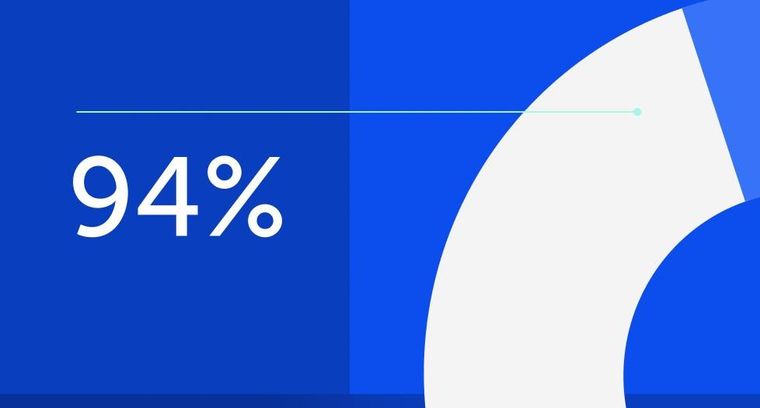
94% of researchers rate our articles as excellent or good
Learn more about the work of our research integrity team to safeguard the quality of each article we publish.
Find out more
ORIGINAL RESEARCH article
Front. Physiol., 19 May 2021
Sec. Invertebrate Physiology
Volume 12 - 2021 | https://doi.org/10.3389/fphys.2021.663040
This article is part of the Research TopicInsect Olfactory Proteins (From Gene Identification to Functional Characterization), Volume IIView all 20 articles
The olfactory system is used by insects to find hosts, mates, and oviposition sites. Insects have different types of olfactory proteins, including odorant-binding proteins (OBPs), chemosensory proteins (CSPs), odorant receptors (ORs), ionotropic receptors (IRs), and sensory neuron membrane proteins (SNMPs) to perceive chemical cues from the environment. The greater wax moth, Galleria mellonella, is an important lepidopteran pest of apiculture. However, the molecular mechanism underlying odorant perception in this species is unclear. In this study, we performed transcriptome sequencing of G. mellonella antennae to identify genes involved in olfaction. A total of 42,544 unigenes were obtained by assembling the transcriptome. Functional classification of these unigenes was determined by searching against the Gene Ontology (GO), eukaryotic orthologous groups (KOG), and the Kyoto Encyclopedia of Genes and Genomes (KEGG) databases. We identified a total of 102 olfactory-related genes: 21 OBPs, 18 CSPs, 43 ORs, 18 IRs, and 2 SNMPs. Results from BLASTX best hit and phylogenetic analyses showed that most of the genes had a close relationship with orthologs from other Lepidoptera species. A large number of OBPs and CSPs were tandemly arrayed in the genomic scaffolds and formed gene clusters. Reverse transcription-quantitative PCR results showed that GmelOBP19 and GmelOR47 are mainly expressed in male antennae. This work provides a transcriptome resource for olfactory genes in G. mellonella, and the findings pave the way for studying the function of these genes.
Olfaction is essential for insect activities such as food seeking, mate recognition, and oviposition. For efficient detection of chemical cues, insects have evolved an olfaction system that consists of many olfactory proteins, including odorant-binding proteins (OBPs), chemosensory proteins (CSPs), odorant receptors (ORs), ionotropic receptors (IRs), and sensory neuron membrane proteins (SNMPs) (Leal, 2013; Robertson, 2019).
OBPs are small, water soluble proteins enriched in the sensillar lymph of insect antennae (Pelosi et al., 2018). OBPs in the pores of the antennal sensillae can bind odorant compounds and deliver them to active ORs (Sun et al., 2018). OBPs typically have six positionally conserved cysteine residues. These cysteine residues form three disulfide bridges, which are necessary for maintaining protein stability (Brito et al., 2016). In Lepidoptera, there are two special subgroups of OBP: general odorant-binding protein (GOBP) and pheromone-binding protein (PBP) (Vogt et al., 2015). GOBPs recognize “general” odorants such as volatiles from host plants, whereas PBPs perceive sex pheromone constituents. However, many studies have demonstrated that GOBPs can bind sex pheromones and PBPs can have strong affinities for plant volatiles (Gong et al., 2009b; Khuhro et al., 2017; Sun et al., 2019a). CSPs are carrier proteins enriched in the sensillar lymph with a function similar to OBPs (Pelosi et al., 2018). CSPs contain four positionally conserved cysteines that form two disulfide bridges (Pelosi et al., 2014). Some CSPs are specifically expressed in the antenna and can bind to plant volatiles and sex pheromone constituents (Zhang et al., 2014; Li et al., 2015; Duan et al., 2019). Other CSPs are highly concentrated in non-olfaction organs, such as pheromone glands and legs, suggesting they may be involved in other physiological processes besides being carriers of odorants (Zhang et al., 2016; Sun et al., 2017).
Insect ORs are located on the dendrite membrane of olfactory sensory neurons (OSNs) (Touhara and Vosshall, 2009). ORs can recognize the odorants transferred by OBPs and CSPs, and convert these chemical signals into electrical signals (Wicher, 2018). Although most insect ORs have a seven-transmembrane domain, they are not G-protein-coupled receptors (GPCRs) because they have a different type of topology (Fleischer et al., 2018). In insects, a functional OR unit comprised of one copy of poorly conserved, conventional OR along with one copy of a highly conserved, non-conventional olfactory co-receptor (Orco) (Touhara and Vosshall, 2009). The OR/Orco complex forms heteromeric ligand gated ion channels that allow insects to rapidly perceive chemical signals (Butterwick et al., 2018). IRs are also key receptors involved in the perception of odorants, such as phenylacetaldehyde, amines and acids (Rytz et al., 2013; Zhang et al., 2019). IRs are transmembrane proteins with an extracellular N-terminus, a bipartite ligand-binding domain (two lobes separated by an ion channel domain), and a short cytoplasmic C-terminus, which have a structural similarity with ionotropic glutamate receptors (iGluRs) (Benton et al., 2009). However, IRs and iGluRs diverge from each other according to their sequence characteristics and phylogenetics (Croset et al., 2010).
Insect SNMPs have homology with the human fatty acid transporter CD36 and are divided into two subfamilies: SNMP1 and SNMP2 (Vogt et al., 2009). SNMP1s are co-expressed with pheromone receptors (PRs) accumulating on the membrane of pheromone-sensitive OSNs, whereas SNMP2s are expressed in the cells surrounding the pheromone-sensitive OSNs (Forstner et al., 2008; Sun et al., 2019b). SNMPs may have the ability to transfer lipophilic sex pheromones to ORs; in fruit fly and several moth species, SNMP1s are crucial for the detection of pheromones (Jin et al., 2008; Zhang et al., 2020).
Identification of olfactory genes will help us understand the molecular mechanism of insect olfaction. This would be useful in developing novel environmentally friendly methods for pest management (Venthur and Zhou, 2018). For example, OBPs, CSPs, and ORs can be used to screen bioactive attractants and repellents and antagonists of Orco could inhibit insect olfactory behavior (Leal et al., 2008; Kepchia et al., 2017; Choo et al., 2018; Zeng et al., 2018). Knockdown and knockout of particular genes by RNA interference and CRISPR techniques, respectively, can effectively block the communication between pest insects and their hosts (Pelletier et al., 2010; Dong et al., 2017; Garczynski et al., 2017; Zhu et al., 2019).
The greater wax moth, Galleria mellonella, is a major pest of honeybees throughout the world (Kwadha et al., 2017). Female G. mellonella lay eggs within the beehive, and the larvae feed on the wax comb and honey. They cause heavy losses in the beekeeping industry (Zhu et al., 2016). Traditional methods for controlling G. mellonella are based on chemical insecticides, but these may cause pesticide contamination of honey products. Adult G. mellonella detect host volatiles and sex pheromone using olfactory adaptations (Payne and Finn, 1977; Li et al., 2019). The molecular mechanisms of olfaction are therefore important for identifying the key genes mediating chemical signal perception and developing RNAi-based management strategies. Zhao et al. (2019) analyzed the antennal transcriptome of G. mellonella and identified a number of chemosensory genes, including 22 OBPs, 20 CSPs, 46 ORs, 17 IRs, and 2 SNMPs. However, these numbers are fewer than the numbers found in other Lepidoptera species and suggest the existence of other, unidentified, genes.
