- 1Department of Animal Sciences, The Ohio State University, Columbus, OH, United States
- 2The Ohio State University Interdisciplinary Human Nutrition Program, The Ohio State University, Columbus, OH, United States
- 3Department of Veterinary Biosciences, College of Veterinary Medicine, The Ohio State University, Columbus, OH, United States
- 4Animal Bioscience and Biotechnology Laboratory, Beltsville Agricultural Research Center, Agricultural Research Service, United States Department of Agriculture, Beltsville, MD, United States
Excessive adipose accretion causes health issues in humans and decreases feed efficiency in poultry. Although vitamin A has been known to be involved in adipogenesis, effects of all-trans retinoic acid (atRA), as a metabolite of vitamin A, on embryonic adipose development have not been studied yet. Avian embryos are developing in confined egg environments, which can be directly modified to study effects of nutrients on embryonic adipogenesis. With the use of quail embryos, different concentrations of atRA (0 M to 10 μM) were injected in ovo at embryonic day (E) 9, and adipose tissues were sampled at E14. Percentages of fat pad weights in embryo weights were significantly increased in the group injected with 300 nM of atRA. Also, among three injection time points, E5, E7, or E9, E7 showed the most significant increase in weight and percentage of inguinal fat at E14. Injection of atRA at E7 increased fat cell size in E14 embryos with up-regulation of pro-adipogenic marker genes (Pparγ and Fabp4) and down-regulation of a preadipocyte marker gene (Dlk1) in adipose tissues. These data demonstrate that atRA promotes hypertrophic fat accretion in quail embryos, implying important roles of atRA in embryonic development of adipose tissues.
Introduction
Excessive fat accretion causes obesity and associated diseases in humans. On the agricultural side, excessive fat is undesirable due to economic concerns of animal producers on low feed efficiency associated with fat accretion and health issues of consumers on high fat contents in meat. Discovery and understanding of roles of genetic and nutritional factors in fat regulation will lead to improving health and efficiency of animal production. Different from mammals, avian species depend on the various nutrient contents in the fertile egg for growth and development of embryos. During embryonic development of the chicken, adipose tissues begin to be visible from embryonic day (E) 10 and grown through hyperplasia and hypertrophy (Chen et al., 2014). Chicken embryos at E10–E12 have preadipocytes and multilocular immature fat cells (Chen et al., 2014). These cells are further developed toward more numbers of unilocular fat cells at E14 (Chen et al., 2014). This study showed how rapidly adipocytes are developed during embryonic development.
Vitamin A (retinol) is an essential nutrient in animals and involved in biological processes of adipose development. Retinol can be converted to all-trans retinoic acid (atRA), which functions as a key regulatory factor in embryonic development by controlling differentiation of many cell types including adipocytes. Previous studies showed that supplementation of high doses of atRA (over 1 μM) on 3T3-L1 cells blocked lipid accumulation (Mercader et al., 2007; Stoecker et al., 2017), but the supplementation of low concentration (10 nM) had pro-adipogenic effects on Ob1771 cells (Safonova et al., 1994). Our previous study also showed that atRA functions as a positive or negative regulator on adipogenic differentiation of 3T3-L1 cells by supplementation of mild or excessive doses, respectively (Kim et al., 2019). Levels of serum retinol were higher in obese children at the ages of 6–14 years (Aeberli et al., 2007). Supplementation of high levels of dietary vitamin A in obese rats significantly reduced body weight gain and adipose tissue weight (Jeyakumar et al., 2008). In addition, injection of atRA into mice reduced fat mass (Felipe et al., 2005; Mercader et al., 2006), but injection of retinol into new-born Angus cattle increased the ratio of intra-muscular fat and marbling score (Harris et al., 2018). However, roles of retinoids in fetal adipose development have not been reported. This might be due to potential difficulties in precisely modulating fetal retinoids such as an uncertain relationship of maternal retinoid levels with fetal levels or issues in direct delivery into fetus.
Avian species are suitable models for the research of developmental origins of health and disease because nutritional, hormonal, and chemical exposures can be strictly modulated by directly injecting exogenous factors into embryos at specific developmental stages, which cannot be easily accomplished in mammalian embryos. In poultry, adipogenic differentiation in vitro can be induced by supplementation of atRA (Kim et al., 2020a,b; Lee et al., 2021). Dietary supplementation of vitamin A during early post-hatch period increased fat accretion in broiler chickens (Savaris et al., 2020). The present study aims to investigate the cellular effects of atRA on adipose growth in quail embryos as an animal model.
Materials and Methods
Visualization of Embryonic Fat Pads
As described in our previous study (Chen et al., 2014), to visualize fat pads, quail embryo was removed from the egg, and feathers were removed by using forceps during washing with phosphate-buffered saline (PBS) three times. Then, to visualize the fat pads, the embryo was transferred into a 1% potassium hydroxide (KOH) solution after fixing in 70% ethanol because KOH, as a strong alkali, makes tissue soften, digest, and clear. After the skin was cleared, the embryos were visualized using a camera (EOS Rebel T7, Canon, Japan).
In ovo Injection and Tissue Sampling
Experiments using poultry embryos are exempt from requiring University Institutional Animal Care and Use Committee approval, because avian embryos are not considered live animals by the Public Health Service Policy (ILAR News, 1991). All fertile eggs of Japanese quail were obtained from The Ohio State University poultry research farm. atRA (#R2625, Sigma-Aldrich, St. Louis, MO, United States) was diluted with dimethyl sulfoxide (DMSO). Before atRA was injected in ovo, the surface of fertile eggs that were confirmed by candling was cleaned with 70% ethanol. Eggshell at the two-thirds point of quail eggs was grinded by twisting with corner tip of blades (#4515, Ettore, Alameda, CA, United States) without disrupting the shell membrane. Two microliters of different concentrations of atRA (final concentrations: 100 nM to 10 μM) was injected through the shell membrane using a micropipette tip (#17000504, Rainin, Columbus, OH, United States), as shown in Figure 2A, at different embryonic ages (E5, E7, or E9) and then sealed with parafilm. The injected eggs were incubated at 37.5°C with 60% relative humidity until sampling inguinal fat pads at E12 or E14. DMSO was injected as a control (0 M).
