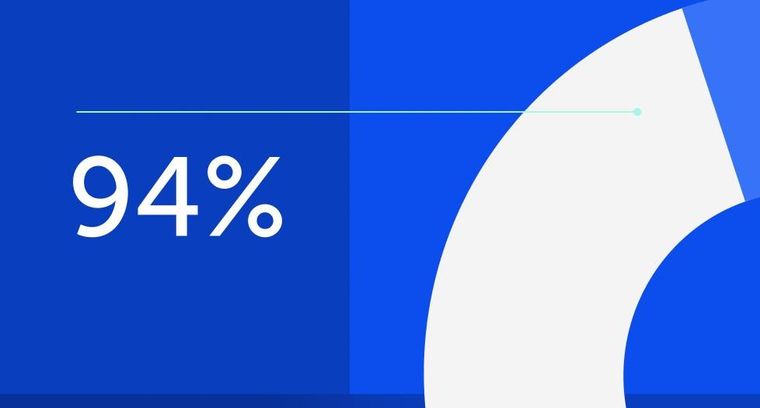
94% of researchers rate our articles as excellent or good
Learn more about the work of our research integrity team to safeguard the quality of each article we publish.
Find out more
REVIEW article
Front. Physiol., 15 March 2021
Sec. Red Blood Cell Physiology
Volume 12 - 2021 | https://doi.org/10.3389/fphys.2021.655393
Normal human red blood cells have an average life span of about 120 days in the circulation after which they are engulfed by macrophages. This is an extremely efficient process as macrophages phagocytose about 5 million erythrocytes every second without any significant release of hemoglobin in the circulation. Despite large number of investigations, the precise molecular mechanism by which macrophages recognize senescent red blood cells for clearance remains elusive. Red cells undergo several physicochemical changes as they age in the circulation. Several of these changes have been proposed as a recognition tag for macrophages. Most prevalent hypotheses for red cell clearance mechanism(s) are expression of neoantigens on red cell surface, exposure phosphatidylserine and decreased deformability. While there is some correlation between these changes with aging their causal role for red cell clearance has not been established. Despite plethora of investigations, we still have incomplete understanding of the molecular details of red cell clearance. In this review, we have reviewed the recent data on clearance of senescent red cells. We anticipate recent progresses in in vivo red cell labeling and the explosion of modern proteomic techniques will, in near future, facilitate our understanding of red cell senescence and their destruction.
Under normal physiological condition, red cell concentration is maintained at a relatively constant value of ∼5 million per μL (4.52–5.90 in men and 4.10–5.10 in women) by equilibration of production and destruction. Red cells are produced when hematopoietic stem cells differentiate into erythroid precursors that subsequently mature into circulating erythrocytes. While the mechanisms involved in erythropoiesis are understood in detail, the processes that underlie physiological clearance of senescent red cells from the circulation are incompletely understood. Red cells have an average life span of about 120 days after which they are cleared by- phagocytosis by reticuloendothelial macrophages due to accumulated changes during their life span. Approximately 5 million erythrocytes (the average number per μl) are removed from the circulation every second.
During circulation, blood cells are exposed to constant physical and chemical stress. The static erythrocyte is a biconcave disk, 8–10 μm in diameter, 2.5 μm thick at the edge, and 1 μm thick at the center. This shape is well suited for gas transport, providing optimal surface area for oxygen and CO2 diffusion. However, red cells must pass innumerable times during their life span without rupturing through capillaries with diameters of approximately 2–4 μm. Physical stress encountered by red cells is especially severe when they traverse interendothelial slits of ∼ 2 μm in the venous sinus of the splenic red pulp. The remarkable pliability of the membrane allows to the red cell to undergo a complex transition from the biconcave shape to a parachute-like configuration through membrane folding and cytoplasmic reorganization during its flow in the microcirculation (Tomaiuolo and Guido, 2011). Following the completion of its journey through the microcirculation the red cells regain its efficient biconcave disk shape, however, repeated physical stress encountered in the microcirculation results in accumulated loss of deformability that adversely affect its life span (Carrell et al., 1977; Rifkind et al., 2019).
During oxygen delivery, a small fraction of the oxygen released from hemoglobin generates highly destructive reactive oxygen species (ROS) including superoxide. ROS oxidize hydroxylated sulfur groups (SH), leading to alterations in protein structure. One of the consequences of this process is transformation of the hemoglobin into to an insoluble product that precipitates as Heinz bodies. ROS also damages proteins in the red cells membrane, leading to greater membrane rigidity that further contributes to decreased red cells survival. In addition, ROS oxidizes ferrous iron (Fe2+) to ferric iron (Fe3+), converting hemoglobin to methemoglobin, which cannot bind oxygen. The red cells have robust redox mechanisms to quench the ROS and protect the hemoglobin and other components including membrane proteins, lipids, and cytoplasmic components from injury. To repair this damage and to allow oxygen to bind to hemoglobin, red cells metabolism must generate reduced nicotinamide adenine dinucleotide phosphate (NADPH) for antioxidant protection mediated principally by glucose-6-phosphate dehydrogenase (G6PD) activity. G6PD-deficient red cells have shorter in vivo survival (Sagiv et al., 2018). Red cells also generate nicotinamide adenine dinucleotide (NADH) that must be available to continuously reduce methemoglobin to hemoglobin. Glycolysis generates ATP to fuel red cell membrane integrity and transport pathways, or alternatively generation of ATP is bypassed (“energy shunt”) to form 2,3 DPG that enhances tissue oxygen delivery in the Rapoport Luebering pathway (Carrell et al., 1977; Rifkind et al., 2019). Several studies, based separation on density gradients, have shown as the cells age in the circulation the activity of several glycolytic enzymes decrease (Haram et al., 1991). The age-dependent decay of red cell enzymes may predispose to greater oxidant injury in senescent red cells. Several of these changes have been studied as tags for macrophage recognition and clearance of senescent red cells, including loss of membrane resulting in impaired deformability, oxidation of membrane proteins, appearance of neoantigens, exposure of membrane phosphatidylserine, changes in enzymatic activity, and decrease in the activity of the thrombospondin-1 receptor (CD47). Despite extensive study, the mechanisms that mediate senescent red cells clearance remain largely speculative (Piomelli and Seaman, 1993; Rifkind and Nagababu, 2013; Lew and Tiffert, 2017; Badior and Casey, 2018).
The spleen is a lymphoid organ that functions primarily as a filter for the blood (Mebius and Kraal, 2005). The structure of the splenic microcirculation is optimized to remove defective red cells, blood-borne microorganisms, and cellular debris. Splenic blood flow consists of both open and closed sites of circulation. Splenic arterioles empty into a reticular meshwork called the red pulp that is rich in macrophages and not lined by endothelial cells. Consequently, erythrocytes transit the red pulp slowly under low shear stress to reach the splenic sinuses. The high density of macrophages in the red pulp results in contact with the red cells. Macrophages recognize damaged, deformed and senescent erythrocytes and remove them from circulation by phagocytosis. In addition, without damaging the red cell, macrophages remodel the cell by removing nuclear remnants (Howell-Jolly bodies) and other inclusions such as Heinz bodies (denatured hemoglobin caused by oxidative damage), Pappenheimer bodies (phagosomes containing excess iron), and siderocytes (iron granules that are not contained within hemoglobin). Endothelial cells of the splenic sinuses are separated by interendothelial fenestrations ∼2 μM in diameter that are lined by basement membrane. The pliability of the membrane allows the red cells to traverse these tight spaces to reach the splenic sinuses. Erythrophagocytosis of senescent red cells readily occurs in vivo but incubation of senescent cells in vitro with cultured splenic macrophages does not induce erythrophagocytosis suggesting that the characteristics of splenic architecture are necessary for clearance of aged red cells (Gottlieb et al., 2012). How precisely the spleen senses senescent red cells is not clear. Altered deformability may not be the principal mechanism. Southeast Asian ovalocytosis or Melanesian ovalocytosis is an inherited disorder characterized by abnormal red cell membrane rigidity due to deletion of nine amino acids within the cytoplasmic domain of the most abundant red cell cytoskeleton protein, Band 3 [anion exchanger 1 (AE1), encoded by SLC4A1)] (Hadley et al., 1983; Saul et al., 1984). Despite decreased membrane deformability, these ovalocytes circulate normally without abnormal sequestration by the spleen (Safeukui et al., 2018). Klei et al. (2020) reported that in vivo, in the splenic microcirculation, senescent red cells adhere to splenic macrophages via laminin-α5. Consequently, some of the red cells undergo hemolysis. In this setting, red pulp macrophages preferentially engulf erythrocyte ghosts over intact red cells. The authors hypothesized that phagocytized red cell ghost are more rapidly degraded than their intact counterparts, and that scavenging heme from within the splenic circulation is more efficient for iron recycling than extraction from phagolysosomes. However, this hypothesis is not supported by the observation that splenectomy does not affect red cell lifespan or iron metabolism (Anosa, 1976). As the red cells age in the circulation, they lose hemoglobin and membrane. This loss occurs due to shedding of hemoglobin-containing microvesicles – a process facilitated by the pitting action spleen (Willekens et al., 2003). While the spleen is essential for remodeling of erythrocytes and clearing of defective red cells, more needs to be learned about splenic contribution to physiological clearance of senescent erythrocytes. It is very likely there are other sites for senescent red blood cell clearance.
