- 1Department of Physiology, School of Medicine, