- 1Sorbonne Université, CNRS, UMR 8227, Integrative Biology of Marine Models, Station Biologique de Roscoff, Roscoff Cedex, France
- 2Laboratoire d'Excellence GR-Ex, Paris, France
- 3Theoretical Medicine and Biosciences, Saarland University, Saarbrucken, Germany
- 4Experimental Physics, Saarland University, Saarbrucken, Germany
Introduction
Ca2+-permeable channels in red blood cells (RBCs) is a timely topic (Filser et al., 2020; Wang et al., 2021). They are of particular importance because the intracellular Ca2+ seems to be a major mediator in numerous RBC-related diseases including sickle cell disease (Mahkro et al., 2020) and numerous unrelated rare hereditary anemias (Hertz et al., 2017). Saying this, the focus (almost a hype) of the past years was on the mechanosensitive channel PIEZO1 since its discovery in 2010 (Coste et al., 2010) and especially the association of mutations in this channel with hereditary xerocytosis (Zarychanski et al., 2012; Albuisson et al., 2013; Andolfo et al., 2013; Bae et al., 2013; Rotordam et al., 2018). However, the entire picture of Ca2+ regulation in general and Ca2+-permeable channels in particular is a lot more versatile (Kaestner et al., 2020).
The TRPV2 Channel–Questions In Relation To The Properties Reported In RBCs
Very recently the transient receptor potential vanilloid type 2 (TRPV2) channel was reported to be present in RBCs (Belkacemi et al., 2021). TRPV2 is a non-selective cation channel conducting also Ca2+ and can be activated by Δ9-tetrahydrocannabinol (Δ9-THC) or cannabidiol (CBD). This is a milestone in RBC electrophysiology but at the same time raises a number of questions in the context of the channels physiological function and RBC hydration status, a discussion we like to stimulate with this opinion paper.
The discovery of the TRPV2 in RBCs is a remarkable finding because the abundance of ion channel copies is very low in the RBC membrane and functional channel recordings are complicated to align with molecular identities (Kaestner, 2015). Even in the study, where the TRPV2 was found, the detection of the Gárdos channel (KCNN4, KCa3.1, hSK4) was below the quality threshold in the proteomic study (Belkacemi et al., 2021).
We like to discuss two particular outcomes in more detail that may be relevant for the understanding of RBC physiology. A key point of the report by Belkacemi et al. (2021) was an increase of the osmotic fragility in TRPV2 KO mouse RBCs. The conclusion drawn is that TRPV2 mediated Ca2+ entry activates the Gárdos channel followed by K+ loss and subsequent loss of water, similar to what has already been suggested for PIEZO1. If this is the case one would expect cell shrinkage/dehydration upon TRPV2 activation with the channels agonists Δ9-THC and CBD, but the authors found in agreement with previous investigations (Chari-Bitron and Shahar, 1979) a cell swelling/overhydration. The explanation of this effect remains completely elusive, although we propose an initial concept of a mechanism (see below: section Putative consequences of TRPV2 activation in RBC after cannabis consumption).
In their paper (Belkacemi et al., 2021) showed that osmotic fragility is decreased upon RBC stimulation with Δ9-THC with Ca2+ being present in the external solution, that could partly be reversed by the additional application of the Gárdos channel inhibitor TRAM34. However, if RBCs are exposed to hypoosmotic conditions as performed in the paper, the cell swelling is expected to activate PIEZO1 or other mechanosensitive channels that mediate Ca2+ influx (Danielczok et al., 2017) i.e., one would expect a Gárdos channel-dependent modulation of the osmotic fragility since internal Ca2+ should increase, mediated by mechanosensitive cation channel activity. Surprisingly and for unknown reasons TRAM34 alone had no effect on the osmotic tolerance.
A Putative Contribution of TRPV2 in RBC Storage Lesions
If new channels are identified to be present in the RBCs membrane at the protein level, the question arises, if previous functional reports of channel activity can be aligned with the molecular discovery which is a complicated task (Kaestner, 2015).
