- 1Departamento de Ciencias Químico-Biológicas, Universidad de Sonora, Hermosillo, Mexico
- 2Departamento de Investigación en Física, Universidad de Sonora, Hermosillo, Mexico
- 3Departamento de Ciencias Químico-Biológicas, Universidad de las Américas, Puebla, Mexico
- 4Departamento de Física, Universidad de Sonora, Hermosillo, Mexico
The storage lesions and the irradiation of blood cellular components for medical procedures in blood banks are events that may induce nanochanges in the membrane of red blood cells (RBCs). Alterations, such as the formation of pores and vesicles, reduce flexibility and compromise the overall erythrocyte integrity. This review discusses the alterations on erythrocytic lipid membrane bilayer through their characterization by confocal scanning microscopy, Raman, scanning electron microscopy, and atomic force microscopy techniques. The interrelated experimental results may address and shed light on the correlation of biomechanical and biochemical transformations induced in the membrane and cytoskeleton of stored and gamma-irradiated RBC. To highlight the main advantages of combining these experimental techniques simultaneously or sequentially, we discuss how those outcomes observed at micro- and nanoscale cell levels are useful as biomarkers of cell aging and storage damage.
Introduction
Irradiation of blood components is the only accepted procedure for preventing transfusion-associated graft-versus-host disease (TA-GVHD; Santacruz-Gomez et al., 2016). The standard dose is 25 Gy of gamma rays or X-rays (Manduzio, 2018). The effects of ionizing radiation on biochemical properties of red blood cell (RBC) membrane are well-documented and disclosing that lymphocyte apoptosis is responsible for lymphocytopenia in the early stage after irradiation (Sellins and Cohen, 1987; Moroff and Luban, 1994; Moroff and Luban, 1997; Cui et al., 1999). Radiation acts negatively on the mitotic activity of lymphocytes responsible for triggering TA-GVHD, rendering them unviable (Xu et al., 2012). The radiation accentuates the effects of storage, promoting the generation of reactive oxygen species, membrane lipid peroxidation, and increased permeability of RBC’s membrane to potassium, sodium, hemoglobin (Hb), and lactate dehydrogenase, and a decrease in RBC’s deformability (Ramirez et al., 1987; Anand et al., 1997; Hirayama et al., 2005; Zbikowska et al., 2014; Acosta-Elías et al., 2017; Adams et al., 2018).
In blood banks, RBC undergoes time-dependent biochemical changes collectively known as storage lesions (Antonelou and Seghatchian, 2016; Kozlova et al., 2017). During storage, it is common to observe reductions of internal pH, depletion of adenosine triphosphate (ATP), 2,3-diphosphoglycerate (2,3-DPG), nitric oxide (NO), and glucose, as well as the loss of activity of glutathione and glutathione peroxidase. Conversely, there is an increase in the levels of free Hb, potassium, lactic acid, and calcium (D’Alessandro et al., 2015; Kim et al., 2015; D’Alessandro et al., 2017; Barshtein et al., 2018; Yoshida et al., 2019). These changes produce structural modifications at the nanoscale level, such as pores and vesicle formation, compromising essential functional and structural properties of RBC (Santacruz-Gomez et al., 2014; D’Alessandro et al., 2015; Acosta-Elías et al., 2017; Koch et al., 2019).
The depletion of ATP impacts the membrane elasticity, intracellular viscosity, and the optimal surface area/volume ratio (Kim et al., 2015). Over time, the morphology changes from normal biconcave disc cells to irreversibly deformed spherocytes (Bosman et al., 2008; Koch et al., 2019), mainly due to the membrane’s shedding of vesicles. These vesicles are generally produced during maturation to remove damaged cell components, preventing their removal from circulation by the spleen (Huisjes et al., 2018). Vesiculation is a cellular process that may trigger intracellular communication. The RBCs do not undergo classical apoptosis. Instead, they can be removed from the circulation by cell death due to hemolysis (osmotic shock) or through the antagonistic effects of phosphatidylserine (PS) and CD47 on the phagocytic activity of macrophages, present in the spleen and liver. Recently, RBCs are not considered inert bystanders but active agents in intercellular signaling, immune function, and inflammatory processes (Arias and Arias, 2017). In this sense, the RBC vesiculation process promotes cell-cell communication. They function to store and transport proteins and lipids, activate cytokine cascades, growth factors, and even activate signaling pathways in target cells (Alaarg et al., 2013; Huang-Doran et al., 2017; Karsten and Herbert, 2019; Zhang et al., 2019). The vesicles are produced by the dislocation of the phospholipids of the plasmatic membrane and cytoskeleton involving protein oxidation (Sut et al., 2017). Along the life span of RBCs, their surface area decreases by approximately 20% due to vesicle release (Werre et al., 2004). This decrease in size is used as a marker for aging and storage lesions (Girasole et al., 2010; Dinarelli et al., 2018).
On the other hand, albumin is the most abundant plasma protein. When albumin concentration falls (in low circulation flow in vitro; Jay, 1975; Talstad et al., 1983; Reinhart and Nagy, 1995; Reinhart et al., 2015), RBCs suffer reversible alterations until transfusion, like echinocytic morphology transformation, increasing aggregation, sedimentation, and viscosity. These phenomena occur since this protein is responsible for eliminating substances from the outer half of the lipid bilayer of the RBC, for example, lysophosphatidylcholine, which accumulates during metabolic depletion.
Some biochemical values already mentioned are currently used in the blood bank as quality markers in blood cells, such as potassium leakage manifested by changes in cations concentration due to falling ATP levels, or hemolysis, a phenomenon occurring in a more rigid membrane, which is susceptible to rupture (Wallas, 1979; Antwi-Baffour et al., 2019). Nanoscale analysis can be a handy tool to determine biochemical and structural changes, such as alterations in protein domains of the erythrocyte membrane caused by storage injury and irradiation. These nanoalterations cannot be detected earlier through current blood bank techniques until the damage is clearly present in the RBC (Zheng and Li, 2012).
The atomic force microscopy (AFM) technique allows studying the erythrocyte membrane’s roughness, considered as a parameter proportional to the cell deformability (Santacruz-Gomez et al., 2014). The main disadvantage of AFM is that it is possible to confuse two nanostructures that look the same physical but have different biochemical compositions. On the other hand, Raman spectroscopy can detect the biochemical fingerprint of micro-conformational changes associated with membrane proteins and lipids and Hb’s oxygenation states in erythrocytes (Li et al., 2012). In this sense, the use of techniques, such as AFM and Raman, allows the detection of potential early alterations to make better decisions when administering the patient’s blood bag. This information would help broaden the panorama toward an early medical diagnosis and, in the future, could adapt these techniques to the blood bank for routine analysis (Dulińska et al., 2006).
Understanding both the micro- and nanobiochemical alterations that preclude the healthy function of RBC is a challenging task with huge pharmaceutical and medical implications. This mini-review explores a set of experimental biophysics and microscopy techniques that may address and shed light on the correlation of biomechanical and biochemical transformations induced in stored and gamma-irradiated RBCs.
