- 1Department of Biological Sciences, Macquarie University, Sydney, NSW, Australia
- 2Department of Biology, University of Turku, Turku, Finland
- 3Institute of Biodiversity, Animal Health and Comparative Medicine, University of Glasgow, Glasgow, United Kingdom
- 4School of Molecular and Life Sciences, Curtin University, Perth, WA, Australia
Human-induced climate change is increasing the frequency, duration, and intensity of heat waves and exposure to these extreme temperatures impacts individual physiology and performance (e.g., metabolism, water balance, and growth). These traits may be susceptible to thermal conditions experienced during embryonic development, but experiments focusing on post-natal development are scant. Documented effects of heat waves on whole-body metabolism may reflect changes in mitochondrial function, but most studies do not measure physiological traits at both the cellular and whole organism levels. Here, we exposed nests of zebra finches to experimentally simulated heat waves for 18 days after hatching and measured body mass, growth rate, whole-body metabolic rate, body temperature, wet thermal conductance, evaporative water loss, and relative water economy of chicks at three ages corresponding to ectothermic (day 5), poikilothermic (day 12), and homoeothermic (day 50) stages. Additionally, we measured mitochondrial bioenergetics of blood cells 80 days post-hatch. While early-life exposure to heat wave conditions did not impact whole body metabolic and hygric physiology, body temperature was lower for birds from heated compared with control nests at both 12 and 50 days of age. There was also an effect of nest heating at the cellular level, with mitochondria from heated birds having higher endogenous and proton-leak related respiration, although oxidative phosphorylation, maximum respiratory capacity, and coupling efficiency were not impacted. Our results suggest that early-life exposure to high ambient temperature induces programming effects on cellular-level and thermal physiology that may not be apparent for whole-animal metabolism.
Introduction
Physiological and life history traits that impact fitness can be influenced by environmental conditions, in particular temperature, experienced during early life stages (Lindström, 1999; Monaghan, 2008; Conradie et al., 2019). Exposure to high temperature can elicit stress responses (Boddicker et al., 2014), alter metabolism (O’Steen and Janzen, 1999; Moraes et al., 2003; Schnurr et al., 2014), modify water balance (Williams and Tieleman, 2000; McWhorter et al., 2018), impact growth and body size (O’Steen, 1998; Andrew et al., 2017; Andreasson et al., 2018; Sauve et al., 2021), and disrupt functional processes at the subcellular level (Paital and Chainy, 2014). Understanding the physiological impacts of high temperature is particularly important considering that anthropogenic climate change is resulting in an increase in the duration, frequency, and intensity of heat waves (Meehl and Tebaldi, 2004; Tebaldi et al., 2006; Pachauri et al., 2014; Conradie et al., 2020).
For a variety of taxa, it is hypothesized that changes in whole body metabolism and other physiological and life history traits reflect functional variation at the subcellular level, such as mitochondrial performance (Tattersall et al., 2012; Jimenez et al., 2014a,b; Hood et al., 2018a,b). Despite the crucial role of mitochondrial physiology for maintenance of homeostasis (Pörtner, 2001), few experimental studies examine temperature effects on mitochondrial function together with metabolism, growth, and body size (Price et al., 2017). Consequently, the impacts of temperature-induced variation at different levels of physiological organization are currently unclear.
Some physiological variations emerge early in life from temperature effects during the sensitive stages of embryonic development (Durant et al., 2010; Nord and Nilsson, 2011; DuRant et al., 2012; Nord and Giroud, 2020; Stier et al., 2020). Embryonic stages of all organisms are ectothermic and their mitochondria are particularly sensitive to thermal variation (Chung and Schulte, 2020). However, while phenotypic responses to high temperatures during development for some domestic animals have been investigated (e.g., Mujahid et al., 2007; Huang et al., 2015), comparatively little is known about the effects of heat during the early post-natal stage on the physiology of wild animals and potential repercussions into adulthood (Andreasson et al., 2018, 2020; Halevy, 2020).
The thermoregulatory stages of altricial birds (Price and Dzialowski, 2018) make them a useful model for examining effects of high ambient temperature (Ta) experienced during the post-natal stage. Like embryos, nestlings are ectothermic after hatching (Price and Dzialowski, 2018), so if responses to heat observed during the egg stage are related to ectothermy, then nestlings may be as sensitive as embryos to changing Ta (Webb, 1987). Nestlings gradually transition to an endothermic-poikilothermic stage when their feathers begin to erupt, before finally developing full homeothermy after fledging (Sirsat et al., 2016a). Therefore, altricial passerines offer a unique opportunity for examining plastic responses to prior exposure to high Ta during three substantially different thermoregulatory states within the same individual.
Metabolic, hygric, growth, and mitochondrial consequences of exposure to high Ta may differ among populations due to genetic adaptation (Harada et al., 2019), while both acute and chronic physiological plasticity may also modify thermal responses (Williams and Tieleman, 2000; Tieleman et al., 2002, 2003; Noakes et al., 2016; Cooper et al., 2020a,b). Recent experimental data for zebra finches (Taeniopygia guttata) indicated that prior exposure of adults to simulated heat waves had little effect on their subsequent physiology (Cooper et al., 2020b). However, early life experiences can prepare subcellular and whole-animal phenotypes for subsequent thermal conditions (DuRant et al., 2013; Jonsson and Jonsson, 2014; Hepp et al., 2015; Gyllenhammer et al., 2020; Koch et al., 2021); these developmental effects can be as substantial as inter-population differences (Tracy and Walsberg, 2001). Physiological consequences of heat waves experienced during post-natal growth by altricial birds have received little attention, despite this being the period when thermoregulation develops. Here we examine the impact of exposure during the nestling period to simulated heat waves on physiological traits of zebra finches.
The zebra finch is an arid-habitat Australian passerine with a well-appreciated physiological capacity to withstand high Ta (Zann, 1996; Cooper et al., 2019). Despite this, zebra finches have been involved in mass-mortality events associated with heat waves (Finlayson, 1932; Towie, 2009). Thermal, hygric, and reproductive effects of climate change are predicted to reduce the zebra finch’s distribution and abundance (McKechnie et al., 2012; Conradie et al., 2020), but the potential for developmental plasticity to mitigate these impacts needs to be considered (Fuller et al., 2010; Boyles et al., 2011). We quantified the effects of exposure to high Ta (simulated heat wave) during the post-natal stage on physiological variables at the whole body and mitochondrial level. We measured metabolic rate (MR; measured as oxygen consumption, VO2), body temperature (Tb), and evaporative water loss (EWL) and calculated wet thermal conductance (Cwet) and relative water economy (RWE), at three thermoregulatory stages (ectothermic, poikilothermic, and endothermic; Sirsat et al., 2016a). We also measured the nestlings’ growth rate, and assessed mitochondrial function of red blood cells once they reached adulthood (e.g., early-life programming effects; Gyllenhammer et al., 2020).
Materials and Methods
Species and Housing
Groups of three male and three female zebra finches were placed in outdoor aviaries containing four shaded nest boxes. Birds were randomly selected from a population derived from wild-caught birds from western New South Wales (31.3°S, 141.6°E) and bred in captivity at Macquarie University (33.7°S, 151.1°E) for 3–7 generations. After an initial week of acclimation, the birds were provided with nesting material. Dates of clutch initiation and completion were recorded and clutches were monitored occasionally during incubation and then multiple times a day when approaching hatch date. Experiments were performed on an individual bird from each of 11 control and 11 experimentally heated nests with at least three nestlings in each nest.
Heating Protocol
The nestling stage for zebra finches lasts 18–22 days, so nests were heated for 18 days starting on the day after hatch using a Kapton flexible heating device [Omega Engineering KHA-404(10)-P], powered by a DC regulated Powertech power supply (HW1200R-12). A Vemer digital heat regulator (HT NIPT-1P3A VM628500) set at 40 ± 0.2°C controlled the heat output via a digital time switch (Vemer MICRO-D) based on the nest temperature recorded by a Vemer VE122800 double injection IP68 probe placed in the nest, for 6 h a day from 9:00 until 15:00. This temperature was selected because it approaches Tb of small songbirds (Pollock et al., 2021) challenging their capacity for heat dissipation, and approximates the daily maximal Ta recorded during the breeding season within the natural habitat for this species (Griffith et al., 2016). Control nests were fitted with sham heating devices.
Temperature was measured every 24 s at two positions inside each nest box (3 cm below the roof = Ta and on the bottom of the nest in contact with the nestlings = brood temperature; Tbr) between days 1 and 8 post-hatch. Temperature was recorded only during the ectothermic period because at later stages Tbr is influenced by the nestlings’ metabolic heat production and older nestlings tended to cluster in the corners of the box, making Tbr data less reliable. Both temperature probes were wired to a Gemini Tinytag Plus 2 data logger and temperature data analyzed using Tinytag Explorer (ver. 4.7). During the 6 h of experimental heating mean Ta (37.3 ± 1.33°C) and Tbr (35.4 ± 1.84°C) in the treatment nest boxes were significantly higher than in the control boxes (21.5 ± 1.84°C and 33.7 ± 1.33°C; t1,11 = 19.99, p < 0.001; t1,11 = 2.49, p = 0.01, respectively; Figure 1). As a consequence, the mean differential between Tbr and Ta was substantially smaller for treated nests (1.9 ± 2.5°C) compared to control nests (12.2 ± 2.6°C).