In this study, we performed transcriptome sequencing of the G. mellonella antennae. We identified 102 olfactory-related genes, including 11 novel genes, from the transcriptome dataset. We analyzed the sequence characteristics, phylogeny, genomic distribution, and exon–intron organization of these genes. We also determined the expression profiles of the newly identified genes using reverse transcription-quantitative PCR (RT-qPCR).
The G. mellonella used in this study originated from a colony collected from infested beehives on a bee farm in Hefei, China. The larvae were reared on an artificial diet and the adults were fed on a 10% (v/v) honey solution. Insects were reared at 27°C ± 1°C, 65 ± 5% relative humidity and a photoperiod of 14:10 h (L:D).
Adult males and females (2-day-old, unmated) were sampled and different tissues were dissected. These included 300 male antennae, 300 female antennae, 60 heads (without antennae; 30 males and 30 females, pooled), 60 abdomens (30 males and 30 females, pooled), and 300 legs (150 males and 150 females, pooled). Total RNA was isolated using Trizol reagent (Life Technologies, Carlsbad, CA, United States) following manufacturer protocol. The integrity and concentration of the RNA was determined using agarose gel electrophoresis and spectrophotometry, respectively.
Total RNA (20 μg) from male and female antennae were used to create cDNA libraries. In brief, poly(A)+ mRNA was purified from total RNA using oligo(dT) magnetic beads and was digested to short fragments in a fragmentation buffer. The fragmented mRNA was used to generate first-strand cDNA using a random hexamer primer and MMLV reverse transcriptase, and second-strand cDNA was subsequently synthetized in a mixture of DNA polymerase I, dNTPs and RNaseH. The double-stranded cDNA was treated with T4 DNA polymerase for end-repair and T4 polynucleotide kinase for dA-tailing. After ligation of the sequencing adapters with T4 DNA ligase, these fragments were used as templates for PCR amplification. Finally, the PCR product was heat-denatured and the single-stranded cDNA was cyclized by splint oligonucleotide and DNA ligase to generate the library.
The cDNA libraries from male and female antennae were sequenced on a BGISEQ-500 system using a paired-end sequencing method according to manufacturer instructions at the Beijing Genomics Institute (BGI-Wuhan, Wuhan, China). Before de novo assembly, the adapters and low-quality reads were filtered, and removed, from the raw data. Clean reads from males and females were assembled into a single assembly using Trinity software (v2.0.6; Grabherr et al., 2011). Reads were combined to form contigs, from which scaffolds were extended by paired-end joining and gap-filling. If a scaffold could not be extended on either end, it was defined as a unigene. Functional annotation of each unigene was performed using the BLASTX program against the NCBI non-redundant (NR) database, Gene Ontology (GO), and eukaryotic orthologous groups (KOG) with a cut-off e-value of 10–5. The Kyoto Encyclopedia of Genes and Genomes (KEGG) pathways annotations were performed using the KEGG automatic annotation server (Yoshizawa et al., 2007).
Candidate olfactory genes were identified by retrieving the transcriptome dataset with the TBLASTN program (Altschul et al., 1997). The annotated protein sequences of OBPs, CSPs, ORs, IRs, and SNMPs from other Lepidoptera species, including Bombyx mori, Plutella xylostella, Manduca sexta, Helicoverpa armigera, Spodoptera litura, Chilo suppressalis, Cnaphalocrocis medinalis, and Ostrinia furnacalis, were used as queries. The cut-off e-value was set as 10–5. The output was manually checked, and overlapping variants were eliminated. Finally, all the candidates were confirmed by searching against the NCBI NR database using the BLASTX online program1 (cut-off e-value: 10–5). In addition, all the candidate genes were compared with those reported by Zhao et al. (2019) using BLASTN program (cut-off e-value: 10–5; Altschul et al., 1997), in order to find novel olfactory genes in G. mellonella.
The open reading frame (ORF) was predicted using ORF Finder2. The theoretical molecular weight (Mw) and isoelectric point (pI) were obtained using an ExPASy tool3. Putative signal peptide and the transmembrane domain were predicted with SignalP4 and TMHMM5, respectively. The Clustal Omega program6 was used to align deduced protein sequences. The phylogenetic trees were constructed with MEGA7 software using the neighbor-joining method with 1,000-fold bootstrap resampling (Kumar et al., 2016). The trees were viewed and edited using FigTree software7. The GenBank accession numbers of sequences used in the phylogenetic analyses are listed in Supplementary Table 1. Motif pattern analysis was performed using the MEME program8; insect OBPs and CSPs used in the analysis are listed in Supplementary Table 2. The genomic distribution of each gene was determined by mapping the cDNA with the G. mellonella genomic DNA (Lange et al., 2018) using the Splign program9.
Total RNA from different adult tissues (see “Sample Collection and RNA Extraction” section) was reverse transcribed to generate first-strand cDNA using ReverTra Ace qPCR RT Master Mix with gDNA Remover (Toyobo, Osaka, Japan). Each cDNA sample was diluted to 10 ng/μL using nuclease-free water. RT-qPCR was performed in a 20 μL reaction mixture containing 10 μL SYBR Green Real-time PCR Master Mix (Toyobo, Osaka, Japan), 1 μL (10 ng) cDNA template, 0.4 μL (0.2 μM) of forward primer, 0.4 μL (0.2 μM) of reverse primer, and 8.2 μL nuclease-free water. Primers for RT-qPCR are listed in Supplementary Table 3, and the glyceraldehyde-3-phosphate dehydrogenase (GAPDH) gene was used as an internal reference to normalize target gene expression. RT-qPCR reactions were conducted in 96-well plates and run on a CFX96 Real-time System (Bio-Rad, Hercules, CA, United States). The thermal cycle parameters were one cycle of 95°C for 2 min, 40 cycles of 95°C for 5 s, and 60°C for 20 s. At the end of each thermal cycle, the PCR products were analyzed using a heat-dissociation protocol to confirm that only one single gene was amplified. A no-template control and a no-reverse transcriptase control were both included in each reaction plate to detect possible contamination. The experiment was biologically repeated three times (each with four technical replicates). Relative expression levels were calculated by using the 2–ΔΔCt method (Livak and Schmittgen, 2001).
Data were analyzed using Data Processing System (DPS) software version 9.5 (Tang and Zhang, 2013). One-way analysis of variance (ANOVA) with Tukey’s post hoc test was performed to analyze differences of gene expression levels among multiple samples. Comparisons were considered significant at a p < 0.05.
In total, 73.8 and 73.9 Mb raw reads were generated from the transcriptomes of male and female antennae, respectively (Table 1). These data have been deposited into the NCBI Sequence Read Archive (SRA) database under accession numbers SRR8307568 (male antennae) and SRR8307567 (female antennae). After data filtration, 70.3 Mb (male antennae) and 70.6 Mb (female antennae) clean reads were obtained. Clean reads from the two transcriptomes were assembled into 42,544 unigenes (Table 1). The size distribution analysis showed that the lengths of 18,844 unigenes (44.3% of all unigenes) were greater than 1,000 bp (Figure 1A).
Figure 1. Summary of the G. mellonella unigene assembly. (A) Size distribution of unigenes. (B) Species distribution of unigenes based on homology searching against the NCBI NR database. (C) GO classification of unigenes.
We annotated the G. mellonella unigenes by searching against the NCBI NR database. A total of 14,481 (34%) of the 42,544 unigenes resulted from the search (Figure 1B). For species distribution, the G. mellonella unigenes were best matched to those from other species of Lepidoptera, including B. mori (40.6%), P. xylostella (23.7%), and Danaus plexippus (23.2%) (Figure 1B). Next, we performed a GO analysis to better classify the functions of the G. mellonella unigenes. The results indicated that 8,887 (20.9%) of the unigenes could be annotated to at least one GO term (Figure 1C). Among the GO categories, the G. mellonella unigenes were mostly enriched in “binding” and “catalytic activity” categories in the “molecular function” level, followed by “cell” and “membrane part” categories in the “cellular component” level, and “cellular process” category in the “biological process” level (Figure 1C). We also performed the functional classification for the unigenes by searching against KOG and KEGG databases, and the results are shown in Supplementary Figures 1, 2, respectively.