Storage-Induced Alterations in RBC and Their Characterization
Among the changes induced by blood bank storing, RBC’s vesiculation is probably the result of spontaneous curvature, compression, and altered membrane protein conformation (Li and Lykotrafitis, 2015). Disturbances in the interaction between the cytoskeleton and the lipid bilayer may lead to microparticle detachment (Leal et al., 2018; Freitas Leal et al., 2020). This cooperative phenomenon affects the structure and conformation of spectrin and actin proteins, located in the cytoskeleton, the two domain structures of band 3, one of them present in the cytosol and the other in the lipid bilayer and the ankyrin-binding protein (Kim et al., 2015; Lux, 2016; Dinarelli et al., 2018). The formation of band 3-IgG complexes and phosphatidylserine exposure can also promote vesiculation (Girasole et al., 2010; Huisjes et al., 2018). Thus, clustered band 3 induces autologous IgG binding, and the impaired interaction of band 3 to ankyrin/spectrin network induces the shedding of vesicles containing the clustered band 3 and prevents IgG opsonization and RBC clearance. Therefore, vesiculation is suggested as an RBC mechanism to avoid cell elimination from circulation. Hence, vesicles are removed by recognition of RBC “eat me” signals, such as PS and specific band 3 cleavage products that react with senescent antigens (Alaarg et al., 2013). The composition of the microparticles may vary depending on the duration and storage conditions (Piccin et al., 2015).
Micro- and nanoscale characterization techniques have been used to elucidate the processes that deteriorate RBC functionality (Chasis and Mohandas, 1986; Girasole et al., 2001, 2010; Huang et al., 2011; Bhaduri et al., 2014; Santacruz-Gomez et al., 2014; Mukherjee et al., 2015; Acosta-Elías et al., 2017; Rico et al., 2018; Depond et al., 2020; Lima et al., 2020).
For surface studies, the laser confocal scanning microscopy (LCSM) is the most commonly used technique, as it allows the localization of a specific molecule in the cell and organelles (Collazo et al., 2005), but its resolution cannot reach the nanometric scale for alterations. However, this simple technique facilitates the understanding of the dynamics of proteins and lipids in the RBCs membrane, e.g., the bicarbonate/chloride exchanger band 3, cytoskeleton proteins, such as spectrin, ankyrin, and actin, 4.1 protein, glycophorin C, and even Hb and ATP (Campanella et al., 2005; Antonelou et al., 2010; Chu et al., 2012). Even more, LCSM for immunostaining protocols (Antonelou et al., 2010) disclosed how the distribution of erythrocyte proteins band 3, spectrin, and Hb is affected after 42 days of storage. Also, different oxidative effects on RBC can be analyzed using LCSM, such as alterations on the band 3 protein and spectrin network that can cause changes in biconcave morphology (e.g., equinocitic, stomatocytic, or spherocitic shape) and may provoke the loss of cell functionality (deformability and elasticity; Oore-ofe et al., 2017). A deeper study of these conformational changes of membrane proteins and lipids, as well as the oxygenation states of Hb in erythrocytes, can be made using Raman spectroscopy (RS; Asghari-Khiavi et al., 2010; Chu et al., 2012; Parshina et al., 2013; Acosta-Elías et al., 2017). In this way, RS is a precise technique that can provide information about vibrational states as chemical composition, molecular structure, and molecular interactions in cells and tissues (Choo-Smith et al., 2002; Parshina et al., 2013). It can follow the evolution of biological events in real time, but sometimes the fluorescence signal from water or related aqueous substances may hinder the Raman signal. This analytical tool has been widely used to study blood and its components, ranging from basic research on hemoglobin oxygenation to forensic investigations (Kang et al., 2008; Huang et al., 2011; Santacruz-Gomez et al., 2014; Buckley et al., 2016; Acosta-Elías et al., 2017; Atkins et al., 2017; Gautam et al., 2018; Burgara-Estrella et al., 2020). In particular, the oxygenated and deoxygenated Raman resonant hemoglobin modes have been used as indicators to describe RBC’s integrity (Wood et al., 2001). In a particular case of Raman setup called resonant Raman, we can select the modes to measure only isolated Hb changes. For a given resonant wavelength, the response generated exceeds the signal from the membrane’s proteins (Acosta-Elías et al., 2017). Besides, resonant Raman can track not only the structure of Hb during storage but also the fluidity of the RBC membrane changes during cell aging.
Additionally, scanning electron microscopy (SEM) allows us to visualize the progressive changes in RBC morphology related to storage injury (Usry et al., 1975; Berezina et al., 2002; Mustafa et al., 2016). SEM techniques provide us with an excellent image of the topology of the RBC membrane surface with the capacity to perform an elemental analysis (through EDS) of selected regions. The main drawback of this technique is the complicated preparation of biological samples, generating the alteration of them in many cases. Among the changes observed by SEM, the most significant are the alterations in the typical biconcave RBC conformation, making them stiffer and prone to breakage with diminished resistance to hemolysis (Girasole et al., 2007; Kor et al., 2009; Acosta-Elías et al., 2017). In this line, the SEM recorded changes during storage, including the loss of RBC’s discoid shape and their transformation to spherocytes because of the decrease in the surface/volume ratio following the vesiculation process (Geekiyanage et al., 2019). These phenomena are successfully observed after 14 days with nanoalterations by day 21 in pores and high free Hb (Mustafa et al., 2016; Acosta-Elías et al., 2017). Despite these remarkable results, preparation of biological (non-conductive) samples in SEM involves a gold coating that complicates to see fast changes in RBC structure and preclude following in short times their evolution.
The rapid assessment of membrane nanomodifications in RBCs may be accomplished using AFM (Girasole et al., 2001, 2007; Asghari-Khiavi et al., 2010; Santacruz-Gomez et al., 2014; Marzec et al., 2015; Acosta-Elías et al., 2017; Kozlova et al., 2018; Spyratou et al., 2019; Burgara-Estrella et al., 2020). This technique allows the tracking in short times with minimal sample preparation, during the blood storage, the progressive impairment of the spectrin tetramers network’s dynamical properties connected to actin complexes, and the bilayer’s integral proteins (Moroz et al., 2010; Dinarelli et al., 2018; Kozlova et al., 2018, 2019). Here, the biochemical changes render a diminishing of roughness membrane. This fact is noteworthy because roughness is considered a cell health parameter proportional to its deformability. The storage process induces the appearance of nanopores and nanovesicles that modify the microstructure and deformability of RBC and, therefore, their function (Girasole et al., 2007, 2010; Santacruz-Gomez et al., 2014; Acosta-Elías et al., 2017; Kozlova et al., 2019). Consequently, the storage process triggers a deterioration in the functionality of RBC, mainly in the oxidative metabolism at the level of protein oxidation, including hemoglobin, lipid oxidation, and metabolic alterations, such as lactate accumulation 2,3-DPG depletion. Stored erythrocytes may cause adverse effects on the circulatory cycle, non-transferrin bound iron, insufficient nitric oxide bioavailability, as well as altered infusion and damage to immune modulation (e.g., inflammation), and also cardiac electrophysiological alterations (Yoshida et al., 2019; Reilly et al., 2020).