Histological Processing and Measurement of Fat Cell Size
Inguinal fat pads were 1fixed in 10% neutral buffered formalin for 48 h and then processed to embed in paraffin. Paraffin embedded fat tissues were cut into 5-μm slices. To measure fat cell size, serial sections of the embedded samples were de-paraffinized in xylene, re-hydrated in serial diluted ethanol, and then stained with hematoxylin and eosin (H&E). Stained slides were observed and imaged under a microscope (EVOS cell imaging system, Thermo Fisher Scientific, Waltham, MA, United States). ImageJ software (NIH ImageJ 1.52s) was used to determine fat cell size. The average of the adipocyte cross-sectional area was calculated by measuring randomly selected areas having large numbers of cells and dividing this by the total number of cells found within the area. At least 800 cells at E12 and 500 cells at E14 were evaluated per animal.
Analysis of Gene Expression
After extraction of total RNA from inguinal fat tissues and cDNA synthesis by the methods described in our previous study (Kim et al., 2020a), quantitative PCR (qPCR) was performed in duplicate per sample to analyze gene expression associated with adipogenesis with specific primer sets; Peroxisome proliferator-activated receptor gamma (Pparγ, NCBI Reference Sequence: NM_001001460.1, F: 5′-GTGAATCTTGACCTGAATGATC AGGT, R: 5′-AGATTATCTTGTATATCTTCAATGGGCTTCA CAT), fatty acid-binding protein 4 (Fabp4, NCBI Reference Sequence: XM_015855897.2, F: 5′-CAAGCTGGGTGAAGAGT TTGATG, R: 5′-CTCTTTTGCTGGTAACATTATTCATGGT GCA), and Delta like non-canonical notch ligand 1 (Dlk1, NCBI Reference Sequence: XM_032445023.1, F: 5′-CTGCCATCT CAGGAAAGGACC, R: 5′-ACATGGGTTGGATTCACAGTC ATC). Glyceraldehyde 3-phosphate dehydrogenase (Gapdh, NCBI Reference Sequence: NM_204305.1, F: 5′-CTCTGTTGTTGAC CTGACCTG, R: 5′-CAAGTCCACAACACGGTTGCT) was used as a reference gene. The expression levels were normalized to those of genes by the 0 M group at E12, and all of the data were analyzed using the ddCt method (Livak and Schmittgen, 2001).
Statistical Analysis
All data were expressed as means ± SEM. The data were analyzed by the Student or multiple t-test using GraphPad Prism software, version 6.02. p < 0.05 was considered as a statistically significant difference.
Results
Visualization of Developing Adipose Tissues at Different Embryonic Ages
Although adipose tissue in chicken embryos appears around E10 (Chen et al., 2014), embryonic ontogeny of adipose tissues in quail has not been reported yet. Therefore, to investigate the developmental stages of adipose growth during embryonic ages, ontogeny of embryonic adipose development was studied. When adipose tissues begin to appear, they are too small to be visible and distinguished from other tissues. Therefore, intact fat pads in the embryo were visualized using KOH that enables soft tissues transparent by alkaline hydrolysis of proteins and fat tissues distinguishably visible by saponification of lipids (Culling et al., 1974). This method can be applied for visualization of fat pads in various species.
The current study showed that adipose tissues in quail embryos were not found at E8 but initially appeared around the neck at E9 (Figure 1). Interestingly, the appearance of fat pads coincided with the time of feather development at E9 in quail and E10 in chickens (Hamburger and Hamilton, 1951; Chen et al., 2014; Nakamura et al., 2019), indicating that fat and feather development might initiate at similar developmental stages. At E10, fat pads around the neck were extended more but did not appear in other areas (Figure 1). In addition to neck fat pads, supraclavicular and inguinal fat pads first appeared at E11, and breast and leg fat pads were additionally shown from E12 (Figure 1).
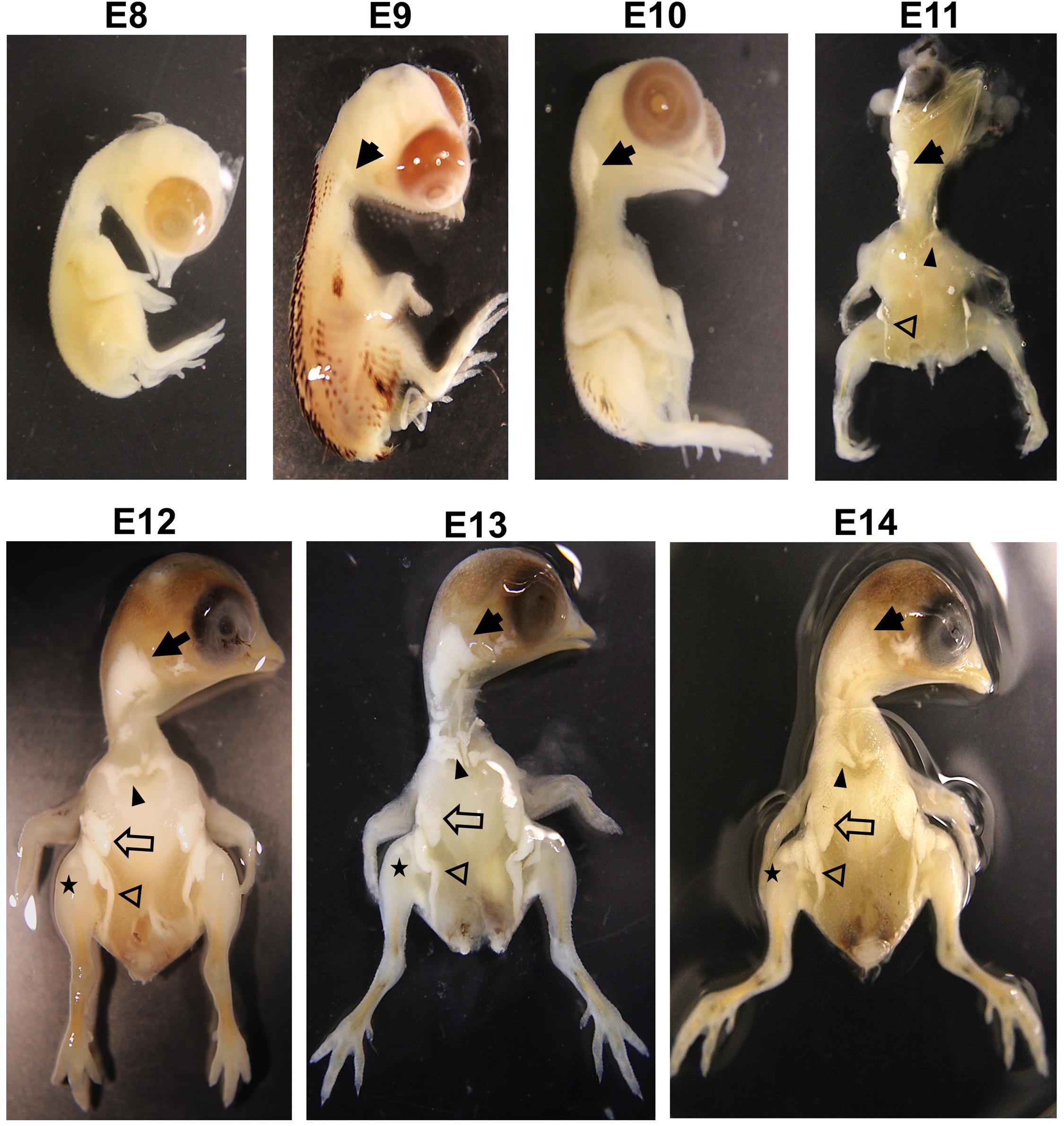
Figure 1. Visualization of fat pad on embryos in quail with 1% KOH at E8, E9, E10, E11, E12, E13, and E14. Solid arrow, neck fat; solid arrowhead, supraclavicular fat; opened arrow, breast fat; opened arrowhead, inguinal fat; star, leg fat. Images are not to scale.