Zhao et al. (2019) identified 22 OBPs from G. mellonella antennae, including 2 GOBPs, 3 PBPs, and 17 OBPs. In the present study, we screened the antennal transcriptome dataset and identified 21 genes (Supplementary Table 4). Of these, four (GmelOBP18 to GmelOBP21) are novel genes. A comprehensive list of G. mellonella OBPs is shown in Supplementary Figure 3, and the nucleotide and amino acid sequences of genes identified are listed in Supplementary Table 5. We found at least 26 OBPs expressed in the antennae of G. mellonella. Of the identified OBPs, 16 sequences had complete ORFs, while GmelOBP4, GmelOBP20, and GmelOBP21 lacked the 5′- and/or 3′-terminus (Supplementary Table 4). Most of the OBPs shared ≥51% amino acid identities with orthologs from other Lepidoptera species, whereas three OBPs, GmelOBP8, GmelOBP13, and GmelOBP18, shared 28, 47, and 38% amino acid identities, respectively, with their respective orthologs (Supplementary Table 4).
The multiple sequence alignment result showed that six positionally conserved cysteine residues were presented in all OBP proteins except for GmelOBP14, which only had four cysteine residues (Supplementary Figure 4). A phylogenetic tree was constructed and the results indicated that the G. mellonella OBPs were well-segregated from each other with high bootstrap support; most of them were clustered with at least one lepidopteran ortholog (Figure 2). We used the MEME program to investigate the motif patterns in the identified OBPs and found eight conserved motifs (Figure 3A). GmelGOBP1, GmelPBP2, and GmelPBP3 have the same motif pattern 4-3-1-5-6-2-8; GmelGOBP2 is similar to GmelGOBP1 but lacks the seventh and eighth motifs at its C-terminus, whereas GmelPBP1 lacks the first motif at the N-terminus (Figure 3B). The most conserved motif pattern (4-1-2) was observed in nine OBPs (GmelOBP1/2/3/8/13/16/18/20/21), whereas GmelOBP7 and GmelOBP17 only had the fourth motif (Figure 3B).
Figure 2. Phylogenetic analysis of OBPs from G. mellonella (Gmel-prefix) and other lepidopterans, including Bombyx mori (Bmor), Plutella xylostella (Pxyl), Helicoverpa armigera (Harm), Chilo suppressalis (Csup), and Cnaphalocrocis medinalis (Cmed). Bootstrap values are indicated by colors from green (0) to red (100). The G. mellonella OBPs are highlighted in red. GenBank accession numbers of genes are listed in Supplementary Table 1.
Figure 3. Motif pattern analysis of G. mellonella OBPs. (A) The discovered eight motifs (motif1–8) in GmelOBP proteins and their homologs from other lepidopterans. The number in the parentheses indicated the expect-value (e-value) of each motif calculated by MEME program. (B) Locations of each motif in the protein sequences. The numbers in the colored boxes correspond to the numbered motifs in (A). The protein sequences of the OBPs used are listed in Supplementary Table 2.
A total of 18 CSP genes were retrieved from the transcriptome dataset (Supplementary Tables 4, 5). All of these CSPs had complete ORFs and the length of the deduced proteins ranged from 97 to 131 amino acids (Supplementary Table 4). BLASTX best hit results showed that three CSPs (GmelCSP9, GmelCSP12, and GmelCSP13) had low amino acid identities (33–42%) to other known CSPs, whereas the other 15 CSPs had high amino acid identities (61–85%) to their lepidopteran orthologs (Supplementary Table 4).
Multiple sequence alignment showed that all the deduced GmelCSP proteins had four positionally conversed cysteines (Supplementary Figure 5). Phylogenetic analysis showed that, like GmelOBPs, most GmelCSPs were spread across different branches and that they were clustered with at least one lepidopteran ortholog (Figure 4). The MEME program revealed that the motif pattern 8-3-5-1-6-2-7-4 is most conserved, which existed in 10 (GmelCSP1/2/3/4/5/8/10/16/18/20) of the 20 CSPs (Figure 5). GmelCSP7 and GmelCSP11 had the motif pattern 8-3-5-1-6-2-4, and GmelCSP13 and GmelCSP15 had the pattern 8-3-1-6-2-4. Other GmelOBPs had distinct patterns (Figure 5).
Figure 4. Phylogenetic analysis of CSPs from G. mellonella (Gmel-prefix) and other lepidopterans, including Bombyx mori (Bmor), Plutella xylostella (Pxyl), Helicoverpa armigera (Harm), Chilo suppressalis (Csup), and Cnaphalocrocis medinalis (Cmed). Bootstrap values are indicated by colors from green (0) to red (100). The G. mellonella CSPs are highlighted in red. GenBank accession numbers of genes are listed in Supplementary Table 1.
Figure 5. Motif pattern analysis of G. mellonella CSPs. (A) Eight motifs (motif 1–8) discovered in GmelCSP proteins and their lepidopteran homologs. The number in the parentheses indicated the expect-value (e-value) calculated by MEME program. (B) Locations of each motif in the protein sequences. The numbers in the colored boxes correspond to the numbered motifs in (A). The CSP protein sequences used are listed in Supplementary Table 2.
We identified 43 putative ORs from the transcriptome (Supplementary Tables 4, 5). Of these, five (GmelOR46 to GmelOR50) were novel genes, and other sequences were previously identified by Zhao et al. (2019) (Supplementary Figure 3). The total number of GmelORs is expected to reach 51. Of the ORs, 27 sequences had complete ORFs, whereas other sequences had truncations in the 5′- and/or 3′-ternimus (Supplementary Table 4). The length of the deduced OR proteins ranged from 163 to 474 amino acids, and the transmembrane domains were predicted in all the OR proteins (Supplementary Table 4). BLASTX best hit results showed that all the GmelORs had orthologs in other species of Lepidoptera, including O. furnacalis, Amyelois transitella, H. armigera, and B. mori (Supplementary Table 4). In the phylogenetic analysis, GmelORs were well-segregated from each other with high bootstrap support, and most of them were clustered with at least one lepidopteran ortholog (Figure 6). As expected, the olfactory co-receptor, GmelOrco, was clustered into a branch with Orcos from C. suppressalis, O. furnacalis, P. xylostella, and B. mori (Figure 6). Additionally, GmelOR13 and GmelOR50 fell into the “Lepidopteran pheromone receptors (PRs)” clade with PRs from other Lepidoptera species, e.g., BmorOR1 and BmorOR3 from B. mori; PxylOR1 and PxylOR4 from P. xylostella (Figure 6).
Figure 6. Phylogenetic relationships of lepidopteran ORs. Gmel: Galleria mellonella; Bmor: Bombyx mori; Ofur: Ostrinia furnacalis; Csup: Chilo suppressalis; Pxyl: Plutella xylostella. Bootstrap values are indicated by colors from green (0) to red (100). The G. mellonella ORs are highlighted in red. GmelOR30 was not used for the analysis due to its short length. GenBank accession numbers of ORs used are listed in Supplementary Table 1.
We identified 18 putative IRs, including two novel genes (Supplementary Tables 4, 5). Together with the results of Zhao et al., the expected number of IRs in G. mellonella antennae is at least 19 (Supplementary Figure 3). Of these, 15 IRs had complete ORFs and the length of the deduced proteins ranged from 451 to 931 amino acid residues. All of the GmelIRs were transmembrane proteins which contained 2–4 transmembrane domains (Supplementary Table 4). Most of the GmelIRs shared ≥52% amino acid identities with their respective orthologs from other lepidopterans except for GmelIR7d and GmelIR75q1, which shared 45 and 48% amino acid identities, respectively, with other insect IRs (Supplementary Table 4). Phylogenetic analysis showed that most of the GmelIRs were segregated from each other, and that most GmelIRs were located on branches with other lepidopteran IRs (Figure 7). In addition, two putative co-receptors, GmelIR8a and GmelIR25a, were also identified (Supplementary Table 4 and Figure 7).
