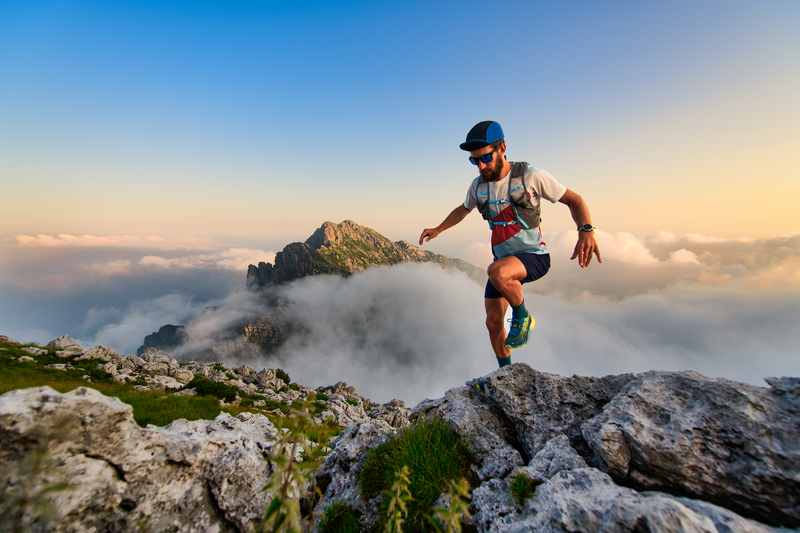
95% of researchers rate our articles as excellent or good
Learn more about the work of our research integrity team to safeguard the quality of each article we publish.
Find out more
ORIGINAL RESEARCH article
Front. Physiol. , 01 April 2021
Sec. Cardiac Electrophysiology
Volume 12 - 2021 | https://doi.org/10.3389/fphys.2021.650964
This article is part of the Research Topic Atrial Fibrillation: Technology for Diagnosis, Monitoring, and Treatment, Volume I View all 38 articles
In search of more efficacious and safe pharmacological treatments for atrial fibrillation (AF), atria-selective antiarrhythmic agents have been promoted that target ion channels principally expressed in the atria. This concept allows one to engage antiarrhythmic effects in atria, but spares the ventricles from potentially proarrhythmic side effects. It has been suggested that cardiac small conductance Ca2+-activated K+ (SK) channels may represent an atria-selective target in mammals including humans. However, there are conflicting data concerning the expression of SK channels in different stages of AF, and recent findings suggest that SK channels are upregulated in ventricular myocardium when patients develop heart failure. To address this issue, RNA-sequencing was performed to compare expression levels of three SK channels (KCNN1, KCNN2, and KCNN3) in human atrial and ventricular tissue samples from transplant donor hearts (no cardiac disease), and patients with cardiac disease in sinus rhythm or with AF. In addition, for control purposes expression levels of several genes known to be either chamber-selective or differentially expressed in AF and heart failure were determined. In atria, as compared to ventricle from transplant donor hearts, we confirmed higher expression of KCNN1 and KCNA5, and lower expression of KCNJ2, whereas KCNN2 and KCNN3 were statistically not differentially expressed. Overall expression of KCNN1 was low compared to KCNN2 and KCNN3. Comparing atrial tissue from patients with AF to sinus rhythm samples we saw downregulation of KCNN2 in AF, as previously reported. When comparing ventricular tissue from heart failure patients to non-diseased samples, we found significantly increased ventricular expression of KCNN3 in heart failure, as previously published. The other channels showed no significant difference in expression in either disease. Our results add weight to the view that SK channels are not likely to be an atria-selective target, especially in failing human hearts, and modulators of these channels may prove to have less utility in treating AF than hoped. Whether targeting SK1 holds potential remains to be elucidated.
Treatment of atrial fibrillation (AF) with antiarrhythmic drugs is hampered by the propensity of pharmacological interventions to induce ventricular proarrhythmic effects. Attempts to circumvent this problem have led to the concept of atria-selective drugs that target ion channels expressed only or predominantly in the atria, but not in the ventricles (for recent review see Peyronnet and Ravens, 2019). Presently, four groups of ion channels are considered to be atria-selective, i.e., the ultra-rapidly activating, outward rectifier K+ channel, Kv1.5 (IKur) (Ravens and Wettwer, 2011), the acetylcholine-activated inward rectifier K+ channel, Kir2.x (IK,ACh) (Koumi and Wasserstrom, 1994), the two-pore domain K+ (K2P) channels, TWIK-1 (tandem of two-pore-domain, weak inward rectifying K+-1) channel and TASK-1 (TWIK-related acid-sensitive K+) channel (Gaborit et al., 2007; Schmidt et al., 2015), as well as the small conductance Ca2+-activated K+ (SK) channels, SK1, SK2, and SK3 (Skibsbye et al., 2014). Novel drugs that selectively target each group of these ion channels have been associated with great expectations for a breakthrough in pharmacological therapy of AF. However, the results of clinical trials related to IKur and IK,ACh inhibitors have been disappointing, due to lack of efficacy or on-target toxicity (Pavri et al., 2012; Walfridsson et al., 2015; Podd et al., 2016; Shunmugam et al., 2018). A clinical trial regarding the efficacy and safety of a (non-selective) TASK-1 channel inhibitor is still ongoing [Doxapram conversion to sinus rhythm (SR) “DOCTOS” study, EudraCT No: 2018-002979-17, Protocol-Code: K620]. For SK-channel inhibition, preclinical research is ongoing (Saljic et al., 2019; Shamsaldeen et al., 2019; Diness et al., 2020), and this atria-selective antiarrhythmic drug target appears to be the only one not yet investigated in patients (Heijman and Dobrev, 2017).
Apamin, a polypeptide bee venom toxin has been shown to selectively inhibit SK channel current in mouse atrial and ventricular myocytes, as well as in human atrial myocytes (Xu et al., 2003). In addition, apamin prolongs action potential (AP) duration to a much larger extent in atrial than in ventricular murine and human myocytes. In these cells, the atria-selective expression of SK2 channels has been confirmed by Western blot and polymerase chain reaction (Xu et al., 2003; Tuteja et al., 2005). Interestingly, already the first description of cardiac SK channels closes with suggesting them as putative target for treating atrial arrhythmias (Xu et al., 2003). However, results from subsequent work are conflicting. Genetic knock-out of the SK2 channel gene in mice leads to marked atrial AP prolongation with no change in ventricle, but knock-out animals exhibit increased susceptibility to AF (Li et al., 2009). Nagy and co-workers could not detect any effect of apamin on either atrial or ventricular AP duration in rat, dog and human, though determination in human atrial tissue was not carried out (Nagy et al., 2009). Importantly, SK channels can form heteromers from which some were reported to be apamin-insensitive, yet sensitive to small molecule inhibitors such as UCL1684 (Hancock et al., 2015; Shamsaldeen et al., 2019), a feature which may explain some apparent discrepancies between studies. Experimental evidence for the involvement of SK channels in electrical remodeling during AF is also equivocal. In human atrial tissue, upregulation (Zhou et al., 2014) or downregulation (Yu et al., 2012; Ling et al., 2013; Skibsbye et al., 2014) of SK channel expression and apamin-sensitive currents in AF have been reported.
The situation becomes even more unclear when recent experimental evidence in animal and human cardiac tissue is taken into account, which suggests that atria-selectivity of SK channel inhibitors could be compromised in certain clinical conditions (Chua et al., 2011; Chang et al., 2013a,b; Hsueh et al., 2013; Bonilla et al., 2014). For example, ventricular expression of SK2 channels is increased in a rat model of cardiac hypertrophy due to thoracic aortic banding (Hamilton et al., 2020).
Given that AF and heart failure (HF) have been shown to be pathological partners (Santhanakrishnan et al., 2016), expression of SK channels in atrial and left ventricular (LV) tissue from donor hearts not suitable for transplantation (no structural cardiac disease) was compared to expression of SK channels in LV tissue from patients with HF due to ischemic (ICM) or dilated cardiomyopathy (DCM), who required a LV assist device. In addition, expression of the SK channels was studied in human atrial tissue from patients with structural cardiac disease with SR or sustained AF. Expression data were analyzed using a next generation sequencing effort. We confirmed atria-selective expression of Kv1.5, Kir3.1, and SK1 channels but not of SK2 and SK3. Our results suggest that SK channels are expressed both in atria and ventricles, and hence should not be considered as an atria-selective target overall.
