- 1Laboratory of Applied Physiology, Department of Biological and Environmental Sciences and Technologies, University of Salento, Lecce, Italy
- 2Department of Biological Sciences, University of Bergen, Bergen, Norway
Food intake is a vital process that supplies necessary energy and essential nutrients to the body. Information regarding luminal composition in the gastrointestinal tract (GIT) collected through mechanical and nutrient sensing mechanisms are generally conveyed, in both mammals and fish, to the hypothalamic neurocircuits. In this context, ghrelin, the only known hormone with an orexigenic action, and the intestinal peptide transporters 1 and 2, involved in absorption of dietary di- and tripeptides, exert important and also integrated roles for the nutrient uptake. Together, both are potentially involved in signaling pathways that control food intake originating from different segments of the GIT. However, little is known about the role of different paralogs and their response to fasting. Therefore, after 3 weeks of acclimatization, 12 Atlantic salmon (Salmo salar) post-smolt were fasted for 4 days to explore the gastrointestinal response in comparison with fed control (n = 12). The analysis covered morphometric (weight, length, condition factor, and wet content/weight fish %), molecular (gene expression variations), and correlation analyses. Such short-term fasting is a common and recommended practice used prior to any handling in commercial culture of the species. There were no statistical differences in length and weight but a significant lower condition factor in the fasted group. Transcriptional analysis along the gastrointestinal segments revealed a tendency of downregulation for both paralogous genes slc15a1a and slc15a1b and with significant lowered levels in the pyloric ceca for slc15a1a and in the pyloric ceca and midgut for slc15a1b. No differences were found for slc15a2a and slc15a2b (except a higher expression of the fasted group in the anterior midgut), supporting different roles for slc15 paralogs. This represents the first report on the effects of fasting on slc15a2 expressed in GIT in teleosts. Transcriptional analysis of ghrelin splicing variants (ghrl-1 and ghrl-2) showed no difference between treatments. However, correlation analysis showed that the mRNA expression for all genes (restricted to segment with the highest levels) were affected by the residual luminal content. Overall, the results show minimal effects of 4 days of induced fasting in Atlantic salmon, suggesting that more time is needed to initiate a large GIT response.
Introduction
Food intake is an important mechanism that allows for acquiring all necessary energy and essential nutrients for subsistence, activity, and growth (Schwartz et al., 2000). In this process, the gastrointestinal tract (GIT) serves a key role, being constantly exposed to vastly different chemical substances, ions, micro-/macronutrients, and microorganisms. The regulation of the digestive and absorption processes is based on information about luminal content and filling, which are first conveyed through nutrient sensing and stretch sensory mechanisms that underlie the initiation of neuronal and humoral signals (Rønnestad et al., 2014, 2017; Daniel and Zietek, 2015; Zietek and Daniel, 2015; Conde-Sieira and Soengas, 2017) and then sent to the main regulatory centers of food intake, feeding behavior, and energy expenditure in the hypothalamic neurocircuits (both for mammals and fish) (Cerdá-Reverter and Canosa, 2009; Blouet and Schwartz, 2010; Morton et al., 2014), through orexigenic and anorexigenic neuropeptides (Volkoff, 2016; Conde-Sieira and Soengas, 2017; Delgado et al., 2017; Rønnestad et al., 2017).
Ghrelin (GHRL) is a hormone secreted mainly in the stomach and the only known to have a stimulating-appetite action (orexigenic) in all the vertebrate systems (Higgins et al., 2007; Patton and Mistlberger, 2013). In fish, Ghrl is mainly secreted in the stomach, as in mammals; however, for stomach-less species, such as the cyprinids goldfish (Carassius auratus) and zebrafish (Danio rerio), early studies confirmed the intestine to be the major site of ghrl expression and release (Unniappan et al., 2002; Olsson et al., 2008). Further to this, a recent study has shown that among agastric fish species, the ghrl gene is only found in the cyprinid family (Lie et al., 2018), which means that several of the known model teleost species like medaka (Oryzias latipes) and fugu (Takifugu rubripes) lack the gene coding for Ghrl. Comparative studies among fish species suggest that the generalized orexigenic role of Ghrl in appetite and food intake is not as clear as for mammals. In fact, anorexigenic and contradictory effects have been assessed in some species, such as Mozambique tilapia (Oreochromis mossambicus) (Peddu et al., 2009), rainbow trout (Oncorhynchus mykiss) (Jönsson et al., 2010; Velasco et al.,2016a,b), and Atlantic salmon (Salmo salar) (Murashita et al., 2009; Hevrøy et al., 2011; Vikesa et al., 2015), suggesting a probable species- and form-specific Ghrl role in the regulation of feeding and metabolism in fish (Volkoff, 2016).
During digestion of dietary proteins, a multitude of peptides of different sizes and composition can be found, and the intestinal peptide transporter 1 (PEPT1), also known as SoLute carrier family 15 member a1 (SLC15A1), serves a key role in the uptake of dietary amino acids in di- and tripeptide forms in all the vertebrates (Daniel, 2004; Smith et al., 2013a). Several of these absorbed peptides have been shown to have also a bioactive function in the complex mechanism of nutrient sensing and regulation of dietary metabolism (Rønnestad et al., 2014; Daniel and Zietek, 2015; Zietek and Daniel, 2015), suggesting that the PEPT1 is also involved in the gut–brain signaling axis. The peptide transporter has been identified and characterized in many vertebrate species, and lately, much attention has also been placed in lower vertebrates, teleost species included, where less is known. The studied fish species include zebrafish (Verri et al., 2003), Atlantic cod (Gadus morhua) (Rønnestad et al., 2007), European sea bass (Dicentrarchus labrax) (Sangaletti et al., 2009), Atlantic salmon (Rønnestad et al., 2010), Antarctic Icefish (Chionodraco hamatus) (Rizzello et al., 2013), and Grass Carp (Ctenopharyngodon idella) (Liu et al., 2013). The increased availability of genome sequences for teleosts has progressively clarified the co-presence of two transporters, as a result of an additional teleost-specific whole-genome duplication (WGD) event (Gonçalves et al., 2007; Bucking and Schulte, 2012; Con et al., 2017; Orozco et al., 2017; Chourasia et al., 2018). The two transporters are called Pept1a, expressed by slc15a1a gene, and Pept1b, expressed by slc15a1b gene, respectively. The emerging slc15a1a paralog has just recently been identified, and therefore, limited information is still available for this gene. So far, only two studies on teleost species are found, i.e., in zebrafish (Vacca et al., 2019) and in Atlantic salmon (Gomes et al., 2020). Consequently, the largest part of the data available so far on teleost species about the functional role of Pept1 proteins refers to the Pept1b type (Verri et al., 2010; Romano et al., 2014; Verri et al., 2017).
The intestinal peptide transporter peptide transporter 2 (PEPT2) is another plasma membrane transporter of di- and tripeptides belonging to the same Solute carrier family 15 (member a2) as PEPT1. PEPT2 is well characterized in mammals, where high expressions are found in the kidney, peripheral, and central nervous system, lung (Rubio-Aliaga and Daniel, 2002, 2008; Daniel and Rubio-Aliaga, 2003; Kamal et al., 2008), and GIT, where it seems to be not expressed by epithelial cells but, rather, by the enteric glial cells and tissue-resident macrophages (Rühl et al., 2005). As for Pept1, compared to mammals and higher vertebrates, there is little knowledge about this transporter in lower vertebrates. In teleost species, Pept2 has been, in fact, characterized only in zebrafish (Romano et al., 2006), whereas no data are available in Atlantic salmon so far.
Thus, based on the available information, it is likely that both Ghrl and Pept1 and/or Pept2 may be involved in signaling the quantitative and qualitative presence of nutrients in different sections of the GIT. Moreover, due to both teleost-specific and later salmonid WGD events (Davidson et al., 2010; Berthelot et al., 2014; Macqueen and Johnston, 2014; Pasquier et al., 2016), Atlantic salmon has several paralogs of these genes and, therefore, identifying any probable functional evolutionary adaptations of paralogs is also important. In fish, Pept1 represents an important part of the absorptive capacity of protein nitrogen for the animal, especially for teleosts, where it plays an important role in animal growth (Verri et al., 2011). Its messenger RNA (mRNA) expression is known to change in response to a fasting period: reduced expressions (for both paralogous genes) are found in 7 days fasted Nile tilapia (Oreochromis niloticus) (Huang et al., 2015), in both short- and long-term fasted zebrafish (Tian et al., 2015), in 6 days fasted juvenile Atlantic salmon (Rønnestad et al., 2010), and in sea bass (Dicentrarxus labrax) (Terova et al., 2009). The peptide hormone Ghrl has received attention in aquaculture since stimulation of food intake is important, particularly since fish meal has been replaced with plant-based dietary ingredients and the palatability reduced (Kousoulaki et al., 2013). Ghrl has shown orexigenic effects in induced fasting goldfish (Kang et al., 2011; Nisembaum et al., 2014) and in Nile tilapia (Schwandt et al., 2010), whereas low Ghrl plasma levels and contradictory effects are found in fasted Atlantic salmon (Hevrøy et al., 2011).
In the Atlantic salmon aquaculture production, a period of 2–4 days of fasting is a common and recommended practice prior to any handling, transportation, and harvest in order to allow the complete evacuation of the gut to minimize impacts on stress, metabolism, fish welfare, and even mortality (Waagbø et al., 2017). Moreover, fasting also ensures proper hygiene after harvest (Einen et al., 1998; Robb, 2008). To date, analysis on metabolic rate, stress response, and morphometric parameters on Atlantic salmon postsmolts has assessed that a fasting period up to 4 weeks has no negligible effects on salmon welfare (Hvas et al., 2020). To learn more about the GIT properties and how it responds to a standard fasting period, the effects of 4 days of induced fasting on morphometric parameters (length, weight, condition factor, and status of GIT digesta content) and on the transcriptional changes (ghrelin splicing variants and peptide transporters paralogs) along the GIT segments were evaluated. Moreover, how the presence/absence of the digesta in the GIT affects the mRNA expression of the investigated genes through correlation analysis was also assessed.
