanosensors mediating myogenic vasoconstriction. EMBO J. 27, 3092–3103. doi: 10.1038/emboj.2008.233
Meininger, G. A., and Trzeciakowski, J. P. (1988). Vasoconstriction is amplified by autoregulation during vasoconstrictor-induced hypertension. Am. J. Phys. 254, H709–H718. doi: 10.1152/ajpheart.1988.254.4.H709
Meininger, G. A., and Trzeciakowski, J. P. (1990). Combined effects of autoregulation and vasoconstrictors on hindquarters vascular resistance. Am. J. Phys. 258, H1032–H1041. doi: 10.1152/ajpheart.1990.258.4.H1032
Moosmang, S., Schulla, V., Welling, A., Feil, R., Feil, S., Wegener, J. W., et al. (2003). Dominant role of smooth muscle L-type calcium channel Cav1.2 for blood pressure regulation. EMBO J. 22, 6027–6034. doi: 10.1093/emboj/cdg583
Moreno-Dominguez, A., Colinas, O., El-Yazbi, A., Walsh, E. J., Hill, M. A., Walsh, M. P., et al. (2013). Ca2+ sensitization due to myosin light chain phosphatase inhibition and cytoskeletal reorganization in the myogenic response of skeletal muscle resistance arteries. J. Physiol. 591, 1235–1250. doi: 10.1113/jphysiol.2012.243576
Mortensen, S. P., and Saltin, B. (2014). Regulation of the skeletal muscle blood flow in humans. Exp. Physiol. 99, 1552–1558. doi: 10.1113/expphysiol.2014.081620
Navar, L. G., Arendshorst, W. J., Pallone, T. L., Inscho, E. W., Imig, J. D., and Bell, P. D. (2011). “The renal microcirculation,” in Comprehensive Physiology. ed. R. L. Terjung (Hoboken, NJ: John Wiley & Sons, Inc.), 550–683.
Nelson, M. T., Cheng, H., Rubart, M., Santana, L. F., Bonev, A. D., Knot, H. J., et al. (1995). Relaxation of arterial smooth muscle by calcium sparks. Science 270, 633–637. doi: 10.1126/science.270.5236.633
Nemeth, Z., Hildebrandt, E., Ryan, M. J., Granger, J. P., and Drummond, H. A. (2020). Pressure-induced constriction of the middle cerebral artery is abolished in TrpC6 knockout mice. Am. J. Physiol. Heart Circ. Physiol. 319, H42–H50. doi: 10.1152/ajpheart.00126.2020
Ngo, A. T., Riemann, M., Holstein-Rathlou, N. H., Torp-Pedersen, C., and Jensen, L. J. (2013). Significance of K(ATP) channels, L-type Ca2+ channels and CYP450-4A enzymes in oxygen sensing in mouse cremaster muscle arterioles in vivo. BMC Physiol. 13:8. doi: 10.1186/1472-6793-13-8
Pemberton, M., Anderson, G. L., and Barker, J. H. (1996). Characterization of microvascular vasoconstriction following ischemia/reperfusion in skeletal muscle using videomicroscopy. Microsurgery 17, 9–16. doi: 10.1002/(SICI)1098-2752(1996)17:1<9::AID-MICR2>3.0.CO;2-K
Peter, B. F., Lidington, D., Harada, A., Bolz, H. J., Vogel, L., Heximer, S., et al. (2008). Role of sphingosine-1-phosphate phosphohydrolase 1 in the regulation of resistance artery tone. Circ. Res. 103, 315–324. doi: 10.1161/CIRCRESAHA.108.173575
Pries, A. R., and Secomb, T. W. (2011). “Blood flow in microvascular networks,” in Comprehensive Physiology. ed. R. L. Terjung (Hoboken, NJ: John Wiley & Sons, Inc.), 3–36.
Pires, P. W., Ko, E. A., Pritchard, H. A. T., Rudokas, M., Yamasaki, E., and Earley, S. (2017). The angiotensin II receptor type 1b is the primary sensor of intraluminal pressure in cerebral artery smooth muscle cells. J. Physiol. 595, 4735–4753. doi: 10.1113/JP274310
Renkin, E. M. (1984). “Control of microcirculation and blood-tissue exchange,” in Handbook of Physiology: Section 2, The Cardiovascular System, Vol. IV, Microcirculation, part 2. eds. E. M. Renkin and C. C. Michel (Bethesda, MD: American Physiological Society), 627–687.