Human atrial tissue samples were obtained from patients with SR or AF, undergoing open heart surgery at the University Heart Center Freiburg-Bad Krozingen or at the Clinic for Cardiac Surgery, Heart Center Dresden. These samples were processed via the CardioVascular BioBank (CVBB) Freiburg im Breisgau, as approved by the Ethics Committee of the University of Freiburg, Freiburg im Breisgau, Germany (CVBB ethical approval reference 393/16; study approval reference 10719) and the Ethics Committee of the Medical Faculty of the Technical University Dresden, Dresden, Germany (ethical approval No. 15.1/01031/006/2008 and No. EK790799). LV tissue from patients with HF due to ICM or DCM was provided by the Department of Cardiology, University Hospital Heidelberg, Heidelberg, Germany. The study protocol was approved by the Ethics Committee of the Medical Faculty of Heidelberg University, Heidelberg, Germany (S-017/2013). Atrial and LV myocardium from donor hearts not suitable for transplantation was obtained from the University of Szeged, Szeged, Hungary [Study approval by the Medical Research Council Budapest, Scientific and Research Ethics Committee, Budapest, Hungary No. 4991-0/2010-1018EKU (339/PI/010)]. Hearts were not suitable for transplantation because they could not have reached a recipient in time or because no suitable recipient was found within time. Written and informed consent was obtained from all patients and the study was conducted in accordance with the Declaration of Helsinki.
Atrial and LV myocardium from donor hearts was used as control (no structural cardiac disease) and will be referred to as non-diseased henceforth. Furthermore, one patient was affected by both, coronary artery disease (CAD) and heart valve disease (HVD) and this tissue sample was grouped under both conditions. From another patient two tissue samples from the right atrial appendage (RAA) were sequenced. In order to stay consistent with the number of samples per patient per sample provenance and based on the principal component analysis (PCA) plot (not shown), one of those samples was withdrawn from analysis (Supplementary Figure 1). According to the guidelines of the European Society of Cardiology, the American Heart Association, the American College of Cardiology, and the Heart Rhythm Society (January et al., 2014; Kirchhof et al., 2016), for AF, one can distinguish between paroxysmal which is self-terminating or cardioverted within 7 days, and sustained AF which includes patients with persistent (lasting > 7 days), long-standing persistent (lasting > 1 year, therapeutically addressed), and permanent (lasting > 1 year, not therapeutically addressed). This classification is widely accepted both in clinical and research settings (Jahangir et al., 2007; Mittal, 2014). In this study, only tissue from patients with sustained AF was included in the AF group. Of note, all patients with AF also had CAD or HVD, i.e., a structural heart disease, as reason for cardiac surgery. Patient characteristics are summarized in Table 1.
Due to the limited availability of patient tissue samples, studies on human tissue often compare samples form patients with AF or HF to SR. In order to relate better our results to published work, especially results of functional experiments, LV samples with DCM and ICM were pooled as HF and samples from RAA with CAD and HVD were pooled for patients with SR.
Total ribonucleic acid (RNA) was extracted from 5 to 15 mg frozen tissue using the RNeasy Mini kit or the RNeasy Micro kit from Qiagen (Germany) following the manufacturer’s protocols. Samples were first reduced to powder using a Spectrum Bessman pulverizer (Thermo Fisher Scientific, United States), chilled in liquid nitrogen, and homogenized in RLT lysis buffer using a TissueLyser LT (Qiagen) with 5 mm stainless steel beads for 2 × 2 min cycles at 30 Hz. After proteinase K treatment for 10 min at 55°C, homogenates were cleared by centrifugation at 10,000 × g for 3 min and loaded on RNeasy Mini Spin Columns or RNeasy MinElute Spin Columns (Qiagen). After on-column deoxyribonuclease treatment and washing, RNA was eluted in 20–30 μL ribonuclease free water and stored at −80°C. In total, RNA was extracted from 40 tissue samples from 39 patients and from 25 tissue samples from 9 donor hearts.
RNA samples were quantified using the Qubit HS RNA kit (Thermo Fisher Scientific) with a Qubit 3.0 Fluorometer (Thermo Fisher Scientific), following manufacturer’s instructions. The integrity of the RNA was determined for all samples using an Agilent Bioanalyzer 2100 (Agilent Technologies, United States) with an Agilent RNA 6000 kit (Agilent Technologies). RNA samples with a RNA integrity number > 7.0 were considered intact and < 7.0 were considered degraded. Based on this, 14 samples were not sequenced (Supplementary Figure 1), including 1x non-diseased (LV), 2x DCM (LV), 3x ICM (LV), 1x non-diseased (RAA), 1x CAD (RAA), 1x CAD and HVD (RAA), 1x ICM (RAA), 2x AF (RAA), 2x non-diseased (LA).
Next generation sequencing libraries were prepared from 200 ng total RNA using the Truseq Stranded messenger RNA (mRNA) kit from Illumina (United States) that includes polyA purification. After quality controls, libraries were pooled in equimolar manner and run on Hiseq 4000 (Illumina) using a 2 × 150 base pairs mode (paired-end) to obtain at least 35 million reads per library.
The RNA-sequencing (RNA-seq) data analysis was carried out using Galaxy platform1 (Afgan et al., 2018), following guidelines from the tutorial “Reference-based RNA-Seq data analysis” (Batut et al., 2018, 2020). In short, quality control checks on raw sequence data was performed using FastQC (Andrews, 2010) and MultiQC (Ewels et al., 2016), taking into account the per base sequence quality (Phred score), per sequence quality scores (Phred score), per base sequence content, per sequence GC content, sequence duplication levels and over-represented sequences. Then, Cutadapt (Marcel, 2011) was used to remove adapter sequences. The splice-aware aligner STAR (Dobin et al., 2012) was used to map the RNA-seq reads onto the human reference genome (hg19). The mapping results were visualized by the integrative genome viewer, IGV (Robinson et al., 2011). Thereafter, the strandness of the RNA-seq data (reads mapping to the forward or reverse DNA strand) was estimated using Infer Experiment from the RSeQC (Wang et al., 2012). Gene expression was measured by featureCounts (Liao et al., 2013). At this step, one sample had to be removed from the study due to assignment of only 41% of the reads (Supplementary Figure 1). To compare between samples, gene counts were normalized for sequencing depth and gene length using transcripts per kilobase million (TPM) (Li and Dewey, 2011). The PCA of gene expression was performed using DESeq2 R package (Love et al., 2014).
Unless otherwise indicated, values are expressed as mean ± standard deviation (SD). N-numbers refer to the number of tissue samples, i.e., number of patients. Significance of the difference between means was assessed using the non-parametric Kruskal–Wallis test and Dunn’s test for post-hoc analysis. When pooling conditions into HF and SR, significance of the difference between means was tested with the non-parametric Mann–Whitney test. Figure 1 illustrates the comparisons of interest. A p-value < 0.05 was taken to indicate a significant difference between means. Statistical analysis and data representation was performed with OriginPro 2020.
Figure 1. Template figure for presentation of mRNA expression assessed by RNA-seq. Some p-values will be indicated in all graphs (p-value), whereas others are only indicated when p < 0.05 (only p < 0.05). Sample provenance: left ventricle (LV), right atrial appendage (RAA), left atrium (LA). Patient’s health status: non-diseased (ND); heart failure (HF; blue) summarizing data from dilated cardiomyopathy (DCM) and ischemic cardiomyopathy (ICM); sinus rhythm (SR; red) summarizing data from coronary artery disease (CAD) and heart valve disease (HVD); atrial fibrillation (AF). Statistical significance was assessed by Dunn’s test. For HF and SR, statistical significance was assessed by the Mann–Whitney test.
The PCA shows that the first dimension (PC1) roughly separated samples originating from LV from those originating from atria, and the second dimension (PC2) roughly separated samples originating from diseased hearts from those originating from non-diseased hearts (Figure 2). Differential gene expression between atria and LV accounted for 45% of the variability between individual samples, whereas disease-associated differences in gene expression account for 11% of variability. One data point (indicated by ∗) corresponded to a patient with CAD and clustered within the non-diseased group. Because of this discrepancy between clinical and experimental data, we decided to exclude this from subsequent analysis (Supplementary Figure 1). One patient was affected by both, CAD and HVD. The PCA plot showed that this data point lied within the clusters of these biological conditions and was retained for analysis.
Figure 2. PCA plot illustrating inter- and intra-group variability of samples assessed by RNA-seq. Each individual point represents data from one tissue sample. Dashed circles highlight clusters. ∗ indicates a data point showing discrepancy between clinical and experimental data. Sample provenance: left ventricle (LV; •), right atrial appendage (RAA; ▲), left atrium (LA; ▼). Patient’s health status: non-diseased (ND; green, open symbols), dilated cardiomyopathy (DCM; light blue), ischemic cardiomyopathy (ICM; blue), coronary artery disease (CAD; ocher), heart valve disease (HVD; orange), atrial fibrillation (AF; red).