Materials and Methods
Ethics Statement
The experiment and sampling were conducted in accordance with Norwegian Animal Research Authority regulations and were approved by local representative of Animal Welfare at the Department of Biological Sciences, University of Bergen (Norway).
Experimental Design and Sampling
This study is part of a larger experiment where the main data on fish growth and performance were assessed (Kalananthan et al., 2020a). A subgroup of fish was used to evaluate the transcriptional changes of ghrelin splicing variants and peptide transporters paralogs along the GIT segments. In brief, Atlantic salmon postsmolt (ca. 250 g; n = 96) obtained from Engesund farm (Fitjar, Norway) were randomly distributed into two 2,000-L tanks (48 fish per tank) at the Industrial Lab fish facility (ILAB, Bergen, Norway). The rearing tanks were connected to a flow through system at 10°C with a salinity of 27 ppt, water flow of 16 L/min, oxygen saturation always above 80%, and under constant light (all parameters checked daily). Fish were fed to satiety with commercial dry feed pellets (Biomar intro 75 HH 50 mg Q) using an automatic feeder throughout 24 h. After 3 weeks of acclimatization, the two tanks were randomly labeled into two experimental groups; one tank was kept as control (fed group), i.e., fish fed normally as described above, whereas the other tank was kept as treated (fasted group) 21 fish per tank (263 ± 13.06 and 275.7 ± 15.68 g; mean ± SEM) were sampled as a baseline control. After 4 days, 12 fish were sampled from the fed group (281.41 ± 25.20 g; mean ± SEM) and 12 from the fasted group (256.66 ± 21.14 g, mean ± SEM) using a lethal dose of 200 mg/L of MS222 (tricaine methanesulfonate, Scan-Vacc, Hvam, Norway). Length (L) and weight (W) were recorded and used to calculate Fulton’s condition factor (K) using the following equation (Froese, 2006):
where L is expressed in cm and W in g.
Gastrointestinal tract was carefully, but rapidly, dissected by cutting at the extremity of the esophagus and hindgut (HG), using surgical clamps to avoid loss of content. Stomach (ST), anterior midgut (AMG), midgut (MG), and HG compartments were separated by surgical clamps and, then, carefully opened in order to collect and weight the inner content of each segment (see Supplementary Figure 1). Next, tissue samples of the stomach [two samples: anterior stomach (ASt) and posterior stomach (PSt)], pyloric ceca (PC), anterior midgut (AMG), MG and HG [two samples: anterior hindgut (AHG) and posterior hindgut (PHG)] were collected, transferred into RNAlater solution (Invitrogen, Carlsbad, CA, United States), kept at 4°C overnight, and then stored in –80°C until further analysis.
Total RNA Extraction and cDNA Synthesis
Total RNA was isolated using TRI reagent (Sigma-Aldrich, St. Louis, MO, United States) according to the manufacturer’s instructions. To avoid any remnants of genomic DNA, 10 μg of total RNA samples were treated with TURBO DNase-free Kit (Ambion Applied Biosystems, Foster City, CA, United States) following the manufacturer’s instructions. A NanoDrop ND-1000 spectrophotometer (Thermo Fisher Scientific, Waltham, MA, United States) and an Agilent 2100 Bioanalyzer (Agilent Technologies, Sta Clara, CA, United States) were used to evaluate the quantity (ng/μl) and purity and quality (RNA integrity number, RIN) of the total RNA extracted, respectively. Only RNAs with RIN values >6 were used for further analysis. First-strand complementary DNA (cDNA) was synthetized from 2 μg of DNase-treated total RNA using SuperScript III First-Strand Synthesis kit (Invitrogen, Carlsbad, CA, United States) according to the manufacture’s protocol.
Primer Design and RT-qPCR
Specific primers for all the target genes (slc15a1a, slc15a1b, slc15a2a, slc15a2b, ghrl-1, and ghrl-2) were designed spanning an exon–exon junction when possible (Table 1). All the designed primers were tested for cycle of quantification (Cq), primers efficiency (E), and melting peaks. Next, reverse transcription quantitative PCR (RT-qPCR) products were resolved in a 2% agarose gel, purified using E.Z.N.A. Gel Extraction Kit (Omega Bio-Tek, Norcross, GA, United States) and cloned into a pCR4-TOPO vector (Thermo Fisher Scientific, Waltham, MA, United States). Sequencing was performed at the University of Bergen Sequencing Facility (Bergen, Norway) and sequences identity confirmed using blastn analysis against the Atlantic salmon genome GenBank database.
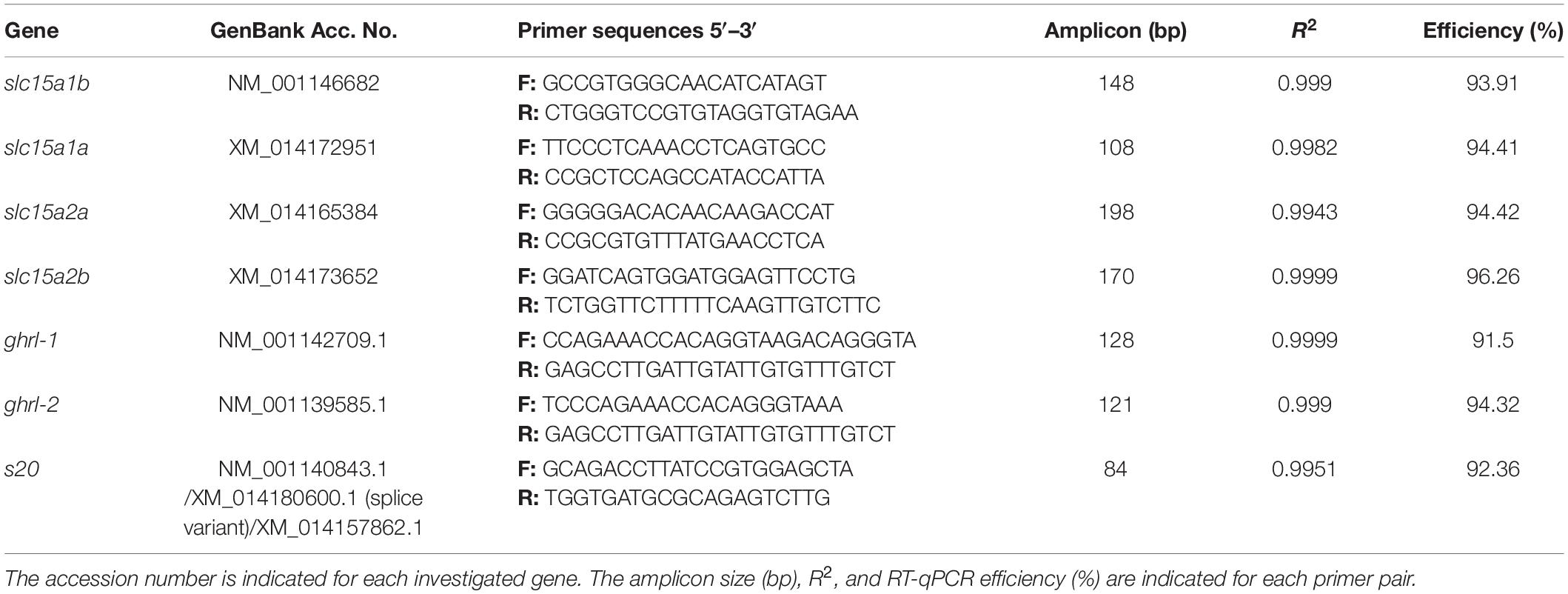
Table 1. Primer sequences for reverse transcription quantitative PCR (RT-qPCR) expression analysis in Atlantic salmon.
Standard curves for each target and reference gene ribosomal protein s20 (s20) were generated from the gene cloned in pCR4-TOPO vector using a 10-fold serial dilution. All the RT-qPCR reactions were performed in duplicate using iTaq Universal SYBR Green Supermix (Bio-Rad, Hercules, CA, United States) in a 20-μl final reaction volume. The following RT-qPCR protocol was performed: (1) 95°C for 30 s, (2) 95°C for 5 s, (3) 60°C for 25 s (steps 2–4 repeated for 40 cycles) in a CFX96 Real-Time System (Bio-Rad Laboratories, Hercules, CA, United States) in connection to CFX Manager Software version 3.1 (Bio-Rad, Laboratories, Hercules, CA, United States). Melting curve analysis over a range of 65–95°C (increment of 0.5°C for 2 s) allowed for the detection of possible nonspecific products and/or primer dimers. Finally, absolute quantification of mRNA expression level was calculated for each gene using the respective standard curve and following the equation:
The copy number was divided by the nanogram of total RNA used for each target gene. The copy number/ng of total RNA of the reference gene s20 was used to normalize the RT-qPCR data.
Statistical Analysis
All the morphometric (L, W, K, and wet content/fish weight %) and mRNA expression (ghrl-1, ghrl-2, slc15a1a, slc15a1b, slc15a2a, and slc15a2b) data were tested for normality and equal variance using D’Agostino-Pearson test and F-test ratio. Grubb’s outlier test was run prior to statistical evaluations. To achieve normal distribution, gene expression data were log-transformed, and the analysis of differential expression between the fed and fasted groups was performed with two-tailed t-test. When either the F-test or the normality test failed, the nonparametric Mann–Whitney test was performed. For ghrelin splice variants, to assess eventual effects of stomach segments and/or presence/absence of food, a two-way ANOVA test, followed by a Sidak’s multiple comparisons test was performed. All the statistical analyses and the graphs were produced using GraphPad Prism 9.1.0 (GraphPad Software, La Jolla, CA, United States). For correlation analysis data, exploration was first performed to identify possible outliers and collinearity, and subsequent analysis was restricted to tissues with relevant mRNA expression levels. Correlation analysis between mRNA expression of target genes and the content in GIT sections was analyzed using generalized linear models (GLMs). Because gene expression was best described by a log-normal distribution, log-transformed gene expression was modeled as a function of tissue-specific content. The model selection procedure was elaborated as follows: (1) all the GIT section contents were tested for each gene, and the region that explained gene expression best was selected (i.e., models with content in ST, AMG, MG, and HG as covariate were compared and selected based on Akaike information criterion); (2) the best model was used to analyze the relationship between content of GIT section and gene expression. To account for different locations of expression, an interaction term between the location of expression and GIT section content was added. This allowed for different relationships between gut content and expression in different tissues, i.e., it represents a common model for each gene with shared intercept for all tissues but different slopes. A graphic depiction of the fitted model was created. Analyses were performed in R 4.0.2 (R Core Team, 2020) using Tidyverse packages (Wickham et al., 2019).