A major limitation in the study of the processing of senescent cells is the absence of a reliable technique to isolate the target cells just before clearance. A variety of techniques have used including separation based on density (Borun et al., 1957; Cohen et al., 1976), differential agglutination (Allison and Burn, 1955), osmotic fragility (Marks and Johnson, 1958), induction of bone marrow arrest (Reissmann and Ito, 1966; Van Dilla and Spalding, 1967), and isolation of red cells from patients with acute aplastic anemia (Linderkamp et al., 1993). The most commonly used method of separation is based on density. When a cohort of red cells, labeled with radioactive iron (53Fe), were centrifuged, radioactivity was detected primarily in the low-density red cells concentrated near the top of a centrifuged cell sample. Over time, radioactivity progressively shifted toward higher density fractions at the bottom of centrifuged sample. The conclusion from these studies was that red cells become progressively denser as they age within the circulation and that density-dependent cell separation methods can be used to isolate cell populations based on age related density. Other studies showed putatively older cells separated based on density had decreased sodium efflux (Bernstein, 1959; Seaman et al., 1980; Hentschel et al., 1986), resulting in progressive dehydration during the red cell life span. However, concerns have been raised about using density as the sole criterion for red cell aging. Studies have shown that labeled cohorts of human red cells do not increase in density uniformly throughout their life-span. Rather, during the latter half of the life-span of the cohort, density becomes diffusely distributed (Bernstein, 1959) with only a slight increase in dense cells. The Ganzoni hypertransfusion protocol suppresses endogenous erythropoiesis by maintaining polycythemia by transfusion in mice or rats such that progressively older cells can be obtained for analysis (Ganzoni et al., 1971). Results from using this model showed that older red cells are not denser despite their shorter life span following autologous transfusion (Mueller et al., 1987). Other concerns about density fractionation such as low-resolution of gradient separations and the confounding effects of reticulocytes in the less dense fractions have been raised (Clark, 1988). Furthermore, some senescent cells can undergo terminal density reversal shortly before clearance by poorly understood mechanisms further complicating the correlation between cell density and senescence (Lew and Tiffert, 2013). Nevertheless, a studies continue to be, and have been performed, based on red cells density gradient separation.
Clinical studies have also given conflicting information. A human study showed that the denser fractions of 56Cr labeled cells disappeared more quickly than red cell in lighter fractions (ten Brinke and de Regt, 1970). However, Linderkamp et al. (1993) examined the red cells of children with transient erythroblastopenia of childhood, a disorder causing temporary cessation of erythropoiesis. The red cells from these patients exhibited no changes in density and only a small fraction of deformable red cells was diminished. The tag for macrophage removal of senescent red cells appears to behave in a binary fashion, becoming expressed at the time when the cell reaches the end of its life span.
An alternative method to identify senescent red cells based on biotinylation of cell surface proteins have been developed by Dale et al. Dale and Norenberg (1990) and Waugh et al. (1992). Red cells are labeled ex vivo and infused into animals or labeled by intravenous injection of biotin in vivo. At various time intervals, the biotinylated red cells can be isolated using an avidin matrix. Studies of biotinylated rabbit red cells showed that old cells had both decreased surface area (10.5%) and volume (8.4%) resulting in little change in surface-to-volume ratio during aging. The aged cells were found to have normal membrane elasticity with only a minority of the cells being recovered from denser fractions following centrifugation. A rapid and robust method compliant with good practice guidelines was developed to generate biotin-labeled red cells for clinical research (de Back et al., 2018). Furthermore, a stable isotope-based mass spectrometric method using non-radioactive 15N-glycine for cohort labeling has been developed and it do not involve membrane modification or radioactivity (Browne et al., 1993). This method show good correlation with the biotin method (Khera et al., 2015). We expect that application of these procedures will advance our understanding of the molecular basis of red cell aging in humans by providing a uniform approach to analysis of erythrocyte physiology and pathophysiology.
Band 3 (SLC4A1) is the most abundant integral membrane protein in the red cell with more than a million copies per cell. It is a 911-amino acid glycoprotein and the founding member of the anion exchanger gene family (AE-1). In the membrane, Band 3 associates with a number of other membrane proteins including the Rh complex, glycophorins, and CD47. On the cytoplasmic side, it attaches to the membrane cytoskeleton through interactions with Band 4.2 and ankyrin, and this complex is a component of the cytoskeleton that is required to maintain the shape and integrity of red cells. In addition, its carboxyl terminus is attached to carbonic anhydrase, which catalyzes the conversion of CO2 to HCO3− and H+(Vince and Reithmeier, 1998). Band 3 mediates the electroneutral exchange of bicarbonate for plasma chloride allowing CO2 to be transported as HCO3− in the plasma. This process is reversed in the lungs. The crystal structure of transmembrane domain of Band 3 has been solved, providing insights into its functional interactions (Hatae et al., 2018). Deoxyhemoglobin binds to Band 3 and this interaction is implicated in sensing changes in oxygen tension and controlling red cell deformability thereby capillary velocity during hypoxia (Zhou et al., 2019). Furthermore, this interaction is also implicated in senescence induced clearance. Alteration in Band 3 has been studied as senescent tag for macrophage recognition and clearance if senescent red cells. Oxidant stress-mediated denaturation of hemoglobin produces hemichromes that accumulate in the cytoplasm and copolymerize with the cytoplasmic domain of Band 3, forming an insoluble macromolecular aggregate (Low et al., 1985; Kannan et al., 1988). Oxidation of hemichrome-mediated clustering of Band 3 is thought to expose neoantigens that are recognized by naturally occurring antibodies and cleared by macrophages. In addition to hemichrome-induced clustering, calcium-dependent proteolytic degradation of Band 3 has been postulated to expose a senescent tag (Kay et al., 1986; Schwarz-Ben Meir et al., 1991). Furthermore, Band 3 clustering has been proposed to bind to an endothelial cell receptor for products of advanced glycation (Wautier and Wautier, 2020).
Naturally occurring antibodies (39) have germline immunoglobulin sequences that are polyreactive, binding with low affinity to multiple epitopes (Turman et al., 1991). Numerous functions have been attributed to these antibodies, including serving as the first line of defense against pathogens, clearance of cellular debris, and recognition of oxidation-specific epitopes in lipids and proteins. Naturally occurring antibodies that bind to clustered Band 3 or neoantigens in Band 3 have been proposed as mediators of senescent red cell clearance (Kay, 1985; Lutz, 2012). Studies by Kay et al. suggested that senescent antigen generation is the result proteolytic cleavage of Band 3 (Kay and Goodman, 1984). Old red cells were reported to have autologous serum antibodies bound to discrete regions of Band 3 localized to amino acids 538–554 and 778–827 (Kay and Lin, 1990). In addition to antibodies against Band 3, naturally occurring antigalactosyl antibodies have been postulated to bind these cryptic antigens exposed at senescence (Galili et al., 1986). However, there is no alteration in red cell clearance in agammaglobulinemic mice, questioning the physiological significance of antibody-mediated clearance of senescent red cell (Connor et al., 1994; Bratosin et al., 2002; Hudson et al., 2017). Advances in proteomics should further refine analysis of changes in Band 3 during aging in vivo (Bosman et al., 2008).