Effect of in ovo Injection With Different Concentrations of All-Trans Retinoic Acid on Adipose Weight
Based on the ontogenetic data showing an appearance of adipose tissues in the neck area from E9, different dosages of atRA (final concentration: 0 M, 100 nM, 300 nM, 1 μM, 3 μM, or 10 μM) were injected into quail eggs at E9 to investigate the effect of atRA on embryonic development of adipose tissues (Figure 2A). Embryos injected with atRA were sampled at E14 to measure embryo weight (EW) and weight of inguinal fat tissues (Figure 2A). Although there was no difference in EW among all groups (Figure 2B), the percentages of weight of inguinal fat tissues in EW were gradually increased with increasing concentrations of atRA up to 300 nM and somewhat reduced at higher concentrations from 300 nM to 10 μM (Figure 2C). Especially, the group injected with 300 nM atRA showed a significantly increased percentage of inguinal fat tissue compared with those at the 0 M (1.25-fold, p < 0.05) (Figure 2C). Thus, the current data indicate that adipose growth can be influenced by atRA.
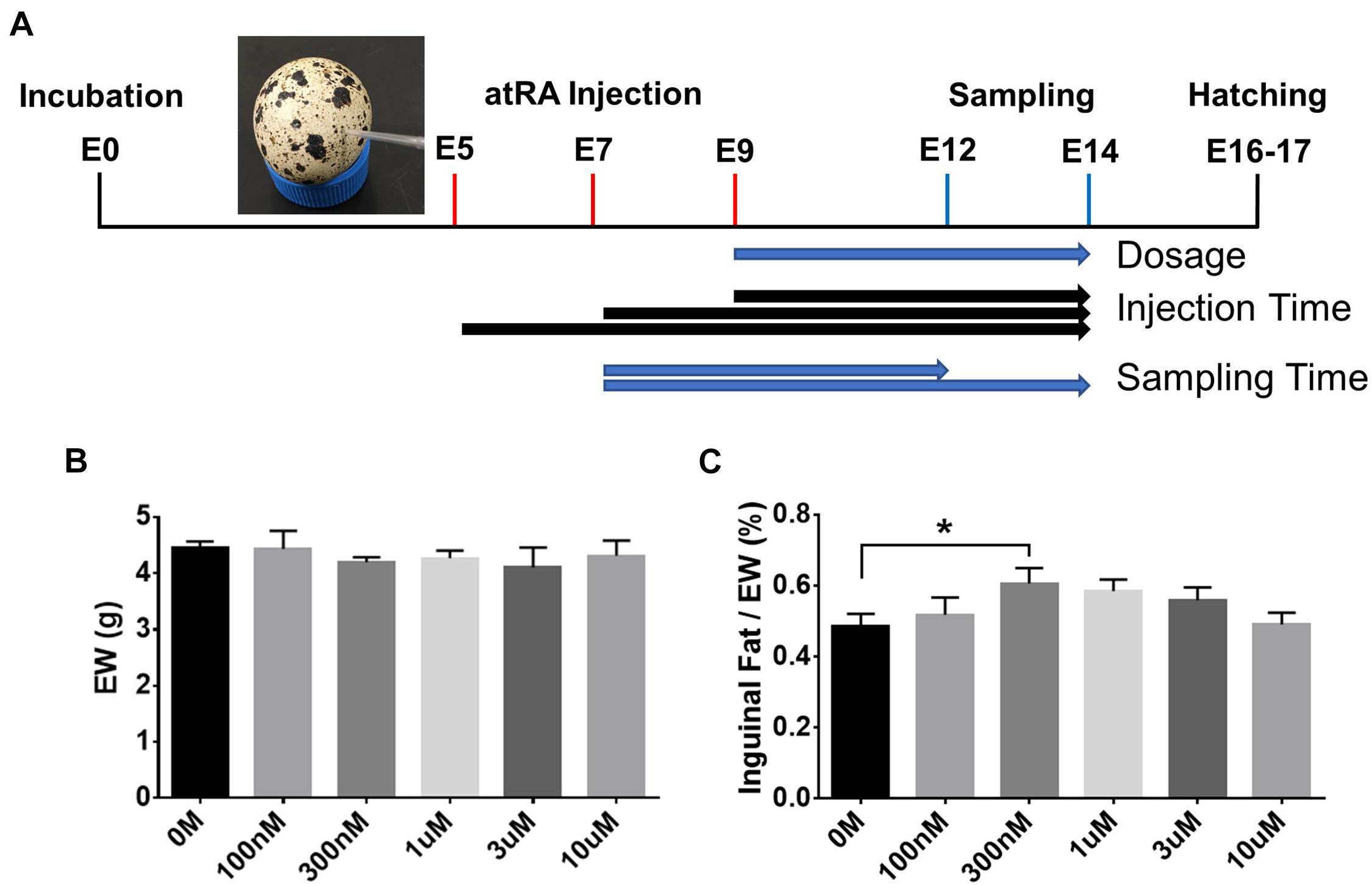
Figure 2. In ovo all-trans retinoic acid injection. (A) Schematic diagram of in ovo all-trans retinoic acid (atRA) injection. Quail eggs were injected with atRA at embryonic day (E) 5, E7, or E9 and sampled at E12 or E14. Embryo weight (EW, B) and percentages of inguinal fat weight in total EW (C). Dose of 0 M, 100 nM, 300 nM, 1 μM, 3 μM, or 10 μM of atRA was injected in ovo at E9, and injected embryos were sampled at E14. n = 11, 7, 12, 12, 5, and 7 embryos for 0 M, 100 nM, 300 nM, 1 μM, 3 μM and 10 μM of atRA, respectively. All data were expressed as means ± SEM. The data were analyzed by multiple t-test using GraphPad Prism software, version 6.02. *p < 0.05.