We analyzed the expression of three housekeeping genes as internal controls. Housekeeping genes are defined as genes with a constant expression throughout chambers and throughout health status. We selected the popular GAPDH (Molina et al., 2018), PPIA (Molina et al., 2018), and HPRT1 (Cabiati et al., 2012). All three housekeeping genes showed stable mRNA expression between and within heart chambers, indicating a good quality of samples and sequencing (Supplementary Figures 2A–C). Each of these housekeeping genes covered a distinct range of TPM: four-digit range for GAPDH, three-digit range for PPIA, and one-digit range for HPRT1.
The two isoforms of myosin heavy chain (MHC-α and MHC-β) are encoded by the MYH6 and MYH7 genes, respectively. Expression of MYH6 was dominant in atria, whereas expression of MYH7 was dominant in the LV, confirming the respective atria- and LV-selective expression of these two genes (Figures 3A,B). Interestingly, in atrial samples from patients with AF, MYH7 expression was significantly higher than in atrial samples from patients with SR. To validate chamber-selective expression of ion channels, we analyzed the mRNA expression levels of KCNA5 (encoding Kv1.5), KCNJ2 (encoding the inward rectifier K+ channel Kir2.1), as well as KCNJ3 and KCNJ5 (encoding the G-protein-activated inward rectifier K+ channels Kir3.1 or GIRK1, and Kir3.4 or GIRK4, respectively). Expression of KCNA5 in the LV was low, whereas it was significantly higher RAA tissue, and no differences between non-diseased and diseased heart tissue were detected, neither in atrial nor LV samples (Figure 3C). Expression of the genes encoding inward rectifier K+ channels is depicted in Figures 3D–F. For Kir2.1, mRNA expression was significantly larger in the LV than in RAA or left atrium (LA), and neither HF nor AF significantly changed the expression level in LV or atria, respectively (Figure 3D). In contrast, expression of KCNJ3 was significantly higher in RAA and LA than in LV tissue and also higher in HF tissue than in non-diseased LV tissue (Figure 3E). Expression of KCNJ5, however, was not significantly different between LV and atrial tissue. In addition, atrial expression of KCNJ5 was not statistically different between samples from patients with SR and AF but compared with RAA non-diseased samples (and also when compared to all RAA samples from patients with SR), KCNJ5 expression was significantly lower (Figure 3F). These findings by-and-large confirm the atria-selective expression of some characteristic genes.
Figure 3. Control of chamber identity and reproducibility of known chamber-selective gene expression in transcripts per kilobase million (TPM) assessed by RNA-seq. (A) MYH6 (myosin heavy chain 6), (B) MYH7 (myosin heavy chain 7), (C) KCNA5 (Kv1.5 or IKur channel), (D) KCNJ2 (Kir2.1 or K+ voltage-gated channel subfamily J member 2), (E) KCNJ3 (GIRK1 or Kir3.1 or K+ inwardly rectifying channel subfamily J member 3), (F) KCNJ5 (GIRK4 or Kir3.4 or K+ inwardly rectifying channel subfamily J member 5). Sample provenance and patient’s health status as in Figure 2. Heart failure (HF): pooled data from DCM and ICM; sinus rhythm (SR): pooled data from CAD and HVD. The mean ± SD data are represented. Statistical significance was assessed by Dunn’s test. For HF and SR, statistical significance was assessed by the Mann–Whitney test.
Furthermore, we show genes encoding for ion channels or exchangers for which HF- or AF-associated modulation of expression has been reported previously (Figure 4). KCND3 contributes to the transient outward K+ current, Ito, via Kv4.3 channels (Figure 4A). Expression of KCND3 was not statistically different in LV and atria but was lower in LV samples from failing than from non-diseased hearts. KCNK3 encodes the background K+ channel TASK-1 or K2P3.1. In contrast to KCND3, KCNK3 was expressed to a larger extent in LA than LV non-diseased samples and was upregulated in AF (Figure 4B). Cardiac L-type Ca2+ channel (Cav1.2 and Cav1.3) genes CACNA1C and CACNA1D were more extensively expressed in RAA and LA than in the LV, respectively (Figures 4C,D). For CACNA1C, no difference was found between non-diseased and diseased tissue samples, whereas CACNA1D was downregulated in HF and AF. Expression of the T-type Ca2+ channel (Cav3.1) gene CACNA1G was higher in RAA and LA than LV non-diseased samples. The low expression of CACNA1G in the LV tissue from non-diseased hearts was upregulated in HF samples (Figure 4E). The Na+/Ca2+ exchanger encoded by SLC8A1 was statistically not differentially expressed in LV and atrial tissue, but upregulated in AF (Figure 4F).
Figure 4. Expression of ion channel encoding genes previously related to AF or HF in transcripts per kilobase million (TPM) assessed by RNA-seq. (A) KCND3 (Kv4.3), (B) KCNK3 (TASK-1 or TWIK-related acid-sensitive K+ channel 1), (C) CACNA1C (Cav1.2 or Ca2+ channel, voltage-dependent, L-type, a1C subunit), (D) CACNA1D (Cav1.3 or Ca2+ channel, voltage-dependent, L-type, a1D subunit), (E) CACNA1G (Cav3.1 or Ca2+ channel, voltage-dependent, T-type, α1G subunit), (F) SLC8A1 (NCX1). Same layout as in Figure 3.
In addition to the above described genes related to ion transport, we also compared the expression of some disease-associated genes (Figure 5). The atrial natriuretic peptide encoding gene NPPA was highly abundant in RAA and LA compared to LV. In addition, it was upregulated in both HF and AF (Figure 5A). The angiotensin II receptor type 1 (AGTR1) gene was more prominently expressed in RAA than LV non-diseased tissue and when the LV was failing, AGTR1 expression was significantly lower than in non-diseased LV tissue (Figure 5B). As expected, expression of ventricular connexin-43 encoding gene GJA1 was more prominent in LV than RAA, whereas the atrial connexin-40 encoding gene GJA5 was more prominent in RAA (Figures 5C,D). No disease-related modulation of expression was detected in either gene. Another gene whose expression was not statistically different between all conditions was CAV1 (Figure 5E). CAV1 encodes for the protein caveolin-1 which is required for forming specialized plasmalemmal invaginations, recruiting and stabilizing large protein complexes involved in signal transduction. The mRNA for transcription factor Pitx2 (paired-like homeodomain transcription factor 2), which is responsible for the development of cardiac left-right asymmetry, was massively expressed in LA non-diseased tissue (Figure 5F).
Figure 5. Control of disease identity and reproducibility of known AF- or HF-related gene expression in transcripts per kilobase million (TPM) assessed by RNA-seq. (A) NPPA (atrial natriuretic peptide A), (B) AGTR1 (AT1 receptor or angiotensin II receptor type 1), (C) GJA1 (connexin-43), (D) GJA5 (connexin-40), (E) CAV1 (caveolin-1), (F) PITX2 (paired-like homeodomain transcription factor 2). Same layout as in Figure 3.
The genes described so far were selected because of their previously reported chamber-selective expression or because of known modulation of expression in HF or AF. Since the main aim of this study was (i) to confirm chamber-selective expression of SK channels and (ii) to comment on the therapeutic potential of these channels in pharmacological treatment of AF, we have analyzed the expression levels of three SK channels encoded by KCNN1, KCNN2, and KCNN3 in our set of human cardiac tissue samples (Figure 6). For KCNN1, significantly lower mRNA expression was found in non-diseased LV than in non-diseased RAA and LA (Figure 6A), whereas KCNN2 and KCNN3 (Figures 6C,E) showed no chamber-selective mRNA expression.
Figure 6. Assessment of chamber-selective and disease-related mRNA expression of SK channels in transcripts per kilobase million (TPM) assessed by RNA-seq. (A) Expression of KCNN1 (SK1) in all sample groups; (B) Comparison of KCNN1 expression in LV tissue from non-diseased and failing hearts, in right atrial tissue from SR and AF patients, and in non-diseased left atrial tissue from non-diseased hearts. (C,D) Expression of KCNN2 (SK2); (E,F) expression of KCNN3 (SK3), figure lay-out as in (A,B). Sample provenance, patient’s health status and statistical tests as in Figure 3. Since disease-associated differences in expression of SK channels were our main concern, we separately depicted the statistical comparisons for HF and AF (B,D,F).