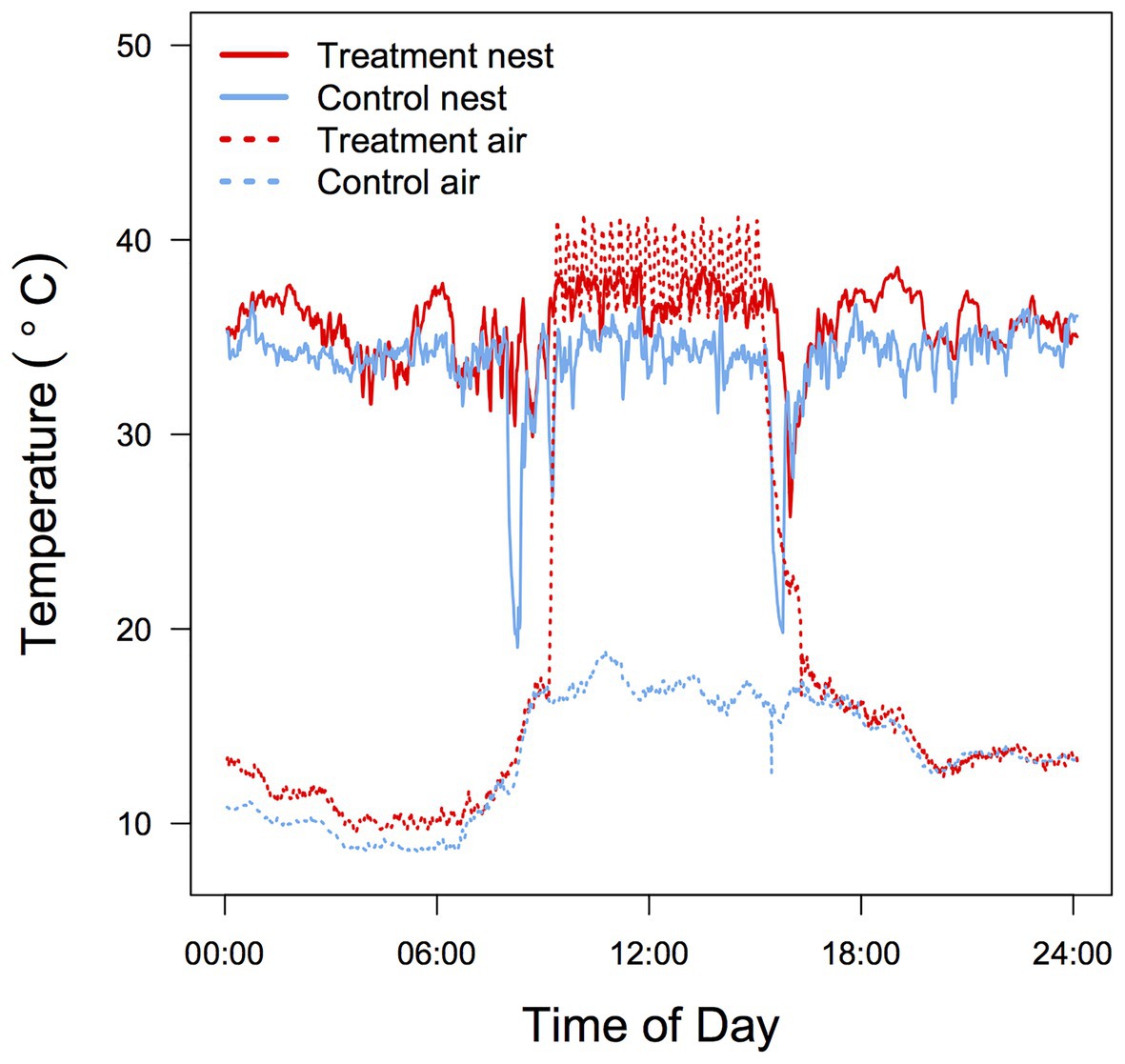
Figure 1. Typical 24 h air Ta and brood Tbr temperature profile in control and heated zebra finch (Taeniopygia guttata) nest boxes. Data were recorded in adjacent aviaries during the same day for two nests with a brood size of four.
Measurements of Metabolic Rate, Evaporative Water Loss, and Body Temperature
We measured resting metabolic rate (RMR) for the second hatched nestling of each brood (and occasionally of one other sibling) at days 5, 12, and 50 post hatch, at Ta = 40°C, a physiologically challenging Ta above thermoneutrality (Calder, 1964; Cade et al., 1965; Cooper et al., 2020b). When hatching was highly synchronous, we randomly selected one individual for measurement. Measurements at day 5 were made between 15:00 and 18:00 to allow the nestling to experience regular feeding and brooding activity. At day 12 measurements commenced at 18:00 and lasted 6–9 h, until physiological variables were stable and minimal (Page et al., 2011). Prior to measurements at day 50 birds were individually caged and fasted from 14:00 (with access to ad libitum water); they were then measured from 19:00 until 05:00 the following morning. Nestlings aged 5 and 12 days were placed in a mesh cup within the 500 ml glass metabolic chamber, while 50 day old offspring rested on a perch.
Measurements of VO2, VCO2 and EWL were made with open-flow respirometry after Withers (2001), using a Sable Systems Foxbox analyzer. Air flow through the chamber was regulated at 100, 200, and 300 ml min−1 for 5, 12, and 50 day old birds, respectively, using the Foxbox’s inbuilt pump and flow regulator. Incurrent air was scrubbed of water vapor with drierite (W Hammond Co). Excurrent chamber air passed through a Vaisala 45A thin-film capacitance RH/Ta probe interfaced to the Foxbox, it was dried with drierite, and then passed through the Foxbox’s CO2 and then O2 sensor. The serial outputs for O2, CO2, Ta, and RH were recorded by a PC every 20 s with a custom-written Visual Basic (VB v6) data acquisition program (P Withers). Two separate open-flow systems allowed for continuous measurement of two birds. Birds were weighed with an electronic scale (Nuweigh; ±0.01 g) before and after each measurement and the mean mass used for calculations. At the end of each experiment Tb (±0.1°C) was measured immediately after the bird was removed from the chamber with a plastic-tipped thermocouple (diameter 1 mm), connected to a calibrated QM1601 Digitech Thermometer (TechBrands, Australia), inserted 0.5–1.5 cm, depending on the size of the bird, into the cloaca.
Baselines of background O2, CO2, and RH were established for at least 30 min before and after each experiment. VO2, VCO2, and EWL were calculated after Withers (2001) for the ~20 min period where these values were steady and minimal, using custom-written data analysis software (VB v6; P. Withers). Since VCO2 mirrors VO2, we present only VO2 here. Wet thermal conductance (Cwet, J.h−1.°C−1) was calculated as MHP/(Tb-Ta), where MHP is metabolic heat production, calculated from the appropriate oxy-calorific conversion (Withers et al., 2016) for VO2 as determined by the respiratory exchange ratio (RER). Relative water economy (RWE) was calculated as MWP/EWL, where MWP is the metabolic water production, determined from the RER and the hygric conversion for VO2 after Withers et al. (2016).
A Sensodyne Gillian Gilibrator was used to calibrate the Foxboxs’ flow meters. Room air (20.95% O2) and nitrogen (0% O2; BOC gases, Perth, WA, Australia) were used to two-point calibrate the O2 analyzers, while the CO2 analyzers were calibrated with a precision gas mix (0.53% CO2; BOC Gases) and N2. The RH probes were calibrated at five RHs from 2% (dry, using drierite) to 85% using a Sable Systems DG4 humidity controller.
Growth Rate
Individual nestlings were marked with non-toxic markers on the tarsi to measure individual growth trajectories. Birds were weighed with an electronic scale (Nuweigh; ±0.01 g) and measurements were made every second day at the same time (16:00 ± 1 h) starting at hatch day until fledging (ca. day 20). The growth rate constant (k) was calculated using logistic regression (Ricklefs, 1968; Remeŝ and Martin, 2002; Sofaer et al., 2013) to determine the slope of the line tangent to the growth curve at the inflection point (a mass-independent estimate of growth rate), as well as asymptotic body mass (i.e., at fledging).