Effect of in ovo Injection of All-Trans Retinoic Acid at Different Embryonic Ages on Adipose Weight
To investigate to what extent atRA injection at three different ages, before (E5 or 7) and after (E9) appearance of adipose tissues, affects adipose growth, atRA was injected into eggs at those ages and adipose tissues were sampled at E14 (Figure 3). Weights of embryos at E14 were not affected by 300 nM of atRA injection at all three ages (Figure 3A). Although weights of inguinal fat pads were significantly increased by atRA injection at E5 (p < 0.05) and E7 (p < 0.01), percentages of inguinal fat weights in EW were significantly increased by injecting at all time points (E5: 1.45-fold, p < 0.01; E7: 1.32-fold, p < 0.01; E9: 1.25-fold, p < 0.05) (Figure 3C). These data demonstrated that injecting atRA before the appearance of fat pads is more effective in enhancing embryonic adipose growth.
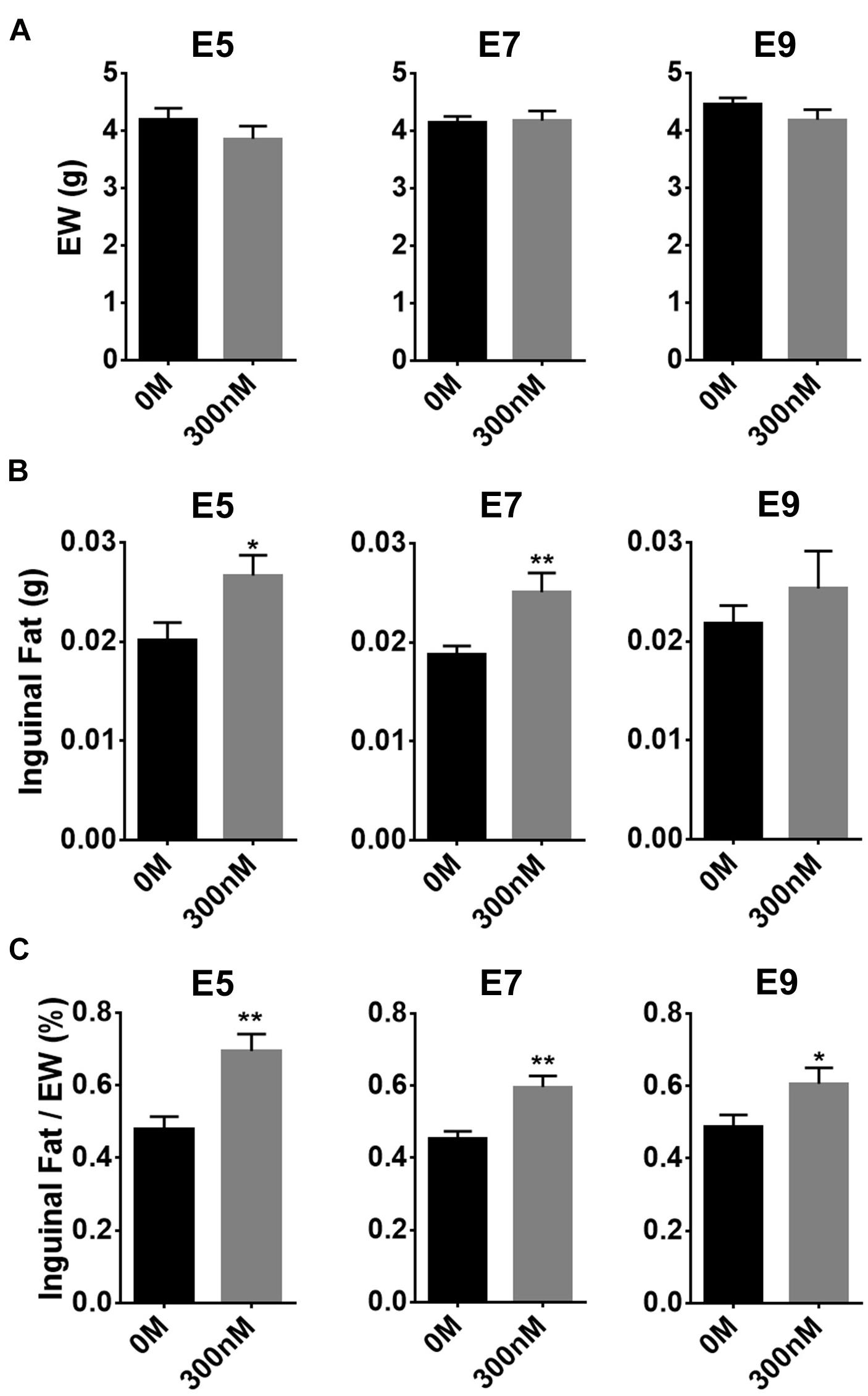
Figure 3. Effect of time of in ovo all-trans retinoic acid (atRA) injection. Embryo weight (EW) (A), inguinal fat pad weights (B), and percentages of weights of inguinal fat tissues in EW (C). Both 0-M and 300-nM concentrations of atRA were injected in ovo at embryonic day (E) 5, E7, or E9; and injected embryos were sampled at E14. For 0 M or 300 nM of atRA, n = 8 and n = 8 embryos at E5, n = 9 and n = 8 embryos at E7, and n = 11 and n = 12 embryos at E9, respectively. All data were expressed as means ± SEM. The data were analyzed by a Student t-test using GraphPad Prism software, version 6.02. *p < 0.05, **p < 0.01.