Band 4.1 is required for maintaining red cell shape by regulating the mechanical properties of deformability through lateral interactions with spectrin, actin and glycophorins. On SDS-PAGE electrophoresis, it appears as two distinct bands with molecular weights of 80 kDa (4.1a) and 78 kDa (4.1b). Inaba et al. (1992) have shown that the basis of the difference in molecular weight is post-translational deamidation of two carboxy-terminal asparagine residues (Asn478 and Asn502), which decreases the electrophoretic mobility of the protein. Deamidation may induce conformational changes that alter the functional characteristics of Band 4.1. The 78 kDa form (4.1b) is the major component in younger cells and reticulocytes (Sauberman et al., 1979). Mueller et al. (1987) analyzed the membrane proteins in young and old red cells by maintaining mice in a state of continuous erythropoietic suppression for up to 8 weeks using a serial hypertransfusion protocol. They showed that during in vivo aging, there is an increase in the ratio of 4.1a:4.1b and proposed this change in ratio as a measure of in vivo senescence (Mueller et al., 1987). The ratio of 4.1a:4.1b correlates with the average life span of red cells in other species, but whether the change in ratio contributes mechanistically to clearance of senescent red cells remains speculative.
CD47 is a heavily glycosylated cell surface protein belonging to the immunoglobulin superfamily that is present in red cells in complex with Rh proteins (Oldenborg, 2004). CD47 binds to its cognate receptor, signal regulatory protein alpha (SIRPα), on macrophages and suppresses phagocytosis by inhibiting inside-out activation of integrin signaling (Morrissey et al., 2020). CD47 deficient red cells are rapidly cleared from the peripheral circulation by splenic macrophages in a process that is independent of complement and antibodies (Oldenborg et al., 2000). Physiologically, CD47 functions to prevent autologous cells from undergoing phagocytosis (Jaiswal et al., 2009). Changes in CD47 have been investigated in relation to erythrocyte storage and senescence. CD47 was reported to be lost from human erythrocytes during storage (Anniss and Sparrow, 2002), and the density of CD47 was reported to decrease during aging of murine erythrocytes surface (Khandelwal et al., 2007), suggesting that reduced expression of CD47 facilitates phagocytosis of stored and senescent red cells. However, Rh(null) cells with reduced CD47 expression do not show heightened interaction with monocytes (Arndt and Garratty, 2004). Furthermore, in autoimmune hemolytic anemia, CD47 expression is normal (Ahrens et al., 2006).
Desialylation of membrane proteins was among the first mechanisms postulated to account for clearance of senescent red cell (Aminoff et al., 1977). Most erythrocyte membrane proteins are rich in sialic acid, which gives the cell a negative charge. It was hypothesized that progressive loss of sialic acid occurred as red cells age thereby providing a marker of senescence and a mechanism for recognition and clearance aged erythrocytes. Despite demonstration that enzymatic desialylation of red cells results in rapid clearance, sialic acid content does not change significantly as erythrocytes age (Shinozuka et al., 1988).
Klei et al. (2018) postulated a novel mechanism involving sialic acid in the clearance of senescent red cells. Lutheran/basal cell adhesion molecule (Lu/BCAM) is a transmembrane adhesion molecule that interacts with the extracellular matrix protein, laminin. This interaction is inhibited by sialic acid moieties of glycophorin C, a minor erythrocyte membrane glycoprotein (Jaskiewicz et al., 2018). Age related loss of glycophorin C sialic acid was hypothesized to contribute to clearance of senescent red cells by decreasing the inhibition of Lu/BCAM with laminin.
Asymmetrical distribution of phospholipids is a common property of all mammalian cells. In the case of red cells, anionic phospholipids reside in the inner leaflet while neutral or zwitterionic phospholipids predominantly comprise the outer leaflet (Bretscher, 1972; Verkleij et al., 1973; Connor et al., 1992). Phosphatidylserine exposure in the outer leaflet is the basis of recognition of apoptotic cells by the macrophages (Penberthy and Ravichandran, 2016). Schroit et al. (1985) reported that red cells enriched with phosphatidylserine analogs (by insertion of controlled amounts of fluorescent phosphatidylserine analogs into mouse red cells) on the outer surface are cleared five times faster than controls. These cells accumulated in splenic macrophages and hepatic Kupffer cells. Cell clearance depended on the amount of exogenously inserted phosphatidylserine, and more rapid clearance was when the cells contained as little as 1% phosphatidylserine (Schroit et al., 1985). However, clearance was incomplete, and this observation was attributed to aminophospholipid translocase activity, which continuously pumps phosphatidylserine to the inner leaflet of the circulating cells, thereby preventing red blood cell recognition by macrophages. Using annexin V binding to quantify phosphatidylserine exposure by biotin labeled red cells, Boas et al. (1998) reported greater binding for aged red cells, with the extent of phosphatidylserine expression paralleling the rate at which the biotinylated red cells were removed from circulation. Other studies, however, have not shown a greater phosphatidylserine in aged red cells compared to their younger counterparts (Wesseling et al., 2016). One explanation for these disparate observation is that phosphatidylserine-expressing red cells are removed from the circulation by macrophages at a rate that makes them undetectable (Schroit et al., 1985; Dasgupta et al., 2008).
Conceivably disturbances in mechanisms involved in the maintenance of membrane phospholipid could contribute to red cell senescence. P-type ATPases (flipases) catalyze the transport of phospholipids from the outer to the inner leaflet, Sebastian et al. (2012) while ABC ATPases (flopases) catalyze the transport of lipids from the inner to the outer leaflet (Coleman et al., 2013). Another enzyme, scramblase, that facilitates movement of lipids between both leaflets, has been identified. In contrast to ATP-dependent lipid translocases, scramblases are generally non-selective and energy independent, being activated by an increase in intracellular Ca2+ (Pomorski and Menon, 2016). Lipid scrambling changes the architecture of the bilayer, promoting exposure of phosphatidylserine and release of extracellular vesicles (Nagata et al., 2016; Whitlock and Hartzell, 2017).
A number of mechanisms have been proposed to explain phosphatidylserine exposure during red cells senescence. Oxidation of phosphatidylserine alters its ability to act as a substrate for aminophospholipid translocase, which transports phosphatidylserine from the outer to inner leaflet (Tyurina et al., 2000). Aging of erythrocytes increases both membrane lipid peroxidation (Ando et al., 1995) and oxidation of phosphatidylserine, consequently allowing phosphatidylserine to persist in the outer layer for macrophage recognition. The cytoskeleton in an important component of the process by which lipid asymmetry is maintained. Phosphatidylserine interacts with the major membrane cytoskeletal protein, spectrin (Kunzelmann-Marche et al., 2001). Disruption of membrane-cytoskeletal interactions, due to senescence-induced aggregation of Band 3, may result in phosphatidylserine exposure. In a mouse model, widespread thrombosis and severe hemolysis due to increased phosphatidylserine exposure was observed in Band 3 null red cells (Hassoun et al., 1998). However, in hereditary spherocytosis, erythrocytes fully conserve lipid asymmetry despite abnormalities in membrane skeleton components (Calvez et al., 1988; Kuypers et al., 1993). This observation suggests that the contribution of cytoskeletal proteins to regulation of phosphatidylserine is insignificant.
An increase in intracellular Ca2+ in red cells activates scramblase, exposing phosphatidylserine similar to that observed in nucleated cells during apoptosis (Fadok et al., 2001). Storage of human red cells under blood bank conditions resulted in a small increase in Ca2+ permeability (Larsson et al., 2016), but an age related mechanism that increases red cell calcium has not been identified (Khandelwal and Saxena, 2008). On the other hand, clustering of Band 3 has bee reported to induce externalization of phosphatidylserine by a calcium- and oxidation-independent mechanism (Koshkaryev et al., 2020).
Eryptosis is the process of cell shrinkage and exposure of phosphatidylserine due influx of calcium ions that activates a scramblase resulting in redistribution of phospholipids in both leaflets (Lang et al., 2008). Eryptosis may contribute to red cell clearance in diseased states, but its contribution to senescence-associated clearance is speculative.
Physiological processes that delay senescence may contribute to hypoxia-induced erythrocytosis Tang et al. (2019) reported a reduction in phosphatidylserine, cytosolic Ca2+, and an increase in CD47 in red cells exposed to chronic hypoxia, and red cells produced in vivo under hypoxic conditions had a longer lifespan than cells produced under normoxic conditions. The studies provided the first evidence suggesting that longer red cell survival, in addition to hypoxia stimulation of erythropoiesis contributes to hypoxia-induced erythrocytosis.