These results put into question the assumed atria-selective expression of SK channels in general. In order to answer the question of changes of ventricular SK channel expression in association with HF, and of atrial SK channel expression in association with AF, we also compared non-diseased LV tissue to all samples from failing hearts, and all diseased RAA samples from patients with SR to RAA tissue from AF patients. Interestingly, of the SK channels, only LV expression of KCNN3 was higher in HF, whereas KCNN1 and KCNN2 were statistically not differentially expressed. When comparing AF vs. SR tissue samples, KCNN1 and KCNN3 were not statistically different (Figures 6B,F), while KCNN2 was downregulated (Figure 6D).
In the present study using next generation sequencing, gene expression in three types of human LV and five types of atrial tissue samples was determined. Our main results are: (i) principal component analysis yields clear clustering of atrial vs. LV samples, and of diseased vs. non-diseased myocardium; (ii) in non-diseased hearts, only SK1 (but not SK2 and SK3) channels are expressed to a significantly larger extent in atrial (RAA and LA) than in LV tissue samples; (iii) HF is associated with an upregulation of SK3 channel, and AF is associated with a downregulation of SK2 isoform. These findings will be discussed below.
The various myocardial samples had to be collected from different hospitals, depending on tissue availability, but all were processed in the same laboratory for RNA extraction and preparation of the next generation sequencing libraries in order to ensure best possible comparability. Despite the high number of samples in which mRNA extraction was of insufficiently high quality – in total, 14 samples had to be rejected – PCA for all sixty thousand genes tested in the remaining 49 samples yielded good clustering (Figure 2). Indeed, PCA demonstrated four large clusters of data sets separating atrial from LV and non-diseased from diseased heart samples. Only one data point from RAA tissue from a CAD patient fell into the cluster for non-diseased tissue and was discarded from further analysis. The dimensions of the clusters demonstrate the scattering of the data points within a biological condition which is inherently due to inter-individual variability.
Given the large scatter in values within each sample group, the expression of several additional genes of known chamber-selective expression or previously reported disease-associated modulation was determined for control purposes. MYH6 and MYH7 encoding the two MHC isoforms MHC-α and MHC-β were utilized for validation of atria- or LV-selective gene expression (Everett, 1986; Kurabayashi et al., 1988). In accordance with the literature, expression of MYH6 was significantly higher in atrial than LV tissue, and the opposite was true for MYH7 expression.
The cardiac K+ channel Kv1.5 is the best studied representative of an atria-selective drug target based on electrophysiological and mRNA expression data (Amos et al., 1996; Gaborit et al., 2007). Numerous small molecule Kv1.5 inhibitors intended for treatment of AF have been developed (Ford and Milnes, 2008). Our results confirm previously published atria-selective expression of human KCNA5 and lack of AF-associated remodeling of mRNA expression (Gaborit et al., 2007). Table 2 summarizes the results of all genes that were tested for preferential expression in atria or LV in comparison with the literature. For the vast majority of cases we confirm the reported chamber-selective expression, although in some cases we were not able to detect the reported differences. However, this is not surprising, given the differences in patient numbers and populations as well as the different experimental conditions. Taken together, the chamber-selective expression of control genes reported here is in good agreement with the published literature (as summarized in Table 2) suggesting that our RNA analysis is reliable.
Since the focus of this study was not only at chamber-selective but also disease-modulated gene expression, some genes that are known to be differentially expressed in HF or AF, as for instance NPPA (Macchia, 1987), have been analyzed. We confirm that expression of NPPA was dominant in non-diseased atria, but LV NPPA expression was activated in response to HF (Chien et al., 1991; Schmitt et al., 2003; Houweling et al., 2005). Moreover, NPPA expression was upregulated in RAA from patients with AF (Büttner et al., 2018). In addition, we also found AF-associated upregulation of MYH7 (Nielsen et al., 2018; Thomas et al., 2019), KCNK3 (Schmidt et al., 2015, 2017), SLC8A1 (Schotten et al., 2002; Gaborit et al., 2005), and downregulation of CACNA1D (Van Wagoner and Nerbonne, 2000; Gaborit et al., 2005). For the other genes, for which AF-induced expression changes have been reported (see Table 2), we have not detected any significant changes in expression between atrial samples from patients with AF or SR. However, for two comparisons, part of the published literature agrees with our inability to detect a difference. Not in a single case did we observe statistically significant differences that were in the opposite direction to previously published reports. Failure to detect a significant difference does not indicate absence of any difference, but could also imply that the number of samples (in either of the analyzed cohorts) may not have been large enough. Overall, the agreement of our expression profiles with previous reports instills confidence that – where we do see differences – we can draw reliable conclusions, such as about SK channel expression in human atrial and LV myocardium.
The main purpose of our analysis was to assess whether SK channels are expressed to a much larger extent in atria than in the LV, which would presumably be necessary for them to serve as atria-selective drug targets. The first detection of SK channels in the heart demonstrated greater abundance in atria than ventricles, although only in mouse and not in human (Xu et al., 2003). Since then, evidence for atria-selective expression of SK channels has been obtained for many species (Tuteja et al., 2005: mouse; Diness et al., 2010: rat, guinea pig, rabbit; Skibsbye et al., 2014: human). To the best of our knowledge, our study is the first report of a direct comparison of atrial and LV SK channel expression in human non-diseased and diseased myocardium. We found a significantly higher expression of KCNN1 in RAA and LA tissue than in LV from non-diseased hearts, suggesting potential atria-selectivity for the SK1 channel. However, despite substantial expression of KCNN2 and KCNN3 in all chambers, these genes were not significantly enriched in the atria (compared to LV) in non-diseased hearts. Since non-diseased hearts do not require therapy, and since atria-selective drugs are intended for treatment of AF, we scrutinized SK channel expression in atrial tissue from patients with AF in comparison to atrial tissue from non-diseased donors or patients (CAD, HVD) with SR. In accordance with previous findings for AF, expression of KCNN1 did not significantly change and KCNN2 was downregulated, whereas we did not observe the previously reported downregulation of KCNN3 expression (Skibsbye et al., 2014). The rationale for suppressing atrial arrhythmias with SK channel blockers is to increase atrial refractoriness (Diness et al., 2020). The observed downregulation of SK2 may be an auto-regulatory mechanism which could be promoted by SK channel inhibitors.
Heart failure is a common comorbidity of AF and is associated with remodeling of ion channel expression in the ventricles (Nattel et al., 2007). Therefore, we also compared SK channel expression in tissue from failing and non-diseased human hearts. Of the three SK channels, KCNN3 was upregulated in failing human LV, while the other two SK channels were not statistically different. In the literature, SK channels in general were reported as being upregulated in failing rabbit hearts (Chua et al., 2011), SK2 channels in human failing (vs. non-failing) ventricular tissue or hypertrophied mouse ventricles (Chang et al., 2013a; Hamilton et al., 2020), and SK3 channels in failing dog hearts (Bonilla et al., 2014). It is an ongoing debate of whether SK channel upregulation is anti- or proarrhythmic (Chang and Chen, 2015; Van Wagoner, 2015).
Of course, it is difficult to correlate mRNA expression and protein function. For SK channels, this is further complicated by the fact that most inhibitors or activators are not selective for one of the three subtypes. For example, the most commonly used SK channel inhibitor, apamin, shows only some selectivity between SK channel subtypes, with SK2 being the most sensitive (Weatherall et al., 2011). Furthermore, the physiological role of SK channels in the heart is complex. There is evidence suggesting that in human atrial myocytes, activation of SK2-SK3-heteromers contributes to repolarization of the AP. These SK2-SK3-heteromers are insensitive to apamin, but inhibited by the small organic molecule inhibitor UCL1684 (Hancock et al., 2015; Shamsaldeen et al., 2019). Therefore, one cannot reliably extrapolate from observed mRNA levels to SK channel subtype function.
In any case, though, upregulation of ventricular SK channels such as SK2 and SK3 in HF, in the presence of unchanged or downregulated expression in the atria, would suggest reduced potential for atria-selectivity of pharmacological interventions. Based on our mRNA expression data from human tissue samples, only SK1 channels fulfilled the criteria for an atria-selective drug target that is conserved in HF. However, the contribution of SK1 channels to repolarization of the action potential is not well established because functional data are usually obtained with the SK channel inhibitor apamin – to which SK1 channels are least sensitive (Grunnet et al., 2001). This calls for further basic research, using SK1-targeting genetic or pharmacological interventions, to fully evaluate its potential as an atria-selective target.
The number of biological replicates was low, due to the limited quantity of patient material and the exclusion of several samples with low RNA integrity numbers, however, it was ≥ 5 for each sub-goup, which we regard as a lower limit. Higher n-numbers would be needed to assess the question of whether the here reported lack of statistically significant changes in certain RNA levels previously reported to be up- or downregulated (Table 2), offers a generalizable view of electrophysiological remodeling in AF.