Hypertrophic Effect of All-Trans Retinoic Acid in Fat Tissues
To determine whether the increased fat pad weights by atRA can be attributed to increasing cell size, embryos were sampled at E12 or E14 after injecting atRA at E7, and a histological examination was performed to measure sizes of fat cells. Injection of atRA at E7 resulted in increased percentages of inguinal fat weights in embryos at E12 and E14 (E12: 1.24-fold, p < 0.05; E14: 1.32-fold, p < 0.01) (Figure 4A). The sizes of fat cells at E12 were not significantly different between the two groups (0 M and 300 nM) (Figures 4B,C). In the samples that were injected with 300 nM of atRA at E7 and sampled at E14, size of inguinal fat cells was significantly increased compared with the 0 M (1.5-fold, p < 0.05) (Figures 4B,C). These data clearly showed that atRA injection resulted in increased fat pad weights and cell sizes during embryonic development in quail.
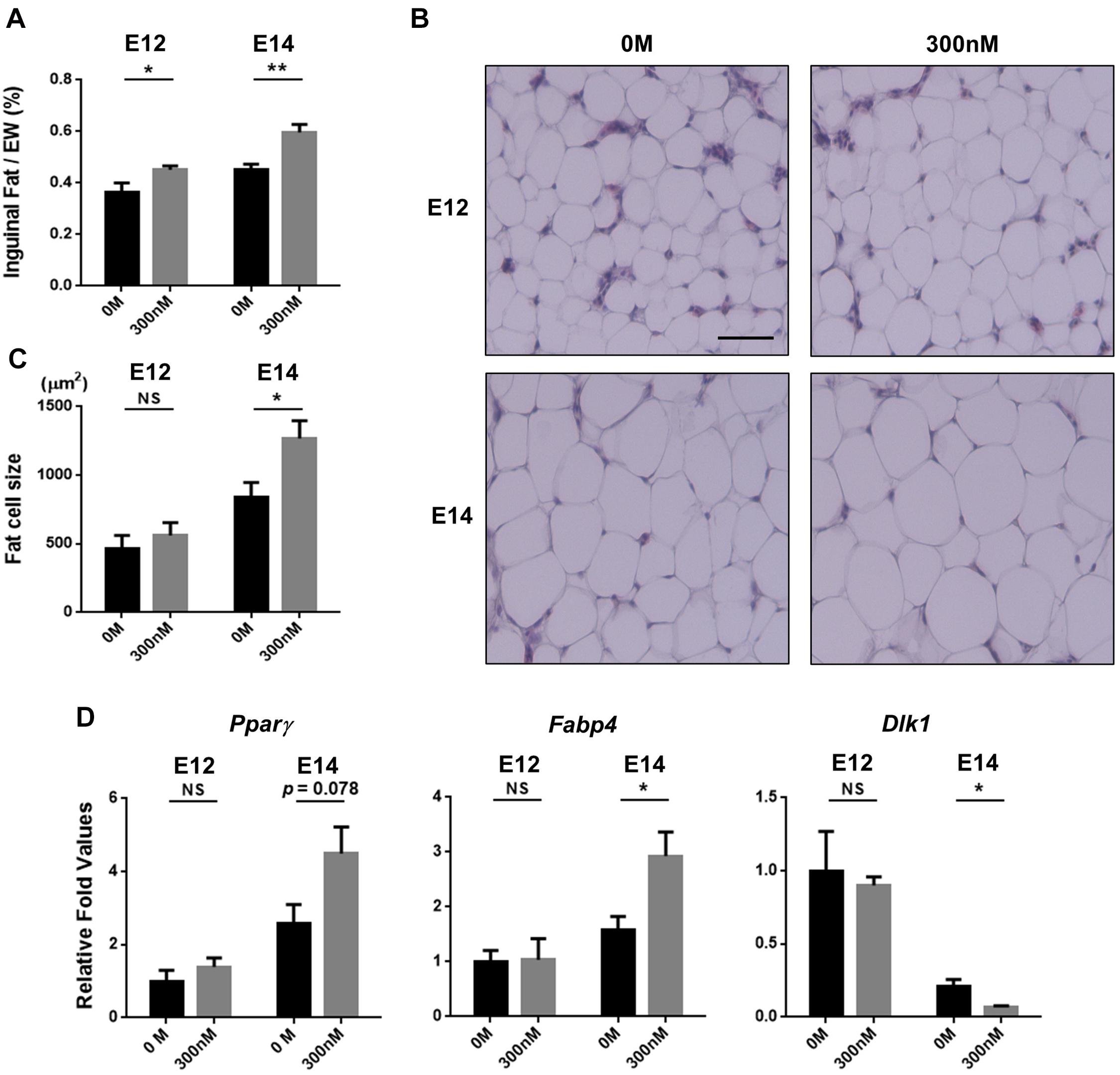
Figure 4. Hypertrophic fat cells by in ovo injection of all-trans retinoic acid (atRA). Percentages of inguinal fat weights in embryo weight (EW) (A). Embryos were injected with 0 M or 300 nM of atRA at E7 and sampled at E12 or E14. For 0 M or 300 nM of atRA, n = 9 and n = 9 embryos at E12 and n = 9 and n = 8 embryos at E14, respectively. Scale bar: 100 μm. H&E stain of inguinal fat tissue (B) and comparisons of fat cell size (C). Quantitative analysis of gene expression involved in adipogenesis by qPCR (D). n = 4 for 0 M and 300 nM of atRA at E12 and E14, respectively. All data were expressed as means ± SEM. The data were analyzed by Student t-test using GraphPad Prism software, version 6.02. NS, no significance; *p < 0.05, **p < 0.01.
Expression Levels of Genes Involved in Adipogenesis
To further investigate effects of atRA on expression of adipogenic marker genes in embryonic days, expression levels of marker genes for preadipocytes (Dlk1) and adipocytes (Pparγ and Fabp4) were analyzed. Injection of 300 nM atRA at E7 enhanced expression levels of Pparγ in E14 inguinal adipose tissues by 75% compared with the 0 M, although the difference was not statistically significant (p = 0.078) (Figure 4D). Expression of Fabp4 in E14 adipose tissues was significantly up-regulated (85%, p < 0.05) by the atRA injection at E7 compared with the 0 M (Figure 4D). In addition, atRA injection at E7 significantly down-regulated expression levels of Dlk1 by 68% (p < 0.05) in the adipose tissues at E14 compared with the 0 M (Figure 4D). These results indicate that in ovo injection of atRA caused enhanced expression of adipogenic markers and reduced expression of the preadipocyte marker, suggesting promotion of embryonic adipogenesis by injecting atRA in ovo in quail.