Lactadherin, also called milk fat globule epidermal growth factor 8, is an opsonin that binds to phosphatidylserine expressing cell, including red cells (Hanayama et al., 2002; Dasgupta et al., 2008). It has a phosphatidylserine binding domain, as well as an Arginine-Glycine-Aspartic acid (RGD) motif in one of its epidermal growth factor domains which mediates binding to integrins (Andersen et al., 1997; Hanayama et al., 2002). Lactadherin-mediated erythrophagocytosis of phosphatidylserine expressing cells by integrins is proposed as a mechanism for clearance of senescent red cells by activated endothelial cells (Fens et al., 2012). However, red cell survival is normal in lactadherin deficient mice, and as discussed above, whether senescent red cells express greater amounts of phosphatidylserine is an issue of active debate (Dasgupta et al., 2008; Wesseling et al., 2016).
Although speculative, other phosphatidylserine binding proteins expressed by macrophages including CD36, SR B1, CD68, CD14, Mer, LOX-1 Bai1, TIM-4 RAGE, and stabilin-2 may be involved in clearance of senescent red cells, Bratton and Henson (2008), Park et al. (2008), and Ravichandran (2010).
Physiologic response to hypoxia is the stimulation of red blood cell production. Hypoxia-inducible factors (HIFs) orchestrate response to hypoxia and HIF-2 is the principal regulator of erythropoietin (EPO) production in kidney as underscored by genetic studies in human populations that live at high-altitude and by mutational analysis of patients with familial erythrocytosis (Prchal, 2015). Upon the rapid return to normoxia, the secondary erythrocytosis is overcorrected, as the accumulated, newly formed red cells undergo preferential destruction. This process, termed neocytolysis, was originally observed in astronauts returning to earth after living in a zero gravity environment (Rice et al., 2001). Return to normoxia from hypoxia results in generation of reactive oxygen species from increased mitochondrial mass that correlates with decreased expression of Bnip3L transcripts, a hypoxia regulated gene (Sandoval et al., 2008; Song et al., 2015). Bnip3L mediates removal of reticulocyte mitochondria that generate increased reactive oxygen species accompanied by reduced catalase activity mediated by hypoxia-regulated miR21 (Song et al., 2015). Rapid changes in hematocrit in human newborns also suggest that neocytolysis also occurs after birth. The hypoxic fetus has erythrocytosis at birth, but the neonate rapidly overcorrects its elevated red cell mass and becomes anemic in first 2 weeks of life (Christensen et al., 2013).
In contrast to our limited understanding of the physiological red blood cell clearance of senescent red cells, the mechanisms involved in removal of abnormal erythrocytes (hemolysis) are understood in greater details. Premature destruction can occur in the circulation by lysis with the release of hemoglobin into the plasma (intravascular hemolysis) or by the macrophages in the spleen and liver (extravascular hemolysis) with little release of hemoglobin. The spleen plays a major role here. Increased splenic clearance occurs due to injuries extrinsic events (immunological targeting, mechanical or chemical injuries) or due to intrinsic defects in red cells (due to inherited defects in red cells cytoskeleton or enzymes).
Red cells with reduced deformability are unable to negotiate through narrow endothelial slits in the human spleen. Consequently, they are retained in the splenic cords and eventually destroyed by red pulp macrophages. The principal determinants of the red blood cell deformability are the ratio of cell surface area to volume (determined by the shape), intracellular viscosity (determined the physical properties of hemoglobin), and membrane elasticity (determined by rheological properties of the membrane). As discussed earlier, red cells traverse the interendothelial slit in splenic sinusoids. When normal deformity is compromised, sustained elongation results in loss of membrane due to vesiculation (Li et al., 2018). Premature destruction occurs in many membrane disorders including hereditary spherocytosis, ovalocytosis, and pyropoikilocytosis. In addition to intrinsic membrane defects, the red cell membrane can be damaged by abnormalities in microcirculation due intravascular fibrin deposition and abnormal shearing due artificial heart valves, or severe aortic stenosis. The fragment erythrocytes are rapidly removed by the reticuloendothelial system. Collectively these processes are called microangiopathic hemolytic anemia.
Red cells efficiently transport oxygen throughout their lifespan unless they are damaged by ROS. Consequently, they have effective mechanism to quench ROS. The red cell spends a significant amount of energy to keep NADP in its reduced form (NADPH), thereby maintaining a readily available pool of electron donors to reduce ROS. Depletion of NADPH can occur in the G6PD deficiency, or structural hemoglobin abnormalities that predispose to hemoglobin oxidation, or exposure to oxidant drugs. Oxidation of hemoglobin alters the quaternary structure allowing them to precipitate within the red cell and to form aggregates called Heinz bodies. Heinz bodies attach to the red cell membrane decreasing deformability, thereby rendering the affected cells susceptible to engulfment by sinusoidal macrophages of the spleen and liver to membranes decreases the deformability and other physical properties pliable of red cell membrane, rendering them to engulfment by macrophages in rich sinusoids of spleen and liver. Macrophage-mediated removal of Heinz bodies leaves a defect in the erythrocyte membrane structure that may be seen as bite cells on microscopic examination of the peripheral blood smear.
Antibody-mediated intravascular hemolysis occurs due to complement activation by the classical pathway. IgM antibodies fix complement more avidly than IgG antibodies because of their they are pentavalent rather than bivalent. However, two IgG molecules in close proximity on the RBC surface can also activate complement. Hence, the antigen density is a critical determinate of complement activation in IgG-mediated immune hemolytic anemia (Garratty, 2008). As the macrophages do not express IgM Fc receptors (Kubagawa et al., 2009), IgM-mediated red cell destruction is mediated directly through complement induced damage and indirectly through macrophage clearance of complement opsonized cells. Immune complexes activate the classical complement pathway by binding the C1q portion of the C1 complex. Exposure of the collagen-like regions of C1q makes it recognizable by macrophage complement receptor 1 (CR1) (Eggleton et al., 2000), however, C1q opsonization does not contribute significantly to the pathophysiology of immune-mediated hemolytic anemia. Rather, during complement activation, the third component of complement (C3) is cleaved to C3b, which can bind covalently to cell surface carbohydrate and peptide moieties. Bound C3b is rapidly cleaved to an inactivated form, iC3b. Macrophages have receptors for C3b (CR1) and iC3b (CR3). Unlike FcγRs, which are constitutively active, the phagocytic function of CR3 requires activation through a distinct pathway (Allen and Aderem, 1996; Caron and Hall, 1998). Cell bound iC3b is rapidly degraded enzymatically to C3dg and C3d. The receptor for C3dg and C3d is CR2, expressed primarily by B lymphocytes. C3d/C3dg binds to CR2 with low affinity. Therefore clearance of C3d/C3dg opsonized red cells is inefficient, such that their lifespan is shortened less than C3b and iC3b coated cells, and red cells opsonized by C3d/C3dg can be found circulating in the peripheral blood of patients with immune hemolytic anemia, particularly in patients with cold agglutinin disease (Aderem and Underhill, 1999).
IgG-mediated extravascular hemolysis is mediated by macrophages in the spleen and liver (Eggleton et al., 2000). IgG antibodies bind red cell antigens, and the Fc portion of the bound immunoglobulin is recognized by specific macrophage receptors that mediate phagocytosis of the opsonized erythrocytes. There are three types of Fcγ receptors that activate phagocytosis, FcγRI (CD64), FcγRIIA (CD32a), and FcγRIII (CD16). Phagocytosed red cells are targeted to phagolysosomes (Mosser and Zhang, 2011). FcγRI has the highest affinity for IgG molecules, while FcγRIIA and FcγRIII have lower affinities (Bruhns et al., 2009). Furthermore, there are four subtypes of IgG – IgG1, IgG2, IgG3, and IgG4 with varying affinities for FcγRs (Vidarsson et al., 2014). FcγRI is a dimer consisting of a ligand-binding α-chain and a signal-transducing γ-chain, which carries immunoreceptor tyrosine based activating motifs (ITAMs) (Bournazos et al., 2016). ITAM-induced phosphorylation activates signaling pathways, including phosphatidylinositol kinase and MAP kinase that induces efficient erythrophagocytosis such that little free hemoglobin is released into the circulation. In addition to activating receptors, macrophages also express FcγRIIB, which is an inhibitory receptor that transmits signals through an immunoreceptor tyrosine-based inhibitory motif (ITIM) contained in cytoplasmic domain of the receptor. Intravenous immunoglobulin (IVIG) is a modestly effective treatment for autoimmune hemolytic anemia (Flores et al., 1993). Although other mechanisms contribute, the therapeutic activity of IVIgG appears to be mediated in part through binding to FcγRIIB.
This review provides an overview of our current knowledge of the mechanisms involved red cell clearance. Despite the plethora of investigations, our understanding of the molecular details of red cell clearance is incomplete. Recent progresses in in vivo red cell labeling and availability of novel proteomic techniques should provide the means to enhance our understanding of the processes that underlie red cell senescence.