RBC Alterations by Ionizing Radiation and Characterization Techniques
Irradiation of blood is recommended to prevent TA-GVHD and to reduce the side effects of transfusions, transplants, and cancer therapies (Weinmann et al., 2000; Agarwal et al., 2005; Walpurgis et al., 2013; Santacruz-Gomez et al., 2014; Tabatabaei et al., 2016; Acosta-Elías et al., 2017). The blood can be irradiated before, during, and after storage, depending on the patient’s necessities, but a common characteristic of irradiated blood is that RBCs are prone to hemolysis and, therefore, they are more fragile in circulation. This effect reduces the time significantly to storage and transfusion. The Guidelines of the AABB (United States) and the Canadian Standards Association establish that blood can be stored up to 42 days without irradiation and, once irradiated, up to 28 days. In contrast, the Protocols of the Council of Europe and the British Committee for Standards in Haematology allow blood to be stored for up to 28 days, and when blood undergoes the irradiation process, it should be stored for 14 additional days (Góes et al., 2008; de Korte et al., 2018). In México, the current regulation is the Official Mexican Standard NOM-253-SSA1-2012. This standard regulates the disposal of human blood and its components for therapeutic purposes. The storage of unirradiated blood is permitted for up to 42 days after their extraction, in agreement with the United States and Canada regulations. Once the blood is subject to irradiation, the storage time reduces to 14 days.
One approach to assure the quality of irradiated blood is to seek parameters closely related to its primary function: oxygen transportation. Thus, the oxygenation state of the Hb molecule is considered as a reliable parameter to evaluate the quality of both stored and irradiated RBCs (Weinmann et al., 2000; Kanias and Acker, 2010; Acosta-Elías et al., 2015; Mustafa et al., 2016; Acosta-Elías et al., 2017). To discriminate changes that could affect the performance of RBC, it is necessary to take into account changes during storage, e.g., the conversion of erythrocytes into acanthocytes: type 1, with a small central pale halo, and type 2, with a flat surface. Alterations in these cells’ central depth seem to be associated with the presence/absence of oxygen at their surface. Usually, oxygen molecules bind to Hb’s available heme group, forming oxygenated Hb (Oxy-Hb). The presence of oxygen at the surface gives RBC’s a pale central appearance (Santacruz-Gomez et al., 2014). An investigation of Hb functionality revealed that Hb’s oxygenation status is closely related to the ν4, νm37, and ν10 bands’ position and intensity in the Raman spectra. Any modification in these bands depends mostly on the symmetry of the porphyrin ring, revealing whether there have been changes from the tense state (Oxy) to the relaxed state (DeOxy) of Hb (Kanias and Acker, 2010; Acosta-Elías et al., 2017). But it has been found that irradiation and storage do not produce changes in Hb’s oxygenation state until day 13, at doses of radiation ranging from 15 to 50 Gy. However, this does not exclude the possibility that damages in the RBC membrane may effectively interrupt oxygen transport (Acosta-Elías et al., 2015; D’Alessandro et al., 2015). In this respect, the AFM studies may close the gap associated with structural changes in morphology and size of RBC when subjected to radiation and storage stressors.
Studies with AFM have shown dose-dependent damage on mice RBCs after gamma irradiation, with the appearance of nanopores, crater-like vesicles, and changes in membrane roughness (Walpurgis et al., 2013; AlZahrani and Al-Sewaidan, 2017; Taqi et al., 2019). Here, AFM renders invaluable information at nanoscale dominions on RBCs under irradiation stress. Therefore, vesiculation evaluated by AFM is a useful marker for cell aging at the nanoscale (Girasole et al., 2007, 2010). Moreover, the AFM-Raman concurrence permits the evaluation of the oxygenation states in erythrocytes in their different morphologies, such as echinocytes and stomatocytes, to help elucidate whether a rearrangement of the membrane affects oxygen transport (Santacruz-Gomez et al., 2016).
Figure 1 depicts an illustrative summary of the changes that occur during storage and irradiation. In A and B AFM images, we observed non-irradiated erythrocytes with homogeneous distribution in shape and size. Under gamma irradiation, it is noticed the appearance of echinocytic morphologies and heterogeneous size distributions. SEM micrographs (C and D) show signs of hemolysis with tiny pores in the membrane and echinocytic morphologies after 28 days of storage. Through fluorescence micrographs (E and F), we can observe the protein modifications associated with band 3, highlighting the formation of clusters after prolonged storage of 42 days. Furthermore, with the help of Raman (G) spectroscopy, we know the oxygenation status of Hb after 13 days of storage for different doses of gamma radiation (15, 25, 35, and 50 Gy). Raman spectra showed that erythrocytes subjected to these conditions do not produce changes in Hb’s oxygenation state.
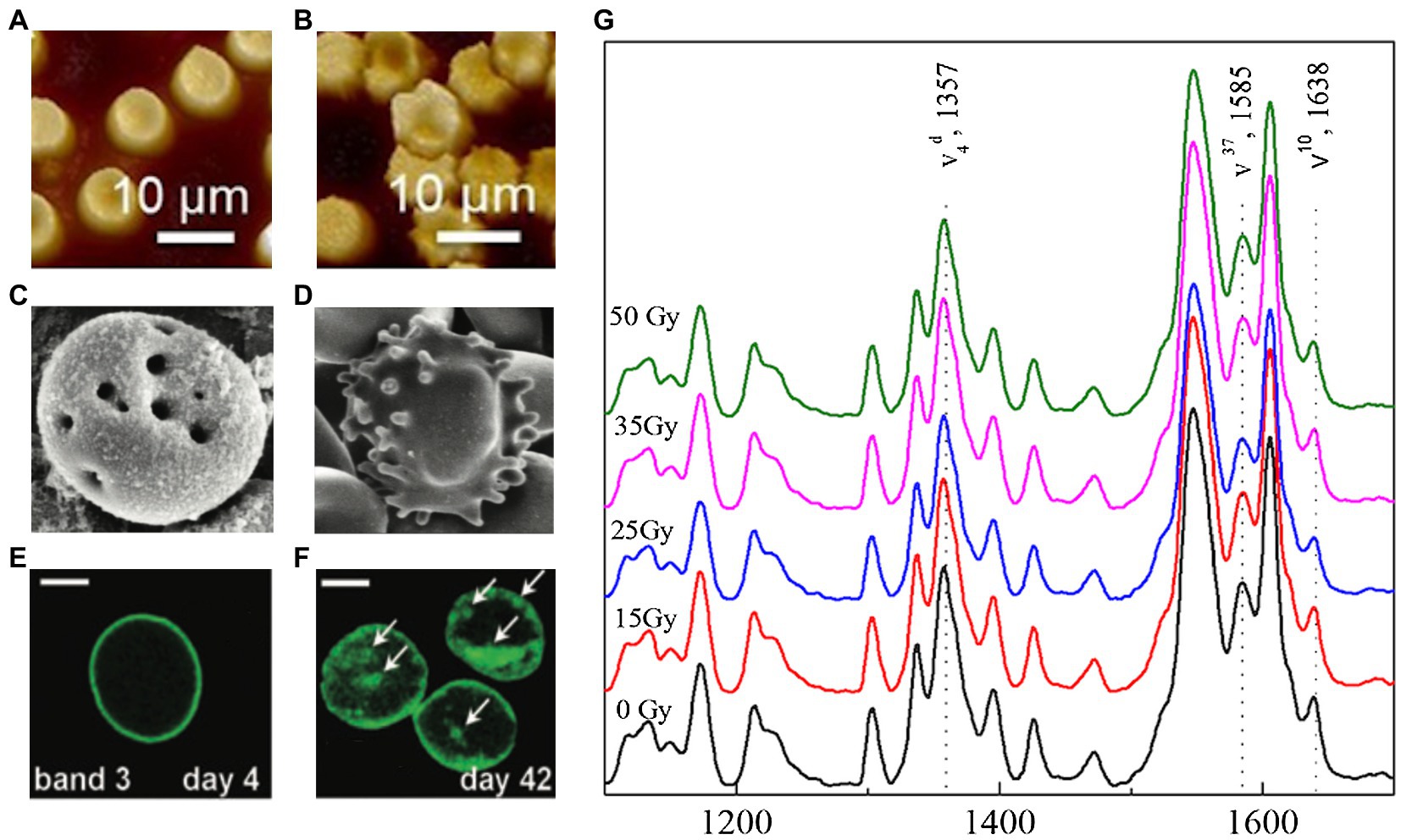
Figure 1. Changes in red blood cells (RBCs) during storage and irradiation. Atomic force microscopy images showing the nanostructure of RBC membrane for (A) non-irradiated erythrocytes with homogeneous distribution in shape and size, and (B) irradiated erythrocytes with echinocytic morphologies and heterogeneous size distributions. The effects of storage revealed by scanning electron microscopy micrographs of erythrocytes stored after 28 days: (C) showing early signs of hemolysis and tiny pores in the membrane and (D) RBC with echinocytic morphology. The biochemical modifications induced by storage are visible in the fluorescence micrograph: (E) showing the band 3 distribution at day 4, and (F) the protein modifications associated with band 3 at day 42. The state of Hb’s oxygenation in RBC subjected to gamma radiation and stored can be tracked with Raman spectra. (G) Raman spectrum of Hb after 13 days of storage and at different doses of gamma radiation (15, 25, 35, and 50 Gy). In both cases, no appreciable changes related to oxygen in the heme group configuration are detected. Figures were modified from (A-B) Santacruz-Gomez et al. (2016), (C-D) Mustafa et al. (2016), (E-F) Antonelou et al. (2010), and (G) Acosta-Elías et al. (2017).
Implications of RBC Membrane Changes
The main issues in using both irradiated and stored blood are closely related to changes in the biomechanical fingerprint of RBC. This fact impacts the structural quality of RBC for a transfusion and the shelf life of treated blood. The impaired function of RBC also is a serious concern that deserves more profound research. Recently, Acosta-Elías et al. (2017) showed that both storage time and radiation modify one of the most important mechanical properties, the cell surface roughness. In non-irradiated erythrocytes, storage from 0 to 13 days promotes a drastic decrease in the roughness from 2.1 to 0.82 nm. This loss of roughness is related to stiffness and then to the rupture susceptibility of the RBC membrane. Under irradiation, this phenomenon is more evident. For a dose around 50 Gy, RBC membrane roughness values fall among 1.1–0.73 nm. The distribution of the biomechanical response over the membrane associated with the change of elastic properties permits the discrimination of healthy RBC. In this case, RBC is stiffer in its center and softer at the cell periphery, as demonstrated by Ciasca et al. (2015). Another factor that plays a determining role in cell surface nanomechanics is the generation of nanovesicles. The biochemical change promotes the transformation of RBC to spherocytes. These cells have an altered lipid composition that makes the RBC stiffer (Dumitru et al., 2018).
In the medical praxis, nanovesicle release can contribute to posttransfusion effects by stimulating an inflammatory response from the body and causing the blood to coagulate due to the externalized phosphatidylserine (Lentz, 2003; Bosman et al., 2005). The hemoglobin within these vesicles can reduce nitric oxide (NO) availability in the recipient by binding to it, leading to the loss of the vasoregulatory function of NO in the vessels. Reduced NO in the recipient can result in impaired endothelial function, platelet aggregation, and oxidative damage. Prolonged blood storage causes potassium accumulation in the supernatant, linked to cardiac arrhythmia in patients. This excess of potassium means that a particular recipient, such as pediatric patients or heart bypass surgery, cannot be transfused without first removing the excess of potassium (Donadee et al., 2011; Kanias and Gladwin, 2012; Alaarg et al., 2013; Mustafa et al., 2016).
In summary, the quality of blood may be hampered by nanoscales changes on the RBC membrane, reducing transfusion’s effectivity. The required assessment of all biochemical parameters can be accomplished with techniques involving the traditional clinical analysis and nanomechanical-chemical microscopy.
Discussion and Remarks
The study of the complex biochemical changes on RBC triggered by storing and irradiation stresses may be elucidated by using a set of micro- and nanomicroscopy techniques. In particular, the combination (sequential or simultaneous) of Raman spectroscopy and AFM imaging from the same area in irradiated RBC is a promising alternative. This composed technique may permit the measurement of nanostructured cellular changes and their relation to the conformation of the molecule structure. Hence, generating information on biochemical phenomena affecting the integrity and function of blood cell components is of particular relevance to public health today and remains an active area of research.
Author Contributions
AL-C, AA-M, AG-E, ES-C, MM-R, and MP-M drafted the work and revised critically for important intellectual content, wrote the paper, and performed the final review of the manuscript. KS-G, MA-E, BC-M, DS-P, OA-B, and AB-E contributed to the conception of the work and performed the final review of the manuscript. All authors contributed to the article and approved the submitted version.
Funding
This research has received funding from CONACYT (Mexico) under Contract PDCPN2014-01/248982 and fellowship AL-C.
Conflict of Interest
The authors declare that the research was conducted in the absence of any commercial or financial relationships that could be construed as a potential conflict of interest.
Acknowledgments
The authors are pleased to acknowledge Sofía Navarro-Espinoza for her technical assistance. Results of this paper are part of a PhD thesis by AL-C under the co-advice of MP-M and MA-E. Supervision of the thesis was conducted by AG-E.