All diseased human hearts samples were from male patients while non-diseased samples came in equal parts from male and female individuals. This is due to the fact that the number of patients was limited and we had to prioritize clinical entities, rather than gender. For this reason, we cannot address sex-specific differences in mRNA expression. Sex-related differences in myocardial gene expression have been reported previously (Guo et al., 2017; Inanloorahatloo et al., 2017), so this forms an area for further research.
Right atrial appendage tissue from AF patients is compared to tissue from patients with SR to describe changes in gene expression in patients with AF. While RAA is the human atrial tissue that is most frequently removed during open heart surgery and therefore available for scientific investigation, analysis of gene expression in the LA would be of even greater interest, as AF is most is often associated with electrophysiological abnormalities in LA and pulmonary veins (Haïssaguerre et al., 1998).
In the past, several groups have searched for altered ion channel expression in human atrial tissue in order to explain the typical changes in AP shapes (electrical remodeling) seen in AF (Van Wagoner and Nerbonne, 2000; Dobrev and Ravens, 2003). Changes in expression of mRNA do not necessarily manifest themselves in matching changes in presence and activity of the proteins they encode. A particular gene product may be further modified during translational or even by post-translational processes. Moreover, newly synthetized proteins such as ion channels can remain trapped in the endoplasmic reticulum for some time before they are recruited to their proper functional compartment, the plasmalemma (Schumacher and Martens, 2010). Therefore, the here presented results do not provide insight into the actual electrophysiological remodeling in AF.
In this study, we assess key genes with selective expression in either atria or LV, and their up- or downregulation in AF or HF. We document SK channel expression in atria and LV of hearts from patients with or without structural cardiac disease. We conclude that only SK1, but not SK2 and 3, shows a preferential expression in the atria in non-diseased and diseased hearts, albeit at low expression levels whose functional relevance remains to be assessed. General blockers of SK channels are not likely to have atria-selective effects, and hence may prove to have less utility in treating AF than hoped.
The datasets presented in this study can be found in online repositories. The names of the repository/repositories and accession number(s) can be found below: https://www.ebi.ac.uk/ena/browser/view/PRJEB42485.
The studies involving human participants were reviewed and approved by the Ethics Committee of the University of Freiburg, Freiburg im Breisgau, Germany, the Medical Faculty of the Technical University Dresden, Dresden, Germany, the Medical Faculty of Heidelberg University, Heidelberg, Germany and the Medical Research Council Budapest, Scientific and Research Ethics Committee, Budapest, Hungary. The patients/participants provided their written informed consent to participate in this study.
ED, TN, MS, PK, SR, UR, and RP contributed to the conception, design, and interpretation of the study. ED, UR, and RP drafted the manuscript. ED analyzed the RNA-seq data. FK, CS, FW, and IB provided human heart samples. All authors contributed to manuscript revision, read, and approved the submitted version.
TN, MS, and SR were employed by Amgen Inc., at the time of submission of the manuscript.
The remaining authors declare that the research was conducted in the absence of any commercial or financial relationships that could be construed as a potential conflict of interest.
The authors declare that this study received financial support from the Amgen Inc., via a preclinical research program agreement. Furthermore, the company had the following involvement: payment for sequencing and approval of final manuscript.
We thank the staff and patients of the Department of Cardiovascular Surgery of the University Heart Center Freiburg-Bad Krozingen for access to human tissue. We are grateful to the staff of CardioVascular BioBank in Freiburg im Breisgau for providing human tissue samples. We acknowledge the support of the Freiburg Galaxy Team: Dr. Björn Grüning and Prof. Rolf Backofen, Bioinformatics, University of Freiburg (Germany) funded by the Collaborative Research Centre 992 Medical Epigenetics (DFG grant SFB 992/1 2012) and the German Federal Ministry of Education and Research (BMBF) grant 031 A538A de.NBI-RBC. We also thank Tony Matschulla from the Institute of Experimental and Clinical Pharmacology and Toxicology, Faculty of Medicine, University of Freiburg, and Eike M. Wülfers from the Institute for Experimental Cardiovascular Medicine, University Heart Center Freiburg-Bad Krozingen for their technical support. We acknowledge the support of the Medical Faculty Carl Gustav Carus, TU Dresden, Germany for providing some atrial tissue samples. ED, FK, CS, FW, PK, UR, and RP are members of SFB1425, funded by the German Research Foundation (DFG #422681845). UR is member of the steering committee of the Atrial Fibrillation NETwork (AFNET), Münster, Germany.
The Supplementary Material for this article can be found online at: https://www.frontiersin.org/articles/10.3389/fphys.2021.650964/full#supplementary-material
Supplementary Figure 1 | Flow chart illustrating the inclusion criteria. Number (N°) of human heart tissue samples entered, removed after assessment at different quality control stages, and finally included in the analyses is shown. PCA, Principal component analysis; TPM, transcripts per kilobase million.
Supplementary Figure 2 | mRNA expression of housekeeping genes in transcripts per kilobase million (TPM) assessed by RNA-seq. (A) GAPDH (glyceraldehyde-3-phosphate dehydrogenase), (B) PPIA (peptidylprolyl-isomerase A), and (C) HPRT1 (hypoxanthine-phosphoribosyltransferase 1). Sample provenance: left ventricle (LV; •, right atrial appendage (RAA; ▲), left atrium (LA; ▼). Patient’s health status: non-diseased (ND; green, open symbols); heart failure (HF): pooled data from dilated cardiomyopathy (DCM; light blue) and ischemic cardiomyopathy (ICM; blue); sinus rhythm (SR): pooled data from coronary artery disease (CAD; ocher) and heart valve disease (HVD; orange); atrial fibrillation (AF; red). The mean ± SD data are represented. Statistical significance was assessed by Dunn’s test. For HF and SR, statistical significance was assessed by the Mann–Whitney test.
AF, Atrial fibrillation; AP, Action potential; Ca2+, Calcium; CAD, Coronary artery disease; CVBB, Cardiovascular biobank; DCM, Dilated cardiomyopathy; GIRK, G protein-coupled inwardly rectifying K+ channel; HF, Heart failure; HVD, Heart valve disease; ICM, Ischemic cardiomyopathy; K+, Potassium; K2P channels, Two-pore-domain K+ channels; LA, Left atrium; LV, Left ventricle; mRNA, Messenger RNA; Na+, Sodium; PCA, Principal component analysis; RAA, Right atrial appendage; RNA, Ribonucleic acid; RNA-seq, RNA-sequencing; SD, Standard deviation; SK channel, Ca2+-activated K+ channel of small conductance; SR, Sinus rhythm; TASK-1 channel, TWIK-related acid-sensitive K+ channel 1; TPM, Transcripts per kilobase million; TWIK channels, Tandem of two-pore-domain weak inward rectifying K+ channels.
Afgan, E., Baker, D., Batut, B., Van Den Beek, M., Bouvier, D., Ech, M., et al. (2018). The galaxy platform for accessible, reproducible and collaborative biomedical analyses: 2018 update. Nucleic Acids Res. 46, 537–544. doi: 10.1093/nar/gky379
Amos, G. J., Wettwer, E., Metzger, F., Li, Q., Himmel, H. M., and Ravens, U. (1996). Differences between outward currents of human atrial and subepicardial ventricular myocytes. J. Physiol. 491, 31–50. doi: 10.1113/jphysiol.1996.sp021194
Andrews, S. (2010). FastQC A Quality Control Tool for High Throughput Sequence Data. Available Online at: http://www.bioinformatics.babraham.ac.uk/projects/fastqc/
Batut, B., Freeberg, M. A., Heydarian, M., Erxleben, A., Videm, P., Blank, C., et al. (2020). Reference-Based RNA-Seq Data Analysis (Galaxy Training Materials).” 2020. Available Online at: https://training.galaxyproject.org/training-material/topics/transcriptomics/tutorials/ref-based/tutorial.html#citing-this-tutorial.