All authors listed have made a substantial, direct and intellectual contribution to the work, and approved it for publication.
This study was supported in part by a grant from the United States Department of Veterans Affairs, Veterans Health Administration (Merit Review grant to JP), Office of Research and Development, Biomedical Laboratory Research and Development and a grant from National Institutes of Health (HL13950 to PT). The contents of this manuscript are solely the responsibility of the authors and do not necessarily represent the views of the “United States Department of Veterans Affairs or the United States Government.”
The authors declare that the research was conducted in the absence of any commercial or financial relationships that could be construed as a potential conflict of interest.
Aderem, A., and Underhill, D. M. (1999). Mechanisms of phagocytosis in macrophages. Annu. Rev. Immunol. 17, 593–623. doi: 10.1146/annurev.immunol.17.1.593
Ahrens, N., Pagenkopf, C., Kiesewetter, H., and Salama, A. (2006). CD47 is expressed at normal levels in patients with autoimmune haemolytic anaemia and/or immune thrombocytopenia. Transfus. Med. 16, 397–402. doi: 10.1111/j.1365-3148.2006.00688.x
Allen, L. A., and Aderem, A. (1996). Molecular definition of distinct cytoskeletal structures involved in complement- and Fc receptor-mediated phagocytosis in macrophages. J. Exp. Med. 184, 627–637. doi: 10.1084/jem.184.2.627
Allison, A. C., and Burn, G. P. (1955). Enzyme activity as a function of age in the human erythrocyte. Br. J. Haematol. 1, 291–303. doi: 10.1111/j.1365-2141.1955.tb05511.x
Aminoff, D., Bruegge, W. F., Bell, W. C., Sarpolis, K., and Williams, R. (1977). Role of sialic acid in survival of erythrocytes in the circulation: interaction of neuraminidase-treated and untreated erythrocytes with spleen and liver at the cellular level. Proc. Natl. Acad. Sci. U.S.A. 74, 1521–1524. doi: 10.1073/pnas.74.4.1521
Andersen, M. H., Berglund, L., Rasmussen, J. T., and Petersen, T. E. (1997). Bovine PAS-6/7 binds alpha v beta 5 integrins and anionic phospholipids through two domains. Biochemistry 36, 5441–5446. doi: 10.1021/bi963119m
Ando, K., Beppu, M., and Kikugawa, K. (1995). Evidence for accumulation of lipid hydroperoxides during the aging of human red blood cells in the circulation. Biol. Pharm. Bull. 18, 659–663. doi: 10.1248/bpb.18.659
Anniss, A. M., and Sparrow, R. L. (2002). Expression of CD47 (integrin-associated protein) decreases on red blood cells during storage. Transfus. Apher. Sci. 27, 233–238. doi: 10.1016/s1473-0502(02)00070-8
Anosa, V. O. (1976). Postsplenectomy blood values, marrow cytology, erythrocyte life-span, and sequestration in mice. Am. J. Physiol. 231, 1254–1257. doi: 10.1152/ajplegacy.1976.231.4.1254
Arndt, P. A., and Garratty, G. (2004). Rh(null) red blood cells with reduced CD47 do not show increased interactions with peripheral blood monocytes. [Letter]. Br. J. Haematol. 125, 412–414. doi: 10.1111/j.1365-2141.2004.04911.x
Badior, K. E., and Casey, J. R. (2018). Molecular mechanism for the red blood cell senescence clock. [Review]. IUBMB Life 70, 32–40. doi: 10.1002/iub.1703
Bernstein, R. E. (1959). Alterations in metabolic energetics and cation transport during aging of red cells. J. Clin. Invest. 38, 1572–1586. doi: 10.1172/JCI103936
Boas, F. E., Forman, L., and Beutler, E. (1998). Phosphatidylserine exposure and red cell viability in red cell aging and in hemolytic anemia. Proc. Natl. Acad. Sci. U.S.A. 95, 3077–3081. doi: 10.1073/pnas.95.6.3077
Borun, E. R., Figueroa, W. G., and Perry, S. M. (1957). The distribution of Fe59 tagged human erythrocytes in centrifuged specimens as a function of cell age. J. Clin. Invest. 36, 676–679. doi: 10.1172/JCI103468
Bosman, G. J., Lasonder, E., Luten, M., Roerdinkholder-Stoelwinder, B., Novotny, V. M., Bos, H., et al. (2008). The proteome of red cell membranes and vesicles during storage in blood bank conditions. Transfusion 48, 827–835. doi: 10.1111/j.1537-2995.2007.01630.x
Bournazos, S., Wang, T. T., and Ravetch, J. V. (2016). The role and function of fcgamma receptors on myeloid cells. Microbiol. Spectr. 4: 0.1128/microbiolsec.MCHD–0045–2016. doi: 10.1128/microbiolspec.MCHD-0045-2016
Bratosin, D., Estaquier, J., Ameisen, J. C., Aminoff, D., and Montreuil, J. (2002). Flow cytometric approach to the study of erythrophagocytosis: evidence for an alternative immunoglobulin-independent pathway in agammaglobulinemic mice. J. Immunol. Methods 265, 133–143. doi: 10.1016/s0022-1759(02)00076-5
Bratton, D. L., and Henson, P. M. (2008). Apoptotic cell recognition: will the real phosphatidylserine receptor(s) please stand up? Curr. Biol. 18, R76–R79. doi: 10.1016/j.cub.2007.11.024
Bretscher, M. S. (1972). Phosphatidyl-ethanolamine: differential labelling in intact cells and cell ghosts of human erythrocytes by a membrane-impermeable reagent. J. Mol. Biol. 71, 523–528. doi: 10.1016/s0022-2836(72)80020-2
Browne, T. R., Szabo, G. K., Ajami, A., and Wagner, D. (1993). Performance of human mass balance/metabolite identification studies using stable isotope (13C, 15N) labeling and continuous-flow isotope-ratio mass spectrometry as an alternative to radioactive labeling methods. J. Clin. Pharmacol. 33, 246–252. doi: 10.1002/j.1552-4604.1993.tb03951.x
Bruhns, P., Iannascoli, B., England, P., Mancardi, D. A., Fernandez, N., Jorieux, S., et al. (2009). Specificity and affinity of human Fcgamma receptors and their polymorphic variants for human IgG subclasses. Blood 113, 3716–3725. doi: 10.1182/blood-2008-09-179754
Calvez, J. Y., Zachowski, A., Herrmann, A., Morrot, G., and Devaux, P. F. (1988). Asymmetric distribution of phospholipids in spectrin-poor erythrocyte vesicles. Biochemistry 27, 5666–5670. doi: 10.1021/bi00415a041
Caron, E., and Hall, A. (1998). Identification of two distinct mechanisms of phagocytosis controlled by different Rho GTPases. Science 282, 1717–1721. doi: 10.1126/science.282.5394.1717
Carrell, R. W., Winterbourn, C. C., and French, J. K. (1977). Haemoglobin–a frustrated oxidase? Implications for red cell metabolism. Hemoglobin 1, 815–827. doi: 10.3109/03630267709003909
Christensen, R. D., Lambert, D. K., Henry, E., Eggert, L. D., Yaish, H. M., Reading, N. S., et al. (2013). Unexplained extreme hyperbilirubinemia among neonates in a multihospital healthcare system. Blood Cells Mol. Dis. 50, 105–109. doi: 10.1016/j.bcmd.2012.10.004
Clark, M. R. (1988). Senescence of red blood cells: progress and problems. Physiol. Rev. 68, 503–554. doi: 10.1152/physrev.1988.68.2.503