References
Acosta-Elías, M., Sarabia-Sainz, A., Pedroso-Santana, S., Silva-Campa, E., Santacruz-Gomez, K., Angulo-Molina, A., et al. (2015). Carboxylated nanodiamond and re-oxygenation process of gamma irradiated red blood cells. Phys. Status Solidi A 212, 2437–2444. doi: 10.1002/pssa.201532197
Acosta-Elías, M. A., Burgara-Estrella, A. J., Sarabia-Sainz, J. A.-I., Silva-Campa, E., Angulo-Molina, A., Santacruz-Gómez, K. J., et al. (2017). Nano alterations of membrane structure on both γ-irradiated and stored human erythrocytes. Int. J. Radiat. Biol. 93, 1306–1311. doi: 10.1080/09553002.2017.1393581
Adams, F., Bellairs, G. R., Bird, A. R., and Oguntibeju, O. O. (2018). Metabolic effects occurring in irradiated and non-irradiated red blood cellular components for clinical transfusion practice: an in vitro comparison. Afr. J. Lab. Med. 7:606. doi: 10.4102/ajlm.v7i1.606
Agarwal, P., Ray, V., Choudhury, N., and Chaudhary, R. (2005). Effect of pre-storage gamma irradiation on red blood cells. Indian J. Med. Res. 122, 385–387.
Alaarg, A., Schiffelers, R., van Solinge, W. W., and Van Wijk, R. (2013). Red blood cell vesiculation in hereditary hemolytic anemia. Front. Physiol. 4:365. doi: 10.3389/fphys.2013.00365
AlZahrani, K., and Al-Sewaidan, H. A. (2017). Nanostructural changes in the cell membrane of gamma-irradiated red blood cells. Indian J. Hematol. Blood Transfus. 33, 109–115. doi: 10.1007/s12288-016-0657-z
Anand, A., Dzik, W., Imam, A., and Sadrzadeh, S. (1997). Radiation-induced red cell damage: role of reactive oxygen species. Transfusion 37, 160–165. doi: 10.1046/j.1537-2995.1997.37297203518.x
Antonelou, M. H., Kriebardis, A. G., Stamoulis, K. E., Economou-Petersen, E., Margaritis, L. H., and Papassideri, I. S. (2010). Red blood cell aging markers during storage in citrate-phosphate-dextrose–saline-adenine-glucose-mannitol. Transfusion 50, 376–389. doi: 10.1111/j.1537-2995.2009.02449.x
Antonelou, M. H., and Seghatchian, J. (2016). Insights into red blood cell storage lesion: toward a new appreciation. Transfus. Apher. Sci. 55, 292–301. doi: 10.1016/j.transci.2016.10.019
Antwi-Baffour, S., Adjei, J. K., Tsyawo, F., Kyeremeh, R., Botchway, F. A., and Seidu, M. A. (2019). A study of the change in sodium and potassium ion concentrations in stored donor blood and their effect on electrolyte balance of recipients. Biomed. Res. Int. 2019:8162975. doi: 10.1155/2019/8162975
Arias, C. F., and Arias, C. F. (2017). How do red blood cells know when to die? R. Soc. Open Sci. 4:160850. doi: 10.1098/rsos.160850
Asghari-Khiavi, M., Wood, B. R., Mechler, A., Bambery, K. R., Buckingham, D. W., Cooke, B. M., et al. (2010). Correlation of atomic force microscopy and Raman micro-spectroscopy to study the effects of ex vivo treatment procedures on human red blood cells. Analyst 135, 525–530. doi: 10.1039/b919245j
Atkins, C. G., Buckley, K., Blades, M. W., and Turner, R. F. (2017). Raman spectroscopy of blood and blood components. Appl. Spectrosc. 71, 767–793. doi: 10.1177/0003702816686593
Barshtein, G., Arbell, D., Livshits, L., and Gural, A. (2018). Is it possible to reverse the storage-induced lesion of red blood cells? Front. Physiol. 9:914. doi: 10.3389/fphys.2018.00914
Berezina, T. L., Zaets, S. B., Morgan, C., Spillert, C. R., Kamiyama, M., Spolarics, Z., et al. (2002). Influence of storage on red blood cell rheological properties. J. Surg. Res. 102, 6–12. doi: 10.1006/jsre.2001.6306
Bhaduri, B., Kandel, M., Brugnara, C., Tangella, K., and Popescu, G. (2014). Optical assay of erythrocyte function in banked blood. Sci. Rep. 4, 1–6. doi: 10.1038/srep06211
Bosman, G., Willekens, F., and Werre, J. (2005). Erythrocyte aging: a more than superficial resemblance to apoptosis? Cell. Physiol. Biochem. 16, 1–8. doi: 10.1159/000087725
Bosman, G. J., Lasonder, E., Luten, M., Roerdinkholder-Stoelwinder, B., Novotný, V. M., Bos, H., et al. (2008). The proteome of red cell membranes and vesicles during storage in blood bank conditions. Transfusion 48, 827–835. doi: 10.1111/j.1537-2995.2007.01630.x
Buckley, K., Atkins, C., Chen, D., Schulze, H., Devine, D., Blades, M., et al. (2016). Non-invasive spectroscopy of transfusable red blood cells stored inside sealed plastic blood-bags. Analyst 141, 1678–1685. doi: 10.1039/C5AN02461G
Burgara-Estrella, A. J., Acosta-Elías, M. A., Álvarez-Bajo, O., Silva-Campa, E., Angulo-Molina, A., Rodríguez-Hernández, I. d. C., et al. (2020). Atomic force microscopy and Raman spectra profile of blood components associated with exposure to cigarette smoking. RSC Adv. 10, 11971–11981. doi: 10.1039/D0RA01384F
Campanella, M. E., Chu, H., and Low, P. S. (2005). Assembly and regulation of a glycolytic enzyme complex on the human erythrocyte membrane. Proc. Natl. Acad. Sci. 102, 2402–2407. doi: 10.1073/pnas.0409741102
Chasis, J. A., and Mohandas, N. (1986). Erythrocyte membrane deformability and stability: two distinct membrane properties that are independently regulated by skeletal protein associations. J. Cell Biol. 103, 343–350. doi: 10.1083/jcb.103.2.343
Choo-Smith, L. P., Edwards, H., Endtz, H. P., Kros, J., Heule, F., Barr, H., et al. (2002). Medical applications of Raman spectroscopy: from proof of principle to clinical implementation. Biopolymers 67, 1–9. doi: 10.1002/bip.10064
Chu, H., Puchulu-Campanella, E., Galan, J. A., Tao, W. A., Low, P. S., and Hoffman, J. F. (2012). Identification of cytoskeletal elements enclosing the ATP pools that fuel human red blood cell membrane cation pumps. Proc. Natl. Acad. Sci. 109, 12794–12799. doi: 10.1073/pnas.1209014109
Ciasca, G., Papi, M., Di Claudio, S., Chiarpotto, M., Palmieri, V., Maulucci, G., et al. (2015). Mapping viscoelastic properties of healthy and pathological red blood cells at the nanoscale level. Nanoscale 7, 17030–17037. doi: 10.1039/C5NR03145A
Collazo, A., Bricaud, O., and Desai, K. (2005). Use of confocal microscopy in comparative studies of vertebrate morphology. Methods Enzymol. 395, 521–543. doi: 10.1016/S0076-6879(05)95027-1
Cui, Y., Gao, Y., Yang, H., Xiong, C., Xia, G., and Wang, D. (1999). Apoptosis of circulating lymphocytes induced by whole body gamma-irradiation and its mechanism. J. Environ. Pathol. Toxicol. Oncol. 18, 185–189.