Mitochondrial Bioenergetic Measurements
Avian erythrocytes are nucleated and have functional mitochondria (Stier et al., 2013), allowing measurements of mitochondrial function from small blood samples (Stier et al., 2017, 2019). Mitochondrial function of blood cells correlates with that of other tissues for birds (e.g., Stier et al., 2017) and mammals (Koch et al., 2021). Using blood rather than organs such as brain or liver allows for measurements where euthanasia is not an option, such as for repeated measurements during long-term studies, or for species of conservation concern. We, therefore, examine if high Ta during the post-natal stage impact blood mitochondrial function. Measurements of mitochondrial respiration were performed on intact blood cells between day 79 and 83 post hatch (hereafter referred as day 80), to prevent any impacts of sampling on the other measurements. Measurements were made for the same individuals as the other physiological variables, except in one instance when a bird did not survive until age 80; it was replaced with a sibling from the same brood. Within 5 min of capturing each bird in the aviary 70 μl of blood was taken from the brachial vein using heparinized capillaries and transferred into Eppendorf tubes. Blood cells were then immediately separated from the plasma by centrifuging the sample for 5 min at 3,000 rpm at 4°C. Plasma was removed from the upper fraction of the sample and the blood cells were then washed by adding 1 ml of ice cold phosphate buffered saline (PBS) and spinning at 800 rpm for 3 min at 4°C.
Before starting measurements of mitochondrial respiration, the PBS was discarded and the blood cells were mixed with 1 ml of MiR05 medium [0 5 mM Egtazic Acid (EGTA), 3 mM MgCl2, 60 mM K-lactobionate, 20 mM taurine, 10 mM KH2PO4, 20 mM Hepes, 110 mM sucrose, free fatty acid bovine serum albumin (1 g L−1), pH 7.1; Stier et al., 2017]. Blood cells were then transferred into the two chambers of an O2k high resolution respirometer (Oroboros Instruments, Innsbruck, Austria) set at 37.5°C for duplicate measurements for each bird. After equilibration for 10 min we recorded mitochondrial ROUTINE respiration, representing endogenous cellular mitochondrial O2 consumption. We then quantified mitochondrial O2 consumption associated with mitochondrial proton leak (LEAK) by injecting 1 μl of 5 mM oligomycin to inhibit ATP synthesis. Oxidative phosphorylation (OXPHOS) was calculated by subtracting LEAK from ROUTINE. We then estimated the maximum capacity of the mitochondrial electron transport system (ETS) by progressive titration with the mitochondrial uncoupler CCCP (carbonyl cyanide m-chlorophenyl hydrazine; 1 μl of 1 mM steps). Finally, we inhibited mitochondrial respiration by injecting 5 μl of antimycin A (a complex III inhibitor) to measure non-mitochondrial O2 consumption and subtract this value from each of the other parameters. We calculated two different mitochondrial flux control ratios (FCRs), namely an index of OXPHOS coupling efficiency [OxCE = 1 − (LEAK/ROUTINE)] and an index of mitochondrial reserve capacity (FCRR/ETS = ROUTINE/ETS). We evaluated the technical repeatability of mitochondrial respiration rates by calculating intra-class coefficients of correlation based on duplicate measurements (ICC, ranged from 0.63 to 0.76, all p < 0.001). To account for individual variation in blood cell density and differences in blood sample volume, we performed a Pierce BCA protein quantification assay (ThermoFisher Scientific, Waltham, MA, United States) and normalized mitochondrial respiration rates for the protein content of the sample by including this value as covariate in statistical analyses (see below).
Statistical Analysis
All analyses were performed with R version 3.5.1 for Mac (R Core Team, 2018). We used a t-test to compare Ta and Tbr of control and heated nests. For each nest, we estimated and compared growth rates (K), and asymptotic size before fledge date (A) for nestling mass using nonlinear mixed models (package nlme Pinheiro et al., 2017) following the methodology of Sofaer et al. (2013). Age and treatment were fixed effects, and nest and nestling identity were included as random effects to account for the lack of independence among siblings and for repeated measures of the same individual over time.
We tested effects of nest heating on whole-animal physiological variables by fitting linear mixed models using the packages lme4 and lmerTest (Bates et al., 2014; Kuznetsova et al., 2017). Treatment and age and their interaction were fixed factors and body mass was a covariate. For Cwet, we only examined data for nestlings at 12 and 50 days because at day 5 Tb closely approximated Ta, so calculation of Cwet was unreliable. We included random slopes for individuals nested within brood to account for repeated measures of individuals belonging to the same brood. Post-hoc pairwise comparisons between groups were made using the emmeans package (Lenth et al., 2019) which applies Tukey and Kenward-Roger degrees of freedom adjustments.
To examine the effect of nest heating on mitochondrial bioenergetics (ROUTINE, LEAK, OXPHOS, and ETS) and we used protein content and temperature treatment as fixed factors, and individual as a random slope to account for our repeated measurements. For analysis of OxCE and FCRR/ETS ratios protein content was not included as a covariate.
Results
Growth of nestlings did not significantly differ between control and heated nests (F1,700 = 0.33, p = 0.560, Figure 2), and there were no significant differences between treatment and control nests for body mass at fledging date (F1,700 = 1.53, p = 0.216, Figure 2) or at 50 days post-hatch (F1,18.8 = 0.08, p = 0.929).
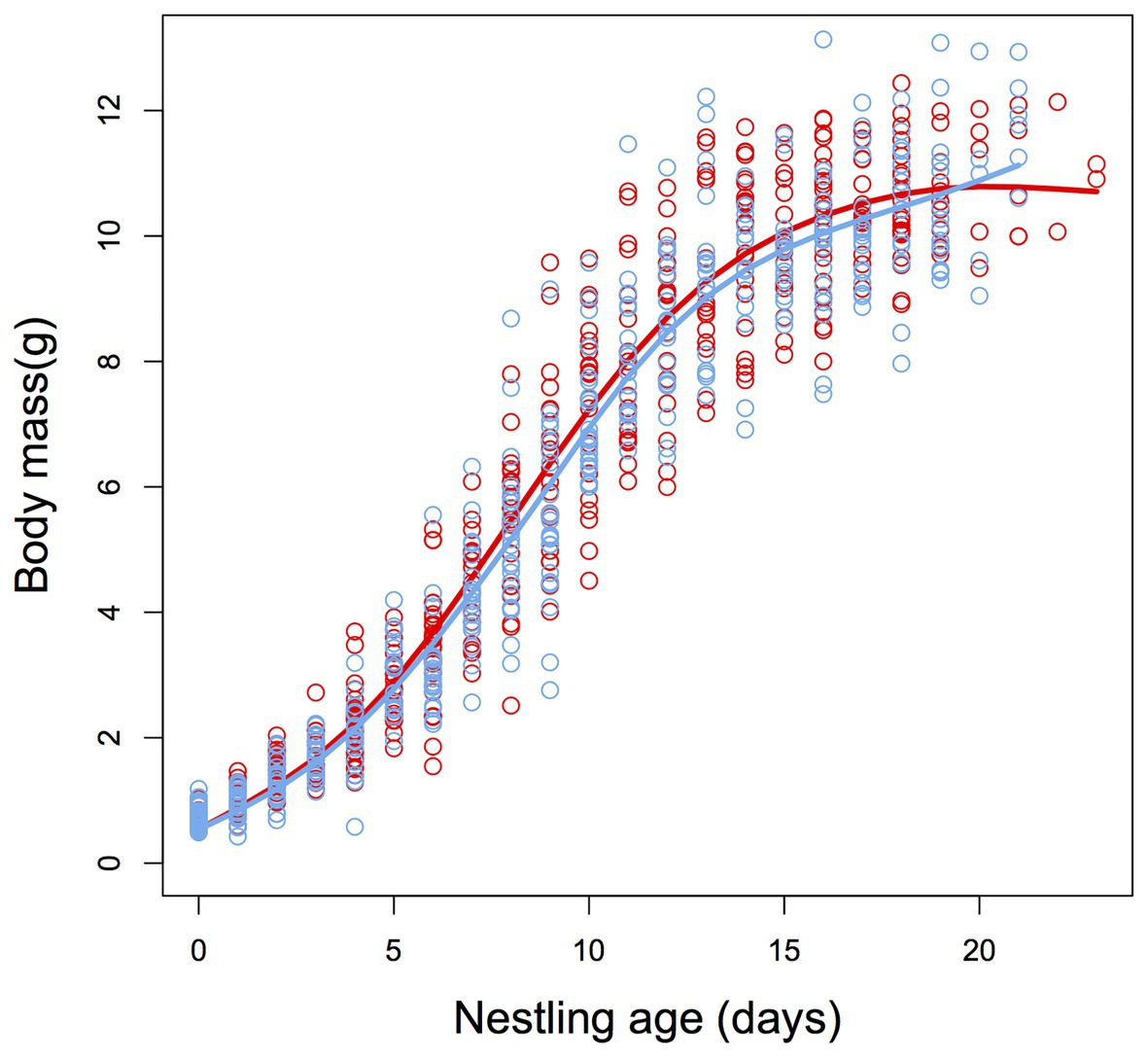
Figure 2. Sigmoidal plot describing the post-natal growth trajectories for body mass of zebra finch (T. guttata) nestlings from control (blue) and heated (red) nests. There was no significant difference in growth rate or asymptotic body mass between treatment groups (see Results section for details). N = 11 and 11.