Figure 7. Phylogenetic relationships of insect IRs. Dmel: Drosophila melanogaster; Bmor: Bombyx mori; Ofur: Ostrinia furnacalis; Pxyl: Plutella xylostella; Cmed: Cnaphalocrocis medinalis. Bootstrap values are indicated by colors from green (0) to red (100). The G. mellonella IRs are highlighted in red. GenBank accession numbers of IRs used are listed in Supplementary Table 1.
We identified two SNMPs in G. mellonella, namely, GmelSNMP1 and GmelSNMP2. GmelSNMP1 shared 71% amino acid identity with SNMP1 in Eogystia hippophaecolus, while GmelSNMP2 was more similar to the O. nubilalis SNMP2 (66% amino acid identity) (Supplementary Table 4). The two deduced GmelSNMP proteins both had two transmembrane domains, and had five positionally conserved cysteine residues (Supplementary Figure 6). Phylogenetic analysis showed that GmelSNMP1 and GmelSNMP2 had a close relationship with their lepidopteran orthologs (Figure 8).
Figure 8. Phylogenetic relationships of insect SNMPs. Gmel: Galleria mellonella; Apol: Antheraea polyphemus; Bmor: Bombyx mori; Csup: Chilo suppressalis; Cmed: Cnaphalocrocis medinalis; Hvir: Heliothis virescens; Msex: Manduca sexta; Ofur: Ostrinia furnacalis; Agam: Anopheles gambiae; Amel: Apis mellifera; Dmel: Drosophila melanogaster; Tcas: Tribolium castaneum. Bootstrap values are indicated by colors from green (0) to red (100). The G. mellonella SNMPs are highlighted in red. GenBank accession numbers of genes used are listed in Supplementary Table 1.
We determined the genomic distribution of the olfactory genes identified from G. mellonella by mapping the cDNA sequences to genome scaffolds. We successfully matched the 118 genes (containing 16 genes identified by Zhao et al., 2019) to 61 scaffolds (Supplementary Table 6). Of the 26 OBPs, 2 GmelGOBPs, and 3 GmelPBPs were located on scaffold53, while another 10 OBPs (GmelOBP3/5/6/8/9/13/16/18/19/21) were tandemly arrayed on scaffold145 (Figure 9A and Supplementary Table 6). Of the 20 CSPs, 17 were found to be clustered within a 123 kb genomic region on scaffold11 (Figure 9B and Supplementary Table 6).
For ORs, we found that most of the scaffolds contained only one or two OR genes; the exceptions were scaffold2, scaffold42, scaffold43, scaffold611, and scaffold681, each of which contained three GmelORs (Supplementary Table 6). For IRs and SNMPs, we mapped GmelIR75p1 and GmelIR75p2 on scaffold319, and GmelIR75q1 and GmelIR75q2 on scaffold172 (Supplementary Table 6). The remaining IRs, as well as the SNMPs, were located individually on a single scaffold (Supplementary Table 6).
The tissue- and sex-biased expression profiles of the newly identified genes (four OBPs, five ORs, and two IRs) were investigated using RT-qPCR. All the tested genes were predominantly or highly expressed in the antennae (Figure 10). Of these, the transcripts of GmelOBP19 and GmelOR47 were enriched in male antennae, with expression levels 1.8-fold (GmelOBP19) and 2.7-fold (GmelOR47) higher in males than in females, respectively (Figure 10). Other genes were expressed at equal or near-equal amounts in the antennae of both sexes (Figure 10).
Figure 10. Expression profiles of olfactory genes in different adult tissues. mA: male antennae, fA: female antennae, H: head (without antennae), Ab: abdomen, L: legs. Gene expression levels in various tissues were normalized relative to that in male antennae (set as onefold). Data are presented as mean (n = 3) ± SE. Different lowercase letters indicate significant differences (p < 0.05, one-way ANOVA with Tukey’s test).
We constructed a transcriptome dataset from the G. mellonella antennae. Zhao et al. (2019) previously identified 22 OBPs, 20 CSPs, 46 ORs, 17 IRs, and 2 SNMPs in G. mellonella antennae. Here, we discovered 102 olfactory-related genes, including 11 novel genes. Our findings, together with the results of Zhao et al. (2019), provide a comprehensive data resource for the olfactory genes in G. mellonella.
We identified 21 OBPs, including four novel genes, in G. mellonella antennae. Therefore, the total number of OBPs in the G. mellonella antennae is at least 26. Although this number is lower than the number in Drosophila melanogaster (52 genes), M. sexta (49 genes), Spodoptera littoralis (49 genes), and B. mori (46 genes) (Gong et al., 2009a; Vieira and Rozas, 2011; Vogt et al., 2015; Walker et al., 2019), it is comparable to those from other Lepidoptera, such as O. furnacalis (23 genes), S. exigua (24 genes); P. xylostella (24 genes), S. frugiperda (25 genes), and C. suppressalis (26 genes) (Cao et al., 2014; Zhang et al., 2015, 2018; Yang et al., 2017; Qiu et al., 2020). A number of OBP genes are specifically expressed in non-olfactory tissues such as abdomen and legs, as well as in larval stages of other insect species (Hull et al., 2014; Vogt et al., 2015). Since we only sequenced the antennal transcriptome of G. mellonella, some OBP genes might have been missed in the present research. Further studies examining additional tissues and developmental stages are needed.
The number of CSP genes in insect genomes appears to be highly variable. For instance, 34 and 33 CSPs were found in lepidopterans D. plexippus and Heliconius melpomene, respectively, whereas only four were discovered in the dipteran D. melanogaster (Vieira and Rozas, 2011; The Heliconius Genome Consortium, 2012). In this study, we identified 18 CSPs in G. mellonella antennae. The number of GmelCSPs is expected to reach 20 when combined with the genes discovered by Zhao et al. (2019). This number is less than the number in D. plexippus (34 genes) and H. melpomene (33 genes), but comparable to those identified in other lepidopterans, including S. exigua (19 genes; Zhang et al., 2018), Plodia interpunctella (15 genes; Jia et al., 2018), and Streltzoviella insularis (12 genes; Yang et al., 2019).
The motif pattern varies in different OBP and CSP proteins in insects (Zhang et al., 2016; Sun et al., 2017). Within G. mellonella OBPs, the most conserved pattern is 4-1-2, whereas 8-3-5-1-6-2-7-4 is most conserved in CSPs. This result implies a conserved function of the two protein families in odor recognition. We found that two GmelGOBPs and three GmelPBPs displayed different motif patterns: GmelGOBP2 lost the seventh and eighth motifs, and GmelPBP1 lacks the first motif, when compared with those in GmelGOBP1, GmelPBP2, and GmelPBP3 (Figure 3B). This difference suggests a possible functional differentiation. Indeed, a number of studies have indicated that lepidopteran GOBPs and PBPs display different affinities to odorants (Liu et al., 2015; Zhang et al., 2017).
We identified 43 ORs from G. mellonella, including 5 novel genes. The total number (51 genes) of ORs in G. mellonella is less than the 66 and 73 genes identified, respectively, in the genomes of B. mori and S. litura, two model lepidopteran insect species (Tanaka et al., 2009; Cheng et al., 2017), but comparable to those in P. xylostella (54 genes; Yang et al., 2017), O. furnacalis (52 genes; Yang et al., 2015), and M. sexta (48 genes; Grosse-Wilde et al., 2011). Numerous studies have reported that a subset of OR genes in insects have higher transcription levels in non-olfactory tissues than in antennae (Fleischer et al., 2018). Thus, our sequencing of the antennae limits our ability to identify potential OR genes enriched in other non-olfactory organs. We also identified GmelOrco, the olfactory co-receptor, from G. mellonella. Insect Orco is an essential component for forming a functional OR unit (Leal, 2013). Therefore, the identification of GmelOrco greatly benefits the development of synthetic inhibitors or genome-editing approaches to control this insect pest (Koutroumpa et al., 2016; Kepchia et al., 2017; Liu et al., 2017a).