Riva, C. E., and Schmetterer, L. (2011). “Microcirculation of the ocular fundus,” in Comprehensive Physiology. ed. R. L. Terjung (Hoboken, NJ: John Wiley & Sons, Inc.), 735–765.
Saltin, B., Radegran, G., Koskolou, M. D., and Roach, R. C. (1998). Skeletal muscle blood flow in humans and its regulation during exercise. Acta Physiol. Scand. 162, 421–436. doi: 10.1046/j.1365-201X.1998.0293e.x
Sauve, M., Hui, S. K., Dinh, D. D., Foltz, W. D., Momen, A., Nedospasov, S. A., et al. (2016). Tumor necrosis factor/sphingosine-1-phosphate signaling augments resistance artery myogenic tone in diabetes. Diabetes 65, 1916–1928. doi: 10.2337/db15-1450
Schleifenbaum, J., Kassmann, M., Szijarto, I. A., Hercule, H. C., Tano, J. Y., Weinert, S., et al. (2014). Stretch-activation of angiotensin II type 1a receptors contributes to the myogenic response of mouse mesenteric and renal arteries. Circ. Res. 115, 263–272. doi: 10.1161/CIRCRESAHA.115.302882
Segal, S. S. (2000). Integration of blood flow control to skeletal muscle: key role of feed arteries. Acta Physiol. Scand. 168, 511–518. doi: 10.1046/j.1365-201x.2000.00703.x
Segal, S. S., and Duling, B. R. (1986). Communication between feed arteries and microvessels in hamster striated-muscle—segmental vascular-responses are functionally coordinated. Circ. Res. 59, 283–290. doi: 10.1161/01.RES.59.3.283
Shepherd, J. T. (1983). “Circulation to skeletal muscle,” in Handbook of Physiology, Section 2, The Cardiovascular System, Volume III. eds. E. M. Renkin and C. C. Michel (Bethesda, MD: American Physiological Society), 319–370.
Soni, H., Peixoto-Neves, D., Matthews, A. T., and Adebiyi, A. (2017). TRPV4 channels contribute to renal myogenic autoregulation in neonatal pigs. Am. J. Physiol. Ren. Physiol. 313, F1136–F1148. doi: 10.1152/ajprenal.00300.2017
Spassova, M. A., Hewavitharana, T., Xu, W., Soboloff, J., and Gill, D. L. (2006). A common mechanism underlies stretch activation and receptor activation of TRPC6 channels. Proc. Natl. Acad. Sci. U. S. A. 103, 16586–16591. doi: 10.1073/pnas.0606894103
Storch, U., Blodow, S., Gudermann, T., and Mederos, Y. S. M. (2015). Cysteinyl leukotriene 1 receptors as novel mechanosensors mediating myogenic tone together with angiotensin II type 1 receptors-brief report. Arterioscler. Thromb. Vasc. Biol. 35, 121–126. doi: 10.1161/ATVBAHA.114.304844
Storch, U., Mederos y Schnitzler, M., and Gudermann, T. (2012). G protein-mediated stretch reception. Am. J. Physiol. Heart Circ. Physiol. 302, H1241–H1249. doi: 10.1152/ajpheart.00818.2011
Suzuki, Y., Yamamura, H., Ohya, S., and Imaizumi, Y. (2013). Caveolin-1 facilitates the direct coupling between large conductance Ca2+−activated K+ (BKCa) and Cav1.2 Ca2+ channels and their clustering to regulate membrane excitability in vascular myocytes. J. Biol. Chem. 288, 36750–36761. doi: 10.1074/jbc.M113.511485
Tuma, R. F. (2011). “The cerebral microcirculation,” in Comprehensive Physiology. ed. R. L. Terjung (Hoboken, NJ: John Wiley & Sons, Inc.), 485–520.