Temperature treatment had no significant influence on MR, EWL, RWE, or Cwet (F1,62 ≤ 4.3, p ≥ 0.055; Figures 3A–D). There was a significant effect of early-life heat waves on Tb (F1,24.6 = 9.0, p = 0.006; Figure 3E) with birds from heated nests having a lower Tb than those from control nests at day 12 (p = 0.026) and 50 (p = 0.007), but not at day 5 (p = 0.82). Body mass was significantly correlated with MR (β = 2.83 ± 0.72; F1,62 = 14.8, p < 0.001) and EWL (β = 9.54 ± 3.58; F1,62 = 7.1, p = 0.010), but not with the other parameters (F1,62 ≤ 1.2, p ≥ 0.26). Metabolic rate, Tb, and RWE increased with age (F2,62 ≥ 4.9, p ≤ 0.011; Figure 3D) but Cwet decreased between day 12 and 50 (F2,62 ≥ 4.5, p < 0.042; Figure 3D) and EWL did not vary with age (F2,52.8 = 0.2, p = 0.83; Figure 3B).
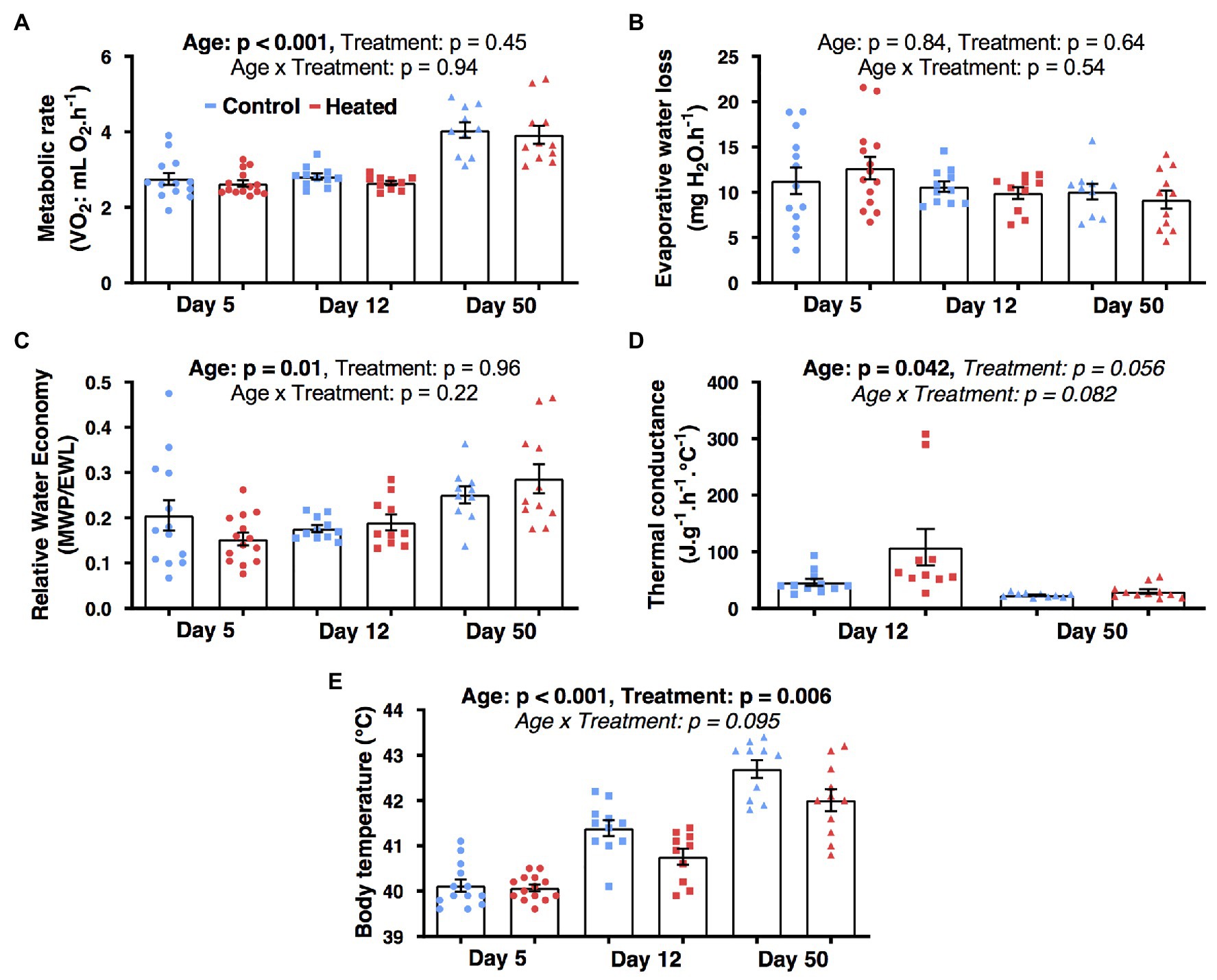
Figure 3. Effects of early-life heat waves exposure in zebra finch on whole-body physiology: (A) metabolic rate (B) evaporative water loss (C) relative water economy (D) thermal conductance, and (E) body temperature after exposure to an ambient temperature of 40°C for >2 h. Individuals have been successively measured at day 5 (ectothermic stage), day 12 (poikilothermic stage), and day 50 (homoeothermic stage; 1 month after the treatment ended) post hatch. Individual data points are presented along with their mean ± SE.
Birds from heated nests had higher mitochondrial respiration rates at day 80 than those from control nests for both ROUTINE (F1,19.6 = 4.6, p = 0.04; Figure 4) and LEAK (F1,20.0 = 8.0, p = 0.01; Figure 4). There was no significant effect of the heat treatment for OXPHOS, ETS, OxCE, or FCRROUTINE/ETS (F1,19–20 ≤ 3.5, p ≥ 0.07; Figure 4).
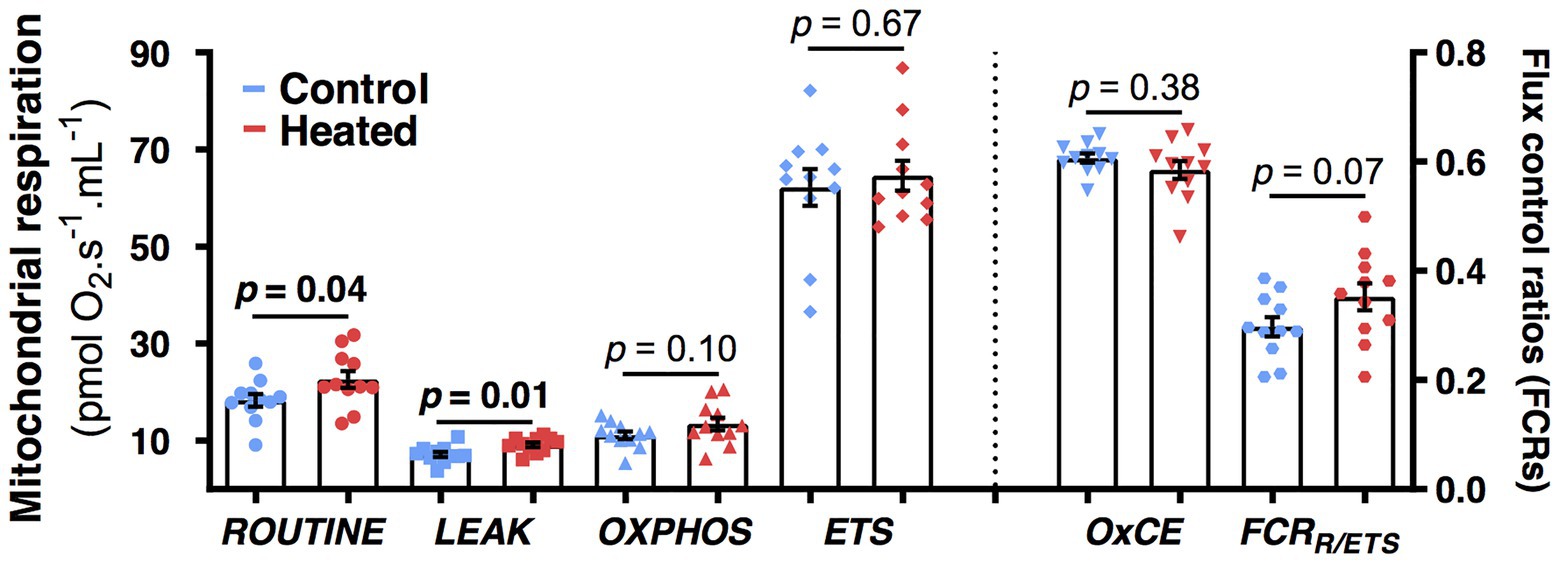
Figure 4. Effects of early-life heat waves exposure in zebra finch on blood cells mitochondrial physiology measured at day 80 (i.e., 2 months after the treatment ended). ROUTINE represents the endogenous mitochondrial respiration, LEAK the proton-leak related mitochondrial respiration, OXPHOS the ATP-synthesis related mitochondrial respiration, and ETS the maximal mitochondrial respiration. OXPHOS coupling efficiency (OxCE) is an index of mitochondrial efficiency to produce ATP and FCRR/ETS an index of the mitochondrial reserve capacity. Individual data points are presented along with their mean ± SE and describe oxygen consumption not corrected by protein content (see Materials and Methods and Results sections for details on statistics).