Apart from ORs, 18 IRs were identified in our transcriptome search. In Lepidoptera, 17, 18, 21, and 21 IRs were found in the antennae of S. littoralis, B. mori, H. armigera, and O. furnacalis, respectively (Croset et al., 2010; Poivet et al., 2013; Liu et al., 2014; Yang et al., 2015). Thus, the IR gene number in G. mellonella antennae is comparable to those in other Lepidoptera. We also identified the orthologs (GmelIR8a and GmelIR25a) of the highly conserved co-receptors IR8a and IR25a. The two genes are expected to encode functional proteins and play a central role in forming a functional IR receptor complex (Abuin et al., 2011, 2019). The M. sexta IR8a is required for acid detection and is involved in the avoidance of acids from caterpillar feces (Zhang et al., 2019).
We identified two SNMPs (GmelSNMP1 and GmelSNMP2) in G. mellonella. Previous research on Heliothis virescens and Antheraea polyphemus demonstrated that SNMP1s are co-expressed with PRs in the pheromone-responsive neurons, whereas SNMP2s are expressed in the supporting cells around the neurons (Forstner et al., 2008). Two SNMPs have distinct expression patterns in the antennal sensilla of Ectropis obliqua (Sun et al., 2019b), suggesting a functional diversification between the two genes. In D. melanogaster, H. virescens, and B. mori, SNMP1s play critical roles in pheromone signaling (Jin et al., 2008; Pregitzer et al., 2014; Zhang et al., 2020). The two GmelSNMPs identified here showed very high identities with orthologs in other insect species, indicating functional conservation among these proteins.
We analyzed the genomic distribution of olfactory genes in G. mellonella and found that a large number of OBPs and CSPs were located on the same scaffolds and formed gene clusters. Two or more OBP or CSP loci located on the same scaffold implies that they were derived through duplication events during evolution (Vieira and Rozas, 2011; Vogt et al., 2015). It is possible that the G. mellonella OBP and CSP families evolved through gene duplication. Clusters of OBP and CSP genes on the same scaffold have also been found in the genomes of many other insect species including D. melanogaster, Apis mellifera, Anopheles gambiae, and B. mori (Hekmat-Scafe et al., 2002; Forêt and Maleszka, 2006; Gong et al., 2009a). Further analysis of OBP or CSP gene duplication events in G. mellonella is needed and will extend our knowledge of gene evolution. G. mellonella adults display a unique pair-forming behavior in which the sex pheromone is produced by males and perceived by conspecific females (Kwadha et al., 2017). Hence, olfactory genes that are primarily expressed in female antennae might be involved in recognizing sex pheromone constituents. Previously, Zhao et al. (2019) identified several female antennae-biased genes and hypothesized that they may contribute to pheromone detection. In this study, we analyzed the expression profiles of the newly identified genes. However, we were unable to identify female antennae-biased genes in G. mellonella; we only found two genes (GmelOBP19 and GmelOR47) that were mainly expressed in the male antennae. The male antennae-biased expression suggests that these genes may play a role in the recognition of volatiles from females and/or beehives. In other insect species, including E. obliqua, O. furnacalis, Cotesia vestalis, Laodelphax striatellus, Leptocorisa acuta, Histia rhodope, Phthorimaea operculella, and C. medinalis, a number of olfactory genes were also mainly expressed in male antennae (Zhang et al., 2015; Liu et al., 2017b, 2020; Sun et al., 2017; Li et al., 2020; Qu et al., 2020; Yang et al., 2020; He et al., 2021).
In conclusion, this study generated a transcriptome dataset of G. mellonella antennae. From the dataset, we identified numerous olfactory genes, including 21 OBPs, 18 CSPs, 43 ORs, 18 IRs, and 2 SNMPs. Several genes displayed tissue- and sex-biased expression patterns, suggesting they may play a role in olfactory processes. These results, together with the data of Zhao et al. (2019) provide a resource for olfactory genes in G. mellonella. Future functional studies on these genes will provide greater understanding of the molecular mechanisms underlying G. mellonella olfaction.
The datasets presented in this study can be found in online repositories. The names of the repository/repositories and accession number(s) can be found below: https://www.ncbi.nlm.nih.gov/, SRR8307567 and SRR8307568.
X-CJ, SL, X-YJ, Z-WW, L-SY, and H-QC conceived and designed the experimental plan. X-CJ, SL, X-YJ, Z-WW, J-JX, QG, C-WS, T-FS, and H-RZ performed the experiments. X-CJ, SL, L-SY, and H-QC analyzed the data. X-CJ and SL drafted the manuscript. L-SY and H-QC refined and approved the final manuscript. All authors contributed to the article and approved the submitted version.
This work was supported by the National Natural Science Foundation of China (Grant No. 31801806), the Major Science and Technology Project of Anhui Province (Grant No. 201903a06020027), the National Key Research and Development Program of China (Grant No. 2017YFD0200902), and the National Undergraduate Training Program for Innovation and Entrepreneurship (Grant No. 201910364078).
The authors declare that the research was conducted in the absence of any commercial or financial relationships that could be construed as a potential conflict of interest.
The Supplementary Material for this article can be found online at: https://www.frontiersin.org/articles/10.3389/fphys.2021.663040/full#supplementary-material
Supplementary Figure 1 | KOG classification of the Galleria mellonella unigenes.
Supplementary Figure 2 | KEGG classification of the G. mellonella unigenes.
Supplementary Figure 3 | A comprehensive list of G. mellonella OBP, CSP, OR, and IR genes.
Supplementary Figure 4 | Partial alignment of the protein sequences of G. mellonella OBPs.
Supplementary Figure 5 | Alignment of the deduced protein sequences of G. mellonella CSPs.
Supplementary Figure 6 | Alignment of deduced G. mellonella SNMP protein sequences with orthologs from other insect species.
Supplementary Table 1 | Accession numbers of OBPs, CSPs, ORs, IRs, and SNMPs used in phylogenetic analyses.
Supplementary Table 2 | Amino acid sequences of OBPs and CSPs used in motif pattern analyses.
Supplementary Table 3 | Primers used in this study.
Supplementary Table 4 | Details of the olfactory genes identified in the G. mellonella antennal transcriptome.
Supplementary Table 5 | Nucleotide and amino acid sequences of chemosensory genes identified in G. mellonella.
Supplementary Table 6 | Details of the genomic distribution of the G. mellonella olfactory genes.