Batut, B., Hiltemann, S., Bagnacani, A., Baker, D., Bhardwaj, V., Blank, C., et al. (2018). Community-driven data analysis training for biology. Cell. Syst. 6, 752–758. doi: 10.1016/j.cels.2018.05.012
Bonilla, I. M., Long, V. P., Vargas-Pinto, P., Wright, P., Belevych, A., Lou, Q., et al. (2014). Calcium-activated potassium current modulates ventricular repolarization in chronic heart failure. PLoS One 9:e0108824. doi: 10.1371/journal.pone.0108824
Brundel, B. J. J. M. I, Van Gelder, C., Henning, R. H., Tuinenburg, A. E., Wietses, M., Grandjean, J. G., et al. (2001). Alterations in potassium channel gene expression in atria of patients with persistent and paroxysmal atrial fibrillation: differential regulation of protein and MRNA levels for K+ channels. J. Am. Coll. Cardiol. 37, 926–932. doi: 10.1016/S0735-1097(00)01195-5
Büttner, P., Schumacher, K., Dinov, B., Zeynalova, S., Sommer, P., Bollmann, A., et al. (2018). Role of NT-ProANP and NT-ProBNP in patients with atrial fibrillation: association with atrial fibrillation progression phenotypes. Heart Rhythm 15, 1132–1137. doi: 10.1016/j.hrthm.2018.03.021
Cabiati, M., Raucci, S., Caselli, C., Guzzardi, M. A., D’Amico, A., Prescimone, T., et al. (2012). Tissue-specific selection of stable reference genes for real-time PCR normalization in an obese rat model. J. Mol. Endocrinol. 48, 251–260. doi: 10.1530/JME-12-0024
Chang, P. C., and Chen, P. S. (2015). SK channels and ventricular arrhythmias in heart failure. Trends Cardiovas. Med. 25, 508–514. doi: 10.1016/j.tcm.2015.01.010
Chang, P. C., Hsieh, Y. C., Hsueh, C. H., Weiss, J. N., Lin, S. F., and Chen, P. S. (2013a). apamin induces early afterdepolarizations and torsades de pointes ventricular arrhythmia from failing rabbit ventricles exhibiting secondary rises in intracellular calcium. Heart Rhythm 10, 1516–1524. doi: 10.1016/j.hrthm.2013.07.003
Chang, P. C., Turker, I., Lopshire, J. C., Masroor, S., Nguyen, B. L., Tao, W., et al. (2013b). Heterogeneous upregulation of apamin-sensitive potassium currents in failing human ventricles. J. Am. Heart Assoc. 2:e004713. doi: 10.1161/JAHA.112.004713
Chien, K. R., Knowlton, K. U., Zhu, G., and Chien, S. (1991). Regulation of cardiac gene expression during myocardial growth and hypertrophy: molecular studies of an adaptive physiologic response. FASEB J. 5, 3037–3064. doi: 10.1096/fasebj.5.15.1835945
Chinchilla, A., Daimi, H., Lozano-Velasco, E., Dominguez, J. N., Caballero, R., Delpón, E., et al. (2011). PITX2 insufficiency leads to atrial electrical and structural remodeling linked to arrhythmogenesis. Circ. Cardiovas. Genet. 4, 269–279. doi: 10.1161/CIRCGENETICS.110.958116
Chua, S. K., Chang, P. C., Maruyama, M., Turker, I., Shinohara, T., Shen, M. J., et al. (2011). Small-conductance calcium-activated potassium channel and recurrent ventricular fibrillation in failing rabbit ventricles. Circ. Res. 108, 971–979. doi: 10.1161/CIRCRESAHA.110.238386
Diness, J. G., Kirchhoff, J. E., Speerschneider, T., Abildgaard, L., Edvardsson, N., Sørensen, U. S., et al. (2020). The KCa2 channel inhibitor AP30663 selectively increases atrial refractoriness, converts vernakalant-resistant atrial fibrillation and prevents its reinduction in conscious pigs. Front. Pharmacol. 11:159. doi: 10.3389/fphar.2020.00159
Diness, J. G., Sørensen, U. S., Nissen, J. D., Al-Shahib, B., Jespersen, T., Grunnet, M., et al. (2010). Inhibition of small-conductance Ca2+-activated K+ channels terminates and protects against atrial fibrillation. Circ. Arrhythmia Electrophysiol. 3, 380–390. doi: 10.1161/CIRCEP.110.957407
Dobin, A., Davis, C. A., Schlesinger, F., Drenkow, J., Zaleski, C., Jha, S., et al. (2012). STAR: ultrafast universal RNA-seq aligner. Bioinformatics 29, 15–21. doi: 10.1093/bioinformatics/bts635
Dobrev, D., Graf, E., Wettwer, E., Himmel, H. M., Haìla, O., Doerfel, C., et al. (2001). Molecular basis of downregulation of g-protein–coupled inward rectifying K+ current (IK,ACh) in chronic human atrial fibrillation. Circulation 104, 2551–2557. doi: 10.1161/hc4601.099466
Dobrev, D., and Ravens, U. (2003). Remodeling of cardiomyocyte ion channels in human atrial fibrillation. Basic Res. Cardiol. 98, 137–148. doi: 10.1007/s00395-003-0409-8
Everett, A. W. (1986). Isomyosin expression in human heart in early pre- and post-natal life. J. Mol. Cell. Cardiol. 18, 607–615. doi: 10.1016/S0022-2828(86)80968-3
Ewels, P., Magnusson, M., Lundin, S., and Käller, M. (2016). MultiQC: summarize analysis results for multiple tools and samples in a single report. Bioinformatics 32, 3047–3048. doi: 10.1093/bioinformatics/btw354
Ford, J. W., and Milnes, J. T. (2008). New drugs targeting the cardiac ultra-rapid delayed-rectifier current (ikur): rationale, pharmacology and evidence for potential therapeutic value. J. Cardiovasc. Pharmacol. 52, 105–120. doi: 10.1097/FJC.0b013e3181719b0c
Gaborit, N., Bouter, S. L., Szuts, V., Varro, A., Escande, D., Nattel, S., et al. (2007). Regional and tissue specific transcript signatures of ion channel genes in the non-diseased human heart. J. Physiol. 582, 675–693.
Gaborit, N., Steenman, M., Lamirault, G., Le Meur, N., Le Bouter, S., Lande, G., et al. (2005). Human atrial ion channel and transporter subunit gene-expression remodeling associated with valvular heart disease and atrial fibrillation. Circulation 112, 471–481. doi: 10.1161/CIRCULATIONAHA.104.506857
Goette, A., Arndt, M., Röcken, C., Spiess, A., Staack, T., Geller, J. C., et al. (2000). Regulation of angiotensin ii receptor subtypes during atrial fibrillation in humans. Circulation 101, 2678–2681. doi: 10.1161/01.cir.101.23.2678
Grammer, J. B., Bosch, R. F., Kühlkamp, V., and Seipel, L. (2000). Molecular remodeling of Kv4.3 potassium channels in human atrial fibrillation. J. Cardiovas. Electrophysiol. 11, 626–633. doi: 10.1111/j.1540-8167.2000.tb00024.x
Grunnet, M., Jensen, B. S., Olesen, S. P., and Klaerke, D. A. (2001). Apamin interacts with all subtypes of cloned small-conductance Ca2+-activated K+ channels. Pflugers Arch. Eur. J. Physiol. 441, 544–550. doi: 10.1007/s004240000447
Guo, L., Zhang, Q., Ma, X., Wang, J., and Liang, T. (2017). MiRNA and MRNA expression analysis reveals potential sex-biased MiRNA expression. Sci. Rep. 7, 1–10. doi: 10.1038/srep39812
Haïssaguerre, M., Jaïs, P., Shah, D. C., Takahashi, A., Hocini, M., Quiniou, G., et al. (1998). Spontaneous initiation of atrial fibrillation by ectopic beats originating in the pulmonary veins. New Eng. J. Med. 339, 659–666. doi: 10.1056/nejm199809033391003
Hamilton, S. I, Polina, R. Terentyeva, Bronk, P., Kim, T. Y., Roder, K., Clements, R. T., et al. (2020). PKA phosphorylation underlies functional recruitment of sarcolemmal SK2 channels in ventricular myocytes from hypertrophic hearts. J. Physiol. 598, 2847–2873. doi: 10.1113/JP277618
Hancock, J. M., Weatherall, K. L., Choisy, S. C., James, A. F., Hancox, J. C., and Marrion, N. V. (2015). Selective activation of heteromeric SK channels contributes to action potential repolarization in mouse atrial myocytes. Heart Rhythm 12, 1003–1015. doi: 10.1016/j.hrthm.2015.01.027
Heijman, J., and Dobrev, D. (2017). Inhibition of small-conductance Ca2+-activated K+ channels: the long-awaited breakthrough for antiarrhythmic drug therapy of atrial fibrillation? Circ. Arrhythmia Electrophysiol. 10:e005776. doi: 10.1161/CIRCEP.117.005776