D’Alessandro, A., Gray, A. D., Szczepiorkowski, Z. M., Hansen, K., Herschel, L. H., and Dumont, L. J. (2017). Red blood cell metabolic responses to refrigerated storage, rejuvenation, and frozen storage. Transfusion 57, 1019–1030. doi: 10.1111/trf.14034
D’Alessandro, A., Kriebardis, A. G., Rinalducci, S., Antonelou, M. H., Hansen, K. C., Papassideri, I. S., et al. (2015). An update on red blood cell storage lesions, as gleaned through biochemistry and omics technologies. Transfusion 55, 205–219. doi: 10.1111/trf.12804
de Korte, D., Thibault, L., Handke, W., Harm, S. K., Morrison, A., Fitzpatrick, A., et al. (2018). Timing of gamma irradiation and blood donor sex influences in vitro characteristics of red blood cells. Transfusion 58, 917–926. doi: 10.1111/trf.14481
Depond, M., Henry, B., Buffet, P., and Ndour, P. A. (2020). Methods to investigate the deformability of RBC during malaria. Front. Physiol. 10:1613. doi: 10.3389/fphys.2019.01613
Dinarelli, S., Longo, G., Krumova, S., Todinova, S., Danailova, A., Taneva, S., et al. (2018). Insights into the morphological pattern of erythrocytes' aging: coupling quantitative AFM data to microcalorimetry and Raman spectroscopy. J. Mol. Recognit. 31:e2732. doi: 10.1002/jmr.2732
Donadee, C., Raat, N. J., Kanias, T., Tejero, J., Lee, J. S., Kelley, E. E., et al. (2011). Nitric oxide scavenging by red blood cell microparticles and cell-free hemoglobin as a mechanism for the red cell storage lesion. Circulation 124, 465–476. doi: 10.1161/CIRCULATIONAHA.110.008698
Dulińska, I., Targosz, M., Strojny, W., Lekka, M., Czuba, P., Balwierz, W., et al. (2006). Stiffness of normal and pathological erythrocytes studied by means of atomic force microscopy. J. Biochem. Biophys. Methods 66, 1–11. doi: 10.1016/j.jbbm.2005.11.003
Dumitru, A. C., Poncin, M. A., Conrard, L., Dufrêne, Y. F., Tyteca, D., and Alsteens, D. (2018). Nanoscale membrane architecture of healthy and pathological red blood cells. Nanoscale Horiz. 3, 293–304. doi: 10.1039/C7NH00187H
Freitas Leal, J. K., Lasonder, E., Sharma, V., Schiller, J., Fanelli, G., Rinalducci, S., et al. (2020). Vesiculation of red blood cells in the blood bank: a multi-omics approach towards identification of causes and consequences. Proteomes 8:6. doi: 10.3390/proteomes8020006
Gautam, R., Oh, J.-Y., Marques, M. B., Dluhy, R. A., and Patel, R. P. (2018). Characterization of storage-induced red blood cell hemolysis using Raman spectroscopy. Lab. Med. 49, 298–310. doi: 10.1093/labmed/lmy018
Geekiyanage, N. M., Balanant, M. A., Sauret, E., Saha, S., Flower, R., Lim, C. T., et al. (2019). A coarse-grained red blood cell membrane model to study stomatocyte-discocyte-echinocyte morphologies. PLoS One 14:e0215447. doi: 10.1371/journal.pone.0215447
Girasole, M., Cricenti, A., Generosi, R., Congiu-Castellano, A., Boumis, G., and Amiconi, G. (2001). Artificially induced unusual shape of erythrocytes: an atomic force microscopy study. J. Microsc. 204, 46–52. doi: 10.1046/j.1365-2818.2001.00937.x
Girasole, M., Pompeo, G., Cricenti, A., Congiu-Castellano, A., Andreola, F., Serafino, A., et al. (2007). Roughness of the plasma membrane as an independent morphological parameter to study RBCs: a quantitative atomic force microscopy investigation. Biochim. Biophys. Acta Biomembr. 1768, 1268–1276. doi: 10.1016/j.bbamem.2007.01.014
Girasole, M., Pompeo, G., Cricenti, A., Longo, G., Boumis, G., Bellelli, A., et al. (2010). The how, when, and why of the aging signals appearing on the human erythrocyte membrane: an atomic force microscopy study of surface roughness. Nanomedicine 6, 760–768. doi: 10.1016/j.nano.2010.06.004
Góes, E., Ottoboni, M., Palma, P., Morais, F., Pelá, C., Borges, J., et al. (2008). Quality control of blood irradiation with a teletherapy unit: damage to stored red blood cells after cobalt-60 gamma irradiation. Transfusion 48, 332–340. doi: 10.1111/j.1537-2995.2007.01527.x
Hirayama, J., Abe, H., Azuma, H., and Ikeda, H. (2005). Leakage of potassium from red blood cells following gamma ray irradiation in the presence of dipyridamole, trolox, human plasma or mannitol. Biol. Pharm. Bull. 28, 1318–1320. doi: 10.1248/bpb.28.1318
Huang, Y. X., Wu, Z. J., Mehrishi, J., Huang, B. T., Chen, X. Y., Zheng, X. J., et al. (2011). Human red blood cell aging: correlative changes in surface charge and cell properties. J. Cell. Mol. Med. 15, 2634–2642. doi: 10.1111/j.1582-4934.2011.01310.x
Huang-Doran, I., Zhang, C.-Y., and Vidal-Puig, A. (2017). Extracellular vesicles: novel mediators of cell communication in metabolic disease. Trends Endocrinol. Metab. 28, 3–18. doi: 10.1016/j.tem.2016.10.003
Huisjes, R., Bogdanova, A., van Solinge, W. W., Schiffelers, R. M., Kaestner, L., and Van Wijk, R. (2018). Squeezing for life–properties of red blood cell deformability. Front. Physiol. 9:656. doi: 10.3389/fphys.2018.00656
Jay, A. (1975). Geometry of the human erythrocyte. I. Effect of albumin on cell geometry. Biophys. J. 15, 205–222. doi: 10.1016/S0006-3495(75)85812-7
Kang, L. L., Huang, Y. X., Liu, W. J., Zheng, X. J., Wu, Z. J., and Luo, M. (2008). Confocal Raman microscopy on single living young and old erythrocytes. Biopolymers 89, 951–959. doi: 10.1002/bip.21042
Kanias, T., and Acker, J. P. (2010). Biopreservation of red blood cells–the struggle with hemoglobin oxidation. FEBS J. 277, 343–356. doi: 10.1111/j.1742-4658.2009.07472.x
Kanias, T., and Gladwin, M. T. (2012). Nitric oxide, hemolysis, and the red blood cell storage lesion: interactions between transfusion, donor, and recipient. Transfusion 52, 1388–1392. doi: 10.1111/j.1537-2995.2012.03748.x
Karsten, E., and Herbert, B. R. (2019). The emerging role of red blood cells in cytokine signalling and modulating immune cells. Blood Rev. 41:100644. doi: 10.1016/j.blre.2019.100644
Kim, J., Lee, H., and Shin, S. (2015). Advances in the measurement of red blood cell deformability: a brief review. J. Cell. Biotechnol. 1, 63–79. doi: 10.3233/JCB-15007
Koch, C. G., Duncan, A. I., Figueroa, P., Dai, L., Sessler, D. I., Frank, S. M., et al. (2019). Real age: red blood cell aging during storage. Ann. Thorac. Surg. 107, 973–980. doi: 10.1016/j.athoracsur.2018.08.073
Kor, D. J., Van Buskirk, C. M., and Gajic, O. (2009). Red blood cell storage lesion. Bosn. J. Basic Med. Sci. 9, S21–S27. doi: 10.17305/bjbms.2009.2750