Abuin, L., Bargeton, B., Ulbrich, M. H., Isacoff, E. Y., Kellenberger, S., and Benton, R. (2011). Functional architecture of olfactory ionotropic glutamate receptors. Neuron 69, 44–60. doi: 10.1016/j.neuron.2010.11.042
Abuin, L., Prieto-Godino, L. L., Pan, H., Gutierrez, C., Huang, L., Jin, R., et al. (2019). In vivo assembly and trafficking of olfactory ionotropic receptors. BMC Biol. 17:34. doi: 10.1186/s12915-019-0651-7
Altschul, S. F., Madden, T. L., Schäffer, A. A., Zhang, J., Zhang, Z., Miller, W., et al. (1997). Gapped BLAST and PSI-BLAST: a new generation of protein database search programs. Nucleic Acids Res. 25, 3389–3402. doi: 10.1093/nar/25.17.3389
Benton, R., Vannice, K. S., Gomez-Diaz, C., and Vosshall, L. B. (2009). Variant ionotropic glutamate receptors as chemosensory receptors in Drosophila. Cell 136, 149–162. doi: 10.1016/j.cell.2008.12.001
Brito, N. F., Moreira, M. F., and Melo, A. C. A. (2016). A look inside odorant-binding proteins in insect chemoreception. J. Insect Physiol. 95, 51–65. doi: 10.1016/j.jinsphys.2016.09.008
Butterwick, J. A., del Mármol, J., Kim, K. H., Kahlson, M. A., Rogow, J. A., Walz, T., et al. (2018). Cryo-EM structure of the insect olfactory receptor Orco. Nature 560, 447–452. doi: 10.1038/s41586-018-0420-8
Cao, D., Liu, Y., Wei, J., Liao, X., Walker, W. B., Li, J., et al. (2014). Identification of candidate olfactory genes in Chilo suppressalis by antennal transcriptome analysis. Int. J. Biol. Sci. 10, 846–860. doi: 10.7150/ijbs.9297
Cheng, T., Wu, J., Wu, Y., Chilukuri, R. V., Huang, L., Yamamoto, K., et al. (2017). Genomic adaptation to polyphagy and insecticides in a major East Asian noctuid pest. Nat. Ecol. Evol. 1, 1747–1756. doi: 10.1038/s41559-017-0314-4
Choo, Y.-M., Xu, P., Hwang, J. K., Zeng, F., Tan, K., Bhagavathy, G., et al. (2018). Reverse chemical ecology approach for the identification of an oviposition attractant for Culex quinquefasciatus. P. Natl. Acad. Sci. U.S.A. 115, 714–719. doi: 10.1073/pnas.1718284115
Croset, V., Rytz, R., Cummins, S. F., Budd, A., Brawand, D., Kaessmann, H., et al. (2010). Ancient protostome origin of chemosensory ionotropic glutamate receptors and the evolution of insect taste and olfaction. PLoS Genet. 6:e1001064. doi: 10.1371/journal.pgen.1001064
Dong, K., Sun, L., Liu, J.-T., Gu, S.-H., Zhou, J.-J., Yang, R.-N., et al. (2017). RNAi-induced electrophysiological and behavioral changes reveal two pheromone binding proteins of Helicoverpa armigera involved in the perception of the main sex pheromone component Z11–16:Ald. J. Chem. Ecol. 43, 207–214. doi: 10.1007/s10886-016-0816-6
Duan, S.-G., Li, D.-Z., and Wang, M.-Q. (2019). Chemosensory proteins used as target for screening behaviourally active compounds in the rice pest Cnaphalocrocis medinalis (Lepidoptera: Pyralidae). Insect Mol. Biol. 28, 123–135. doi: 10.1111/imb.12532
Fleischer, J., Pregitzer, P., Breer, H., and Krieger, J. (2018). Access to the odor world: olfactory receptors and their role for signal transduction in insects. Cell. Mol. Life Sci. 75, 485–508. doi: 10.1007/s00018-017-2627-5
Forêt, S., and Maleszka, R. (2006). Function and evolution of a gene family encoding odorant binding-like proteins in a social insect, the honey bee (Apis mellifera). Genome Res. 16, 1404–1413. doi: 10.1101/gr.5075706
Forstner, M., Gohl, T., Gondesen, I., Raming, K., Breer, H., and Krieger, J. (2008). Differential expression of SNMP-1 and SNMP-2 proteins in pheromone-sensitive hairs of moths. Chem. Senses 33, 291–299. doi: 10.1093/chemse/bjm087
Garczynski, S. F., Martin, J. A., Griset, M., Willett, L. S., Cooper, W. R., Swisher, K. D., et al. (2017). CRISPR/Cas9 editing of the codling moth (Lepidoptera: Tortricidae) CpomOR1 gene affects egg production and viability. J. Econ. Entomol. 110, 1847–1855. doi: 10.1093/jee/tox166
Gong, D.-P., Zhang, H.-J., Zhao, P., Xia, Q.-Y., and Xiang, Z.-H. (2009a). The odorant binding protein gene family from the genome of silkworm. Bombyx mori. BMC Genomics 10:332. doi: 10.1186/1471-2164-10-332
Gong, Z. J., Zhou, W. W., Yu, H. Z., Mao, C. G., Zhang, C. X., Cheng, J. A., et al. (2009b). Cloning, expression and functional analysis of a general odorant-binding protein 2 gene of the rice striped stem borer, Chilo suppressalis (Walker) (Lepidoptera: Pyralidae). Insect Mol. Biol. 18, 405–417. doi: 10.1111/j.1365-2583.2009.00886.x
Grabherr, M. G., Haas, B. J., Yassour, M., Levin, J. Z., Thompson, D. A., Amit, I., et al. (2011). Full-length transcriptome assembly from RNA-Seq data without a reference genome. Nat. Biotechnol. 29, 644–652. doi: 10.1038/nbt.1883
Grosse-Wilde, E., Kuebler, L. S., Bucks, S., Vogel, H., Wicher, D., and Hansson, B. S. (2011). Antennal transcriptome of Manduca sexta. P. Natl. Acad. Sci. U.S.A. 108, 7449–7454. doi: 10.1073/pnas.1017963108
He, X., Cai, Y., Zhu, J., Zhang, M., Zhang, Y., Ge, Y., et al. (2021). Identification and functional characterization of two putative pheromone receptors in the potato tuber moth, Phthorimaea operculella. Front. Physiol. 11:618983. doi: 10.3389/fphys.2020.618983
Hekmat-Scafe, D. S., Scafe, C. R., McKinney, A. J., and Tanouye, M. A. (2002). Genome-wide analysis of the odorant-binding protein gene family in Drosophila melanogaster. Genome Res. 12, 1357–1369. doi: 10.1101/gr.239402
Hull, J. J., Perera, O. P., and Snodgrass, G. L. (2014). Cloning and expression profiling of odorant-binding proteins in the tarnished plant bug. Lygus lineolaris. Insect Mol. Biol. 23, 78–97. doi: 10.1111/imb.12064
Jia, X., Zhang, X., Liu, H., Wang, R., and Zhang, T. (2018). Identification of chemosensory genes from the antennal transcriptome of Indian meal moth Plodia interpunctella. PLoS ONE 13:e0189889. doi: 10.1371/journal.pone.0189889
Jin, X., Ha, T. S., and Smith, D. P. (2008). SNMP is a signaling component required for pheromone sensitivity in Drosophila. Proc. Natl. Acad. Sci. U.S.A. 105, 10996–11001. doi: 10.1073/pnas.0803309105
Kepchia, D., Moliver, S., Chohan, K., Phillips, C., and Luetje, C. W. (2017). Inhibition of insect olfactory behavior by an airborne antagonist of the insect odorant receptor co-receptor subunit. PLoS ONE 12:e0177454. doi: 10.1371/journal.pone.0177454
Khuhro, S. A., Liao, H., Dong, X.-T., Yu, Q., Yan, Q., and Dong, S.-L. (2017). Two general odorant binding proteins display high bindings to both host plant volatiles and sex pheromones in a pyralid moth Chilo suppressalis (Lepidoptera: Pyralidae). J. Asia Pac. Entomol. 20, 521–528. doi: 10.1016/j.aspen.2017.02.015
Koutroumpa, F. A., Monsempes, C., François, M.-C., de Cian, A., Royer, C., Concordet, J.-P., et al. (2016). Heritable genome editing with CRISPR/Cas9 induces anosmia in a crop pest moth. Sci. Rep. 6:29620. doi: 10.1038/srep29620
Kumar, S., Stecher, G., and Tamura, K. (2016). MEGA7: molecular evolutionary genetics analysis version 7.0 for bigger datasets. Mol. Biol. Evol. 33, 1870–1874. doi: 10.1093/molbev/msw054