For all tests, a p < 0.05 was considered significant (∗p < 0.05; ∗∗p < 0.01; ∗∗∗p < 0.001). All data are presented as mean ± SEM, unless otherwise stated.
Results
Morphometric and Gastrointestinal Fullness Analysis
The morphometric analysis after 4 days of fasting revealed no statistical differences in W (g) and L (cm) between the two groups. Conversely, the K was significantly lower (p < 0.05) in the fasted group (1.002 ± 0.015 g/cm3) compared to the fed group (1.083 ± 0.029 g/cm3). The wet content/fish weight % in each GIT segment was significantly lower in the fasted group in ST (empty stomach vs. 0.274 ± 0.007; p < 0.0001), AMG (0.104 ± 0.01 vs. 0.498 ± 0.04; p < 0.0001), MG (0.042 ± 0.01 vs. 0.265 ± 0.03; p < 0.001), and HG (0.054 ± 0.01 vs. 0.316 ± 0.03; p < 0.0001).
Effects of 4 Days of Fasting on mRNA Expression Levels
Di- and Tripeptide Transporters (slc15a1a, slc15a1b, slc15a2a, and slc15a2b)
The analysis of mRNA expression levels of the di- and tripeptide transporters along the GIT of Atlantic salmon revealed a similar trend for both slc15a1a and slc15a1b (Figures 1A,B). In both cases, the highest expression levels were observed in PC, AMG, and MG, with slc15a1b (Figure 1B) always showing much higher mRNA expression levels than slc15a1a (Figure 1A). Fasting induced a significantly (p < 0.05) downregulation in the PC for slc15a1a and in PC (p < 0.01) and MG (p < 0.05) for slc15a1b. Peptide transporters 2 types (slc15a2a and slc15a2b) showed different trends of expression along the GIT (Figures 1C,D). While slc15a2a (Figure 1C) showed a similar low expression level, the paralog slc15a2b (Figure 1D) revealed a progressive increase in expression along the GIT segments. The paralog slc15a2b showed much higher expression levels compared to the paralogous gene slc15a2a. Fasting induced a significantly upregulation in the AMG for slc15a2b (p < 0.05). Furthermore, as for slc15a1, 4 days of fasting induced a tendency of downregulation in slc15a2b, but in this case, no statistical differences were found.
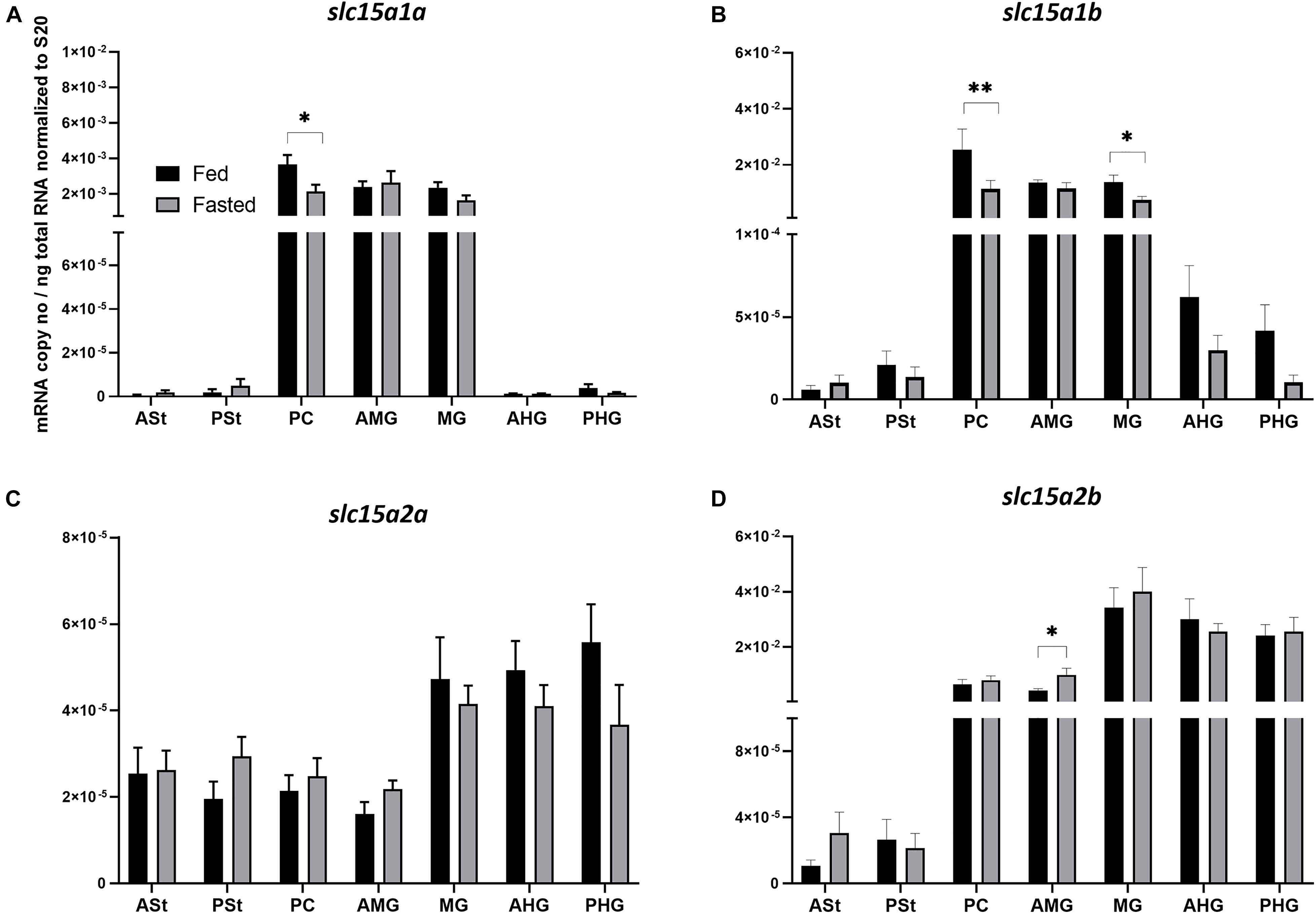
Figure 1. Comparison of mRNA expression levels of (A) slc15a1a, (B) slc15a1b, (C) slc15a2a, and (D) slc15a2b between fed (control) and 4-day fasted groups along the gastrointestinal tract. (A) Data are presented as mean ± SE [n = 12 per group, except for PSt fed and fasted, PC fed, AMG fed, MG fed, and AHG fed (n = 11); ASt fasted (n = 9) and ASt fed (n = 8)] of the normalized expression using the reference gene s20. (B) Data are presented as mean ± SE [n = 12 per group, except for ASt fed, PSt fed, PC fasted, MG fed and fasted and PHG fasted (n = 11), and AMG fed (n = 10)] of the normalized expression using the reference gene s20. (C) Data are presented as mean ± SE [n = 12 per group, except for PSt fed and fasted, PC fed and fasted, AMG fasted and PHG fasted (n = 11), and AMG fed (n = 10)] of the normalized expression using the reference gene s20. (D) Data are presented as mean ± SE [n = 12 per group, except for PSt fed, AMG fed, MG fasted, AHG fasted and PHG fed and fasted (n = 11), and PC fasted (n = 10)] of the normalized expression using the reference gene s20. Statistical analysis: unpaired t-test (*p < 0.05; **p < 0.01). ASt, anterior stomach; PSt, posterior stomach; PC, pyloric ceca; AMG, anterior midgut; MG, midgut; AHG, anterior hindgut; PHG, posterior hindgut.
Ghrelin (ghrl-1 and ghrl-2)
The analysis of ghrl mRNA expression levels along the GIT segments of Atlantic salmon showed a high and similar expression trend for both splice variants (Figures 2A,B), with high expression in the two stomach segments (ASt and PSt) and residual or absent expression along the other GIT segments. ghrl-2 (Figure 2B) was much more abundant than ghrl-1 (Figure 2A). The comparison between fed and fasted groups revealed a tendency of downregulation for fasted group in the stomach sections, and a statistical difference was only found in the PHG for ghrl-2 (p < 0.01). To evaluate different mRNA expression levels in the two stomach segments between the two groups (Figures 2C,D), a two-way ANOVA test (referring to presence/absence of food and stomach segments as variables) was used, and the results showed a significant effect of stomach segment in the fasted group for both splice variants.