Kozlova, E., Chernysh, A., Moroz, V., Sergunova, V., Gudkova, O., and Manchenko, E. (2017). Morphology, membrane nanostructure and stiffness for quality assessment of packed red blood cells. Sci. Rep. 7:7846. doi: 10.1038/s41598-017-08255-9
Kozlova, E., Chernysh, A., Sergunova, V., Gudkova, O., Manchenko, E., and Kozlov, A. (2018). Atomic force microscopy study of red blood cell membrane nanostructure during oxidation-reduction processes. J. Mol. Recognit. 31:e2724. doi: 10.1002/jmr.2724
Kozlova, E., Chernysh, A., Sergunova, V., Manchenko, E., Moroz, V., and Kozlov, A. (2019). Conformational distortions of the red blood cell Spectrin matrix nanostructure in response to temperature changes in vitro. Scanning 2019:8218912. doi: 10.1155/2019/8218912
Leal, J. K., Adjobo-Hermans, M. J., and Bosman, G. J. (2018). Red blood cell homeostasis: mechanisms and effects of microvesicle generation in health and disease. Front. Physiol. 9:703. doi: 10.3389/fphys.2018.00703
Lentz, B. R. (2003). Exposure of platelet membrane phosphatidylserine regulates blood coagulation. Prog. Lipid Res. 42, 423–438. doi: 10.1016/S0163-7827(03)00025-0
Li, H., and Lykotrafitis, G. (2015). Vesiculation of healthy and defective red blood cells. Phys. Rev. E 92:012715. doi: 10.1103/PhysRevE.92.012715
Li, N., Li, S., Guo, Z., Zhuang, Z., Li, R., Xiong, K., et al. (2012). Micro-Raman spectroscopy study of the effect of mid-ultraviolet radiation on erythrocyte membrane. J. Photochem. Photobiol. B Biol. 112, 37–42. doi: 10.1016/j.jphotobiol.2012.04.005
Lima, C. N., Moura, D. S., Silva, Y. S., Souza, T. H., Crisafuli, F. A., Silva, D. C., et al. (2020). Evaluating viscoelastic properties and membrane electrical charges of red blood cells with optical tweezers and cationic quantum dots–applications to β-thalassemia intermedia hemoglobinopathy. Colloids Surf. B: Biointerfaces 186:110671. doi: 10.1016/j.colsurfb.2019.110671
Lux, S. E. (2016). Anatomy of the red cell membrane skeleton: unanswered questions. Blood 127, 187–199. doi: 10.1182/blood-2014-12-512772
Manduzio, P. (2018). Transfusion-associated graft-versus-host disease: a concise review. Hematol. Rep. 10:7724. doi: 10.4081/hr.2018.7724
Marzec, K. M., Rygula, A., Wood, B. R., Chlopicki, S., and Baranska, M. (2015). High-resolution Raman imaging reveals spatial location of heme oxidation sites in single red blood cells of dried smears. J. Raman Spectrosc. 46, 76–83. doi: 10.1002/jrs.4600
Moroff, G., and Luban, N. (1997). The irradiation of blood and blood components to prevent graft-versus-host disease: technical issues and guidelines. Transfus. Med. Rev. 11, 15–26. doi: 10.1016/S0887-7963(97)80006-5
Moroff, G., and Luban, N. L. (1994). The influence of gamma irradiation on red cell and platelet properties. Transfus. Sci. 15, 141–148. doi: 10.1016/0955-3886(94)90088-4
Moroz, V. V., Chernysh, A. M., Kozlova, E. K., Borshegovskaya, P. Y., Bliznjuk, U. A., Rysaeva, R. M., et al. (2010). Comparison of red blood cell membrane microstructure after different physicochemical influences: atomic force microscope research. J. Crit. Care 25, 539.e1–539.e12. doi: 10.1016/j.jcrc.2010.02.007
Mukherjee, R., Saha, M., Routray, A., and Chakraborty, C. (2015). Nanoscale surface characterization of human erythrocytes by atomic force microscopy: a critical review. IEEE Trans. Nanobiosci. 14, 625–633. doi: 10.1109/TNB.2015.2424674
Mustafa, I., Al Marwani, A., Mamdouh Nasr, K., Abdulla Kano, N., and Hadwan, T. (2016). Time dependent assessment of morphological changes: leukodepleted packed red blood cells stored in SAGM. Biomed. Res. Int. 2016:4529434. doi: 10.1155/2016/4529434
Oore-ofe, O., Soma, P., Buys, A. V., Debusho, L. K., and Pretorius, E. (2017). Characterizing pathology in erythrocytes using morphological and biophysical membrane properties: relation to impaired hemorheology and cardiovascular function in rheumatoid arthritis. Biochim. Biophys. Acta Biomembr. 1859, 2381–2391. doi: 10.1016/j.bbamem.2017.09.014
Parshina, E. Y., Sarycheva, A., Yusipovich, A., Brazhe, N., Goodilin, E., and Maksimov, G. (2013). Combined Raman and atomic force microscopy study of hemoglobin distribution inside erythrocytes and nanoparticle localization on the erythrocyte surface. Laser Phys. Lett. 10:075607. doi: 10.1088/1612-2011/10/7/075607
Piccin, A., Van Schilfgaarde, M., and Smith, O. (2015). The importance of studying red blood cells microparticles. Blood Transfus. 13, 172–173. doi: 10.2450/2014.0276-14
Ramirez, A., Woodfield, D., Scott, R., and McLachlan, J. (1987). High potassium levels in stored irradiated blood. Transfusion 27, 444–445. doi: 10.1046/j.1537-2995.1987.27587320546.x
Reilly, M., Bruno, C. D., Prudencio, T. M., Ciccarelli, N., Guerrelli, D., Nair, R., et al. (2020). Potential consequences of the red blood cell storage lesion on cardiac electrophysiology. J. Am. Heart Assoc. 9:e017748. doi: 10.1161/JAHA.120.017748
Reinhart, S. A., Schulzki, T., and Reinhart, W. H. (2015). Albumin reverses the echinocytic shape transformation of stored erythrocytes. Clin. Hemorheol. Microcirc. 60, 437–449. doi: 10.3233/CH-141899
Reinhart, W., and Nagy, C. (1995). Albumin affects erythrocyte aggregation and sedimentation. Eur. J. Clin. Investig. 25, 523–528. doi: 10.1111/j.1365-2362.1995.tb01739.x
Rico, L. G., Juncà, J., Ward, M. D., Bradford, J. A., Bardina, J., and Petriz, J. (2018). Acoustophoretic orientation of red blood cells for diagnosis of red cell health and pathology. Sci. Rep. 8:15705. doi: 10.1038/s41598-018-33411-0
Santacruz-Gomez, K., Silva-Campa, E., Alvarez-Garcia, S., Mata-Haro, V., Soto-Puebla, D., and Pedroza-Montero, M. (2014). An AFM approach of RBC micro and nanoscale topographic features during storage. World Acad. Sci. Eng. Technol. Int. J. 8, 449–452. doi: 10.5281/zenodo.1094122
Santacruz-Gomez, K., Silva-Campa, E., Melendrez-Amavizca, R., Arce, F. T., Mata-Haro, V., Landon, P., et al. (2016). Carboxylated nanodiamonds inhibit γ-irradiation damage of human red blood cells. Nanoscale 8, 7189–7196. doi: 10.1039/C5NR06789H
Sellins, K. S., and Cohen, J. (1987). Gene induction by gamma-irradiation leads to DNA fragmentation in lymphocytes. J. Immunol. 139, 3199–3206.