Discussion
Avian species, as oviparous animals, have embryonic development within a unique egg environment where nutritional and hormonal factors can be directly injected to study the effect of these factors on processes of embryonic adipogenesis. In addition, different stages of embryos can be obtained from the eggs. However, it is difficult to directly modulate nutritional and hormonal factors in mammalian embryos and to study embryonic development without scarifying dams. For these reasons, chicken embryos have been used to visually aid in presenting embryonic development of adipose tissues (Chen et al., 2014) and to study a nutritional factor, selenium, in adipogenic differentiation by injecting in ovo (Hassan et al., 2014). In addition, the current study is the first demonstration that shows the visual evidence of fast-growing adipose tissues during quail embryonic development. Taken together, avian species are suitable models to study for embryonic adipose growth.
Vitamin A and its metabolites such as atRA have been used for the study of adipogenic differentiation in animals. Our previous study (Kim et al., 2019) showed different effects of atRA on differentiation of 3T3-L1 cells depending on atRA concentrations; atRA showed a pro-adipogenic effect at low concentrations but an anti-adipogenic effect at high concentrations. The current study shows the bell-shaped distribution of percentages of fat pad weights in EW with increasing concentrations (0–10 μM) of atRA (Figure 2C). The highest fat percentage was shown at 300 nM, and increased fat percentages were gradually diminished by injecting over 300 nM of atRA. These data are somewhat consistent with the previous study by showing anti-adipogenic activities of high concentrations of atRA in vitro (Muenzner et al., 2013) and reduced size of fat cells by high levels of atRA administration in vivo (Mercader et al., 2006).
The previous study showed that injection of atRA resulted in reduction of adipose tissues in adult mice (Amengual et al., 2010). In newborn Angers calves, injection of vitamin A significantly increased percentages of intramuscular fat (Harris et al., 2018). In broiler chickens, fat deposition rate was increased during 1–21 days after hatching but decreased during 22–42 days by dietary supplementation of vitamin A (Savaris et al., 2020). In the current study, in ovo injection of 300 nM atRA at E5–E7 resulted in an increase of weights and percentages of adipose tissues (Figure 3). The previous and current studies show that atRA can positively or negatively affect fat deposition in animals. In general, rate of fat accretion in adult animals is determined by a balance of lipolysis and lipogenesis (Cornelius, 1994). However, because avian embryos constantly receive required nutrients from the confined egg environments (Kimura and Warshaw, 1983), both adipocyte differentiation and lipogenesis mainly contribute to growth of embryonic adipose tissues until hatching when lipolysis starts to be active to provide energy for breaking the eggshell (Chen et al., 2014). In the current study, because embryos injected with atRA were sampled at E14 approximately 3 days before hatching in quail, increased fat weights with adipocyte hypertrophy in quail embryos by atRA might be attributed from enhanced lipogenesis rather than changes in lipolysis.
Our previous in vitro studies showed that atRA promoted differentiation of murine preadipocyte cell line (3T3-L1) into adipocytes (Kim et al., 2019) and induced adipogenic differentiation of chicken embryonic fibroblast (CEF) cell line (Lee et al., 2021) and primary CEFs (Kim et al., 2020a). These pro-adipogenic roles of atRA were also demonstrated by the finding that it increased lipid accumulation by atRA treatment in vitro. With the support of these in vitro studies, in ovo injection of atRA in the current study resulted in adipose hypertrophy. Interestingly, our previous study with a knockout mouse model demonstrated pro-adipogenic function of retinoids by the finding that mice with knockout of the Raldh1 gene, encoding an enzyme producing atRA from retinaldehyde, are lean with less fat (Yasmeen et al., 2013). In addition, Raldh1-deficient cells have impaired adipogenesis in vitro, which is rescued by RA supplementation (Reichert et al., 2011). Furthermore, atRA enhanced fat accumulation in zebra fish embryos (Fraher et al., 2015). These previous and current studies support the positive roles of atRA in adipocyte differentiation and fat accretion.
As Pparγ and Fabp4 have been used as well-known adipogenic markers, up-regulation of the two genes suggests promotion of adipocyte differentiation in embryonic adipose tissues by atRA. In general, differentiation potential is negatively correlated with proliferative activities. It is possible that promotion of adipocyte differentiation by atRA may reduce proliferation potential and, consequently, reduce population of preadipocytes in embryonic adipose tissues. In this regard, it is interesting to relate adipogenic potential with population of preadipocytes by assessing a reliable preadipocyte maker. Dlk1, also known as Pref-1, was originally discovered by comparing expression profiles before and after differentiation of 3T3-L1 preadipocytes and identifying Dlk1 as a predominantly expressed gene in preadipocytes (Smas and Sul, 1993). Our previous in vivo studies demonstrated anti-adipogenic function of Dlk1 through findings that reduced fat in Dlk1 overexpressed mice (Lee et al., 2003) and obesity phenotype in Dlk1 knockout mice (Moon et al., 2002). Therefore, Dlk1 has been used as a preadipocyte marker in humans (Krautgasser et al., 2019), pigs (Ahn et al., 2013), cattle (Cai et al., 2018), chickens (Shin et al., 2008), and quail (Ahn et al., 2015). In the current study, increased cell size in inguinal adipose tissues with increasing age (E12–E14) is accompanied with up-regulation of Pparγ and Fabp4 and down-regulation of Dlk1 expression regardless of treatment groups (Figure 4D), indicating temporal progresses of adipogenic differentiation during embryonic development in quail. In addition to up-regulation of Pparγ and Fabp4, a lesser expression of Dlk1 by 300 nM atRA compared with the control (0 M) at E14 suggests that adipocyte differentiation might be promoted by atRA and the preadipocyte population might be consequently reduced. In agreement with the current study, supplementation of atRA in 3D cultures of subcutaneous primary human preadipocytes contributed to adipocyte hypertrophy by regulating transcriptional factors involved in adipogenesis (Pope et al., 2020). For these reasons, it is possible that promotion of preadipocyte differentiation by atRA can contribute to increased fat mass in quail embryos. These findings support pro-adipogenic function of atRA during embryonic development.
Our finding showed that in ovo injection of 300 nM of atRA resulted in increased fat pad weights and fat cell size. Although the current study supports pro-adipogenic function of atRA in embryos, effects of dietary vitamin A or administration of RA into animals on fat accretion might be variable depending on concentrations of retinoids and ages of animals. Therefore, results from in vivo studies with retinoids should be carefully interpreted with consideration of these factors. Our experimental approach with in ovo injection in avian species can be applied to investigate effects of nutritional, hormonal, and pharmaceutical factors on embryonic development of various tissues and organs.