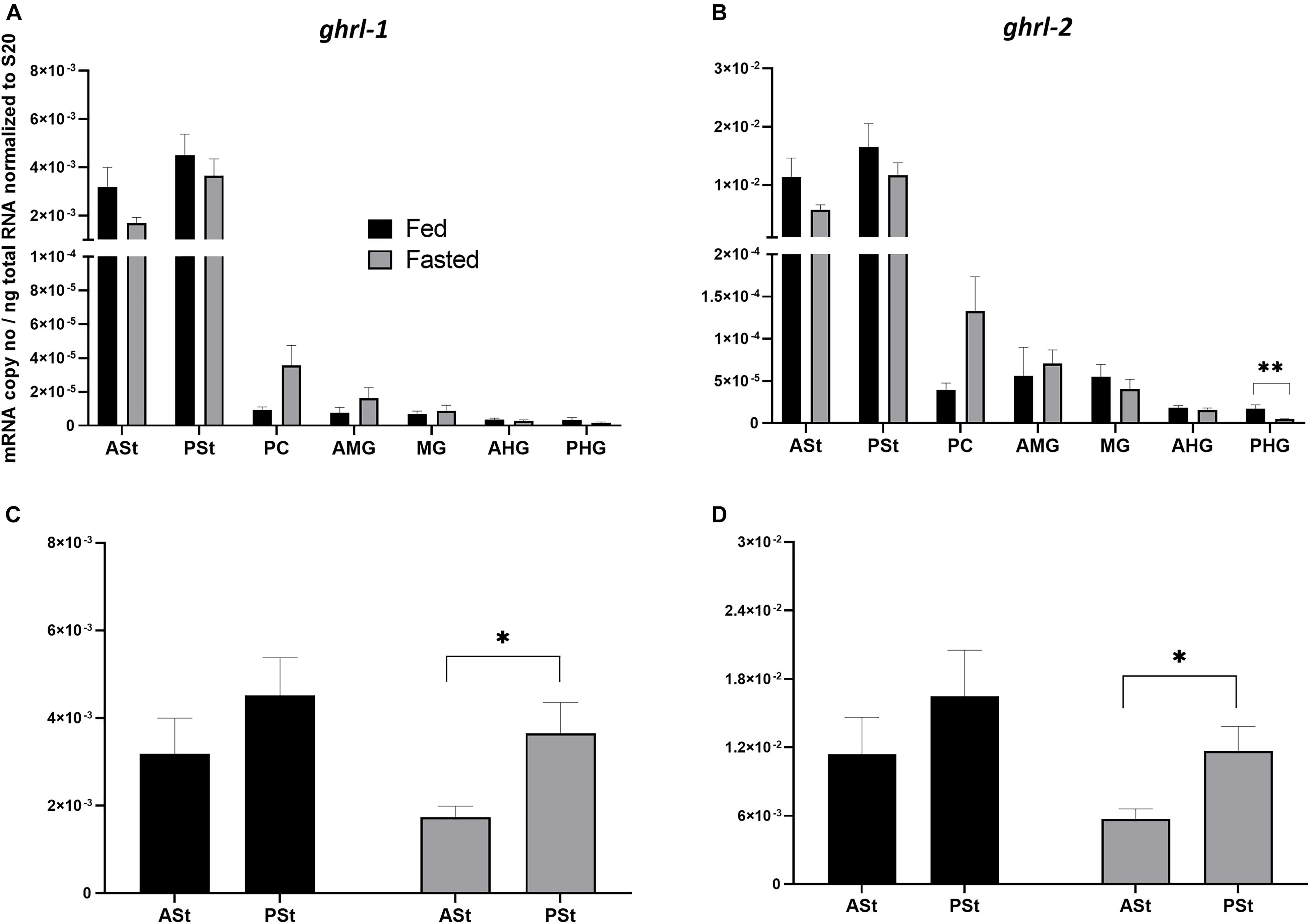
Figure 2. (A,B) Comparison of mRNA expression levels of (A) ghrl-1 and (B) ghrl-2 between fed (control) and 4-day fasted groups along the GIT. (A) Data are presented as mean ± SE [n = 12 per group, except for ASt fasted, PSt fed, PC fed, AMG fed, MG fed, AHG fed, and PHG fasted (n = 11), and PC fasted (n = 10)] of the normalized expression using the reference gene s20. (B) Data are presented as mean ± SE [n = 12 per group, except for ASt fasted, PSt fed, PC fed and fasted, AMG fed and PHG fasted (n = 11), and AMG fed (n = 10)] of the normalized expression using the reference gene s20. Statistical analysis: unpaired t-test. (C,D) Comparison of mRNA expression levels between the two stomach segments (ASt and PSt) and the two groups (control and 4-days fasted) for (C) ghrl-1 and (D) ghrl-2. Two-way ANOVA showed a significant effect of stomach segments [F(1, 41) = 9.753; p < 0.01] but not presence/absence of food [F(1, 41) = 0.3178; p > 0.05] for ghrl-1; a significant effect of stomach segments [F(1, 42) = 9.295; p < 0.01] but not the presence/absence of food [F(1, 42) = 0.2875; p > 0.05] for ghrl-2. A Sidak’s multiple comparisons test was used to assess for specific pair-wise differences. ASt, anterior stomach; PSt, posterior stomach; PC, pyloric ceca; AMG, anterior midgut; MG, midgut; AHG, anterior hindgut; PHG, posterior hindgut.
Correlation Analysis Between mRNA Expression and GIT Sections Content
When exploring how the presence/absence of food (nutrients) in the different sections of the GIT might affect the expression of our target genes (restricted to the tissue with relevant expression levels), ghrl-1 and ghrl-2 (two independent variables) were found highly correlated (Pearson’s correlation > 0.95), and the analysis was therefore performed only for one splicing variant (Supplementary Figure 2). ghrl mRNA expression in the stomach is mainly explained by the HG content and resulted in a statistically significant correlation (p < 0.01) between expression in the PSt and the HG content (Figure 3A and Supplementary Table 1).
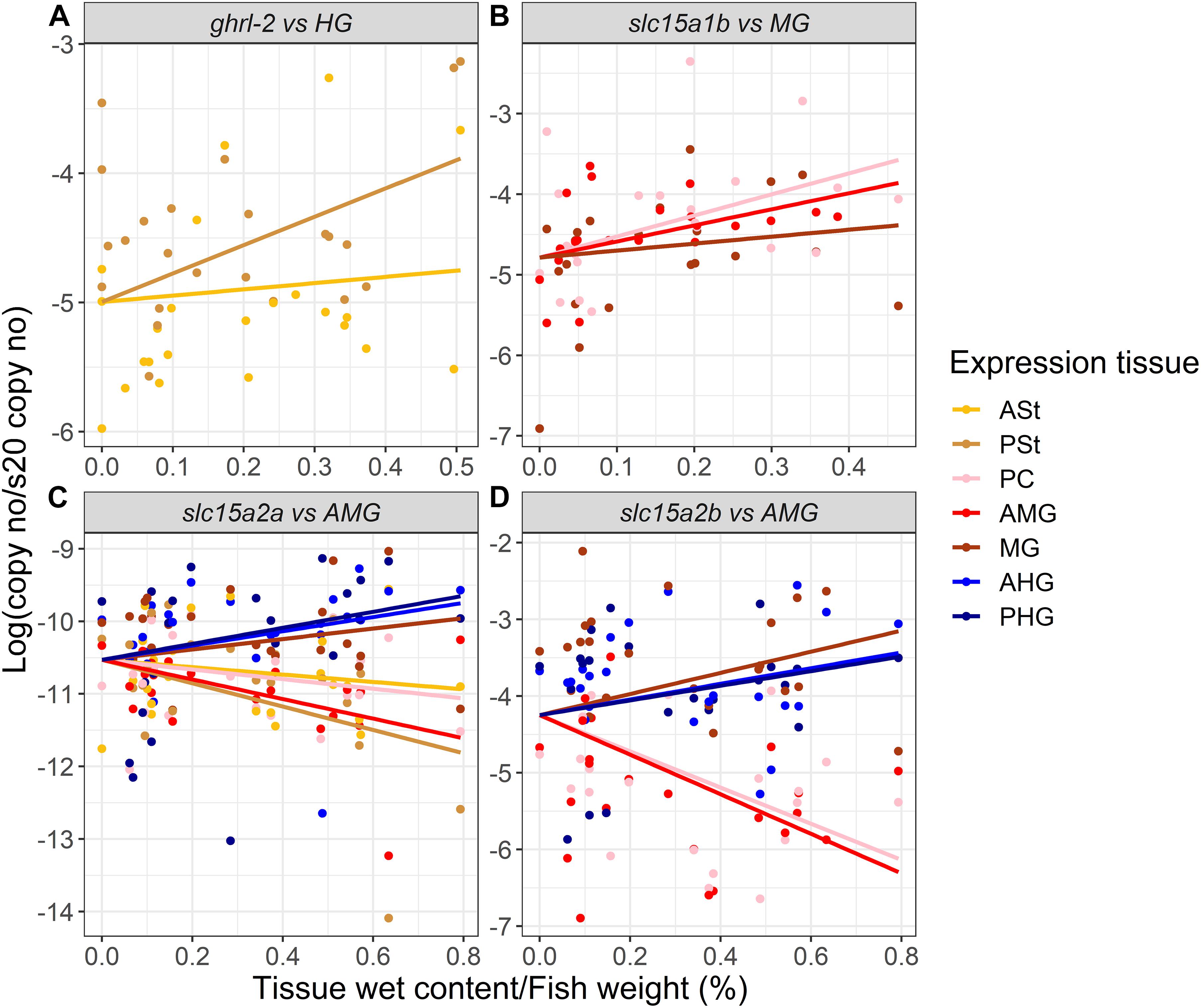
Figure 3. Estimated correlation (solid line) between (A) ghrl-2, (B) slc15a1b, (C) slc15a2a, and (D) slc15a2b mRNA expression and the gastrointestinal tract sections digesta/inner content. Raw data are represented by dots (n = 24, except when outliers were removed as described for Figures 1, 2). ASt, anterior stomach; PSt, posterior stomach; PC, pyloric ceca; AMG, anterior midgut; MG, midgut; AHG, anterior hindgut; PHG, posterior hindgut. Refer to Supplementary Table 1 for detailed information.
slc15a1a and slc15a1b mRNA expressions were also found highly correlated (Pearson’s correlation > 0.95), and therefore, the analysis was carried out using the most abundant paralog, slc15a1b (Supplementary Figure 2). The mRNA expression of slc15a1b in the PC, AMG, and MG (GIT regions with highest expression levels, see Figure 1B) were better explained by the MG inner content, with a statistically significant correlation between MG inner content and PC (p < 0.01) and AMG (p < 0.5) slc15a1b mRNA expression (Figure 3B and Supplementary Table 1). The GIT mRNA expression levels of Atlantic salmon slc15a2a and slc15a2b were better explained by the AMG content. slc15a2a mRNA levels in the PSt (p < 0.001), AMG (p < 0.01), MG (p < 0.05), and AHG (p < 0.05) were significantly correlated with the AMG content (Figure 3C). slc15a2b mRNA expression was found in the AMG and PC significantly highly correlated (p < 0.001) with the AMG inner content, and expression in the MG was also significantly (p < 0.05) correlated with the MG content (Figure 3D and Supplementary Table 1). Interestingly, mRNA expression of slc15a2b was negatively correlated (Pearson’s correlation > 0.85) with the ghrl mRNA expression (Supplementary Figure 2).