Spyratou, E., Dilvoi, M., Patatoukas, G., Platoni, K., Makropoulou, M., and Efstathopoulos, E. P. (2019). Probing the effects of ionizing radiation on young's modulus of human erythrocytes cytoskeleton using atomic force microscopy. J. Med. Phys. 44, 113–117. doi: 10.4103/jmp.JMP_95_18
Sut, C., Tariket, S., Chou, M. L., Garraud, O., Laradi, S., Hamzeh-Cognasse, H., et al. (2017). Duration of red blood cell storage and inflammatory marker generation. Blood Transfus. 15, 145–152. doi: 10.2450/2017.0343-16
Tabatabaei, G., Jaafar, M. S., and Abdullah, W. Z. (2016). The effects of ultraviolet light irradiation on hematological and morphological characteristics and potassium level of human blood for transfusion associated graft versus host disease prevention. Front. Biomed. Technol. 3, 80–85.
Talstad, I., Scheie, P., Dalen, H., and Röli, J. (1983). Influence of plasma proteins on erythrocyte morphology and sedimentation. Scand. J. Haematol. 31, 478–484. doi: 10.1111/j.1600-0609.1983.tb01547.x
Taqi, A. H., Faraj, K., Zaynal, S., Said, J., and Hameed, A. (2019). Effects of high doses of X-Ray on hematological parameters and morphology of red blood cells in human blood. Iran. J. Med. Phys. 16, 112–119. doi: 10.22038/ijmp.2018.31184.1366
Usry, R. T., Moore, G. L., and Manalo, F. W. (1975). Morphology of stored, rejuvenated human erythrocytes. Vox Sang. 28, 176–183. doi: 10.1111/j.1423-0410.1975.tb02756.x
Wallas, C. (1979). Sodium and potassium changes in blood bank stored human erythrocytes. Transfusion 19, 210–215. doi: 10.1046/j.1537-2995.1979.19279160297.x
Walpurgis, K., Kohler, M., Thomas, A., Wenzel, F., Geyer, H., Schänzer, W., et al. (2013). Effects of gamma irradiation and 15 days of subsequent ex vivo storage on the cytosolic red blood cell proteome analyzed by 2D-DIGE and Orbitrap MS. Proteomics Clin. Appl. 7, 561–570. doi: 10.1002/prca.201300009
Weinmann, M., Hoffmann, W., Rodegerdts, E., and Bamberg, M. (2000). Biological effects of ionizing radiation on human blood compounds ex vivo. J. Cancer Res. Clin. Oncol. 126, 584–588. doi: 10.1007/PL00008468
Werre, J., Willekens, F., Bosch, F., De Haans, L., Van Der Vegt, S., Van den Bos, A., et al. (2004). The red cell revisited–matters of life and death. Cell. Mol. Biol. 50, 139–145.
Wood, B. R., Tait, B., and McNaughton, D. (2001). Micro-Raman characterisation of the R to T state transition of haemoglobin within a single living erythrocyte. Biochim. Biophys. Acta Mol. Cell. Res. 1539, 58–70. doi: 10.1016/S0167-4889(01)00089-1
Xu, D., Peng, M., Zhang, Z., Dong, G., Zhang, Y., and Yu, H. (2012). Study of damage to red blood cells exposed to different doses of γ-ray irradiation. Blood Transfus. 10, 321–330. doi: 10.2450/2012.0076-11
Yoshida, T., Prudent, M., and D’Alessandro, A. (2019). Red blood cell storage lesion: causes and potential clinical consequences. Blood Transfus. 17, 27–52. doi: 10.2450/2019.0217-18
Zbikowska, H. M., Antosik, A., Szejk, M., Bijak, M., Olejnik, A. K., Saluk, J., et al. (2014). Does quercetin protect human red blood cell membranes against γ-irradiation? Redox Rep. 19, 65–71. doi: 10.1179/1351000213Y.0000000074
Zhang, D. X., Kiomourtzis, T., Lam, C. K., and Le, M. T. (2019). “The biology and therapeutic applications of red blood cell extracellular vesicles” in Erythrocyte. ed. A. Tombak (UK: IntechOpen Limited), 851–866.
Keywords: RBC membrane, nanoalterations, ionizing radiation, blood storage, confocal microscopy, Raman, scanning electron microscopy, atomic force microscopy
Citation: López-Canizales AM, Angulo-Molina A, Garibay-Escobar A, Silva-Campa E, Mendez-Rojas MA, Santacruz-Gómez K, Acosta-Elías M, Castañeda-Medina B, Soto-Puebla D, Álvarez-Bajo O, Burgara-Estrella A and Pedroza-Montero M (2021) Nanoscale Changes on RBC Membrane Induced by Storage and Ionizing Radiation: A Mini-Review. Front. Physiol. 12:669455. doi: 10.3389/fphys.2021.669455
Edited by:
Sergey S. Shevkoplyas, University of Houston, United StatesCopyright © 2021 López-Canizales, Angulo-Molina, Garibay-Escobar, Silva-Campa, Mendez-Rojas, Santacruz-Gómez, Acosta-Elías, Castañeda-Medina, Soto-Puebla, Álvarez-Bajo, Burgara-Estrella and Pedroza-Montero. This is an open-access article distributed under the terms of the Creative Commons Attribution License (CC BY). The use, distribution or reproduction in other forums is permitted, provided the original author(s) and the copyright owner(s) are credited and that the original publication in this journal is cited, in accordance with accepted academic practice. No use, distribution or reproduction is permitted which does not comply with these terms.
*Correspondence: Martín Pedroza-Montero, bWFydGluLnBlZHJvemFAdW5pc29uLm14; Aracely Angulo-Molina, YXJhY2VseS5hbmd1bG9AdW5pc29uLm14