Cohen, N. S., Ekholm, J. E., Luthra, M. G., and Hanahan, D. J. (1976). Biochemical characterization of density-separated human erythrocytes. Biochim. Biophys. Acta 419, 229–242. doi: 10.1016/0005-2736(76)90349-7
Coleman, J. A., Quazi, F., and Molday, R. S. (2013). Mammalian P4-ATPases and ABC transporters and their role in phospholipid transport. Biochim. Biophys. Acta 1831, 555–574. doi: 10.1016/j.bbalip.2012.10.006
Connor, J., Pak, C. C., and Schroit, A. J. (1994). Exposure of phosphatidylserine in the outer leaflet of human red blood cells. relationship to cell density, cell age, and clearance by mononuclear cells. J. Biol. Chem. 269, 2399–2404. doi: 10.1016/s0021-9258(17)41959-4
Connor, J., Pak, C. H., Zwaal, R. F., and Schroit, A. J. (1992). Bidirectional transbilayer movement of phospholipid analogs in human red blood cells. evidence for an ATP-dependent and protein-mediated process. J. Biol. Chem. 267, 19412–19417. doi: 10.1016/s0021-9258(18)41791-7
Dale, G. L., and Norenberg, S. L. (1990). Density fractionation of erythrocytes by Percoll/hypaque results in only a slight enrichment for aged cells. Biochim. Biophys. Acta 1036, 183–187. doi: 10.1016/0304-4165(90)90032-r
Dasgupta, S. K., Abdel-Monem, H., Guchhait, P., Nagata, S., and Thiagarajan, P. (2008). Role of lactadherin in the clearance of phosphatidylserine-expressing red blood cells. Transfusion 48, 2370–2376. doi: 10.1111/j.1537-2995.2008.01841.x
de Back, D. Z., Vlaar, R., Beuger, B., Daal, B., Lagerberg, J., Vlaar, A. P. J., et al. (2018). A method for red blood cell biotinylation in a closed system. Transfusion 58, 896–904. doi: 10.1111/trf.14535
Eggleton, P., Tenner, A. J., and Reid, K. B. (2000). C1q receptors. Clin. Exp. Immunol. 120, 406–412. doi: 10.1046/j.1365-2249.2000.01218.x
Fadok, V. A., Bratton, D. L., and Henson, P. M. (2001). Phagocyte receptors for apoptotic cells: recognition, uptake, and consequences. J. Clin. Invest. 108, 957–962. doi: 10.1172/jci200114122
Fens, M. H., van Wijk, R., Andringa, G., van Rooijen, K. L., Dijstelbloem, H. M., Rasmussen, J. T., et al. (2012). A role for activated endothelial cells in red blood cell clearance: implications for vasopathology. Haematologica 97, 500–508. doi: 10.3324/haematol.2011.048694
Flores, G., Cunningham-Rundles, C., Newland, A. C., and Bussel, J. B. (1993). Efficacy of intravenous immunoglobulin in the treatment of autoimmune hemolytic anemia: results in 73 patients. Am. J. Hematol. 44, 237–242. doi: 10.1002/ajh.2830440404
Galili, U., Flechner, I., Knyszynski, A., Danon, D., and Rachmilewitz, E. A. (1986). The natural anti-alpha-galactosyl IgG on human normal senescent red blood cells. Br. J. Haematol. 62, 317–324. doi: 10.1111/j.1365-2141.1986.tb02935.x
Ganzoni, A. M., Oakes, R., and Hillman, R. S. (1971). Red cell aging in vivo. J. Clin. Invest. 50, 1373–1378. doi: 10.1172/jci106619
Garratty, G. (2008). The james blundell award lecture 2007: do we really understand immune red cell destruction? [Lecture]. Transfus. Med. 18, 321–334. doi: 10.1111/j.1365-3148.2008.00891.x
Gottlieb, Y., Topaz, O., Cohen, L. A., Yakov, L. D., Haber, T., Morgenstern, A., et al. (2012). Physiologically aged red blood cells undergo erythrophagocytosis in vivo but not in vitro. Haematologica 97, 994–1002. doi: 10.3324/haematol.2011.057620
Hadley, T., Saul, A., Lamont, G., Hudson, D. E., Miller, L. H., and Kidson, C. (1983). Resistance of melanesian elliptocytes (ovalocytes) to invasion by Plasmodium knowlesi and Plasmodium falciparum malaria parasites in vitro. J. Clin. Invest. 71, 780–782. doi: 10.1172/jci110827
Hanayama, R., Tanaka, M., Miwa, K., Shinohara, A., Iwamatsu, A., and Nagata, S. (2002). Identification of a factor that links apoptotic cells to phagocytes. Nature 417, 182–187. doi: 10.1038/417182a
Haram, S., Carriero, D., Seaman, C., and Piomelli, S. (1991). The mechanism of decline of age-dependent enzymes in the red blood cell. Enzyme 45, 47–53. doi: 10.1159/000468864
Hassoun, H., Wang, Y., Vassiliadis, J., Lutchman, M., Palek, J., Aish, L., et al. (1998). Targeted inactivation of murine band 3 (AE1) gene produces a hypercoagulable state causing widespread thrombosis in vivo. Blood 92, 1785–1792. doi: 10.1182/blood.v92.5.1785.417k17_1785_1792
Hatae, H., Inaka, K., Okamura, R., Furubayashi, N., Kamo, M., Kobayashi, T., et al. (2018). Crystallization of human erythrocyte band 3, the anion exchanger, at the international space station “KIBO”. Anal. Biochem. 559, 91–93. doi: 10.1016/j.ab.2018.08.009
Hentschel, W. M., Wu, L. L., Tobin, G. O., Anstall, H. B., Smith, J. B., Williams, R. R., et al. (1986). Erythrocyte cation transport activities as a function of cell age. Clin. Chim. Acta 157, 33–43. doi: 10.1016/0009-8981(86)90315-3
Hudson, K. E., de Wolski, K., Kapp, L. M., Richards, A. L., Schniederjan, M. J., and Zimring, J. C. (2017). Antibodies to senescent antigen and C3 are not required for normal red blood cell lifespan in a murine model. Front. Immunol. 8:1425. doi: 10.3389/fimmu.2017.01425
Inaba, M., Gupta, K. C., Kuwabara, M., Takahashi, T., Benz, E. J. Jr., and Maede, Y. (1992). Deamidation of human erythrocyte protein 4.1: possible role in aging. Blood 79, 3355–3361. doi: 10.1182/blood.v79.12.3355.bloodjournal79123355
Jaiswal, S., Jamieson, C. H., Pang, W. W., Park, C. Y., Chao, M. P., Majeti, R., et al. (2009). CD47 is upregulated on circulating hematopoietic stem cells and leukemia cells to avoid phagocytosis. Cell 138, 271–285. doi: 10.1016/j.cell.2009.05.046
Jaskiewicz, E., Peyrard, T., Kaczmarek, R., Zerka, A., Jodlowska, M., and Czerwinski, M. (2018). The gerbich blood group system: old knowledge, new importance. Transfus. Med. Rev. 32, 111–116. doi: 10.1016/j.tmrv.2018.02.004
Kannan, R., Labotka, R., and Low, P. S. (1988). Isolation and characterization of the hemichrome-stabilized membrane protein aggregates from sickle erythrocytes. major site of autologous antibody binding. J. Biol. Chem. 263, 13766–13773. doi: 10.1016/s0021-9258(18)68308-5
Kay, M. M. (1985). Aging of cell membrane molecules leads to appearance of an aging antigen and removal of senescent cells. Gerontology 31, 215–235. doi: 10.1159/000212706
Kay, M. M., Bosman, G. J., Shapiro, S. S., Bendich, A., and Bassel, P. S. (1986). Oxidation as a possible mechanism of cellular aging: vitamin E deficiency causes premature aging and IgG binding to erythrocytes. Proc. Natl. Acad. Sci. U.S.A. 83, 2463–2467. doi: 10.1073/pnas.83.8.2463
Kay, M. M., and Goodman, J. R. (1984). IgG antibodies do not bind to band 3 in intact erythrocytes; enzymatic treatment of cells is required for IgG binding. Biomed. Biochim. Acta 43, 841–846.