Discussion
Sensing of nutrient luminal composition and quantity provides the vertebrate GIT the ability to modulate the response to feeding or a meal depending on chemical, physical, mechanical, and external signals (Rønnestad et al., 2014; Daniel and Zietek, 2015; Zietek and Daniel, 2015). Therefore, the aim of our study was to investigate the GIT response to a 4-days induced fasting in Atlantic salmon. The study was based on the mRNA expression levels analysis of several gastrointestinal genes modulated by nutrient status in the GIT, i.e., slc15a1a, slc15a1b, slc15a2a, and slc15a2b (as marker of intestinal uptake of di- and tripeptides and peptide chemosensor), and ghrl-1 and ghrl-2 (as marker of enteroendocrine hormone production and gut–brain signaling axis). Furthermore, the analysis also explored if fish size and condition affected evacuation, assessed as gastrointestinal fullness (digesta content/fish weight %) after 4 days of fasting. Additionally, the mRNA expression levels of our target genes were correlated with the content of each GIT compartment.
There were no differences in length and weight between control and fasted salmon, whereas K was significantly lower in the fasted fish, indicating a slightly leaner fish with less fat, which could be expected after 4 days without feed. However, the analysis of the gastrointestinal fullness shows residual digesta in all the GIT compartments of fasted salmon (except in stomach), demonstrating that 4 days of fasting is not enough for a complete evacuation, although at this time, the majority of the digestible nutrients in the feeds could be expected to be absorbed, and the remaining content likely would be nondigestible feed ingredients, microbiota, ions, and water. This is supported by a previous study on Atlantic salmon that showed that 48 h were enough to completely empty stomach and the anterior part of the intestine, and most of the dry matter was only present in the HG at that time point (Aas et al., 2017). Thus, the GIT yet had not been totally cleared for indigestible remnants 4 days after the last meal.
The transcriptional analysis involved, first, the peptide transporters. Pept1 is an important intestinal transporter of di- and tripeptides characterized by an expression largely varying during ontogeny, in response to nutritional status (for, e.g., food limitation/deprivation and fasting/refeeding), dietary amino acids, environmental conditions, and under certain disease state (such as gut inflammation) (Amberg et al., 2008; Bakke et al., 2010; Verri et al., 2010, 2017; Bucking and Schulte, 2012; Romano et al., 2014). The rostro-caudal gene expression analysis along the GIT (high expression in PC, AMG, and MG) and the expression ratio levels of both paralogous genes (slc15a1b mRNA expression levels largely exceeding those of slc15a1a) are in line with previous studies on Atlantic salmon (Rønnestad et al., 2010; Gomes et al., 2020) and other salmonids such as rainbow trout (Ostaszewska et al., 2010; Kamalam et al., 2013). In our study, 4 days of fasting induced a moderate tendency of downregulation of both slc15a1 paralogous genes along the GIT, with statistical differences in the PC for slc15a1a and in PC and MG for slc15a1b. In more detail, 4 days of fasting induced a 24% reduction in mRNA expression levels along the GIT for slc15a1a, with a peak of a 42% reduction in PC. Conversely, for slc15a1b, fasting induced a stronger effect, with a reduction of 42% along the GIT (peaks of ca. 55 and 45% of mRNA expression levels in PC and MG, respectively). Our analysis is in agreement with previous studies showing that fasting induced downregulation of mRNA expression in teleost species, which is the opposite of what happens in mammals (Thamotharan et al., 1998; Ogihara et al., 1999), and this most likely represents a different strategy of adaptation to food deprivation/limitation between ectotherms and endotherms. Six days of fasting induced as much as ca. 70% reduction in intestinal slc15a1b mRNA levels of Atlantic salmon (Rønnestad et al., 2010). Furthermore, in Nile tilapia, 1 week of food deprivation induced ca. 50% reduction of intestinal slc15a1a mRNA levels, although only 15% reduction was recorded for slc15a1b mRNA (Huang et al., 2015); in European seabass, 5 weeks of food deprivation induced ca. 70% reduction of intestinal slc15a1b mRNA levels, with a beginning of the downregulation already detected after 4 days of fasting (Terova et al., 2009); and in zebrafish, a progressive downregulation of slc15a1b mRNA levels was recorded during 5-days induced fasting (Koven and Schulte, 2012). In our analysis, the effects of fasting on the gene expression variation were not evaluated in the complete GIT but, rather, in specific gut compartments and thus identifying PC and MG as the most responsive compartments to fasting. Furthermore, for the first time in Atlantic salmon, slc15a1a is shown to respond to fasting with lowering mRNA expression levels, confirming the high flexibility of both transporters in the context of gut physiology and their ability to respond to different luminal conditions and signals, as previously shown in Mozambique tilapia (Con et al., 2019), in Nile tilapia (Hallali et al., 2018), and in European sea bass (Kokou et al., 2019). Based on correlation analysis, moreover, the slc15a1 expression in PC and AMG appeared to be associated with the residual digesta content of the MG, suggesting high sensitivity of the peptide transporter to external stimuli.
Pept2 is another di- and tripeptide transporter member of the SLC15 family, and together with Pept1 in Atlantic salmon, the paralogs originate in the salmonid-specific WGD events (Davidson et al., 2010; Berthelot et al., 2014; Macqueen and Johnston, 2014). The Pept2-like proteins seems to be encoded by different paralogous genes. Our study, which is the first report of slc15a2 in Atlantic salmon, shows higher expression of slc15a2b (comparable mRNA levels to slc15a1b), with low or absent localization in stomach segments, intermediate expression in PC and AMG, and higher expression levels in the distal intestinal segments. In contrast, slc15a2a, compared to b-type paralog (but also compared to both slc15a1 types), shows a very low and constant mRNA expression levels along the GIT, suggesting a minor role in intestinal uptake of di- and tripeptides. Our data are in line with previous detections of slc15a2 in the intestine (Romano et al., 2006; Ostaszewska et al., 2018) and, in particular, in the distal intestinal segments, as reported in Nile tilapia (Huang et al., 2015), in juvenile turbot (Scophthalamus maximus) (Xu et al., 2016), and in Mozambique tilapia (Con et al., 2019), suggesting a downstream concerted integration of Pept2 with Pept1 in the functionality of gut physiology, in order to completely absorb di- and tripeptides from the intestinal lumen (Smith et al., 2013b; Bisesi et al., 2015). However, in contrast to zebrafish (Tian et al., 2015), 4 days of induced fasting did not seem to affect slc15a2 mRNA levels (for both types) in Atlantic salmon, except for a higher expression of the fasted group in AMG for slc15a2b, and this difference might be due to different teleost species studied (Cypriniformes vs. Salmoniformes family representatives). Furthermore, the utilization of more time points, as done in Tian’s work, should be used to better understand the influence of fasting on the slc15a2 activity. However, the presence of residual digesta content in the GIT sections might have played a role in the absence of an effect exerted by fasting, as suggested by the evidence that both slc15a2 mRNA expression detected in the segments with the highest levels show a significant correlation with residual digesta in AMG.
Next, ghrl mRNA expression was analyzed, a peptide hormone mainly secreted by the stomach. In Atlantic salmon, the two ghrl transcripts (ghrl-1 and ghrl-2) show, as expected, the same tissue distribution along the GIT, with high expression in the two stomach segments (ASt and PSt), and low or absent localization in the remaining distal tracts. Furthermore, higher mRNA expression levels of the ghrl-2 splice variant were found, although this does not agree with comparable mRNA levels recorded by Murashita et al. (2009). Interestingly, both splice variants showed an mRNA expression affected by localization in the stomach (ASt and PSt) in the fasted group, indicating the posterior part as a major site of production. Fasting did not seem to affect ghrl mRNA expression in the stomach segments, and only a moderate, and not significant, downregulation trend was observed. This result does not support an orexigenic function by Ghrl as seen in mammals (Patton and Mistlberger, 2013) and in many teleost fish species, such as in 7-days fasted goldfish (Unniappan et al., 2002), Nile tilapia (Schwandt et al., 2010), and gibel carp (Carassius auratus gibelio) (Zhou et al., 2016). However, in Salmoniformes, contradictory results about Ghrl role are widespread. In rainbow trout, central Ghrl injections and long-term peripheral treatments determined an anorexigenic response (Jönsson et al., 2010), whereas Velasco et al. (2016a, b) observed an orexigenic effect. In Atlantic salmon, plasma Ghrl levels were higher in fasted fish compared to fed fish after 2 days, but after 14 days, there were no differences between treatments (Hevrøy et al., 2011), whereas 6 days of fasting induced increased ghrl-1 mRNA expression levels (Murashita et al., 2009). In our analysis, no correlation was found between stomach content and ghrl mRNA expression levels (stomach is the compartment where ghrl is mostly expressed) but, rather, between mRNA expression in the PSt and inner content in HG. A previous study indicated a correlation between a complete empty stomach with an increase in only one orexigenic factor (i.e., agrp1 mRNA expression levels) in the hypothalamus of 3 days of induced fasting in Atlantic salmon (Kalananthan et al., 2020b), supporting the hypothesis that 4 days of fasting are not sufficient to initiate a significant response. Thus, it is still uncertain how the multiple gastric and intestinal signaling factors responds to fasting with different durations. For this reason, a further analysis using more time points should be performed. Moreover, different parameters, such as condition factor, duration of fasting, and stress, will also affect the physiological response of the fish, and for these reasons, further research is required, focusing also on the link between sensory systems like peptide transporters with hormones to better understand their functional role.