Kwadha, C. A., Ong’amo, G. O., Ndegwa, P. N., Raina, S. K., and Fombong, A. T. (2017). The biology and control of the greater wax moth, Galleria mellonella. Insects 8:61. doi: 10.3390/insects8020061
Lange, A., Beier, S., Huson, D. H., Parusel, R., Iglauer, F., and Frick, J.-S. (2018). Genome sequence of Galleria mellonella (greater wax moth). Genome Announc. 6:e01220. doi: 10.1128/genomeA.01220-17
Leal, W. S. (2013). Odorant reception in insects: roles of receptors, binding proteins, and degrading enzymes. Annu. Rev. Entomol. 58, 373–391. doi: 10.1146/annurev-ento-120811-153635
Leal, W. S., Barbosa, R. M. R., Xu, W., Ishida, Y., Syed, Z., Latte, N., et al. (2008). Reverse and conventional chemical ecology approaches for the development of oviposition attractants for Culex mosquitoes. PLoS One 3:e3045. doi: 10.1371/journal.pone.0003045
Li, Y., Hu, J., Xiang, Y., Zhang, Y., Chen, D., and Liu, F. (2020). Identification and comparative expression profiles of chemosensory genes in major chemoreception organs of a notorious pests, Laodelphax striatellus. Comp. Biochem. Physiol. D 33:100646. doi: 10.1016/j.cbd.2019.100646
Li, Y., Jiang, X., Wang, Z., Zhang, J., Klett, K., Mehmood, S., et al. (2019). Losing the arms race: greater wax moths sense but ignore bee alarm pheromones. Insects 10:81. doi: 10.3390/insects10030081
Li, Z.-Q., Zhang, S., Luo, J.-Y., Zhu, J., Cui, J.-J., and Dong, S.-L. (2015). Expression analysis and binding assays in the chemosensory protein gene family indicate multiple roles in Helicoverpa armigera. J. Chem. Ecol. 41, 473–485. doi: 10.1007/s10886-015-0574-x
Liu, N.-Y., Xu, W., Papanicolaou, A., Dong, S.-L., and Anderson, A. (2014). Identification and characterization of three chemosensory receptor families in the cotton bollworm Helicoverpa armigera. BMC Genomics 15:597. doi: 10.1186/1471-2164-15-597
Liu, N.-Y., Yang, K., Liu, Y., Xu, W., Anderson, A., and Dong, S.-L. (2015). Two general-odorant binding proteins in Spodoptera litura are differentially tuned to sex pheromones and plant odorants. Comp. Biochem. Physiol. A 180, 23–31. doi: 10.1016/j.cbpa.2014.11.005
Liu, Q., Liu, W., Zeng, B., Wang, G., Hao, D., and Huang, Y. (2017a). Deletion of the Bombyx mori odorant receptor co-receptor (BmOrco) impairs olfactory sensitivity in silkworms. Insect Biochem. Molec. 86, 58–67. doi: 10.1016/j.ibmb.2017.05.007
Liu, S., Wang, W.-L., Zhang, Y.-X., Zhang, B.-X., Rao, X.-J., Liu, X.-M., et al. (2017b). Transcriptome sequencing reveals abundant olfactory genes in the antennae of the rice leaffolder, Cnaphalocrocis medinalis (Lepidoptera: Pyralidae). Entomol. Sci. 20, 177–188. doi: 10.1111/ens.12253
Liu, Y., Du, L., Zhu, Y., Yang, S., Zhou, Q., Wang, G., et al. (2020). Identification and sex-biased profiles of candidate olfactory genes in the antennal transcriptome of the parasitoid wasp Cotesia vestalis. Comp. Biochem. Physiol. D 34:100657. doi: 10.1016/j.cbd.2020.100657
Livak, K. J., and Schmittgen, T. D. (2001). Analysis of relative gene expression data using real-time quantitative PCR and the 2−ΔΔCT method. Methods 25, 402–408. doi: 10.1006/meth.2001.1262
Payne, T. L., and Finn, W. E. (1977). Pheromone receptor system in the females of the greater wax moth Galleria mellonella. J. Insect Physiol. 23, 879–881. doi: 10.1016/0022-1910(77)90014-2
Pelletier, J., Guidolin, A., Syed, Z., Cornel, A. J., and Leal, W. S. (2010). Knockdown of a mosquito odorant-binding protein involved in the sensitive detection of oviposition attractants. J. Chem. Ecol. 36, 245–248. doi: 10.1007/s10886-010-9762-x
Pelosi, P., Iovinella, I., Felicioli, A., and Dani, F. R. (2014). Soluble proteins of chemical communication: an overview across arthropods. Front. Physiol. 5:320. doi: 10.3389/fphys.2014.00320
Pelosi, P., Iovinella, I., Zhu, J., Wang, G., and Dani, F. R. (2018). Beyond chemoreception: diverse tasks of soluble olfactory proteins in insects. Biol. Rev. 93, 184–200. doi: 10.1111/brv.12339
Poivet, E., Gallot, A., Montagné, N., Glaser, N., Legeai, F., and Jacquin-Joly, E. (2013). A comparison of the olfactory gene repertoires of adults and larvae in the noctuid moth Spodoptera littoralis. PLoS One 8:e60263. doi: 10.1371/journal.pone.0060263
Pregitzer, P., Greschista, M., Breer, H., and Krieger, J. (2014). The sensory neurone membrane protein SNMP1 contributes to the sensitivity of a pheromone detection system. Insect Mol. Biol. 23, 733–742. doi: 10.1111/imb.12119
Qiu, L., He, L., Tan, X., Zhang, Z., Wang, Y., Li, X., et al. (2020). Identification and phylogenetics of Spodoptera frugiperda chemosensory proteins based on antennal transcriptome data. Comp. Biochem. Physiol. D 34:100680. doi: 10.1016/j.cbd.2020.100680
Qu, M.-Q., Cui, Y., Zou, Y., Wu, Z.-Z., and Lin, J.-T. (2020). Identification and expression analysis of odorant binding proteins and chemosensory proteins from dissected antennae and mouthparts of the rice bug Leptocorisa acuta. Comp. Biochem. Physiol. D 33:100631. doi: 10.1016/j.cbd.2019.100631
Robertson, H. M. (2019). Molecular evolution of the major arthropod chemoreceptor gene families. Annu. Rev. Entomol. 64, 227–242. doi: 10.1146/annurev-ento-020117-043322
Rytz, R., Croset, V., and Benton, R. (2013). Ionotropic receptors (IRs): chemosensory ionotropic glutamate receptors in Drosophila and beyond. Insect Biochem. Molec. 43, 888–897. doi: 10.1016/j.ibmb.2013.02.007
Sun, J. S., Xiao, S., and Carlson, J. R. (2018). The diverse small proteins called odorant-binding proteins. Open Biol. 8:180208. doi: 10.1098/rsob.180208
Sun, L., Mao, T.-F., Zhang, Y.-X., Wu, J.-J., Bai, J.-H., Zhang, Y.-N., et al. (2017). Characterization of candidate odorant-binding proteins and chemosensory proteins in the tea geometrid Ectropis obliqua Prout (Lepidoptera: Geometridae). Arch. Insect Biochem. 94:e21383. doi: 10.1002/arch.21383
Sun, L., Wang, Q., Zhang, Y., Tu, X., Yan, Y., Wang, Q., et al. (2019a). The sensilla trichodea-biased EoblPBP1 binds sex pheromones and green leaf volatiles in Ectropis obliqua Prout, a geometrid moth pest that uses Type-II sex pheromones. J. Insect Physiol. 116, 17–24. doi: 10.1016/j.jinsphys.2019.04.005
Sun, L., Wang, Q., Zhang, Y., Yan, Y., Guo, H., Xiao, Q., et al. (2019b). Expression patterns and co-localization of two sensory neuron membrane proteins in Ectropis obliqua Prout, a geometrid moth pest that uses Type-II sex pheromones. Insect Mol. Biol. 28, 342–354. doi: 10.1111/imb.12555
Tanaka, K., Uda, Y., Ono, Y., Nakagawa, T., Suwa, M., Yamaoka, R., et al. (2009). Highly selective tuning of a silkworm olfactory receptor to a key mulberry leaf volatile. Curr. Biol. 19, 881–890. doi: 10.1016/j.cub.2009.04.035
Tang, Q.-Y., and Zhang, C.-X. (2013). Data processing system (DPS) software with experimental design, statistical analysis and data mining developed for use in entomological research. Insect Sci. 20, 254–260. doi: 10.1111/j.1744-7917.2012.01519.x
The Heliconius Genome Consortium. (2012). Butterfly genome reveals promiscuous exchange of mimicry adaptations among species. Nature 487, 94–98. doi: 10.1038/nature11041