Discussion
For zebra finches, early-life exposure to simulated heat waves had no significant impact on whole-body metabolic rate or hygric physiology but reduced Tb of nestlings at days 12 and 50 (i.e., both during the temperature treatment and 1 month after it ended). A lower Tb allows greater scope for hyperthermia at high Ta (Tieleman and Williams, 1999), but can impose limits on muscle functionality and immune reactions (Boyles et al., 2011). Despite no whole-body metabolic effect, birds from heated nests had higher mitochondrial respiration (both endogenous and proton-leak) than birds from control nests 2 months after the heat treatment. While the consequences of these mitochondrial changes for accommodating high Ta at later life stages are unknown, our results indicate that post-natal developmental programing of mitochondrial function by exposure to elevated Ta does occur. Our results also suggest that the effects of early-life heat exposure emerge after the ectothermic stage (day 5) when endothermy develops. This information improves our understanding of species responses and potential resilience to climate change (Conradie et al., 2020).
Previous thermal acclimation and acclimatization studies for adult birds, including zebra finches, document lower MR and EWL, and higher Tb, for birds during or immediately following or chronic or acute periods of exposure to high Ta (Williams and Tieleman, 2000; Cooper et al., 2020a). These responses create a more favorable trade-off between hyperthermia and dehydration for birds exposed to high Ta. However, our findings are consistent with those for adult zebra finches (Cooper et al., 2020b), that suggest that prior acute exposure to high Ta has little effect on whole-animal metabolism and water balance at later time-points. As these physiological variables correlate with field energy and water expenditure (Nagy, 1987; Cooper et al., 2003), prior experience of high Ta is also unlikely to influence daily energy and water requirements in the field. So while there is evidence that birds adjust their physiology during periods high Ta, our results support the hypothesis that periodic extreme events such as heat waves do little to prepare birds for future warming, and are of greater concern for the persistence of avian populations (McKechnie and Wolf, 2010; Franklin et al., 2014; Cooper et al., 2020a,b).
We detected no effect of early-life exposure to increased Ta on growth rate and body mass at fledging or at 50 days of age, consistent with observations of Hsu et al. (2020) for wild pied flycatchers (Ficedula hypoleuca). Dawson et al. (2005) also found that growth rates of tree swallows (Tachycineta bicolor) from heated nests were the same as those from control nests, although birds from heated nests had higher body mass. However, other studies report lower growth rates for nestlings from heated nests (Rodríguez and Barba, 2016; Andreasson et al., 2018). Various factors, such as physiological and parental care variables, can influence the relationship between nest temperature and nestling growth rate. In colder climates, warmer minimum and mean nest temperatures are correlated with higher nestling growth rates, while in warmer climates, higher nest temperature can negatively impact nesting growth rates (Larson et al., 2015) presumably as a consequence of nest temperature moving closer to or further from an optimal temperature for growth. The absence of a temperature effect on body mass differs from previous results for wild and captive zebra finches (Andrew et al., 2017, 2018). However, thermal responses can vary depending on the ontogenetic stage (Halevy, 2020) and in these previous studies, heating occurred during the embryonic as well as the post-natal period.
There is a considerable body of literature discussing the impact on physiological and life history variables of manipulating temperature during the embryonic stage (Elphick and Shine, 1998; O’Steen, 1998; Gilchrist and Huey, 2001; Du and Ji, 2003; Ardia et al., 2010; Nord and Nilsson, 2011; DuRant et al., 2012; Scott and Johnston, 2012; Hepp et al., 2015; Andrew et al., 2017; Ton and Martin, 2017) in contrast to the scarcity of manipulative studies during the post-natal stage for altricial birds (Andreasson et al., 2020). The significant later-life whole body physiological consequences of high Ta during embryonic development compared to our observations for nestlings suggests that the embryonic phase may be more sensitive to plastic adjustments compared to the post-natal stage. This may be because embryos of all species are ectothermic with potentially greater exposure to variable temperature. However, our experimental treatment induced no whole-animal metabolic or water loss effects, even for ectothermic 5-day-old nestlings. This, together with the absence of Ta effects for body mass and growth rates compared to previous findings (Andrew et al., 2017, 2018) support the idea that conditions experienced during the post-natal stage have fewer subsequent effects for adult birds than those experienced as an embryo. This may be a consequence of the developmental and gene expression processes underlying functional differentiation of tissues during the embryonic stage (Gilbert and Epel, 2009), while the post-natal stage is characterized by cellular proliferation and growth rather than functional differentiation (Starck and Ricklefs, 1998).
We did uncover some functional consequences of early-life exposure to heat waves at the subcellular level. Two months after the end of the temperature treatment, both endogenous (ROUTINE) and proton-leak (LEAK) mitochondrial respiration rates were higher for birds in experimentally heated nests compared to control nests. It is possible that this is a consequence of birds from control and heated groups differing with respect to the thermal optimum for their mitochondrial function. High proton LEAK is functionally linked to thermogenesis in brown adipose tissue of mammals and has been associated with a fast pace of life and higher oxidative stress for birds (Jimenez et al., 2014b). A small increase in proton leak reduces reactive oxygen species (ROS) production and oxidative stress (Divakaruni and Brand, 2011; Koch et al., 2021). Acute exposure to heat generates cellular stress and increases production of ROS (Abele et al., 2002; Mujahid et al., 2005; Tan et al., 2010), which can elicit an upregulation of mitochondrial proton leak as a protective mechanism (Jarmuszkiewicz et al., 2015). Consequently, the increase we observed in LEAK for birds from heated nests may be advantageous for buffering heat-induced oxidative stress. However, higher LEAK increases metabolic heat production, which is detrimental at high Ta and it is unclear why our birds from heated nests had lower rather than higher Tb despite the elevated mitochondrial function. Future studies should examine this by measuring long-term implications for Tb, oxidative stress, and biomarkers of biological aging, such as telomere length (Dupoué et al., 2017).
A correlation between whole-animal metabolism and mitochondrial function is expected (White and Kearney, 2011; Jimenez et al., 2014a), but the interrelationships between physiological function at different levels of organization are debated (Norin and Metcalfe, 2019). The increased mitochondrial ROUTINE and LEAK, we observed at 80 days post-hatch for our heat-treated birds did not reflect an increased whole-body metabolism at early life-history stages and Tb was lower, not higher. This may be due to temporal physiological changes (we observed changes in rates of whole-body metabolism at different ages) and/or elevated Cwet for birds from heated nests which may have dissipated increased MHP. Our results suggest that elevated Cwet may have occurred but we unfortunately did not have sufficient statistical power to detect differences in Cwet for heated and control nets (Figure 3D). There may also be differences in mitochondrial contribution to whole-animal metabolism from different tissues (Else and Hulbert, 1985). Mitochondrial VO2 of muscle or liver, which have a greater contribution to whole-animal metabolism than blood (Sirsat et al., 2016b,c), may have correlated more strongly with whole-body metabolism, but this is unlikely as mitochondrial function of blood correlates with that of other tissues (Stier et al., 2017). These findings emphasize the need for improved understanding of the relationship between cellular and whole body metabolism, and of the utility of blood as a tissue for mitochondrial measurements (Koch et al., 2021).
In summary, our study provides no evidence that exposure to high nest temperature during the neonatal period has any subsequent effect on growth rate, mass, and whole-body metabolic and hygric physiology of zebra finch chicks during any of the three phases of thermoregulatory development. These results may reflect the natural history of the species (Griffith et al., 2021). Yet, there is an impact on Tb and on ROUTINE and LEAK mitochondrial function for endothermic birds, consistent with responses to oxidative damage and life-history tradeoffs (Tan et al., 2010; Hood et al., 2018a,b). This suggests that there is some limited scope for prior experience of heat waves during the neo-natal phase to result in whole organism metabolic changes. Such results force us to reconsider our approaches in exploring environmental influences on physiological traits. Indeed, failing to detect metabolic or growth consequences at the whole body level does not exclude sub-cellular repercussions that may have important implications for other unmeasured aspects of phenotypic and evolutionary fitness.
Data Availability Statement
The data used to produce the results reported in the paper are publicly available at: https://osf.io/s4hyq/files/.
Ethics Statement
The animal study was reviewed and approved by the Macquarie University and Curtin University Animal Ethics Committees (ARA 2017/024 and ARE2017-16).
Author Contributions
RT, CC, and SG designed the study and provided resources and logistical support. RT conducted the experiments and statistically analyzed the resulting data. CC and AS contributed to data collection and analyses. RT, AS, CC, and SG wrote the manuscript. All authors contributed to the article and approved the submitted version.
Funding
This research was supported by the Australian Research Council’s Discovery Project funding to CC and SG (DP170103619), and AS was supported by a Marie Sklodowska-Curie Individual Fellowship (#658085) and a Turku Collegium for Science and Medicine Fellowship.
Conflict of Interest
The authors declare that the research was conducted in the absence of any commercial or financial relationships that could be construed as a potential conflict of interest.
Acknowledgments
We thank Laura Hurly and Fabio Musanni for their conceptual and practical support during the study, and Mikaela Symes for her assistance with data entry. Macquarie Animal Research Services (M.A.R.S.) staff provided daily assistance in caring for the birds, and Philip Withers provided the custom-written data acquisition and analysis software for respirometry.
References
Abele, D., Heise, K., Pörtner, H., and Puntarulo, S. (2002). Temperature-dependence of mitochondrial function and production of reactive oxygen species in the intertidal mud clam Mya arenaria. J. Exp. Biol. 205, 1831–1841.
Andreasson, F., Nilsson, J.-Å., and Nord, A. (2020). Avian reproduction in a warming world. Front. Ecol. Evol. 8:576331. doi: 10.3389/fevo.2020.576331
Andreasson, F., Nord, A., and Nilsson, J. (2018). Experimentally increased nest temperature affects body temperature, growth and apparent survival in blue tit nestlings. J. Avian Biol. 49:jav-01620. doi: 10.1111/jav.01620
Andrew, S. C., Awasthy, M., Griffith, A. D., Nakagawa, S., and Griffith, S. C. (2018). Clinal variation in avian body size is better explained by summer maximum temperatures during development than by cold winter temperatures. Auk 135, 206–217. doi: 10.1642/AUK-17-129.1
Andrew, S., Hurley, L., Mariette, M., and Griffith, S. (2017). Higher temperatures during development reduce body size in the zebra finch in the laboratory and in the wild. J. Evol. Biol. 30, 2156–2164. doi: 10.1111/jeb.13181
Ardia, D. R., Pérez, J. H., and Clotfelter, E. D. (2010). Experimental cooling during incubation leads to reduced innate immunity and body condition in nestling tree swallows. Proc. R. Soc. B Biol. Sci. 277, 1881–1888. doi: 10.1098/rspb.2009.2138
Bates, D., Maechler, M., Bolker, B., and Walker, S. (2014). lme4: Linear Mixed-Effects Models Using Eigen and S4.R Package Version 1.1–7. Available at: http://CRAN.R-project.org/package=lme4
Boddicker, R. L., Seibert, J. T., Johnson, J. S., Pearce, S. C., Selsby, J. T., Gabler, N. K., et al. (2014). Gestational heat stress alters postnatal offspring body composition indices and metabolic parameters in pigs. PLoS One 9:e110859. doi: 10.1371/journal.pone.0110859
Boyles, J. G., Seebacher, F., Smit, B., and McKechnie, A. E. (2011). Adaptive thermoregulation in endotherms may alter responses to climate change. Integr. Comp. Biol. 51, 676–690. doi: 10.1093/icb/icr053
Cade, T. J., Tobin, C. A., and Gold, A. (1965). Water economy and metabolism of two estrildine finches. Physiol. Zool. 38, 9–33. doi: 10.1086/physzool.38.1.30152342
Calder, W. A. (1964). Gaseous metabolism and water relations of the zebra finch, Taeniopygia castanotis. Physiol. Zool. 37, 400–413. doi: 10.1086/physzool.37.4.30152758
Chung, D. J., and Schulte, P. M. (2020). Mitochondria and the thermal limits of ectotherms. J. Exp. Biol. 223:jeb227801. doi: 10.1242/jeb.227801
Conradie, S. R., Woodborne, S. M., Cunningham, S. J., and McKechnie, A. E. (2019). Chronic, sublethal effects of high temperatures will cause severe declines in southern African arid-zone birds during the 21st century. Proc. Natl. Acad. Sci. U. S. A. 116, 14065–14070. doi: 10.1073/pnas.1821312116
Conradie, S. R., Woodborne, S. M., Wolf, B. O., Pessato, A., Mariette, M. M., and McKechnie, A. E. (2020). Avian mortality risk during heat waves will increase greatly in arid Australia during the 21st century. Conserv. Physiol. 8:coaa048. doi: 10.1093/conphys/coaa048
Cooper, C. E., Hurley, L. L., Deviche, P., and Griffith, S. C. (2020a). Physiological responses of wild zebra finches (Taeniopygia guttata) to heat waves. J. Exp. Biol. 223:jeb225524. doi: 10.1242/jeb.225524
Cooper, C., Hurley, L., and Griffith, S. (2020b). Effect of acute exposure to high ambient temperature on the thermal, metabolic and hygric physiology of a small desert bird. Comp. Biochem. Physiol. Part A Mol. Integr. Physiol. 244:110684. doi: 10.1016/j.cbpa.2020.110684
Cooper, C. E., Withers, P. C., and Bradshaw, S. D. (2003). Field metabolic rate and water turnover of the numbat (Myrmecobius fasciatus). J. Comp. Physiol. B. 173, 687–693. doi: 10.1007/s00360-003-0380-6
Cooper, C. E., Withers, P., Hurley, L., and Griffith, S. C. (2019). The field metabolic rate, water turnover and feeding and drinking behavior of a small avian desert granivore. Front. Physiol. 10:1405. doi: 10.3389/fphys.2019.01405
Dawson, R. D., Lawrie, C. C., and O’Brien, E. L. (2005). The importance of microclimate variation in determining size, growth and survival of avian offspring: experimental evidence from a cavity nesting passerine. Oecologia 144, 499–507. doi: 10.1007/s00442-005-0075-7
Divakaruni, A. S., and Brand, M. D. (2011). The regulation and physiology of mitochondrial proton leak. Physiology 26, 192–205. doi: 10.1152/physiol.00046.2010
Du, W., and Ji, X. (2003). The effects of incubation thermal environments on size, locomotor performance and early growth of hatchling soft-shelled turtles, Pelodiscus sinensis. J. Therm. Biol. 28, 279–286. doi: 10.1016/S0306-4565(03)00003-2
Dupoué, A., Rutschmann, A., Le Galliard, J. F., Clobert, J., Angelier, F., Marciau, C., et al. (2017). Shorter telomeres precede population extinction in wild lizards. Sci. Rep. 7:16976. doi: 10.1038/s41598-017-17323-z
Durant, S. E., Hepp, G. R., Moore, I. T., Hopkins, B. C., and Hopkins, W. A. (2010). Slight differences in incubation temperature affect early growth and stress endocrinology of wood duck (Aix sponsa) ducklings. J. Exp. Biol. 213, 45–51. doi: 10.1242/jeb.034488
DuRant, S. E., Hopkins, W. A., Hawley, D. M., and Hepp, G. R. (2012). Incubation temperature affects multiple measures of immunocompetence in young wood ducks (Aix sponsa). Biol. Lett. 8, 108–111. doi: 10.1098/rsbl.2011.0735
DuRant, S. E., Hopkins, W. A., Hepp, G. R., and Walters, J. (2013). Ecological, evolutionary, and conservation implications of incubation temperature-dependent phenotypes in birds. Biol. Rev. 88, 499–509. doi: 10.1111/brv.12015
Elphick, M. J., and Shine, R. (1998). Longterm effects of incubation temperatures on the morphology and locomotor performance of hatchling lizards (Bassiana duperreyi, Scincidae). Biol. J. Linn. Soc. 63, 429–447. doi: 10.1111/j.1095-8312.1998.tb01527.x
Else, P. L., and Hulbert, A. J. (1985). Mammals: an allometric study of metabolism at tissue and mitochondrial level. Am. J. Phys. 248, R415–R421. doi: 10.1152/ajpregu.1985.248.4.R415
Finlayson, H. (1932). Heat in the interior of South Australia-holocaust of bird-life. S. Aust. Ornithol. 11, 158–160.
Franklin, D. C., Ehmke, G., Van Der Wal, J., and Garnett, S. T. (2014). “The Exposure of Australian Birds to Climate Change,” in Climate Change Adaptation Plan for Australian Birds. Collingwood, VIC: CSIRO Publishing, 7.
Fuller, A., Dawson, T., Helmuth, B., Hetem, R. S., Mitchell, D., and Maloney, S. K. (2010). Physiological mechanisms in coping with climate change. Physiol. Biochem. Zool. 83, 713–720. doi: 10.1086/652242
Gilbert, S. F., and Epel, D. (2009). Ecological Developmental Biology: Integrating Epigenetics, Medicine, and Evolution. Sunderland: Sinauer Associates.
Gilchrist, G. W., and Huey, R. B. (2001). Parental and developmental temperature effects on the thermal dependence of fitness in Drosophila melanogaster. Evolution 55, 209–214. doi: 10.1111/j.0014-3820.2001.tb01287.x
Griffith, S. C., Mainwaring, M. C., Sorato, E., and Beckmann, C. (2016). High atmospheric temperatures and ‘ambient incubation’ drive embryonic development and lead to earlier hatching in a passerine bird. R. Soc. Open Sci. 3:150371. doi: 10.1098/rsos.150371
Griffith, S. C., Ton, R., Hurley, L. L., McDiarmid, C., and Pacheco-Fuentes, H. (2021). The ecology of the Zebra finch makes it a great laboratory model but an outlier amongst passerine birds. Birds. doi: 10.3390/birds2010004, (in press).