In conclusion, our analysis provides a description of multiple genes related to digestive function and with links to appetite control to elucidate their role in the complex mechanism of intestinal luminal sensing in Atlantic salmon. The main changes were related to the expression of slc15a1 paralogous genes with tract specificity, whereas the ghrelin variants were not affected by changes; this suggests a separation of responses between sensing/transport pathways and hormonal pathways. Overall, the marginal effects on mRNA expression levels of the investigated genes suggest that 4 days of fasting might be a too short period to initiate large gastrointestinal transcriptomic responses in Atlantic salmon.
Data Availability Statement
The raw data supporting the conclusions of this article will be made available by the authors, without undue reservation.
Ethics Statement
Ethical review and approval was not required for the animal study because the experiment and sampling were conducted in accordance with Norwegian Animal Research Authority regulations and were approved by local representative of Animal Welfare at the Department of Biological Sciences, University of Bergen (Norway).
Author Contributions
IR, SH, FL, AG, and TK conceived the study. TK executed the experiment. TK, IR, SH, and FL performed the sampling. GDV and FL performed preparatory lab work and RT-qPCR analysis. AG, FL, and GDV performed statistical analysis and related graphs. GDV, AB, and TV prepared the first draft of the manuscript. All authors contributed to the interpretation of the data, writing of the manuscript, and reading and approving the submitted version.
Funding
This study is supported by Regional Research Fund West (Grant 259183) and Research Council of Norway projects LeuSense (Grant 267626), GUTASTE (Grant 262096), G2B2020 (Grant 311627), and Mobility grants from Meltzer Foundation (to IR). GDV and TV received support from Italian Ministry of University and Research (“Dottorato innovativo con caratterizzazione industriale,” Borsa PON 2014-2020 Ricerca e Innovazione, cod. DOT1412034).
Conflict of Interest
The authors declare that the research was conducted in the absence of any commercial or financial relationships that could be construed as a potential conflict of interest.
Acknowledgments
The authors thank Pablo Balseiro Vigo, Enrique Pino Martinez, Muhammad Rahmad Royan, Silei Xia, and Ann-Elise Olderbakk Jordal for assistance with sampling. A special thanks to Pablo Balseiro Vigo for assistance during the transport of the fish to the ILAB facility, Dr. Virginie Gelebart for assistance during analysis, and to Engesund fiskeoppdrett for supplying the fish.
Supplementary Material
The Supplementary Material for this article can be found online at: https://www.frontiersin.org/articles/10.3389/fphys.2021.666670/full#supplementary-material
References
Aas, T. S., Sixten, H. J., Hillestad, M., Sveier, H., Ytrestøyl, T., Hatlen, B., et al. (2017). Mesaurement of gastrointestinal passage rate in Atlantic salmon (Salmo salar) fed dry or soaked feed. Aquac. Rep. 8, 49–57. doi: 10.1016/j.aqrep.2017.10.001
Amberg, J. J., Myr, C., Kamisaka, Y., Jordal, A. E., Rust, M. B., Hardy, R. W., et al. (2008). Expression of the oligopeptide transporter PepT1, in larval Atlantic cod (Gadus morhua). Comp. Biochem. Physiol. B Biochem. Mol. Biol. 150, 177–182. doi: 10.1016/j.cbpb.2008.02.011
Bakke, S., Jordal, A. E., Gómez-Requeni, P., Verri, T., Kousoulaki, K., Aksnes, A., et al. (2010). Dietary protein hydrolysates and free amino acids affect the spatial expression of peptide transporter PepT1 in the digestive tract of Atlantic cod (Gadus morhua). Comp. Biochem. Physiol. B Biochem. Mol. Biol. 156, 48–55. doi: 10.1016/j.cbpb.2010.02.002
Berthelot, C., Brunet, F., Chalopin, D., Juanchich, A., Bernard, M., Noël, B., et al. (2014). The rainbow trout genome provides novel insights into evolution after whole-genome duplication in vertebrates. Nat. Commun. 5:3657. doi: 10.1038/ncomms4657
Bisesi, J. H., Ngo, T., Ponnavolu, S., Liu, K., Lavelle, C. M., Afrooz, A. R. M. N., et al. (2015). Examination of single-walled carbon nanotubes uptake and toxicity from dietary exposure: tracking movement and impacts in the gastrointestinal system. Nanomaterials. 5, 1066–1086. doi: 10.3390/nano5021066
Blouet, C., and Schwartz, G. J. (2010). Hypothalamic nutrient sensing in the control of energy homeostasis. Behav. Brain Res. 209, 1–12. doi: 10.1016/j.bbr.2009.12.024
Bucking, C., and Schulte, P. M. (2012). Environmental and nutritional regulation of expression and function of two peptide transporter (PepT1) isoforms in a euryhaline teleost. Comp. Biochem. Physiol. A Mol. Integr. Physiol. 161, 379–387. doi: 10.1016/j.cbpa.2011.12.008
Cerdá-Reverter, J. M., and Canosa, L. F. (2009). Neuroendocrine systems of the fish brain. Fish. Neuroendocrinol. 28, 3–74.
Chourasia, T. K., D’Cotta, H., Baroiller, J. F., Slosman, T., and Cnaani, A. (2018). Effects of the acclimation to high salinity on intestinal ion and peptide transporters in two tilapia species that differ in their salinity tolerance. Comp. Biochem. Physiol. A Mol. Integr. Physiol. 218, 16–23. doi: 10.1016/j.cbpa.2018.01.004
Con, P., Nitzan, T., and Cnaani, A. (2017). Salinity dependent shift in the localization of three peptide transporters along the intestine of the Mozambique tilapia (Oreochromis mossambicus). Front. Physiol. 8:8. doi: 10.3389/fphys.2017.00008
Con, P., Nitzan, T., Slosman, T., Harpaz, S., and Cnaani, A. (2019). Peptide transporters in the primary gastrointestinal tract of pre-feeding mozambique tilapia larva. Front. Physiol. 10:808. doi: 10.3389/fphys.2019.00808
Conde-Sieira, M., and Soengas, J. L. (2017). Nutrient sensing systems in fish: impact on food intake regulation and energy homeostasis. Front. Neurosci. 10:603. doi: 10.3389/fnins.2016.00603
Daniel, H. (2004). Molecular and integrative physiology of intestinal peptide transport. Annu. Rev. Physiol. 66, 361–384. doi: 10.1146/annurev.physiol.66.032102.144149
Daniel, H., and Rubio-Aliaga, I. (2003). An update on renal peptide transporters. Am. J. Physiol. Renal. Physiol. 284, F885–F892. doi: 10.1152/ajprenal.00123.2002
Daniel, H., and Zietek, T. (2015). Taste and move: glucose and peptide transporters in the gastrointestinal tract. Exp. Physiol. 100, 1441–1450. doi: 10.1113/EP085029
Davidson, W. S., Koop, B. F., Jones, S. J., Iturra, P., Vidal, R., Maass, A., et al. (2010). Sequencing the genome of the Atlantic salmon (Salmo salar). Genome. Biol. 11:403. doi: 10.1186/gb-2010-11-9-403
Delgado, M. J., Cerdá-Reverter, J. M., and Soengas, J. L. (2017). Hypothalamic integration of metabolic, endocrine, and circadian signals in fish: involvement in the control of food intake. Front. Neurosci. 11:354. doi: 10.3389/fnins.2017.00354
Einen, O., Waagan, B., and Thomassen, M. S. (1998). Starvation prior to slaughter in Atlantic salmon (Salmo salar): i. effects on weight loss, body shape, slaughter- and fillet-yield, proximate and fatty acid composition. Aquaculture. 166, 85–104. doi: 10.1016/s0044-8486(98)00332-9
Froese, B. R. (2006). Cube law, condition factor and weight-length relationships: history, meta-analysis and recommendations. J. Appl. Ichthyol. 22, 241–253. doi: 10.1111/j.1439-0426.2006.00805.x
Gomes, A. S., Vacca, F., Cinquetti, R., Murashita, K., Barca, A., Bossi, E., et al. (2020). Identification and characterization of the Atlantic salmon peptide transporter 1a. Am. J. Physiol. Cell. Physiol. 318, C191–C204. doi: 10.1152/ajpcell.00360.2019
Gonçalves, A. F., Castro, L. F., Pereira-Wilson, C., Coimbra, J., and Wilson, J. M. (2007). Is there a compromise between nutrient uptake and gas exchange in the gut of Misgurnus anguillicaudatus, an intestinal air-breathing fish? Comp. Biochem. Physiol. Part D Genom. Proteom. 2, 345–355. doi: 10.1016/j.cbd.2007.08.002