Tykocki, N. R., Boerman, E. M., and Jackson, W. F. (2017). Smooth muscle ion channels and regulation of vascular tone in resistance arteries and arterioles. Compr. Physiol. 7, 485–581. doi: 10.1002/cphy.c160011
VanLandingham, L. G., Gannon, K. P., and Drummond, H. A. (2009). Pressure-induced constriction is inhibited in a mouse model of reduced betaENaC. Am. J. Phys. Regul. Integr. Comp. Phys. 297, R723–R728. doi: 10.1152/ajpregu.00212.2009
Wang, Q., Leo, M. D., Narayanan, D., Kuruvilla, K. P., and Jaggar, J. H. (2016). Local coupling of TRPC6 to ANO1/TMEM16A channels in smooth muscle cells amplifies vasoconstriction in cerebral arteries. Am. J. Phys. Cell Physiol. 310, C1001–C1009. doi: 10.1152/ajpcell.00092.2016
Welsh, D. G., Jackson, W. F., and Segal, S. S. (1998). Oxygen induces electromechanical coupling in arteriolar smooth muscle cells: a role for L-type Ca2+ channels. Am. J. Phys. 274, H2018–H2024. doi: 10.1152/ajpheart.1998.274.6.H2018
Welsh, D. G., Morielli, A. D., Nelson, M. T., and Brayden, J. E. (2002). Transient receptor potential channels regulate myogenic tone of resistance arteries. Circ. Res. 90, 248–250. doi: 10.1161/hh0302.105662
Westcott, E. B., Goodwin, E. L., Segal, S. S., and Jackson, W. F. (2012). Function and expression of ryanodine receptors and inositol 1,4,5-trisphosphate receptors in smooth muscle cells of murine feed arteries and arterioles. J. Physiol. 590, 1849–1869. doi: 10.1113/jphysiol.2011.222083
Westcott, E. B., and Jackson, W. F. (2011). Heterogeneous function of ryanodine receptors, but not IP3 receptors, in hamster cremaster muscle feed arteries and arterioles. Am. J. Physiol. Heart Circ. Physiol. 300, H1616–H1630. doi: 10.1152/ajpheart.00728.2010
Wilson, P. C., Fitzgibbon, W. R., Garrett, S. M., Jaffa, A. A., Luttrell, L. M., Brands, M. W., et al. (2015). Inhibition of sphingosine kinase 1 ameliorates angiotensin II-induced hypertension and inhibits transmembrane calcium entry via store-operated calcium channel. Mol. Endocrinol. 29, 896–908. doi: 10.1210/me.2014-1388
Yagi, K., Lidington, D., Wan, H., Fares, J. C., Meissner, A., Sumiyoshi, M., et al. (2015). Therapeutically targeting tumor necrosis factor-alpha/sphingosine-1-phosphate signaling corrects myogenic reactivity in subarachnoid hemorrhage. Stroke 46, 2260–2270. doi: 10.1161/STROKEAHA.114.006365
Yang, J., Noyan-Ashraf, M. H., Meissner, A., Voigtlaender-Bolz, J., Kroetsch, J. T., Foltz, W., et al. (2012). Proximal cerebral arteries develop myogenic responsiveness in heart failure via tumor necrosis factor-alpha-dependent activation of sphingosine-1-phosphate signaling. Circulation 126, 196–206. doi: 10.1161/CIRCULATIONAHA.111.039644
Yasuda, N., Miura, S., Akazawa, H., Tanaka, T., Qin, Y., Kiya, Y., et al. (2008). Conformational switch of angiotensin II type 1 receptor underlying mechanical stress-induced activation. EMBO Rep. 9, 179–186. doi: 10.1038/sj.embor.7401157
Zhang, C., Rogers, P. A., Merkus, D., Muller-Delp, J. M., Tiefenbacher, C. P., Potter, B., et al. (2011). “Regulation of coronary microvascular resistance in health and disease,” in Comprehensive Physiology. ed. R. L. Terjung (Hoboken, NJ: John Wiley & Sons, Inc.), 521–549.
Zou, H., Ratz, P. H., and Hill, M. A. (1995). Role of myosin phosphorylation and [Ca2+]i in myogenic reactivity and arteriolar tone. Am. J. Phys. 269, H1590–H1596. doi: 10.1152/ajpheart.1995.269.5.H1590
Keywords: myogenic tone, arterioles, resistance arteries, ion channels, G-proteins, mechanotransduction
Citation: Jackson WF (2021) Myogenic Tone in Peripheral Resistance Arteries and Arterioles: The Pressure Is On! Front. Physiol. 12:699517. doi: 10.3389/fphys.2021.699517
Edited by:
Markus Hecker, Heidelberg University, GermanyReviewed by:
Gerald A. Meininger, University of Missouri, United StatesCor de Wit, University of Lübeck, Germany
Timothy W. Secomb, University of Arizona, United States
Christian Aalkjaer, Aarhus University, Denmark
Copyright © 2021 Jackson. This is an open-access article distributed under the terms of the Creative Commons Attribution License (CC BY). The use, distribution or reproduction in other forums is permitted, provided the original author(s) and the copyright owner(s) are credited and that the original publication in this journal is cited, in accordance with accepted academic practice. No use, distribution or reproduction is permitted which does not comply with these terms.
*Correspondence: William F. Jackson, amFja3M3ODNAbXN1LmVkdQ==