Data Availability Statement
The original contributions presented in the study are included in the article/supplementary material, further inquiries can be directed to the corresponding author/s.
Ethics Statement
Ethical review and approval was not required for the animal study because Experiments using poultry embryos are exempt from requiring University Institutional Animal Care and Use Committee approval, because avian embryos are not considered live animals by the Public Health Service Policy (ILAR News, 1991).
Author Contributions
KL: conceptualization and supervision. D-HK: data curation and visualization. D-HK, JL, SK, and HL: formal analysis. D-HK, JL, and KL: investigation. D-HK and JL: methodology and writing—original draft. KL and HL: writing—review and editing. All authors have read and agreed to the published version of the manuscript.
Funding
This research was funded by the United States Department of Agriculture National Institute of Food and Agriculture Hatch Grant (Project No. OHO01304).
Conflict of Interest
The authors declare that the research was conducted in the absence of any commercial or financial relationships that could be construed as a potential conflict of interest.
Acknowledgments
We are grateful to Michelle Milligan for her invaluable assistance by proofreading this manuscript.
References
Aeberli, I., Biebinger, R., Lehmann, R., L’allemand, D., Spinas, G. A., and Zimmermann, M. B. (2007). Serum retinol-binding protein 4 concentration and its ratio to serum retinol are associated with obesity and metabolic syndrome components in children. J. Clin. Endocrinol. Metab. 92, 4359–4365. doi: 10.1210/jc.2007-0468
Ahn, J., Oh, S. A., Suh, Y., Moeller, S. J., and Lee, K. (2013). Porcine G0/G1 switch gene 2 (G0S2) expression is regulated during adipogenesis and short-term in-vivo nutritional interventions. Lipids 48, 209–218. doi: 10.1007/s11745-013-3756-8
Ahn, J., Shin, S., Suh, Y., Park, J. Y., Hwang, S., and Lee, K. (2015). Identification of the avian RBP7 gene as a new adipose-specific gene and RBP7 promoter-driven GFP expression in adipose tissue of transgenic quail. PLoS One 10:e0124768. doi: 10.1371/journal.pone.0124768
Amengual, J., Ribot, J., Bonet, M. L., and Palou, A. (2010). Retinoic acid treatment enhances lipid oxidation and inhibits lipid biosynthesis capacities in the liver of mice. Cell. Physiol. Biochem. 25, 657–666. doi: 10.1159/000315085
Cai, H., Li, M., Sun, X., Plath, M., Li, C., Lan, X., et al. (2018). Global transcriptome analysis during adipogenic differentiation and involvement of transthyretin gene in adipogenesis in cattle. Front. Genet. 9:463.
Chen, P., Suh, Y., Choi, Y. M., Shin, S., and Lee, K. (2014). Developmental regulation of adipose tissue growth through hyperplasia and hypertrophy in the embryonic leghorn and broiler. Poult. Sci. 93, 1809–1817. doi: 10.3382/ps.2013-03816
Cornelius, P. (1994). Regulation of adipocyte development. Annu. Rev. Nutr. 14, 99–129. doi: 10.1146/annurev.nutr.14.1.99
Culling, C. F. A., Reid, P. E., Clay, M. G., and Dunn, W. L. (1974). The histochemical demonstration of O-acylated sialic acid in gastrointestinal mucins their association with the potassium hydroxide-periodic acid-schiff effect. J. Histochem. Cytochem. 22, 826–831. doi: 10.1177/22.8.826
Felipe, F., Mercader, J., Ribot, J., Palou, A., and Bonet, M. L. (2005). Effects of retinoic acid administration and dietary vitamin a supplementation on leptin expression in mice: lack of correlation with changes of adipose tissue mass and food intake. Biochim. Biophys. Acta 1740, 258–265. doi: 10.1016/j.bbadis.2004.11.014
Fraher, D., Ellis, M. K., Morrison, S., McGee, S. L., Ward, A. C., Walder, K., et al. (2015). Lipid abundance in zebrafish embryos is regulated by complementary actions of the endocannabinoid system and retinoic acid pathway. Endocrinology 156, 3596–3609. doi: 10.1210/EN.2015-1315
Hamburger, V., and Hamilton, H. L. (1951). A series of normal stages in the development of the chick embryo. J. Morphol. 88, 49–92. doi: 10.1002/jmor.1050880104
Harris, C. L., Wang, B., Deavila, J. M., Busboom, J. R., Maquivar, M., Parish, S. M., et al. (2018). Vitamin A administration at birth promotes calf growth and intramuscular fat development in angus beef cattle. J. Anim. Sci. Biotechnol. 9, 1–9. doi: 10.1186/s40104-018-0268-7
Hassan, A., Ahn, J., Suh, Y., Choi, Y. M., Chen, P., and Lee, K. (2014). Selenium promotes adipogenic determination and differentiation of chicken embryonic fibroblasts with regulation of genes involved in fatty acid uptake, triacylglycerol synthesis and lipolysis. J. Nutr. Biochem. 25, 858–867. doi: 10.1016/j.jnutbio.2014.03.018
ILAR News. (1991). Office of Laboratory animal Welfare, the Public Health Service Responds to Commonly Asked Questions, Question #1. Accessed Feb. 2021. Available Online at: https://olaw.nih.gov/guidance/articles/ilar91.html.