Hallali, E., Kokou, F., Chourasia, T. K., Nitzan, T., Con, P., Harpaz, S., et al. (2018). Dietary salt levels affect digestibility, intestinal gene expression, and the microbiome, in Nile tilapia (Oreochromis niloticus). PLoS One. 13:e0202351. doi: 10.1371/journal.pone.0202351
Hevrøy, E. M., Azpeleta, C., Shimizu, M., Lanzén, A., Kaiya, H., Espe, M., et al. (2011). Effects of short- term starvation on ghrelin, GH-IGF system, and IGF-binding proteins in Atlantic salmon. Fish Physiol. Biochem. 37, 217–232. doi: 10.1007/s10695-010-9434-3
Higgins, S. C., Gueorguiev, M., and Korbonits, M. R. (2007). Ghrelin, the peripheral hunger hormone. Ann. Med. 39, 116–136. doi: 10.1080/07853890601149179
Huang, Q., Vera Delgado, J. M., Seni Pinoargote, O. D., and Llaguno, R. A. (2015). Molecular evolution of the Slc15 family and its response to waterborne copper and mercury exposure in tilapia. Aquat. Toxicol. 163, 140–147. doi: 10.1016/j.aquatox.2015.04.011
Hvas, M., Stien, L. H., and Oppedal, F. (2020). The metabolic rate response to feed withdrawal in Atlantic salmon post-smolts. Aquaculture 529:735690. doi: 10.1016/j.aquaculture.2020.735690
Jönsson, E., Kaiya, H., and Björnsson, B. T. (2010). Ghrelin decreases food intake in juvenile rainbow trout (Oncorhynchus mykiss) through the central anorexigenic corticotropin-releasing factor system. Gen. Comp. Endocrinol. 166, 39–46. doi: 10.1016/j.ygcen.2009.11.001
Kalananthan, T., Lai, F., Gomes, A. S., Murashita, K., Handeland, S., and Rønnestad, I. (2020a). The melanocortin system in Atlantic salmon (Salmo salar L.) and its role in apetite control. Front. Neuroanat. 14:48. doi: 10.3389/fnana.2020.00048
Kalananthan, T., Murashita, K., Rønnestad, I., Ishigaki, M., Takahahaski, K., Silva, M. S., et al. (2020b). Hypothalamic agrp and pomc mRNA responses to gastrointestinal fullness and fasting in Atlantic Salmon (Salmo salar, L.). Front. Physiol. 11:61. doi: 10.3389/fphys.2020.00061
Kamal, M. A., Keep, R. F., and Smith, D. E. (2008). Role and relevance of PEPT2 in drug disposition, dynamics, and toxicity. Drug Metab. Pharmacokinet. 23, 236–242. doi: 10.2133/dmpk.23.236
Kamalam, B. S., Panserat, S., Aguirre, P., Geurden, I., Fontagné-Dicharry, S., and Médale, F. (2013). Selection for high muscle fat in rainbow trout induces potentially higher chylomicron synthesis and PUFA biosynthesis in the intestine. Comp. Biochem. Physiol. A Mol. Integr. Physiol. 164, 417–427. doi: 10.1016/j.cbpa.2012.11.020
Kang, K. S., Yahashi, S., and Matsuda, K. (2011). The effects of ghrelin on energy balance and psychomotor activity in a goldfish model: an overview. Int. J. Pept. 2011:171034. doi: 10.1155/2011/171034
Kokou, F., Con, P., Barki, A., Nitzan, T., Slosman, T., Mizrahi, I., et al. (2019). Short- and long-term low-salinity acclimation effects on the branchial and intestinal gene expression in the European seabass (Dicentrarchus labrax). Comp. Biochem. Physiol. A Mol. Integr. Physiol. 231, 11–18. doi: 10.1016/j.cbpa.2019.01.018
Kousoulaki, K., Rønnestad, I., Olsen, H. J., Rathore, R., Campbell, P., Nordrum, S., et al. (2013). Krill hydrolysate free amino acids responsible for feed intake stimulation in Atlantic salmon (Salmo salar). Aquacult. Nutr. 19, 47–61. doi: 10.1111/anu.12094
Koven, W., and Schulte, P. (2012). The effect of fasting and refeeding on mRNA expression of PepT1 and gastrointestinal hormones regulating digestion and food intake in zebrafish (Danio rerio). Fish. Physiol. Biochem. 38, 1565–1575. doi: 10.1007/s10695-012-9649-6
Lie, K. K., Tørresen, O. K., Solbakken, M. H., Rønnestad, I., Tooming-Klunderud, A., Nederbragt, A. J., et al. (2018). Loss of stomach, loss of appetite? Sequencing of the ballan wrasse (Labrus bergylta) genome and intestinal transcriptomic profiling illuminate the evolution of loss of stomach function in fish. BMC Genom. 19:186. doi: 10.1186/s12864-018-4570-8
Liu, Z., Zhou, Y., Feng, J., Lu, S., Zhao, Q., and Zhang, J. (2013). Characterization of oligopeptide transporter (PepT1) in grass carp (Ctenopharyngodon idella). Comp. Biochem. Physiol. B Biochem. Mol. Biol. 164, 194–200. doi: 10.1016/j.cbpb.2012.11.008
Macqueen, D. J., and Johnston, I. A. (2014). A well-constrained estimate for the timing of the salmonid whole genome duplication reveals major decoupling from species diversification. Proc. Biol. Sci. 281:20132881. doi: 10.1098/rspb.2013.2881
Morton, G. J., Meek, T. H., and Schwartz, M. W. (2014). Neurobiology of food intake in health and disease. Nat. Rev. Neurosci. 15, 367–378. doi: 10.1038/nrn3745
Murashita, K., Kurokawa, T., Ebbesson, L. O. E., Stefansson, S. O., and Rønnestad, I. (2009). Characterization, tissue distribution, and regulation of agouti-related protein (AgRP), cocaine- and amphetamine-regulated transcript (CART) and neuropeptide Y (NPY) in Atlantic salmon (Salmo salar). Gen. Comp. Endocrinol. 162, 160–171. doi: 10.1016/j.ygcen.2009.03.015
Nisembaum, L. G., de Pedro, N., Delgado, M. J., and Isorna, E. (2014). Cross talking between the “gut-brain” hormone ghrelin and the circadian system in the goldfish. Effects on clock gene expression and food anticipatory activity. Gen. Comp. Endocrinol. 205, 287–295. doi: 10.1016/j.ygcen.2014.03.016
Ogihara, H., Suzuki, T., Nagamachi, Y., Inui, K., and Takata, K. (1999). Peptide transporter in the rat small intestine: ultrastructural localization and the effect of starvation and administration of amino acids. Histochem. J. 31, 169–174. doi: 10.1023/a:1003515413550
Olsson, C., Holbrook, J. D., Bompadre, G., Jönsson, E., Hoyle, C. H., Sanger, G. J., et al. (2008). Identification of genes for the ghrelin and motilin receptors and a novel related gene in fish, and stimulation of intestinal motility in zebrafish (Danio rerio) by ghrelin and motilin. Gen. Comp. Endocrinol. 155, 217–226. doi: 10.1016/j.ygcen.2007.05.016
Orozco, Z. G., Soma, S., Kaneko, T., and Watanabe, S. (2017). Effects of fasting and refeeding on gene expression of slc15a1a, a gene encoding an oligopeptide transporter (PepT1), in the intestine of Mozambique tilapia. Comp. Biochem. Physiol. B Biochem. Mol. Biol. 203, 76–83. doi: 10.1016/j.cbpb.2016.09.006
Ostaszewska, T., Dabrowski, K., Kamaszewski, M., Grochowski, P., Verri, T., Rzepkowska, M., et al. (2018). The effect of plant protein-based diet supplemented with dipeptide or free amino acids on digestive tract morphology and PepT1 and PepT2 expressions in common carp (Cyprinus carpio L.). Comp. Biochem. Physiol. A Mol. Integr. Physiol. 157, 158–169. doi: 10.1016/j.cbpa.2010.06.162
Ostaszewska, T., Kamaszewski, M., Grochowski, P., Dabrowski, K., Verri, T., Aksakal, E., et al. (2010). The effect of peptide absorption on PepT1 gene expression and digestive system hormones in rainbow trout (Oncorhynchus mykiss). Comp. Biochem. Physiol. A Mol. Integr. Physiol. 155, 107–114. doi: 10.1016/j.cbpa.2009.10.017
Pasquier, J., Cabau, C., Nguyen, T., Jouanno, E., Severac, D., Braasch, I., et al. (2016). Gene evolution and gene expression after whole genome duplication in fish: the PhyloFish database. BMC Genom. 17:368. doi: 10.1186/s12864-016-2709-z
Patton, D. F., and Mistlberger, R. E. (2013). Circadian adaptations to meal timing; neuroendocrine mechanisms. Front. Neurosci. 7:185. doi: 10.3389/fnins.2013.00185
Peddu, S. C., Breves, J. P., Kaiya, H., Grau, E. G., and Riley, L. G. Jr. (2009). Pre- and postprandial effects on ghrelin signalling in the brain and on the GH/IGF-I axis in the Mozambique tilapia (Oreochromis mossambicus). Gen. Comp. Endocrinol. 161, 412–418. doi: 10.1016/j.ygcen.2009.02.008
R Core Team. (2020). R: A Language and Environment for Statistical Computing. R Foundation for Statistical Computing. Vienna: R Core Team.
Rizzello, A., Romano, A., Kottra, G., Acierno, R., Storelli, C., Verri, T., et al. (2013). Protein cold adaptation strategy via a unique seven-amino acid domain in the icefish (Chionodraco hamatus) PEPT1 transporter. Proc. Natl. Acad. Sci. U.S.A. 110, 7068–7073. doi: 10.1073/pnas.1220417110
Robb, D. H. F. (2008). “Welfare of fish at harvest,” in Fish Welfare, ed. E. J. Branson (London: Blackwell Publishing), 217–242.