Houweling, A. C., Van Borren, M. M., Moorman, A. F. M., and Christoffels, V. M. (2005). Expression and regulation of the atrial natriuretic factor encoding gene nppa during development and disease. Cardiovas. Res. 67, 583–593. doi: 10.1016/j.cardiores.2005.06.013
Hsueh, C. H., Chang, P. C., Hsieh, Y. C., Reher, T., Chen, P. S., and Lin, S. F. (2013). Proarrhythmic effect of blocking the small conductance calcium activated potassium channel in isolated canine left atrium. Heart Rhythm 10, 891–898. doi: 10.1016/j.hrthm.2013.01.033
Inanloorahatloo, K., Liang, G., Vo, D., Ebert, A., Nguyen, I., and Nguyen, P. K. (2017). Sex-based differences in myocardial gene expression in recently deceased organ donors with no prior cardiovascular disease. PLoS One 12:e0183874. doi: 10.1371/journal.pone.0183874
Jahangir, A., Lee, V., Friedman, P. A., Trusty, J. M., Hodge, D. O., Kopecky, S. L., et al. (2007). Long-term progression and outcomes with aging in patients with lone atrial fibrillation: a 30-year follow-up study. Circulation 115, 3050–3056. doi: 10.1161/CIRCULATIONAHA.106.644484
January, C. T., Wann, L. S., Alpert, J. S., Calkins, H., Cigarroa, J. E., Cleveland, J. C., et al. (2014). 2014 AHA/ACC/HRS guideline for the management of patients with atrial fibrillation: a report of the american college of cardiology/american heart association task force on practice guidelines and the heart rhythm society. J. Am. Coll. Cardiol. 64, e1–e76. doi: 10.1016/j.jacc.2014.03.022
Kirchhof, P., Benussi, S., Kotecha, D., Ahlsson, A., Atar, D., Casadei, B., et al. (2016). 2016 ESC guidelines for the management of atrial fibrillation developed in collaboration with EACTS. Eur. J. Cardiothorac. Surg. 50, e1–e88. doi: 10.1093/ejcts/ezw313
Kirchhof, P., Kahr, P. C., Kaese, S., Piccini, I., Vokshi, I., Scheld, H.-H., et al. (2011). PITX2c Is expressed in the adult left atrium, and reducing Pitx2c expression promotes atrial fibrillation inducibility and complex changes in gene expression. Circ. Cardiovas. Genet. 4, 123–133. doi: 10.1161/CIRCGENETICS.110.958058
Koumi, S. I., and Wasserstrom, J. A. (1994). Acetylcholine-sensitive muscarinic K+ channels in mammalian ventricular myocytes. Am. J.Physiol. 266, 1812–1821. doi: 10.1152/ajpheart.1994.266.5.h1812
Kurabayashi, M., Tsuchimochi, H., Komuro, I., Takaku, F., and Yazaki, Y. (1988). Molecular cloning and characterization of human CARDIAC α- and β-form myosin heavy chain complementary DNA clones. regulation of expression during development and pressure overload in human atrium. J. Clin. Investig. 82, 524–531. doi: 10.1172/JCI113627
Li, B., and Dewey, C. N. (2011). RSEM: accurate transcript quantification from RNA-seq data with or without a reference genome. BMC Bioinform. 12:323. doi: 10.1186/1471-2105-12-323
Li, N., Timofeyev, V., Tuteja, D., Xu, D., Lu, L., Zhang, Q., et al. (2009). Ablation of a Ca2+-activated K+ Channel (SK2 Channel) results in action potential prolongation in atrial myocytes and atrial fibrillation. J. Physiol. 587, 1087–1100. doi: 10.1113/jphysiol.2008.167718
Liao, Y., Smyth, G. K., and Shi, W. (2013). Feature Counts: an efficient general purpose program for assigning sequence reads to genomic features. Bioinformatics 30, 923–930. doi: 10.1093/bioinformatics/btt656
Ling, T. Y., Wang, X. L., Chai, Q., Lau, T. W., Koestler, C. M., Park, S. J., et al. (2013). Regulation of the SK3 channel by microRNA-499 - potential role in atrial fibrillation. Heart Rhythm 10, 1001–1009. doi: 10.1016/j.hrthm.2013.03.005
Love, M. I., Huber, W., and Anders, S. (2014). Moderated estimation of fold change and dispersion for RNA-seq data with DESeq2. Genome Biol. 15:550. doi: 10.1186/s13059-014-0550-8
Macchia, D. D. (1987). Atrial natriuretic factor: a hormone secreted by the heart. Pharm. Weekbl. Sci. 9, 305–314. doi: 10.1007/BF01956510
Marcel, M. (2011). Cutadapt removes adapter sequences from high-throughput sequencing reads. EMBnet. J. 17:200. doi: 10.14806/ej.17.1.200
Martin, R. I. R., Babaei, M. S., Choy, M. K., Owens, W. A., Chico, T. J. A., Keenan, D., et al. (2015). Genetic variants associated with risk of atrial fibrillation regulate expression of PITX2, CAV1, MYOZ1, C9orf3 and FANCC. J. Mol. Cell. Cardiol. 85, 207–214. doi: 10.1016/j.yjmcc.2015.06.005
Mittal, S. (2014). Differentiating paroxysmal from persistent atrial fibrillation: long-term electrocardiographic monitoring is mightier than the clinician. J. Am. Coll. Cardiol. 63, 2849–2851. doi: 10.1016/j.jacc.2014.04.020
Miyata, S., Minobe, W., Bristow, M. R., and Leinwand, L. A. (2000). Myosin heavy chain isoform expression in the failing and nonfailing human heart. Circ. Res. 86, 386–390. doi: 10.1161/01.RES.86.4.386
Molina, C. E., Jacquet, E., Ponien, P., Muñoz-Guijosa, C., Baczkó, I., Maier, L. S., et al. (2018). Identification of optimal reference genes for transcriptomic analyses in normal and diseased human heart. Cardiol. Res. 114, 247–258. doi: 10.1093/cvr/cvx182
Nagy, N., Szuts, V., Horváth, Z., Seprényi, G., Farkas, A. S., Acsai, K., et al. (2009). Does small-conductance calcium-activated potassium channel contribute to cardiac repolarization? J. Mol. Cell Cardiol. 47, 656–663. doi: 10.1016/j.yjmcc.2009.07.019
Nao, T., Ohkusa, T., Hisamatsu, Y., Inoue, N., Matsumoto, T., Yamada, J., et al. (2003). Comparison of expression of connexin in right atrial myocardium in patients with chronic atrial fibrillation versus those in sinus rhythm. Am. J. Cardiol. 91, 678–683. doi: 10.1016/S0002-9149(02)03403-3
Nattel, S., Maguy, A., Le Bouter, S., and Yeh, Y. H. (2007). Arrhythmogenic ion-channel remodeling in the heart: heart failure, myocardial infarction, and atrial fibrillation. Physiol. Rev. 87, 425–456. doi: 10.1152/physrev.00014.2006
Nielsen, J. B., Thorolfsdottir, R. B., Fritsche, L. G., Zhou, W., Skov, M. W., Graham, S. E., et al. (2018). Biobank-driven genomic discovery yields new insight into atrial fibrillation biology. Nat. Genet. 50, 1234–1239. doi: 10.1038/s41588-018-0171-3
Pavri, B. B., Greenberg, H. E., Kraft, W. K., Lazarus, N., Lynch, J. J., Salata, J. J., et al. (2012). MK-0448, a specific Kv1.5 inhibitor safety, pharmacokinetics, and pharmacodynamic electrophysiology in experimental animal models and humans. Circ. Arrhythmia Electrophysiol. 5, 1193–1201. doi: 10.1161/CIRCEP.111.969782
Peyronnet, R., and Ravens, U. (2019). Atria-selective antiarrhythmic drugs in need of alliance partners. Pharmacol. Res. 145:104262. doi: 10.1016/j.phrs.2019.104262
Podd, S. J., Freemantle, N., Furniss, S. S., and Sulke, N. (2016). First clinical trial of specific IKACh blocker shows no reduction in atrial fibrillation burden in patients with paroxysmal atrial fibrillation: pacemaker assessment of BMS 914392 in patients with paroxysmal atrial fibrillation. Europace 18, 340–346. doi: 10.1093/europace/euv263
Ravens, U., and Wettwer, E. (2011). Ultra-rapid delayed rectifier channels: molecular basis and therapeutic implications. Cardiovas. Res. 89, 776–785. doi: 10.1093/cvr/cvq398
Robinson, J. T., Thorvaldsdóttir, H., Winckler, W., Guttman, M., Lander, E. S., Getz, G., et al. (2011). Integrative genomics viewer. Nat. Biotechnol. 29, 24–26.