TRPV2, like many members of the TRP family, shows a Ca2+-dependent desensitization, inhibition, or inactivation by extracellular Ca2+. More importantly, this function is maintained albeit TRPV2 does not contain the binding sites for calmodulin (CaM), adenosine triphosphate (ATP), or phosphatidylinositol-4,5-bisphosphate (PIP2) (Mercado et al., 2010). Such a behavior echoes a recent report of an unrecognized non-selective cation channel in human RBCs activated upon extracellular Ca2+ depletion and thought to potentially be a non-negligible part of the leaky pathways that contribute to the cation gradients dissipation upon storage with Ca2+ depleted solutions (Petkova-Kirova et al., 2018). To illustrate the similarity in functional behavior, we compiled Figures 1A–C to compare the I-V behavior of TRPV2 as described by Belkacemi et al. (2021) and the non-selective cation channel reported by Petkova-Kirova et al. (2018). When comparing the I-V relationship one should keep in mind the different recording conditions in two different laboratories. Furthermore, the ionic composition of both the internal and external solution differs as detailed in the figure legend. All these differences may explain particular deviations between the curves. Overall they look very similar (outward rectified), what is compatible with an agreement of TRPV2 (Belkacemi et al., 2021) and the channel activated by Ca2+ removal (Petkova-Kirova et al., 2018). However, this is by far not a proof. Therefore, future investigations need to confirm that TRPV2 is involved in RBC storage lesions. Nevertheless, for illustrative purposes, in Figure 1D we provide a scheme of the hypothesized contribution of TRPV2 to RBC cation gradient dissipation in storage lesions. We maintain the vision to have with TRPV2 a molecular player that could be pharmacologically addressed and potentially improve RBC storage conditions.
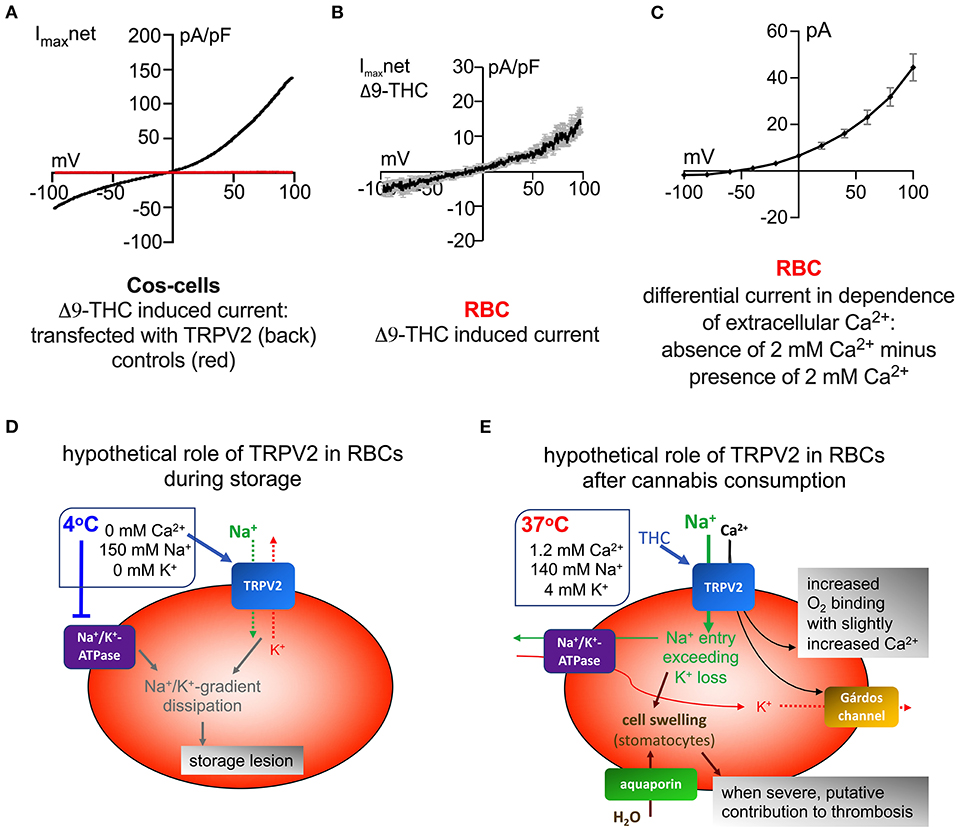
Figure 1. Comparison of I-V behavior of ion channels. (A) Whole cell currents measured in Cos-cells overexpressing TRPV2 (black trace) and control cells (red trace), both stimulated with 30 μM Δ9-THC. The internal solution contained (in mM): 120 Cs-glutamate, 8 NaCl, 1 MgCl2, 10 HEPES, 10 Cs-BAPTA, 3.1 CaCl2 (corresponding to100 nM free Ca2+), pH adjusted to 7.2 with CsOH, and the external solution contained (in mM): 140 NaCl, 2 MgCl2, 1 CaCl2, 10 HEPES, 10 glucose, pH adjusted to 7.2 with NaOH. For TRPV2 stimulation Δ9-THC was added to the external solution and applied directly to the patch clamped cell via an application pipette. Voltage ramps of 400 ms duration spanning a voltage range from −100 to 100 mV were applied at 0.5 Hz from a holding potential of 0 mV over a period of 300–400 s. (B) Whole cell currents measured in RBCs stimulated with 30 μM Δ9-THC. Recording conditions were identical to the ones described in (A). (C) Differential whole cell current measured in RBCs when 2 mM Ca2+ is removed from the extracellular solution. The current recorded in 2 mM CaCl2-external solution was subtracted from the current recorded in 0 mM CaCl2 -external solution. The internal solution contained (in mM): 50 CsCl, 20 NaCl, 60 CsF, 5 MgATP, 10 HEPES, 20 EGTA, pH adjusted to 7.2 with CsOH, and the external solution contained (in mM): 0 or 2 mM Ca2+, 125 TEACl, 10 HEPES, 5 MgCl2, 45 glucose, pH adjusted to 7.3 with TEA-OH. Please note that tetraethylammonium (TEA) is non-permeant to most cation channels and therefore cationic outward currents (corresponding to negative membrane potentials) are not expected. For the same reason, the reversal potential (membrane potential when no currents occur–intersection of curve with x-axis) is expected to be shifted toward more negative values. Recordings were conducted using voltage steps from −100 to 100 mV for 500 ms in 20 mV increments at 5 s intervals, the holding potential being set at −30 mV. In (A,B) the current is normalized to the cell capacitance (pA/pF), while in (C) the authors felt that in the planar chips, the capacitance does not correctly reflect the cell surface and therefore just provided the current per cell recording (pA). Additionally, the recording protocols were completely different, for (A,B), voltage ramps were applied and for (C) discreate voltage steps were recorded to compile the I-V relationship. (A,B) are reprints with permission from (Belkacemi et al., 2021) and (C) is reproduced from (Petkova-Kirova et al., 2018). (D) Suggested channel activity in RBCs during storage. The rectangle next to the RBC describes the main components of typical storage conditions. (E) Putative membrane transport interactions in RBCs after cannabis consumption. The rectangle next to the RBC describes the main components relevant for cation transport in the blood plasma.
TRPV2 IN RBC Physiology and Pathophysiology
We like to raise another aspect that is likely to be important to judge the relevance of TRPV2 in RBC. TRPV2 was reported to be a mechanosensitive channel (Muraki et al., 2003), and although we have no data on this mechanic activation in RBCs it is an appealing concept that TRPV2 could act similar as PIEZO1 and under certain conditions even compensate impaired PIEZO1 activity or may have in normal conditions a complimentary function. To this end we provide Table 1, comparing characteristic properties of TRPV2 and PIEZO1. Indeed, the PIEZO1 channel has a very particular activation signature. The range of pressure (or membrane tension) necessary for its activation is absolutely compatible with those encountered in the circulation or even in the passage of the splenic filtration. After opening the channel closes extremely quickly, probably rendering the increase in Ca2+ most of the time inoperative for a significant activation of the Gárdos channel leading to an alteration in cell volume (Please note that the PIEZO1 opening kinetics upon mechanical stimulation is different to activation by its agonist Yoda1). Indeed, the propensity of Ca2+ to be an efficient effector of RBC homeostasis is not only linked to its capacity to enter cells rapidly via conductive pathways, but also to the capacity of the plasma membrane Ca2+ ATPase (PMCA) to counterbalance this massive influx. Therefore, the PMCA limits the irreversibility of the subsequent activation of the Gárdos channel (Lew and Tiffert, 2017). This last point is of high interest regarding RBCs homeostasis, since Ca2+ flux plays most of the time the pivotal role, both in RBC physiology and pathophysiology. Such the Ca2+ homeostasis is also linked to RBC metabolism.