Kay, M. M., and Lin, F. B. (1990). Molecular mapping of the active site of an aging antigen: senescent cell antigen requires lysine(s) for antigenicity and is located on an anion-binding segment of band 3 membrane transport protein. Gerontology 36, 293–305. doi: 10.1159/000213214
Khandelwal, S., and Saxena, R. K. (2008). A role of phosphatidylserine externalization in clearance of erythrocytes exposed to stress but not in eliminating aging populations of erythrocyte in mice. Exp. Gerontol. 43, 764–770. doi: 10.1016/j.exger.2008.05.002
Khandelwal, S., van Rooijen, N., and Saxena, R. K. (2007). Reduced expression of CD47 during murine red blood cell (RBC) senescence and its role in RBC clearance from the circulation. Transfusion 47, 1725–1732. doi: 10.1111/j.1537-2995.2007.01348.x
Khera, P. K., Smith, E. P., Lindsell, C. J., Rogge, M. C., Haggerty, S., Wagner, D. A., et al. (2015). Use of an oral stable isotope label to confirm variation in red blood cell mean age that influences HbA1c interpretation. Am. J. Hematol. 90, 50–55. doi: 10.1002/ajh.23866
Klei, T. R. L., Dalimot, J., Nota, B., Veldthuis, M., Mul, F. P. J., Rademakers, T., et al. (2020). Hemolysis in the spleen drives erythrocyte turnover. Blood 136, 1579–1589. doi: 10.1182/blood.2020005351
Klei, T. R. L., de Back, D. Z., Asif, P. J., Verkuijlen, P. J. J. H., Veldthuis, M., Ligthart, P. C., et al. (2018). Glycophorin-C sialylation regulates Lu/BCAM adhesive capacity during erythrocyte aging. Blood Adv. 2, 14–24. doi: 10.1182/bloodadvances.2017013094
Koshkaryev, A., Livshits, L., Pajic-Lijakovic, I., Gural, A., Barshtein, G., and Yedgar, S. (2020). Non-oxidative band-3 clustering agents cause the externalization of phosphatidylserine on erythrocyte surfaces by a calcium-independent mechanism. Biochim. Biophys. Acta Biomembr. 1862, 183231. doi: 10.1016/j.bbamem.2020.183231
Kubagawa, H., Oka, S., Kubagawa, Y., Torii, I., Takayama, E., Kang, D. W., et al. (2009). Identity of the elusive IgM Fc receptor (FcmuR) in humans. J. Exp. Med. 206, 2779–2793. doi: 10.1084/jem.20091107
Kunzelmann-Marche, C., Freyssinet, J. M., and Martinez, M. C. (2001). Regulation of phosphatidylserine transbilayer redistribution by store-operated Ca2+ entry: role of actin cytoskeleton. J. Biol. Chem. 276, 5134–5139. doi: 10.1074/jbc.M007924200
Kuypers, F. A., Lubin, B. H., Yee, M., Agre, P., Devaux, P. F., and Geldwerth, D. (1993). The distribution of erythrocyte phospholipids in hereditary spherocytosis demonstrates a minimal role for erythrocyte spectrin on phospholipid diffusion and asymmetry. Blood 81, 1051–1057. doi: 10.1182/blood.v81.4.1051.bloodjournal8141051
Lang, F., Gulbins, E., Lerche, H., Huber, S. M., Kempe, D. S., and Foller, M. (2008). Eryptosis, a window to systemic disease. Cell Physiol. Biochem. 22, 373–380. doi: 10.1159/000185448
Larsson, A., Hult, A., Nilsson, A., Olsson, M., and Oldenborg, P. A. (2016). Red blood cells with elevated cytoplasmic Ca(2+) are primarily taken up by splenic marginal zone macrophages and CD207+ dendritic cells. Transfusion 56, 1834–1844. doi: 10.1111/trf.13612
Lew, V. L., and Tiffert, T. (2013). The terminal density reversal phenomenon of aging human red blood cells. Front. Physiol. 4:171. doi: 10.3389/fphys.2013.00171
Lew, V. L., and Tiffert, T. (2017). On the mechanism of human red blood cell longevity: roles of calcium, the sodium pump, PIEZO1, and gardos channels. [Review]. Front. Physiol. 8:977. doi: 10.3389/fphys.2017.00977
Li, H., Lu, L., Li, X., Buffet, P. A., Dao, M., Karniadakis, G. E., et al. (2018). Mechanics of diseased red blood cells in human spleen and consequences for hereditary blood disorders. Proc. Natl. Acad. Sci. U.S.A. 115, 9574–9579. doi: 10.1073/pnas.1806501115
Linderkamp, O., Friederichs, E., Boehler, T., and Ludwig, A. (1993). Age dependency of red blood cell deformability and density: studies in transient erythroblastopenia of childhood. Br. J. Haematol. 83, 125–129. doi: 10.1111/j.1365-2141.1993.tb04642.x
Low, P. S., Waugh, S. M., Zinke, K., and Drenckhahn, D. (1985). The role of hemoglobin denaturation and band 3 clustering in red blood cell aging. Science 227, 531–533. doi: 10.1126/science.2578228
Lutz, H. U. (2012). Naturally occurring autoantibodies in mediating clearance of senescent red blood cells. Adv. Exp. Med. Biol. 750, 76–90. doi: 10.1007/978-1-4614-3461-0_6
Marks, P. A., and Johnson, A. B. (1958). Relationship between the age of human erythrocytes and their osmotic resistance: a basis for separating young and old erythrocytes. J. Clin. Invest. 37, 1542–1548. doi: 10.1172/JCI103746
Mebius, R. E., and Kraal, G. (2005). Structure and function of the spleen. [Review]. Nat. Rev. Immunol. 5, 606–616. doi: 10.1038/nri1669
Morrissey, M. A., Kern, N., and Vale, R. D. (2020). CD47 ligation repositions the inhibitory receptor SIRPA to suppress integrin activation and phagocytosis. Immunity 53, 290-302.e6. doi: 10.1016/j.immuni.2020.07.008
Mosser, D. M., and Zhang, X. (2011). Measuring opsonic phagocytosis via Fcγ receptors and complement receptors on macrophages. Curr. Protoc. Immunol. 95, 14.27.1–14.27.11. doi: 10.1002/0471142735.im1427s95
Mueller, T. J., Jackson, C. W., Dockter, M. E., and Morrison, M. (1987). Membrane skeletal alterations during in vivo mouse red cell aging. increase in the band 4.1a:4.1b ratio. J. Clin. Invest. 79, 492–499. doi: 10.1172/JCI112839
Nagata, S., Suzuki, J., Segawa, K., and Fujii, T. (2016). Exposure of phosphatidylserine on the cell surface. Cell Death Differ. 23, 952–961. doi: 10.1038/cdd.2016.7
Oldenborg, P. A. (2004). Role of CD47 in erythroid cells and in autoimmunity. Leuk. Lymphoma 45, 1319–1327. doi: 10.1080/1042819042000201989
Oldenborg, P. A., Zheleznyak, A., Fang, Y. F., Lagenaur, C. F., Gresham, H. D., and Lindberg, F. P. (2000). Role of CD47 as a marker of self on red blood cells. Science 288, 2051–2054. doi: 10.1126/science.288.5473.2051
Park, S. Y., Jung, M. Y., Kim, H. J., Lee, S. J., Kim, S. Y., Lee, B. H., et al. (2008). Rapid cell corpse clearance by stabilin-2, a membrane phosphatidylserine receptor. Cell Death Differ. 15, 192–201. doi: 10.1038/sj.cdd.4402242
Penberthy, K. K., and Ravichandran, K. S. (2016). Apoptotic cell recognition receptors and scavenger receptors. Immunol. Rev. 269, 44–59. doi: 10.1111/imr.12376
Piomelli, S., and Seaman, C. (1993). Mechanism of red blood cell aging: relationship of cell density and cell age. Am. J. Hematol. 42, 46–52. doi: 10.1002/ajh.2830420110
Pomorski, T. G., and Menon, A. K. (2016). Lipid somersaults: uncovering the mechanisms of protein-mediated lipid flipping. Prog. Lipid Res. 64, 69–84. doi: 10.1016/j.plipres.2016.08.003
Prchal, J. T. (2015). “Secondary polycythemia (Erythrocytosis),” in, Williams Hematology, 9th Edn, eds K. Kaushansky, M. A. Lichtman, J. T. Prcha, M. Levi, O. W. Press, L. J. Burns, et al. (New York, NY: McGraw Hill).