Saljic, A., Soattin, L., Trachsel, D. S., Boddum, K., and Jespersen, T. (2019). In vivo knockdown of SK3 channels using antisense oligonucleotides protects against atrial fibrillation in rats. J. Mol. Cell. Cardiol. 147, 18–26. doi: 10.1016/j.yjmcc.2020.07.011
Santhanakrishnan, R., Wang, N., Larson, M. G., Magnani, J. W., McManus, D. D., Lubitz, S. A., et al. (2016). Atrial fibrillation begets heart failure and vice versa: temporal associations and differences in preserved versus reduced ejection fraction. Circulation 133, 484–492. doi: 10.1161/CIRCULATIONAHA.115.018614
Schmidt, C., Wiedmann, F., Voigt, N., Zhou, X. B., Heijman, J., Lang, S., et al. (2015). Upregulation of K2P3.1 K+ current causes action potential shortening in patients with chronic atrial fibrillation. Circulation 132, 82–92. doi: 10.1161/CIRCULATIONAHA.114.012657
Schmidt, C., Wiedmann, F., Zhou, X.-B., Heijman, J., Voigt, N., Ratte, A., et al. (2017). Inverse remodelling of K2P3.1 K+ channel expression and action potential duration in left ventricular dysfunction and atrial fibrillation: implications for patient-specific antiarrhythmic drug therapy. Eur. Heart J. 38, 1764–1774. doi: 10.1093/eurheartj/ehw559
Schmitt, M., Cockcroft, J. R., and Frenneaux, M. P. (2003). Modulation of the natriuretic peptide system in heart failure: from bench to bedside? Clin. Sci. 105, 141–160.
Schotten, U., Greiser, M., Benke, D., Buerkel, K., Ehrenteidt, B., Stellbrink, C., et al. (2002). Atrial fibrillation-induced atrial contractile dysfunction: a tachycardiomyopathy of a different sort. Cardiovas. Res. 53, 192–201. doi: 10.1016/S0008-6363(01)00453-9
Schumacher, S. M., and Martens, J. R. (2010). Ion channel trafficking: a new therapeutic horizon for atrial fibrillation. Heart Rhythm 7, 1309–1315. doi: 10.1016/j.hrthm.2010.02.017
Shamsaldeen, Y. A., Culliford, L., Clout, M., James, A. F., Ascione, R., Hancox, J. C., et al. (2019). Role of SK channel activation in determining the action potential configuration in freshly isolated human atrial myocytes from the SKArF study. Biochem. Biophys. Res. Commun. 512, 684–690. doi: 10.1016/j.bbrc.2019.03.074
Shunmugam, S. R., Sugihara, C., Freemantle, N., Round, P., Furniss, S., and Sulke, N. (2018). A double-blind, randomised, placebo-controlled, cross-over study assessing the use of XEN-D0103 in patients with paroxysmal atrial fibrillation and implanted pacemakers allowing continuous beat-to-beat monitoring of drug efficacy. J. Int. Cardiac. Electrophysiol. 51, 191–197. doi: 10.1007/s10840-018-0318-2
Skibsbye, L., Poulet, C., Diness, J. G., Bentzen, B. H., Yuan, L., Kappert, U., et al. (2014). Small-conductance calcium-activated potassium (SK) channels contribute to action potential repolarization in human atria. Cardiovas. Res. 103, 156–167. doi: 10.1093/cvr/cvu121
Thomas, A. M., Cabrera, C. P., Finlay, M., Lall, K., Nobles, M., Schilling, R. J., et al. (2019). Differentially expressed genes for atrial fibrillation identified by rna sequencing from paired human left and right atrial appendages. Physiol. Genom. 51, 323–332. doi: 10.1152/physiolgenomics.00012.2019
Tuteja, D., Xu, D., Timofeyev, V., Lu, L., Sharma, D., Zhang, Z., et al. (2005). Differential expression of small-conductance Ca2+-activated K+ channels SK1, SK2, and SK3 in mouse atrial and ventricular myocytes. Am. J. Physiol. Heart Circ. Physiol. 289, H2714–H2723. doi: 10.1152/ajpheart.00534.2005
Van Wagoner, D. R. (2015). Are SK channels a logical target for treating ventricular arrhythmias? first, do no harm. Trends Cardiovas. Med. 25, 515-516. doi: 10.1016/j.tcm.2015.03.002
Van Wagoner, D. R., and Nerbonne, J. M. (2000). Molecular basis of electrical remodeling in atrial fibrillation. J. Mol. Cell. Cardiol. 32, 1101–1117. doi: 10.1006/jmcc.2000.1147
Walfridsson, H., Anfinsen, O. G., Berggren, A., Frison, L., Jensen, S., Linhardt, G., et al. (2015). Is the acetylcholine-regulated inwardly rectifying potassium current a viable antiarrhythmic target? translational discrepancies of AZD2927 and A7071 in dogs and humans. Europace 17, 473–482. doi: 10.1093/europace/euu192
Wang, L., Wang, S., and Li, W. (2012). RSeQC: quality control of RNA-seq experiments. Bioinformatics 28, 2184–2185. doi: 10.1093/bioinformatics/bts356
Weatherall, K. L., Seutin, V., Liégeois, J. F., and Marrion, N. V. (2011). Crucial role of a shared extracellular loop in apamin sensitivity and maintenance of pore shape of small-conductance calcium-activated potassium (SK) channels. Proc. Natl. Acad. Sci. USA 108, 18494–18499. doi: 10.1073/pnas.1110724108
Xu, Y., Tuteja, D., Zhang, Z., Xu, D., Zhang, Y., Rodriguez, J., et al. (2003). Molecular identification and functional roles of a Ca(2+)-activated K+ channel in human and mouse hearts. J. Biol. Chem. 278, 49085–49094. doi: 10.1074/jbc.M307508200
Yi, S.-L., Liu, X.-J., Zhong, J.-Q., and Zhang, Y. (2014). Role of caveolin-1 in atrial fibrillation as an anti-fibrotic signaling molecule in human atrial fibroblasts.” edited by Ali A. Sovari. PLoS One 9:e85144. doi: 10.1371/journal.pone.0085144
Yu, T., Deng, C., Wu, R., Guo, H., Zheng, S., Yu, X., et al. (2012). Decreased expression of small-conductance Ca2+-activated K+ channels SK1 and SK2 in human chronic atrial fibrillation. Life Sci. 90, 219–227. doi: 10.1016/j.lfs.2011.11.008
Zhou, X., Sun, Q., Voigt, N., Heijman, J., Lang, S., Borggrefe, M., et al. (2014). V859 - contributions of small-conductance Ca2+-activated K+ channels to shortening of action potential duration in human chronic atrial fibrillation (80Th annual meeting of the german cardiac society – cardiac and circulat. Clin. Res. Cardiol. 103, 1–9. doi: 10.1007/s00392-014-1100-9
Keywords: RNA-seq, SK channels, AF marker genes, atria-selective drugs, atrial fibrillation
Citation: Darkow E, Nguyen TT, Stolina M, Kari FA, Schmidt C, Wiedmann F, Baczkó I, Kohl P, Rajamani S, Ravens U and Peyronnet R (2021) Small Conductance Ca2 +-Activated K+ (SK) Channel mRNA Expression in Human Atrial and Ventricular Tissue: Comparison Between Donor, Atrial Fibrillation and Heart Failure Tissue. Front. Physiol. 12:650964. doi: 10.3389/fphys.2021.650964
Received: 08 January 2021; Accepted: 01 March 2021;
Published: 01 April 2021.
Edited by:
Omer Berenfeld, University of Michigan, United StatesReviewed by:
David R. Van Wagoner, Case Western Reserve University, United StatesCopyright © 2021 Darkow, Nguyen, Stolina, Kari, Schmidt, Wiedmann, Baczkó, Kohl, Rajamani, Ravens and Peyronnet. This is an open-access article distributed under the terms of the Creative Commons Attribution License (CC BY). The use, distribution or reproduction in other forums is permitted, provided the original author(s) and the copyright owner(s) are credited and that the original publication in this journal is cited, in accordance with accepted academic practice. No use, distribution or reproduction is permitted which does not comply with these terms.
*Correspondence: Rémi Peyronnet, cmVtaS5wZXlyb25uZXRAdW5pdmVyc2l0YWV0cy1oZXJ6emVudHJ1bS5kZQ==
†These authors have contributed equally to this work
Disclaimer: All claims expressed in this article are solely those of the authors and do not necessarily represent those of their affiliated organizations, or those of the publisher, the editors and the reviewers. Any product that may be evaluated in this article or claim that may be made by its manufacturer is not guaranteed or endorsed by the publisher.
Research integrity at Frontiers
Learn more about the work of our research integrity team to safeguard the quality of each article we publish.