Considering that with PIEZO1 and TRPV2, two mechanically sensitive channels carrying Ca2+ has a definite advantage, allowing for finer modulation to ensure Ca2+ signaling which, if left unchecked, can rapidly alter the filterability of RBCs and lead to a reduction in their lifespan within the circulation. However, it is completely elusive if putative genetic variants of TRPV2 are molecular contributors to hereditary xerocytosis or any other hemolytic anemias.
Moreover, one of the aspects often overlooked when considering the effects of Ca2+ influx via conductive pathways into RBCs is the direct impact on the membrane potential. A Ca2+ entry, even minimal, will immediately cause a substantial depolarization of the RBCs from a resting membrane potential of ~-12 mV even before secondary effects like the activation of the Gárdos channel take place. This is not negligible and could explain some unexplained experimental observations (Kaestner et al., 2018; Jansen et al., 2021).
Putative Consequences of TRPV2 Activation in RBC After Cannabis Consumption
Since TRPV2 is activated by the cannabinoids Δ9-THC and CBD one can expect a TRPV2 activation in RBCs after cannabis consumption. In Figure 1E we sketched the molecular interactions of membrane transport proteins as we would expect from the known RBC membrane transport functions (Bernhardt and Ellory, 2003) and the observation that exposure of RBCs to Δ9-THC results in cell swelling (Chari-Bitron and Shahar, 1979; Belkacemi et al., 2021). We hypothesize two distinct effects. If there is only a slight activation of TRPV2, a minor increase in the RBC Ca2+ concentration could be stimulating and advantageous toward an increased oxygen binding affinity (Makhro et al., 2013). In contrast, a strong activation of TRPV2 leading to the afore mentioned cell swelling is likely to impair the capillary flow, where the RBC diameter exceeds the vessel diameter (Kihm et al., 2021). The cannabinoid induced increase in RBC volume could very well-contribute to the thrombotic events, frequently reported to occur after cannabis consumption especially in combination with vasoconstriction that is also related to cannabis consumption (Disdier et al., 2001; Mittleman et al., 2001; Peyrot et al., 2007; Wolff et al., 2011).
The molecular regulation as depicted in Figure 1E is a bit more complicated as the scheme in Figure 1D and is not limited to the Ca2+ permeability of TRPV2. Indeed, an increase in the intracellular Na+ concentration seems the only plausible explanation for the RBC swelling and as a consequence the Na+ influx must exceed the K+ efflux. This means the Na+ influx via TRPV2 must exceed the K+ efflux by the Gárdos channel, especially since the Na+/K+-ATPase has a Na+:K+ stoichiometry of 3:2. However, a variable abundance ratio of TRPV2 and Gárdos channel in individual RBCs could well explain the cellular variability in the RBC hydration state after Δ9-THC stimulation.
Conclusion
The biochemical data of the recently reported abundance of the TRPV2 in RBCs are sound and convincing (Belkacemi et al., 2021). However, further research on the functional properties of TRPV2 in RBCs and the involvement of TRPV2 in: (i) RBC physiology inclusive effects caused by cannabis consumption, (ii) the genesis of RBC related disease, (iii) in the treatment of malaria as proposed by the authors in the original report and (iv) its contribution to the cation gradients dissipation upon storage as outlined above, are now required.
Author Contributions
SE and LK wrote the manuscript and agree to be accountable for the content of the work.