Ravichandran, K. S. (2010). Find-me and eat-me signals in apoptotic cell clearance: progress and conundrums. J. Exp. Med. 207, 1807–1817. doi: 10.1084/jem.20101157
Reissmann, K. R., and Ito, K. (1966). Selective eradication of erythropoiesis by actinomycin D as the result of interference with hormonally controlled effector pathway of cell differentiation. Blood 28, 201–212. doi: 10.1182/blood.v28.2.201.201
Rice, L., Ruiz, W., Driscoll, T., Whitley, C. E., Tapia, R., Hachey, D. L., et al. (2001). Neocytolysis on descent from altitude: a newly recognized mechanism for the control of red cell mass. Ann. Intern. Med. 134, 652–656. doi: 10.7326/0003-4819-134-8-200104170-00010
Rifkind, J. M., Berkowitz, D. E., and Mohanty, J. G. (2019). Editorial: regulation of vascular function by circulating blood. [Editorial]. Front. Physiol. 10:492. doi: 10.3389/fphys.2019.00492
Rifkind, J. M., and Nagababu, E. (2013). Hemoglobin redox reactions and red blood cell aging. Antioxid. Redox Signal. 18, 2274–2283. doi: 10.1089/ars.2012.4867
Safeukui, I., Buffet, P. A., Deplaine, G., Perrot, S., Brousse, V., Sauvanet, A., et al. (2018). Sensing of red blood cells with decreased membrane deformability by the human spleen. Blood Adv. 2, 2581–2587. doi: 10.1182/bloodadvances.2018024562
Sagiv, E., Fasano, R. M., Luban, N. L. C., Josephson, C. D., Stowell, S. R., Roback, J. D., et al. (2018). Glucose-6-phosphate-dehydrogenase deficient red blood cell units are associated with decreased posttransfusion red blood cell survival in children with sickle cell disease. Am. J. Hematol. 93, 630–634. doi: 10.1002/ajh.25051
Sandoval, H., Thiagarajan, P., Dasgupta, S. K., Schumacher, A., Prchal, J. T., Chen, M., et al. (2008). Essential role for Nix in autophagic maturation of erythroid cells. Nature 454, 232–235. doi: 10.1038/nature07006
Sauberman, N., Fortier, N. L., Fairbanks, G., O’Connor, R. J., and Snyder, L. M. (1979). Red cell membrane in hemolytic disease. studies on variables affecting electrophoretic analysis. Biochim. Biophys. Acta 556, 292–313. doi: 10.1016/0005-2736(79)90049-x
Saul, A., Lamont, G., Sawyer, W. H., and Kidson, C. (1984). Decreased membrane deformability in Melanesian ovalocytes from Papua New Guinea. J. Cell Biol. 98, 1348–1354. doi: 10.1083/jcb.98.4.1348
Schroit, A. J., Madsen, J. W., and Tanaka, Y. (1985). In vivo recognition and clearance of red blood cells containing phosphatidylserine in their plasma membranes. J. Biol. Chem. 260, 5131–5138. doi: 10.1016/s0021-9258(18)89189-x
Schwarz-Ben Meir, N., Glaser, T., and Kosower, N. S. (1991). Band 3 protein degradation by calpain is enhanced in erythrocytes of old people. Biochem. J. 275(Pt. 1), 47–52. doi: 10.1042/bj2750047
Seaman, C., Wyss, S., and Piomelli, S. (1980). The decline in energetic metabolism with aging of the erythrocyte and its relationship to cell death. Am. J. Hematol. 8, 31–42. doi: 10.1002/ajh.2830080105
Sebastian, T. T., Baldridge, R. D., Xu, P., and Graham, T. R. (2012). Phospholipid flippases: building asymmetric membranes and transport vesicles. Biochim. Biophys. Acta 1821, 1068–1077. doi: 10.1016/j.bbalip.2011.12.007
Shinozuka, T., Takei, S., Yanagida, J., Watanabe, H., and Ohkuma, S. (1988). Binding of lectins to “young” and “old” human erythrocytes. Blut 57, 117–123. doi: 10.1007/bf00320150
Song, J., Yoon, D., Christensen, R. D., Horvathova, M., Thiagarajan, P., and Prchal, J. T. (2015). HIF-mediated increased ROS from reduced mitophagy and decreased catalase causes neocytolysis. J. Mol. Med. 93, 857–866. doi: 10.1007/s00109-015-1294-y
Tang, F., Feng, L., Li, R., Wang, W., Liu, H., Yang, Q., et al. (2019). Inhibition of suicidal erythrocyte death by chronic hypoxia. High Alt. Med. Biol. 20, 112–119. doi: 10.1089/ham.2017.0159
ten Brinke, M., and de Regt, J. (1970). 51Cr-half life time of heavy and light human erythrocytes. Scand. J. Haematol. 7, 336–341. doi: 10.1111/j.1600-0609.1970.tb01911.x
Tomaiuolo, G., and Guido, S. (2011). Start-up shape dynamics of red blood cells in microcapillary flow. Microvasc. Res. 82, 35–41. doi: 10.1016/j.mvr.2011.03.004
Turman, M. A., Casali, P., Notkins, A. L., Bach, F. H., and Platt, J. L. (1991). Polyreactivity and antigen specificity of human xenoreactive monoclonal and serum natural antibodies. Transplantation 52, 710–717. doi: 10.1097/00007890-199110000-00024
Tyurina, Y. Y., Shvedova, A. A., Kawai, K., Tyurin, V. A., Kommineni, C., Quinn, P. J., et al. (2000). Phospholipid signaling in apoptosis: peroxidation and externalization of phosphatidylserine. Toxicology 148, 93–101. doi: 10.1016/s0300-483x(00)00199-2
Van Dilla, M. A., and Spalding, J. F. (1967). Erythrocyte volume distribution during recovery from bone marrow arrest. Nature 213, 708–709. doi: 10.1038/213708a0
Verkleij, A. J., Zwaal, R. F., Roelofsen, B., Comfurius, P., Kastelijn, D., and van Deenen, L. L. (1973). The asymmetric distribution of phospholipids in the human red cell membrane. a combined study using phospholipases and freeze-etch electron microscopy. Biochim. Biophys. Acta 323, 178–193. doi: 10.1016/0005-2736(73)90143-0
Vidarsson, G., Dekkers, G., and Rispens, T. (2014). IgG subclasses and allotypes: from structure to effector functions. Front. Immunol. 5:520. doi: 10.3389/fimmu.2014.00520
Vince, J. W., and Reithmeier, R. A. (1998). Carbonic anhydrase II binds to the carboxyl terminus of human band 3, the erythrocyte C1-/HCO3- exchanger. J. Biol. Chem. 273, 28430–28437. doi: 10.1074/jbc.273.43.28430
Waugh, R. E., Narla, M., Jackson, C. W., Mueller, T. J., Suzuki, T., and Dale, G. L. (1992). Rheologic properties of senescent erythrocytes: loss of surface area and volume with red blood cell age. Blood 79, 1351–1358. doi: 10.1182/blood.v79.5.1351.bloodjournal7951351
Wautier, J. L., and Wautier, M. P. (2020). Cellular and Molecular aspects of blood Cell-Endothelium interactions in vascular disorders. Int. J. Mol. Sci. 21:5315. doi: 10.3390/ijms21155315
Wesseling, M. C., Wagner-Britz, L., Huppert, H., Hanf, B., Hertz, L., Nguyen, D. B., et al. (2016). Phosphatidylserine Exposure in human red blood cells depending on cell age. Cell Physiol. Biochem. 38, 1376–1390. doi: 10.1159/000443081
Whitlock, J. M., and Hartzell, H. C. (2017). Anoctamins/TMEM16 Proteins: chloride channels flirting with lipids and extracellular vesicles. Annu. Rev. Physiol. 79, 119–143. doi: 10.1146/annurev-physiol-022516-034031
Willekens, F. L., Roerdinkholder-Stoelwinder, B., Groenen-Dopp, Y. A., Bos, H. J., Bosman, G. J., van den Bos, A. G., et al. (2003). Hemoglobin loss from erythrocytes in vivo results from spleen-facilitated vesiculation. Blood 101, 747–751. doi: 10.1182/blood-2002-02-0500
Keywords: red cell deformability, phosphatidylserine (PS) exposure, complement, Band 3, spleen
Citation: Thiagarajan P, Parker CJ and Prchal JT (2021) How Do Red Blood Cells Die? Front. Physiol. 12:655393. doi: 10.3389/fphys.2021.655393
Received: 18 January 2021; Accepted: 24 February 2021;
Published: 15 March 2021.
Edited by:
Dmitry A. Fedosov, Julich-Forschungszentrum, Helmholtz-Verband Deutscher Forschungszentren (HZ), GermanyReviewed by:
Syed Qadri, Ontario Tech University, CanadaCopyright © 2021 Thiagarajan, Parker and Prchal. This is an open-access article distributed under the terms of the Creative Commons Attribution License (CC BY). The use, distribution or reproduction in other forums is permitted, provided the original author(s) and the copyright owner(s) are credited and that the original publication in this journal is cited, in accordance with accepted academic practice. No use, distribution or reproduction is permitted which does not comply with these terms.
*Correspondence: Perumal Thiagarajan, cGVydW1hbHRAYmNtLmVkdQ==
Disclaimer: All claims expressed in this article are solely those of the authors and do not necessarily represent those of their affiliated organizations, or those of the publisher, the editors and the reviewers. Any product that may be evaluated in this article or claim that may be made by its manufacturer is not guaranteed or endorsed by the publisher.
Research integrity at Frontiers
Learn more about the work of our research integrity team to safeguard the quality of each article we publish.