Romano, A., Barca, A., Storelli, C., and Verri, T. (2014). Teleost fish models in membrane transport research: the PEPT1 (SLC15A1) H+-oligopeptide transporter as a case study. J. Physiol. 592, 881–897. doi: 10.1113/jphysiol.2013.259622
Romano, A., Kottra, G., Barca, A., Tiso, N., Maffia, M., Argenton, F., et al. (2006). High-affinity peptide transporter PEPT2 (SLC15A2) of the zebrafish Danio rerio: functional properties, genomic organization, and expression analysis. Physiol. Genomics. 24, 207–217. doi: 10.1152/physiolgenomics.00227.2005
Rønnestad, I., Akiba, Y., Kaji, I., and Kaunitz, J. D. (2014). Duodenal luminal nutrient sensing. Curr. Opin. Pharmacol. 19, 67–75. doi: 10.1016/j.coph.2014.07.010
Rønnestad, I., Gavaia, P. J., Viegas, C. S. B., Verri, T., Romano, A., Nilsen, T. O., et al. (2007). Oligopeptide transporter PepT1 in Atlantic cod (Gadus morhua L.): cloning, tissue expression and comparative aspects. J. Exp. Biol. 210, 3883–3896. doi: 10.1242/jeb.007898
Rønnestad, I., Gomes, A. S., Murashita, K., Angotzi, R., Jönsson, E., and Volkoff, H. (2017). Appetite-controlling endocrine systems in teleosts. Front. Endocrinol. 8:73. doi: 10.3389/fendo.2017.00073
Rønnestad, I., Murashita, K., Kottra, G., Jordal, A. E., Narawane, S., Jolly, C., et al. (2010). Molecular cloning and functional expression of Atlantic salmon peptide transporter 1 in Xenopus oocytes reveals efficient intestinal uptake of lysine-containing and other bioactive di- and tripeptides in teleost fish. J. Nutr. 140, 893–900. doi: 10.3945/jn.109.118240
Rubio-Aliaga, I., and Daniel, H. (2002). Mammalian peptide transporters as targets for drug delivery. Trends Pharmacol. Sci. 23, 434–440. doi: 10.1016/s0165-6147(02)02072-2
Rubio-Aliaga, I., and Daniel, H. (2008). Peptide transporters and their roles in physiological processes and drug disposition. Xenobiotica 38, 1022–1042. doi: 10.1080/00498250701875254
Rühl, A., Hoppe, S., Frey, I., Daniel, H., and Schemann, M. (2005). Functional expression of the peptide transporter PEPT2 in the mammalian enteric nervous system. J. Comp. Neurol. 490, 1–11. doi: 10.1002/cne.20617
Sangaletti, R., Terova, G., Peres, A., Bossi, E., Corà, S., and Saroglia, M. (2009). Functional expression of the oligopeptide transporter PepT1 from the sea bass (Dicentrarchus labrax). Pflügers Arch. 459, 47–54. doi: 10.1007/s00424-009-0700-0
Schwandt, S. E., Peddu, S. C., and Riley, L. G. (2010). Differential roles for octanoylated and decanoylated ghrelins in regulating appetite and metabolism. Int. J. Pept. 2010:275804. doi: 10.1155/2010/275804
Schwartz, M. W., Woods, S. C., Porte, D. Jr., Seeley, R., and Baskin, D. G. (2000). Central nervous system control of food intake. Nature 404, 661–671. doi: 10.1038/35007534
Smith, D. E., Clémençon, B., and Hediger, M. A. (2013a). Proton-coupled oligopeptide transporter family SLC15: physiological, pharmacological and pathological implications. Mol. Aspects Med. 34, 323–336. doi: 10.1016/j.mam.2012.11.003
Smith, D. L. Jr., Barry, R. J., Powell, M. L., Nagy, T. R., D’Abramo, L. R., and Watts, S. A. (2013b). Dietary protein source influence on body size and composition in growing zebrafish. Zebrafish 10, 439–446. doi: 10.1089/zeb.2012.0864
Terova, G., Corà, S., Verri, T., Rimoldi, S., Bernardini, G., and Saroglia, M. (2009). Impact of feed availability on PEPT1 mRNA expression levels in sea bass (Dicentrarchus labrax). Aquaculture 294, 288–299. doi: 10.1016/j.aquaculture.2009.06.014
Thamotharan, M., Bawani, S., Zhou, X., and Adibi, S. (1998). Mechanism of dipeptide stimulation of its own transport in a human intestinal cell line. Proc. Assoc. Am. Physicians. 110, 361–368.
Tian, J., He, G., Mai, K., and Liu, C. (2015). Effects of postprandial starvation on mRNA expression of endocrine-, amino acid and peptide transporter-, and metabolic enzyme-related genes in zebrafish (Danio rerio). Fish Physiol. Biochem. 41, 773–787. doi: 10.1007/s10695-015-0045-x
Unniappan, S., Lin, X., Cervini, L., Rivier, J., Kaiya, H., Kangawa, K., et al. (2002). Goldfish ghrelin: molecular characterization of the complementary deoxyribonucleic acid, partial gene structure and evidence for its stimulatory role in food intake. Endocrinology 143, 4143–4146. doi: 10.1210/en.2002-220644
Vacca, F., Barca, A., Gomes, A. S., Mazzei, A., Piccinni, B., Cinquetti, R., et al. (2019). The peptide transporter 1a of the zebrafish Danio rerio, an emerging model in nutrigenomics and nutrition research: molecular characterization, functional properties, and expression analysis. Genes Nutr. 14:33. doi: 10.1186/s12263-019-0657-3
Velasco, C., Librán-Pérez, M., Otero-Rodiño, C., López-Patiño, M. A., Míguez, J. M., Cerdá-Reverter, J. M., et al. (2016a). Ghrelin modulates hypothalamic fatty acid-sensing and control of food intake in rainbow trout. J. Endocrinol. 228, 25–37. doi: 10.1530/JOE-15-0391
Velasco, C., Librán-Pérez, M., Otero-Rodiño, C., López-Patiño, M. A., Míguez, J. M., and Soengas, J. L. (2016b). Ceramides are involved in regulation of food intake in rainbow trout (Oncorhynchus mykiss). Am. J. Physiol. Regul. Integr. Comp. Physiol. 311, R658–R668. doi: 10.1152/ajpregu.00201.2016
Verri, T., Barca, A., Pisani, P., Piccinni, B., Storelli, C., and Romano, A. (2017). Di- and tripeptide transport in vertebrates: the contribution of teleost fish models. J. Comp. Physiol. B 187, 395–462. doi: 10.1007/s00360-016-1044-7
Verri, T., Kottra, G., Romano, A., Tiso, N., Peric, M., Maffia, M., et al. (2003). Molecular and functional characterisation of the zebrafish (Danio rerio) PEPT1-type peptide transporter. FEBS Lett. 549, 115–122. doi: 10.1016/s0014-5793(03)00759-2
Verri, T., Romano, A., Barca, A., Kottra, G., Daniel, H., and Storelli, C. (2010). Transport of di- and tripeptides in teleost fish intestine. Aquacult. Res. 41, 641–653. doi: 10.1111/j.1365-2109.2009.02270.x
Verri, T., Terova, G., Dabrowski, K., and Saroglia, M. (2011). Peptide transport and animal growth: the fish paradigm. Biol. Lett. 7, 597–600. doi: 10.1098/rsbl.2010.1164
Vikesa, V., Nankervis, L., Remo, S. C., Waagbo, R., and Hevrøy, E. M. (2015). Pre and post prandial regulation of ghrelin, aminoacids and IGF1 in Atlantic salmon (Salmo salar L.) at optimal and elevated sea water temperatures. Aquaculture 438, 159–169. doi: 10.1016/j.aquaculture.2014.12.021
Volkoff, H. (2016). The neuroendocrine regulation of food intake in fish: a review of current knowledge. Front. Neurosci. 10:540. doi: 10.3389/fnins.2016.00540
Waagbø, R., Jørgensen, S. M., Timmerhaus, G., Breck, O., and Olsvik, P. A. (2017). Short-term starvation at low temperature prior to harvest does not impact the health and acute stress response of adult Atlantic salmon. Peer J. 5:e3273. doi: 10.7717/peerj.3273
Wickham, H., Averick, M., Bryan, J., Chang, W., D’Agostino-McGowan, L., François, R., et al. (2019). Welcome to the Tidyverse. J. Open Source Softw. 4:1686. doi: 10.21105/joss.01686
Xu, D., He, G., Mai, K., Zhou, H., and Song, F. (2016). Expression pattern of peptide and amino acid transporter genes in digestive tract of juvenile turbot (Scophthalmus maximus L.). J. Ocean Univ. China. 15, 334–340. doi: 10.1007/s11802-016-2768-4
Zhou, C., Zheng, J., Lei, L., Yuan, D., Zhu, C., Ye, H., et al. (2016). Evidence that ghrelin may be associated with the food intake of gibel carp (Carassius auratus gibelio). Fish Physiol. Biochem. 42, 1637–1646. doi: 10.1007/s10695-016-0246-y
Keywords: ghrelin, peptide transporters, slc15, fasting, digestive tract, fish
Citation: Del Vecchio G, Lai F, Gomes AS, Verri T, Kalananthan T, Barca A, Handeland S and Rønnestad I (2021) Effects of Short-Term Fasting on mRNA Expression of Ghrelin and the Peptide Transporters PepT1 and 2 in Atlantic Salmon (Salmo salar). Front. Physiol. 12:666670. doi: 10.3389/fphys.2021.666670
Received: 10 February 2021; Accepted: 11 May 2021;
Published: 21 June 2021.
Edited by:
Carlo C. Lazado, Norwegian Institute of Food, Fisheries and Aquaculture Research (Nofima), NorwayReviewed by:
María Jesús Delgado, Complutense University of Madrid, SpainMahmoud M. Alagawany, Zagazig University, Egypt
Copyright © 2021 Del Vecchio, Lai, Gomes, Verri, Kalananthan, Barca, Handeland and Rønnestad. This is an open-access article distributed under the terms of the Creative Commons Attribution License (CC BY). The use, distribution or reproduction in other forums is permitted, provided the original author(s) and the copyright owner(s) are credited and that the original publication in this journal is cited, in accordance with accepted academic practice. No use, distribution or reproduction is permitted which does not comply with these terms.
*Correspondence: Ivar Rønnestad, SXZhci5Sb25uZXN0YWRAdWliLm5v, orcid.org/0000-0001-8789-0197