Funding
This study was supported by the European Framework Horizon 2020 under grant agreement number 860436 (EVIDENCE) and we acknowledge support by the Deutsche Forschungsgemeinschaft (DFG, German Research Foundation) and Saarland University within the funding programme Open Access Publishing.
Conflict of Interest
The authors declare that the research was conducted in the absence of any commercial or financial relationships that could be construed as a potential conflict of interest.
References
Albuisson, J., Murthy, S. E., Bandell, M., Coste, B., Louis-dit-Picard, H., Mathur, J., et al. (2013). Dehydrated hereditary stomatocytosis linked to gain-of-function mutations in mechanically activated PIEZO1 ion channels. Nat. Commun. 4:2440. doi: 10.1038/ncomms3440
Andolfo, I., Alper, S. L., Franceschi, L. D., Auriemma, C., Russo, R., Falco, L. D., et al. (2013). Multiple clinical forms of dehydrated hereditary stomatocytosis arise from mutations in PIEZO1. Blood 121, 3925–3935. doi: 10.1182/blood-2013-02-482489
Bae, C., Gnanasambandam, R., Nicolai, C., Sachs, F., and Gottlieb, P. A. (2013). Xerocytosis is caused by mutations that alter the kinetics of the mechanosensitive channel PIEZO1. Proc. Natl. Acad. Sci. U.S.A. 110, E1162–E1168. doi: 10.1073/pnas.1219777110
Bae, C., Sachs, F., and Gottlieb, P. A. (2011). The mechanosensitive ion channel Piezo1 is inhibited by the peptide GsMTx4. Biochemistry 50, 6295–6300. doi: 10.1021/bi200770q
Belkacemi, A., Trost, C. F., Tinschert, R., Flormann, D., Malihpour, M., Wagner, C., et al. (2021). The TRPV2 channel mediates Ca2+ influx and the D9-THC-dependent decrease in osmotic fragility in red blood cells. Haematologica. doi: 10.3324/haematol.2020.274951. [Epub ahead of print].
Bernhardt, I., and Ellory, J. C. (2003). Red Cell Membrane Transport in Health and Disease. Berlin; Heidelberg: Springer Science & Business Media. doi: 10.1007/978-3-662-05181-8
Chari-Bitron, A., and Shahar, A. (1979). Changes in rat erythrocyte membrane induced byΔ1-tetrahydrocannabinol, scanning electron microscope study. Experientia 35, 365–366. doi: 10.1007/BF01964355
Coste, B., Mathur, J., Schmidt, M., Earley, T. J., Ranade, S., Petrus, M. J., et al. (2010). Piezo1 and Piezo2 are essential components of distinct mechanically activated cation channels. Science 330, 55–60. doi: 10.1126/science.1193270
Coste, B., Xiao, B., Santos, J. S., Syeda, R., Grandl, J., Spencer, K. S., et al. (2012). Piezo proteins are pore-forming subunits of mechanically activated channels. Nature 483, 176–181. doi: 10.1038/nature10812
Danielczok, J. G., Terriac, E., Hertz, L., Petkova-Kirova, P., Lautenschläger, F., Laschke, M. W., et al. (2017). Red blood cell passage of small capillaries is associated with transient Ca2+-mediated adaptations. Front. Physiol. 8, 979. doi: 10.3389/fphys.2017.00979
Disdier, P., Granel, B., Serratrice, J., Constans, J., Michon-Pasturel, U., Hachulla, E., et al. (2001). Cannabis arteritis revisited. Angiology 52, 1–5. doi: 10.1177/000331970105200101
Filser, M., Giansily-Blaizot, M., Grenier, M., Alonso, D. M., Bouyer, G., Peres, L., et al. (2020). Increased incidence of germline PIEZO1 mutations in individuals with idiopathic erythrocytosis. Blood 137, 1828–1832. doi: 10.1182/blood.2020008424
Ge, J., Li, W., Zhao, Q., Li, N., Chen, M., Zhi, P., et al. (2015). Architecture of the mammalian mechanosensitive Piezo1 channel. Nature 527, 64–69. doi: 10.1038/nature15247
Gnanasambandam, R., Bae, C., Gottlieb, P. A., and Sachs, F. (2015). Ionic selectivity and permeation properties of human PIEZO1 channels. PLoS ONE 10, e0125503–e0125516. doi: 10.1371/journal.pone.0125503
Hertz, L., Huisjes, R., Llaudet-Planas, E., Petkova-Kirova, P., Makhro, A., Danielczok, J. G., et al. (2017). Is increased intracellular calcium in red blood cells a common component in the molecular mechanism causing anemia? Front. Physiol. 8:673. doi: 10.3389/fphys.2017.00673
Jansen, J., Qiao, M., Hertz, L., Wang, X., Fermo, E., Zaninoni, A., et al. (2021). Mechanistic ion-channel interactions in red cells of Gárdos channelopathy patients. Blood Adv.