Touhara, K., and Vosshall, L. B. (2009). Sensing odorants and pheromones with chemosensory receptors. Annu. Rev. Physiol. 71, 307–332. doi: 10.1146/annurev.physiol.010908.163209
Venthur, H., and Zhou, J.-J. (2018). Odorant receptors and odorant-binding proteins as insect pest control targets: a comparative analysis. Front. Physiol. 9:1163. doi: 10.3389/fphys.2018.01163
Vieira, F. G., and Rozas, J. (2011). Comparative genomics of the odorant-binding and chemosensory protein gene families across the Arthropoda: origin and evolutionary history of the chemosensory system. Genome Biol. Evol. 3, 476–490. doi: 10.1093/gbe/evr033
Vogt, R. G., Große-Wilde, E., and Zhou, J.-J. (2015). The Lepidoptera odorant binding protein gene family: gene gain and loss within the GOBP/PBP complex of moths and butterflies. Insect Biochem. Molec. 62, 142–153. doi: 10.1016/j.ibmb.2015.03.003
Vogt, R. G., Miller, N. E., Litvack, R., Fandino, R. A., Sparks, J., Staples, J., et al. (2009). The insect SNMP gene family. Insect Biochem. Molec. 39, 448–456. doi: 10.1016/j.ibmb.2009.03.007
Walker, W. B., Roy, A., Anderson, P., Schlyter, F., Hansson, B. S., and Larsson, M. C. (2019). Transcriptome analysis of gene families involved in chemosensory function in Spodoptera littoralis (Lepidoptera: Noctuidae). BMC Genomics 20:428. doi: 10.1186/s12864-019-5815-x
Wicher, D. (2018). Tuning insect odorant receptors. Front. Cell. Neurosci. 12:94. doi: 10.3389/fncel.2018.00094
Yang, B., Ozaki, K., Ishikawa, Y., and Matsuo, T. (2015). Identification of candidate odorant receptors in Asian corn borer Ostrinia furnacalis. PLoS One 10:e0121261. doi: 10.1371/journal.pone.0121261
Yang, H., Dong, J., Sun, Y., Hu, Z., Lv, Q., and Li, D. (2020). Antennal transcriptome analysis and expression profiles of putative chemosensory soluble proteins in Histia rhodope Cramer (Lepidoptera: Zygaenidae). Comp. Biochem. Physiol. D 33:100654. doi: 10.1016/j.cbd.2020.100654
Yang, S., Cao, D., Wang, G., and Liu, Y. (2017). Identification of genes involved in chemoreception in Plutella xyllostella by antennal transcriptome analysis. Sci. Rep. 7:11941. doi: 10.1038/s41598-017-11646-7
Yang, Y., Li, W., Tao, J., and Zong, S. (2019). Antennal transcriptome analyses and olfactory protein identification in an important wood-boring moth pest, Streltzoviella insularis (Lepidoptera: Cossidae). Sci. Rep. 9:17951. doi: 10.1038/s41598-019-54455-w
Yoshizawa, A. C., Itoh, M., Okuda, S., Moriya, Y., and Kanehisa, M. (2007). KAAS: an automatic genome annotation and pathway reconstruction server. Nucleic Acids Res. 35, W182–W185. doi: 10.1093/nar/gkm321
Zeng, F.-F., Liu, H., Zhang, A., Lu, Z.-X., Leal, W. S., Abdelnabby, H., et al. (2018). Three chemosensory proteins from the rice leaf folder Cnaphalocrocis medinalis involved in host volatile and sex pheromone reception. Insect Mol. Biol. 27, 710–723. doi: 10.1111/imb.12503
Zhang, H.-J., Xu, W., Chen, Q.-m, Sun, L.-N., Anderson, A., Xia, Q.-Y., et al. (2020). A phylogenomics approach to characterizing sensory neuron membrane proteins (SNMPs) in Lepidoptera. Insect Biochem. Molec. 118:103313. doi: 10.1016/j.ibmb.2020.103313
Zhang, J., Bisch-Knaden, S., Fandino, R. A., Yan, S., Obiero, G. F., Grosse-Wilde, E., et al. (2019). The olfactory coreceptor IR8a governs larval feces-mediated competition avoidance in a hawkmoth. Proc. Natl. Acad. Sci. U.S.A. 116, 21828–21833. doi: 10.1073/pnas.1913485116
Zhang, L.-W., Kang, K., Jiang, S.-C., Zhang, Y.-N., Wang, T.-T., Zhang, J., et al. (2016). Analysis of the antennal transcriptome and insights into olfactory genes in Hyphantria cunea (Drury). PLoS One 11:e0164729. doi: 10.1371/journal.pone.0164729
Zhang, T., Coates, B. S., Ge, X., Bai, S., He, K., and Wang, Z. (2015). Male- and female-biased gene expression of olfactory-related genes in the antennae of Asian corn borer, Ostrinia furnacalis (Guenée) (Lepidoptera: Crambidae). PLoS One 10:e0128550. doi: 10.1371/journal.pone.0128550
Zhang, T., Sun, Y., Wanner, K. W., Coates, B. S., He, K., and Wang, Z. (2017). Binding affinity of five PBPs to Ostrinia sex pheromones. BMC Mol. Biol. 18:4. doi: 10.1186/s12867-017-0079-y
Zhang, Y.-N., Qian, J.-L., Xu, J.-W., Zhu, X.-Y., Li, M.-Y., Xu, X.-X., et al. (2018). Identification of chemosensory genes based on the transcriptomic analysis of six different chemosensory organs in Spodoptera exigua. Front. Physiol. 9:432. doi: 10.3389/fphys.2018.00432
Zhang, Y.-N., Ye, Z.-F., Yang, K., and Dong, S.-L. (2014). Antenna-predominant and male-biased CSP19 of Sesamia inferens is able to bind the female sex pheromones and host plant volatiles. Gene 536, 279–286. doi: 10.1016/j.gene.2013.12.011
Zhao, H.-X., Xiao, W.-Y., Ji, C.-H., Ren, Q., Xia, X.-S., Zhang, X.-F., et al. (2019). Candidate chemosensory genes identified from the greater wax moth, Galleria mellonella, through a transcriptomic analysis. Sci. Rep. 9:10032. doi: 10.1038/s41598-019-46532-x
Zhu, G.-H., Zheng, M.-Y., Sun, J.-B., Khuhro, S. A., Yan, Q., Huang, Y., et al. (2019). CRISPR/Cas9 mediated gene knockout reveals a more important role of PBP1 than PBP2 in the perception of female sex pheromone components in Spodoptera litura. Insect Biochem. Molec. 115:103244. doi: 10.1016/j.ibmb.2019.103244
Keywords: Galleria mellonella, antenna, transcriptome, olfactory genes, expression pattern, genomic distribution
Citation: Jiang X-C, Liu S, Jiang X-Y, Wang Z-W, Xiao J-J, Gao Q, Sheng C-W, Shi T-F, Zeng H-R, Yu L-S and Cao H-Q (2021) Identification of Olfactory Genes From the Greater Wax Moth by Antennal Transcriptome Analysis. Front. Physiol. 12:663040. doi: 10.3389/fphys.2021.663040
Received: 02 February 2021; Accepted: 22 April 2021;
Published: 19 May 2021.
Edited by:
Ya-Nan Zhang, Huaibei Normal University, ChinaReviewed by:
Tiantao Zhang, Chinese Academy of Agricultural Sciences (CAAS), ChinaCopyright © 2021 Jiang, Liu, Jiang, Wang, Xiao, Gao, Sheng, Shi, Zeng, Yu and Cao. This is an open-access article distributed under the terms of the Creative Commons Attribution License (CC BY). The use, distribution or reproduction in other forums is permitted, provided the original author(s) and the copyright owner(s) are credited and that the original publication in this journal is cited, in accordance with accepted academic practice. No use, distribution or reproduction is permitted which does not comply with these terms.
*Correspondence: Hai-Qun Cao, aGFpcXVuY2FvQDE2My5jb20=
†These authors have contributed equally to this work
Disclaimer: All claims expressed in this article are solely those of the authors and do not necessarily represent those of their affiliated organizations, or those of the publisher, the editors and the reviewers. Any product that may be evaluated in this article or claim that may be made by its manufacturer is not guaranteed or endorsed by the publisher.
Research integrity at Frontiers
Learn more about the work of our research integrity team to safeguard the quality of each article we publish.