Jeyakumar, S. M., Vajreswari, A., and Giridharan, N. V. (2008). Vitamin A regulates obesity in WNIN/Ob obese rat; independent of stearoyl-CoA desaturase-1. Biochem. Biophys. Res. Commun. 370, 243–247. doi: 10.1016/j.bbrc.2008.03.073
Kim, D. H., Lee, J., Suh, Y., Cressman, M., and Lee, K. (2020a). Research note: all-trans retinoic acids induce adipogenic differentiation of chicken embryonic fibroblasts and preadipocytes. Poult. Sci. 99, 7142–7146. doi: 10.1016/j.psj.2020.09.006
Kim, D. H., Lee, J., Suh, Y., Cressman, M., Lee, S. S., and Lee, K. (2020b). Adipogenic and myogenic potentials of chicken embryonic fibroblasts in vitro: combination of fatty acids and insulin induces adipogenesis. Lipids 55, 163–171. doi: 10.1002/lipd.12220
Kim, D. H., Lee, J. W., and Lee, K. (2019). Supplementation of all-trans-retinoic acid below cytotoxic levels promotes adipogenesis in 3T3-L1 cells. Lipids 54, 99–107. doi: 10.1002/lipd.12123
Kimura, R. E., and Warshaw, J. B. (1983). Metabolic adaptations of the fetus and newborn. J. Pediatr. Gastroenterol. Nutr. 2, S12–S15. doi: 10.1097/00005176-198300201-00003
Krautgasser, C., Mandl, M., Hatzmann, F. M., Waldegger, P., Mattesich, M., and Zwerschke, W. (2019). Reliable reference genes for expression analysis of proliferating and adipogenically differentiating human adipose stromal cells. Cell. Mol. Biol. Lett. 24:14. doi: 10.1186/s11658-019-0140-6
Lee, J., Kim, D. H., Suh, Y., and Lee, K. (2021). Research note: potential usage of DF-1 cell line as a new cell model for avian adipogenesis. Poult. Sci. 100:101057. doi: 10.1016/j.psj.2021.101057
Lee, K., Villena, J. A., Moon, Y. S., Kim, K.-H., Lee, S., Kang, C., et al. (2003). Inhibition of adipogenesis and development of glucose intolerance by soluble preadipocyte factor–1 (Pref-1). J. Clin. Invest. 111, 453–461. doi: 10.1172/jci200315924
Livak, K. J., and Schmittgen, T. D. (2001). Analysis of relative gene expression data using real-time quantitative PCR and the 2-ΔΔCT method. Methods 25, 402–408. doi: 10.1006/meth.2001.1262
Mercader, J., Madsen, L., Felipe, F., Palou, A., Kristiansen, K., and Bonet, M. L. (2007). All-trans retinoic acid increases oxidative metabolism in mature adipocytes. Cell. Physiol. Biochem. 20, 1061–1072. doi: 10.1159/000110717
Mercader, J., Ribot, J., Murano, I., Felipe, F., Cinti, S., Bonet, M. L., et al. (2006). Remodeling of white adipose tissue after retinoic acid administration in mice. Endocrinology 147, 5325–5332. doi: 10.1210/en.2006-0760
Moon, Y. S., Smas, C. M., Lee, K., Villena, J. A., Kim, K.-H., Yun, E. J., et al. (2002). Mice lacking paternally expressed Pref-1/Dlk1 display growth retardation and accelerated adiposity. Mol. Cell. Biol. 22, 5585–5592. doi: 10.1128/mcb.22.15.5585-5592.2002
Muenzner, M., Tuvia, N., Deutschmann, C., Witte, N., Tolkachov, A., Valai, A., et al. (2013). Retinol-binding protein 4 and its membrane receptor STRA6 control adipogenesis by regulating cellular retinoid homeostasis and retinoic acid receptor activity. Mol. Cell. Biol. 33, 4068–4082. doi: 10.1128/mcb.00221-13
Nakamura, Y., Nakane, Y., and Tsudzuki, M. (2019). Developmental stages of the blue-breasted quail (Coturnix chinensis). Anim. Sci. J. 90, 35–48. doi: 10.1111/asj.13119
Pope, B. D., Warren, C. R., Dahl, M. O., Pizza, C. V., Henze, D. E., Sinatra, N. R., et al. (2020). Fattening chips: hypertrophy, feeding, and fasting of human white adipocytes: in vitro. Lab. Chip 20, 4152–4165. doi: 10.1039/d0lc00508h
Reichert, B., Yasmeen, R., Jeyakumar, S. M., Yang, F., Thomou, T., Alder, H., et al. (2011). Concerted action of aldehyde dehydrogenases influences depot-specific fat formation. Mol. Endocrinol. 25, 799–809. doi: 10.1210/me.2010-0465
Safonova, I., Darimont, C., Amri, E. Z., Grimaldi, P., Ailhaud, G., Reichert, U., et al. (1994). Retinoids are positive effectors of adipose cell differentiation. Mol. Cell. Endocrinol. 104, 201–211. doi: 10.1016/0303-7207(94)90123-6
Savaris, V. D. L., Souza, C., Wachholz, L., Broch, J., Polese, C., Carvalho, P. L. O., et al. (2020). Interactions between lipid source and vitamin A on broiler performance, blood parameters, fat and protein deposition rate, and bone development. Poult. Sci. 100, 174–185. doi: 10.1016/j.psj.2020.09.001
Shin, J., Lim, S., Latshaw, J. D., and Lee, K. (2008). Cloning and expression of delta-like protein 1 messenger ribonucleic acid during development of adipose and muscle tissues in chickens. Poult. Sci. 87, 2636–2646. doi: 10.3382/ps.2008-00189
Smas, C. M., and Sul, H. S. (1993). Pref-1, a protein containing EGF-like repeats, inhibits adipocyte differentiation. Cell 73, 725–734. doi: 10.1016/0092-8674(93)90252-l
Stoecker, K., Sass, S., Theis, F. J., Hauner, H., and Pfaffl, M. W. (2017). Inhibition of fat cell differentiation in 3T3-L1 pre-adipocytes by all-trans retinoic acid: integrative analysis of transcriptomic and phenotypic data. Biomol. Detect. Quantif. 11, 31–44. doi: 10.1016/j.bdq.2016.11.001
Keywords: all-trans retinoic acid, in ovo injection, adipocyte hypertrophy, fat accretion, embryonic development, quail
Citation: Kim D-H, Lee J, Kim S, Lillehoj HS and Lee K (2021) Hypertrophy of Adipose Tissues in Quail Embryos by in ovo Injection of All-Trans Retinoic Acid. Front. Physiol. 12:681562. doi: 10.3389/fphys.2021.681562
Received: 16 March 2021; Accepted: 14 April 2021;
Published: 21 May 2021.
Edited by:
Elizabeth Ruth Gilbert, Virginia Tech, United StatesReviewed by:
Woo Kyun Kim, University of Georgia, United StatesYang Xiao, Mayo Clinic, United States
Copyright © 2021 Kim, Lee, Kim, Lillehoj and Lee. This is an open-access article distributed under the terms of the Creative Commons Attribution License (CC BY). The use, distribution or reproduction in other forums is permitted, provided the original author(s) and the copyright owner(s) are credited and that the original publication in this journal is cited, in accordance with accepted academic practice. No use, distribution or reproduction is permitted which does not comply with these terms.
*Correspondence: Kichoon Lee, bGVlLjI2MjZAb3N1LmVkdQ==
†These authors have contributed equally to this work and share first authorship