Kaestner, L. (2015). Channelizing the red blood cell: molecular biology competes with patch-clamp. Front. Mol. Biosci. 2:46. doi: 10.3389/fmolb.2015.00046
Kaestner, L., Bogdanova, A., and Egee, S. (2020). Calcium channels and calcium-regulated channels in human red blood cells. Adv. Exp. Med. Biol. 1131, 625–648. doi: 10.1007/978-3-030-12457-1_25
Kaestner, L., Wang, X., Hertz, L., and Bernhardt, I. (2018). Voltage-Activated ion channels in non-excitable cells-A viewpoint regarding their physiological justification. Front. Physiol. 9:450. doi: 10.3389/fphys.2018.00450
Kihm, A., Quint, S., Laschke, M. W., Menger, M. D., John, T., Kaestner, L., et al. (2021). Lingering dynamics in microvascular blood flow. Biophys. J. 120, 432–439. doi: 10.1016/j.bpj.2020.12.012
Lacroix, J. J., Botello-Smith, W. M., and Luo, Y. (2018). Probing the gating mechanism of the mechanosensitive channel Piezo1 with the small molecule Yoda1. Nat. Commun. 9:2029. doi: 10.1038/s41467-018-04405-3
Lew, V. L., and Tiffert, T. (2017). On the mechanism of human red blood cell longevity: roles of calcium, the sodium pump, PIEZO1, and gardos channels. Front. Physiol. 8:977. doi: 10.3389/fphys.2017.00977
Mahkro, A., Hegemann, I., Seiler, E., Simionato, G., Claveria, V., Bogdanov, N., et al. (2020). A pilot clinical phase II trial MemSID: acute and durable changes of red blood cells of sickle cell disease patients on memantine treatment. Ejhaem 1, 23–34. doi: 10.1002/jha2.11
Makhro, A., Hanggi, P., Goede, J. S., Wang, J., Brüggemann, A., Gassmann, M., et al. (2013). N-methyl D-aspartate (NMDA) receptors in human erythroid precursor cells and in circulating red blood cells contribute to the intracellular calcium regulation. AJP Cell Phys. 305, C1123–C1138. doi: 10.1152/ajpcell.00031.2013
Mercado, J., Gordon-Shaag, A., Zagotta, W. N., and Gordon, S. E. (2010). Ca2+-dependent desensitization of TRPV2 channels is mediated by hydrolysis of phosphatidylinositol 4,5-bisphosphate. J. Neurosci. 30, 13338–13347. doi: 10.1523/JNEUROSCI.2108-10.2010
Mittleman, M. A., Lewis, R. A., Maclure, M., Sherwood, J. B., and Muller, J. E. (2001). Triggering myocardial infarction by marijuana. Circulation 103, 2805–2809. doi: 10.1161/01.CIR.103.23.2805
Moore, C., and Liedke, W. B. (2017). “Osmomechanical-Sensitive TRPV Channels in Mammals,” in Neurobiology of TRP Channels, ed T. L. R. Emir (Boca Raton: CRC Press). doi: 10.4324/9781315152837-5
Muller, C., Morales, P., and Reggio, P. H. (2019). Cannabinoid ligands targeting TRP channels. Front. Mol. Neurosci. 11:487. doi: 10.3389/fnmol.2018.00487
Muraki, K., Iwata, Y., Katanosaka, Y., Ito, T., Ohya, S., Shigekawa, M., et al. (2003). TRPV2 Is a component of osmotically sensitive cation channels in murine aortic myocytes. Circulation Res. J Am Hear Assoc 93, 829–838. doi: 10.1161/01.RES.0000097263.10220.0C
Neeper, M. P., Liu, Y., Hutchinson, T. L., Wang, Y., Flores, C. M., and Qin, N. (2007). Activation properties of heterologously expressed mammalian TRPV2 evidence for species dependence*. J. Biol. Chem. 282, 15894–15902. doi: 10.1074/jbc.M608287200