Gyllenhammer, L. E., Entringer, S., Buss, C., and Wadhwa, P. D. (2020). Developmental programming of mitochondrial biology: a conceptual framework and review. Proc. R. Soc. B Biol. Sci. 287:20192713. doi: 10.1098/rspb.2019.2713
Halevy, O. (2020). Timing is everything—the high sensitivity of avian satellite cells to thermal conditions during embryonic and posthatch periods. Front. Physiol. 11:235. doi: 10.3389/fphys.2020.00235
Harada, A. E., Healy, T. M., and Burton, R. S. (2019). Variation in thermal tolerance and its relationship to mitochondrial function across populations of Tigriopus californicus. Front. Physiol. 10:213. doi: 10.3389/fphys.2019.00213
Hepp, G., DuRant, S., and Hopkins, W. (2015). “Influence of Incubation Temperature on Offspring Phenotype and Fitness in Birds,” in Nests, Eggs and Incubation: New Ideas About Avian Reproduction. Oxford: Oxford University Press, 171–178.
Hood, W. R., Austad, S. N., Bize, P., Jimenez, A. G., Montooth, K. L., Schulte, P. M., et al. (2018a). The mitochondrial contribution to animal performance, adaptation, and life-history variation. Integr. Comp. Biol. 58, 480–485. doi: 10.1093/icb/icy089
Hood, W., Zhang, Y., Mowry, A., Hyatt, H., and Kavazis, A. (2018b). Life history trade-offs within the context of mitochondrial hormesis. Integr. Comp. Biol. 58, 567–577. doi: 10.1093/icb/icy073
Hsu, B., Sarraude, T., Cossin-Sevrin, N., Crombecque, M., Stier, A., and Ruuskanen, S. (2020). Testing for context-dependent effects of maternal thyroid hormones on offspring survival and physiology: an experimental approach manipulating temperature in a wild bird species. Sci. Rep. 10, 1–8. doi: 10.1101/2020.05.08.083725
Huang, C., Jiao, H., Song, Z., Zhao, J., Wang, X., and Lin, H. (2015). Heat stress impairs mitochondria functions and induces oxidative injury in broiler chickens. J. Anim. Sci. 93, 2144–2153. doi: 10.2527/jas.2014-8739
Jarmuszkiewicz, W., Woyda-Ploszczyca, A., Koziel, A., Majerczak, J., and Zoladz, J. A. (2015). Temperature controls oxidative phosphorylation and reactive oxygen species production through uncoupling in rat skeletal muscle mitochondria. Free Radic. Biol. Med. 83, 12–20. doi: 10.1016/j.freeradbiomed.2015.02.012
Jimenez, A. G., Cooper-Mullin, C., Calhoon, E. A., and Williams, J. B. (2014a). Physiological underpinnings associated with differences in pace of life and metabolic rate in north temperate and neotropical birds. J. Comp. Physiol. B. 184, 545–561. doi: 10.1007/s00360-014-0825-0
Jimenez, A. G., Van Brocklyn, J., Wortman, M., and Williams, J. B. (2014b). Cellular metabolic rate is influenced by life-history traits in tropical and temperate birds. PLoS One 9:e87349. doi: 10.1371/journal.pone.0087349
Jonsson, B., and Jonsson, N. (2014). Early environment influences later performance in fishes. J. Fish Biol. 85, 151–188. doi: 10.1111/jfb.12432
Koch, R. E., Buchanan, K. L., Casagrande, S., Crino, O., Dowling, D. K., Hill, G. E., et al. (2021). Integrating mitochondrial aerobic metabolism into ecology and evolution. Trends Ecol. Evol. 36, 321–332. doi: 10.1016/j.tree.2020.12.006
Kuznetsova, A., Brockhoff, P. B., and Christensen, R. H. (2017). lmerTest package: tests in linear mixed effects models. J. Stat. Softw. 82, 1–26. doi: 10.18637/jss.v082.i13
Larson, E. R., Eastwood, J. R., Buchanan, K. L., Bennett, A. T., and Berg, M. L. (2015). How does nest box temperature affect nestling growth rate and breeding success in a parrot? Emu 115, 247–255. doi: 10.1071/MU14081
Lenth, R., Singmann, H., Love, J., Buerkner, P., and Herve, M. (2019). Estimated Marginal Means, Aka Least-Squares Means. R Package Version 1.2. Available at: https://CRAN.R-project.org/package=emmeans
Lindström, J. (1999). Early development and fitness in birds and mammals. Trends Ecol. Evol. 14, 343–348. doi: 10.1016/S0169-5347(99)01639-0
McKechnie, A. E., Hockey, P. A., and Wolf, B. O. (2012). Feeling the heat: Australian landbirds and climate change. Emu 112, i–vii. doi: 10.1071/muv112n2_ed
McKechnie, A. E., and Wolf, B. O. (2010). Climate change increases the likelihood of catastrophic avian mortality events during extreme heat waves. Biol. Lett. 6, 253–256. doi: 10.1098/rsbl.2009.0702
McWhorter, T. J., Gerson, A. R., Talbot, W. A., Smith, E. K., McKechnie, A. E., and Wolf, B. O. (2018). Avian thermoregulation in the heat: evaporative cooling capacity and thermal tolerance in two Australian parrots. J. Exp. Biol. 221:jeb168930. doi: 10.1242/jeb.168930
Meehl, G. A., and Tebaldi, C. (2004). More intense, more frequent, and longer lasting heat waves in the 21st century. Science 305, 994–997. doi: 10.1126/science.1098704
Monaghan, P. (2008). Early growth conditions, phenotypic development and environmental change. Philos. Trans. R. Soc. Lond. Ser. B Biol. Sci. 363, 1635–1645. doi: 10.1098/rstb.2007.0011
Moraes, V., Malheiros, R., Bruggeman, V., Collin, A., Tona, K., Van As, P., et al. (2003). Effect of thermal conditioning during embryonic development on aspects of physiological responses of broilers to heat stress. J. Therm. Biol. 28, 133–140. doi: 10.1016/S0306-4565(02)00049-9
Mujahid, A., Pumford, N. R., Bottje, W., Nakagawa, K., Miyazawa, T., Akiba, Y., et al. (2007). Mitochondrial oxidative damage in chicken skeletal muscle induced by acute heat stress. J. Poult. Sci. 44, 439–445. doi: 10.2141/jpsa.44.439
Mujahid, A., Yoshiki, Y., Akiba, Y., and Toyomizu, M. (2005). Superoxide radical production in chicken skeletal muscle induced by acute heat stress. Poult. Sci. 84, 307–314. doi: 10.1093/ps/84.2.307
Nagy, K. A. (1987). Field metabolic rate and food requirement scaling in mammals and birds. Ecol. Monogr. 57, 111–128. doi: 10.2307/1942620
Noakes, M. J., Wolf, B. O., and McKechnie, A. E. (2016). Seasonal and geographical variation in heat tolerance and evaporative cooling capacity in a passerine bird. J. Exp. Biol. 219, 859–869. doi: 10.1242/jeb.132001
Nord, A., and Giroud, S. (2020). Lifelong effects of thermal challenges during development in birds and mammals. Front. Physiol. 11:419. doi: 10.3389/fphys.2020.00419
Nord, A., and Nilsson, J. (2011). Incubation temperature affects growth and energy metabolism in blue tit nestlings. Am. Nat. 178, 639–651. doi: 10.1086/662172
Norin, T., and Metcalfe, N. B. (2019). Ecological and evolutionary consequences of metabolic rate plasticity in response to environmental change. Philos. Trans. R. Soc. B 374:20180180. doi: 10.1098/rstb.2018.0180
O’Steen, S. (1998). Embryonic temperature influences juvenile temperature choice and growth rate in snapping turtles Chelydra serpentina. J. Exp. Biol. 201, 439–449.
O’Steen, S., and Janzen, F. J. (1999). Embryonic temperature affects metabolic compensation and thyroid hormones in hatchling snapping turtles. Physiol. Biochem. Zool. 72, 520–533. doi: 10.1086/316690
Pachauri, R. K., Allen, M. R., Barros, V. R., Broome, J., Cramer, W., Christ, R., et al. (2014). Climate Change 2014: Synthesis Report. Contribution of Working Groups I, II and III to the Fifth Assessment Report of the Intergovernmental Panel on Climate Change. IPCC.
Page, A. J., Cooper, C. E., and Withers, P. C. (2011). Effects of experiment start time and duration on measurement of standard physiological variables. J. Comp. Physiol. B. 181, 657–665. doi: 10.1007/s00360-011-0551-9
Paital, B., and Chainy, G. (2014). Effects of temperature on complexes I and II mediated respiration, ROS generation and oxidative stress status in isolated gill mitochondria of the mud crab Scylla serrata. J. Therm. Biol. 41, 104–111. doi: 10.1016/j.jtherbio.2014.02.013
Pinheiro, J., Bates, D., DebRoy, S., Sarkar, D., Heisterkamp, S., Van Willigen, B., et al. (2017). Package ‘nlme’. Linear and Nonlinear Mixed Effects Models, Version 3.