Perálvarez-Marín, A., Doñate-Macian, P., and Gaudet, R. (2013). What do we know about the transient receptor potential vanilloid 2 (TRPV2) ion channel? Febs J. 280, 5471–5487. doi: 10.1111/febs.12302
Petkova-Kirova, P., Hertz, L., Makhro, A., Danielczok, J., Huisjes, R., Llaudet-Planas, E., et al. (2018). A previously unrecognized Ca2+-inhibited nonselective cation channel in red blood cells. Hemasphere 2:e146. doi: 10.1097/HS9.0000000000000146
Peyrot, I., Garsaud, A., Saint-Cyr, I., Quitman, O., Sanchez, B., and Quist, D. (2007). Cannabis arteritis: a new case report and a review of literature. J. Eur. Acad. Dermatol. 21, 388–391. doi: 10.1111/j.1468-3083.2006.01947.x
Rotordam, G. M., Fermo, E., Becker, N., Barcellini, W., Brüggemann, A., Fertig, N., et al. (2018). A novel gain-of-function mutation of Piezo1 is functionally affirmed in red blood cells by high-throughput patch clamp. Haematologica 104, e179–e183. doi: 10.3324/haematol.2018.201160
Wang, J., Hertz, L., Ruppenthal, S., Nemer, W. E., Connes, P., Goede, J. S., et al. (2021). Lysophosphatidic acid-activated calcium signaling is elevated in red cells from sickle cell disease patients. Cells 10:456. doi: 10.3390/cells10020456
Wolff, V., Lauer, V., Rouyer, O., Sellal, F., Meyer, N., Raul, J. S., et al. (2011). Cannabis Use, Ischemic Stroke, and Multifocal Intracranial Vasoconstriction. Stroke 42, 1778–1780. doi: 10.1161/STROKEAHA.110.610915
Zarychanski, R., Schulz, V. P., Houston, B. L., Maksimova, Y., Houston, D. S., Smith, B., et al. (2012). Mutations in the mechanotransduction protein PIEZO1 are associated with hereditary xerocytosis. Blood 120, 1908–1915. doi: 10.1182/blood-2012-04-422253
Keywords: red cells, ion channels, Ca2+ signaling, volume regulation, storage lesions
Citation: Egée S and Kaestner L (2021) The Transient Receptor Potential Vanilloid Type 2 (TRPV2) Channel–A New Druggable Ca2+ Pathway in Red Cells, Implications for Red Cell Ion Homeostasis. Front. Physiol. 12:677573. doi: 10.3389/fphys.2021.677573
Received: 07 March 2021; Accepted: 17 May 2021;
Published: 10 June 2021.
Edited by:
Richard Van Wijk, Utrecht University, NetherlandsReviewed by:
Immacolata Andolfo, University of Naples Federico II, ItalyGuizouarn Helene, Centre National de la Recherche Scientifique (CNRS), France
Copyright © 2021 Egée and Kaestner. This is an open-access article distributed under the terms of the Creative Commons Attribution License (CC BY). The use, distribution or reproduction in other forums is permitted, provided the original author(s) and the copyright owner(s) are credited and that the original publication in this journal is cited, in accordance with accepted academic practice. No use, distribution or reproduction is permitted which does not comply with these terms.
*Correspondence: Stéphane Egée, ZWdlZSYjeDAwMDQwO3NiLXJvc2NvZmYuZnI=; Lars Kaestner, bGFyc19rYWVzdG5lciYjeDAwMDQwO21lLmNvbQ==