Pollock, H. S., Brawn, J. D., and Cheviron, Z. A. (2021). Heat tolerances of temperate and tropical birds and their implications for susceptibility to climate warming. Funct. Ecol. 35, 93–104. doi: 10.1111/1365-2435.13693
Pörtner, H. (2001). Climate change and temperature-dependent biogeography: oxygen limitation of thermal tolerance in animals. Naturwissenschaften 88, 137–146. doi: 10.1007/s001140100216
Price, E. R., and Dzialowski, E. M. (2018). Development of endothermy in birds: patterns and mechanisms. J. Comp. Physiol. B. 188, 373–391. doi: 10.1007/s00360-017-1135-0
Price, E. R., Sirsat, T. S., Sirsat, S. K., Kang, G., Keereetaweep, J., Aziz, M., et al. (2017). Thermal acclimation in American alligators: effects of temperature regime on growth rate, mitochondrial function, and membrane composition. J. Therm. Biol. 68, 45–54. doi: 10.1016/j.jtherbio.2016.06.016
R Core Team (2018). R: A Language and Environment for Statistical Computing. Vienna, Austria: R Foundation for Statistical Computing.
Remeŝ, V., and Martin, T. E. (2002). Environmental influences on the evolution of growth and developmental rates in passerines. Evolution 56, 2505–2518. doi: 10.1111/j.0014-3820.2002.tb00175.x
Ricklefs, R. E. (1968). Patterns of growth in birds. Ibis 110, 419–451. doi: 10.1111/j.1474-919x.1968.tb00058.x
Rodríguez, S., and Barba, E. (2016). Nestling growth is impaired by heat stress: an experimental study in a Mediterranean great tit population. Zool. Stud. 55:40. doi: 10.6620/ZS.2016.55-40
Sauve, D., Friesen, V. L., and Charmantier, A. (2021). The effects of weather on avian growth and implications for adaptation to climate change. Front. Ecol. Evol. 9:569741. doi: 10.3389/fevo.2021.569741
Schnurr, M. E., Yin, Y., and Scott, G. R. (2014). Temperature during embryonic development has persistent effects on metabolic enzymes in the muscle of zebrafish. J. Exp. Biol. 217, 1370–1380. doi: 10.1242/jeb.094037
Scott, G. R., and Johnston, I. A. (2012). Temperature during embryonic development has persistent effects on thermal acclimation capacity in zebrafish. Proc. Natl. Acad. Sci. U. S. A. 109, 14247–14252. doi: 10.1073/pnas.1205012109
Sirsat, S. K. G., Sirsat, T. S., Crossley, J. L., Sotherland, P. R., and Dzialowski, E. M. (2016a). The 12-day thermoregulatory metamorphosis of red-winged blackbirds (Agelaius phoeniceus). J. Comp. Physiol. B. 186, 651–663. doi: 10.1007/s00360-016-0978-0
Sirsat, S. K., Sirsat, T. S., Faber, A., Duquaine, A., Winnick, S., Sotherland, P. R., et al. (2016b). Development of endothermy and concomitant increases in cardiac and skeletal muscle mitochondrial respiration in the precocial Pekin duck (Anas platyrhynchos domestica). J. Exp. Biol. 219, 1214–1223. doi: 10.1242/jeb.132282
Sirsat, S. K., Sirsat, T. S., Price, E. R., and Dzialowski, E. M. (2016c). Post-hatching development of mitochondrial function, organ mass and metabolic rate in two ectotherms, the American alligator (Alligator mississippiensis) and the common snapping turtle (Chelydra serpentina). Biol. Open 5, 443–451. doi: 10.1242/bio.017160
Sofaer, H. R., Chapman, P. L., Sillett, T. S., and Ghalambor, C. K. (2013). Advantages of nonlinear mixed models for fitting avian growth curves. J. Avian Biol. 44, 469–478. doi: 10.1111/j.1600-048x.2013.05719.x
Starck, J. M., and Ricklefs, R. E. (1998). Avian Growth and Development: Evolution Within the Altricial-Precocial Spectrum. Oxford: Oxford university press.
Stier, A., Bize, P., Hsu, B. Y., and Ruuskanen, S. (2019). Plastic but repeatable: rapid adjustments of mitochondrial function and density during reproduction in a wild bird species. Biol. Lett. 15:20190536. doi: 10.1098/rsbl.2019.0536
Stier, A., Bize, P., Schull, Q., Zoll, J., Singh, F., Geny, B., et al. (2013). Avian erythrocytes have functional mitochondria, opening novel perspectives for birds as animal models in the study of ageing. Front. Zool. 10:33. doi: 10.1186/1742-9994-10-33
Stier, A., Metcalfe, N. B., and Monaghan, P. (2020). Pace and stability of embryonic development affect telomere dynamics: an experimental study in a precocial bird model. Proc. R. Soc. B 287:20201378. doi: 10.1098/rspb.2020.1378
Stier, A., Romestaing, C., Schull, Q., Lefol, E., Robin, J., Roussel, D., et al. (2017). How to measure mitochondrial function in birds using red blood cells: a case study in the king penguin and perspectives in ecology and evolution. Methods Ecol. Evol. 8, 1172–1182. doi: 10.1111/2041-210X.12724
Tan, G., Yang, L., Fu, Y., Feng, J., and Zhang, M. (2010). Effects of different acute high ambient temperatures on function of hepatic mitochondrial respiration, antioxidative enzymes, and oxidative injury in broiler chickens. Poult. Sci. 89, 115–122. doi: 10.3382/ps.2009-00318
Tattersall, G. J., Sinclair, B. J., Withers, P. C., Fields, P. A., Seebacher, F., Cooper, C. E., et al. (2012). Coping with thermal challenges: physiological adaptations to environmental temperatures. Compr. Physiol. 2, 2151–2202. doi: 10.1002/cphy.c110055
Tebaldi, C., Hayhoe, K., Arblaster, J. M., and Meehl, G. A. (2006). Going to the extremes. Clim. Chang. 79, 185–211. doi: 10.1007/s10584-006-9051-4
Tieleman, B. I., and Williams, J. B. (1999). The role of hyperthermia in the water economy of desert birds. Physiol. Biochem. Zool. 75, 87–100. doi: 10.1086/316640
Tieleman, B. I., Williams, J. B., and Buschur, M. E. (2002). Physiological adjustments to arid and Mesic environments in larks (Alaudidae). Physiol. Biochem. Zool. 75, 305–313. doi: 10.1086/341998
Tieleman, B. I., Williams, J. B., Buschur, M. E., and Brown, C. R. (2003). Phenotypic variation of larks along an aridity gradient: are desert birds more flexible? Ecology 84, 1800–1815. doi: 10.1890/0012-9658(2003)084[1800:PVOLAA]2.0.CO;2
Ton, R., and Martin, T. E. (2017). Proximate effects of temperature versus evolved intrinsic constraints for embryonic development times among temperate and tropical songbirds. Sci. Rep. 7:895. doi: 10.1038/s41598-017-00885-3
Tracy, R. L., and Walsberg, G. E. (2001). Developmental and acclimatory contributions to water loss in a desert rodent: investigating the time course of adaptive change. J. Comp. Physiol. B. 171, 669–679. doi: 10.1007/s003600100218
Webb, D. (1987). Thermal tolerance of avian embryos: a review. Condor 89, 874–898. doi: 10.2307/1368537
White, C. R., and Kearney, M. R. (2011). Metabolic scaling in animals: methods, empirical results, and theoretical explanations. Compr. Physiol. 4, 231–256. doi: 10.1002/cphy.c110049
Williams, J. B., and Tieleman, B. I. (2000). Flexibility in basal metabolic rate and evaporative water loss among hoopoe larks exposed to different environmental temperatures. J. Exp. Biol. 203, 3153–3159.
Withers, P. C. (2001). Design, calibration and calculation for flow-through respirometry systems. Aust. J. Zool. 4, 445–461. doi: 10.1071/zo00057
Withers, P. C., Cooper, C. E., Maloney, S. K., Bozinovic, F., and Cruz-Neto, A. P. (2016). Ecological and Environmental Physiology of Mammals. Oxford, U.K.: Oxford University Press.
Keywords: birds (Australian terrestrial), developmental plasticity, metabolic rate, mitochondria, Taeniopygia guttata castanotis (Aves, Passeriformes), water loss
Citation: Ton R, Stier A, Cooper CE and Griffith SC (2021) Effects of Heat Waves During Post-natal Development on Mitochondrial and Whole Body Physiology: An Experimental Study in Zebra Finches. Front. Physiol. 12:661670. doi: 10.3389/fphys.2021.661670
Edited by:
Walter Gay Bottje, University of Arkansas, United StatesReviewed by:
Nima Khodambashi Emami, University of Arkansas, United StatesAndreas Nord, Lund University, Sweden
Copyright © 2021 Ton, Stier, Cooper and Griffith. This is an open-access article distributed under the terms of the Creative Commons Attribution License (CC BY). The use, distribution or reproduction in other forums is permitted, provided the original author(s) and the copyright owner(s) are credited and that the original publication in this journal is cited, in accordance with accepted academic practice. No use, distribution or reproduction is permitted which does not comply with these terms.
*Correspondence: Riccardo Ton, cmljY2FyZG8udG9uQG1zby51bXQuZWR1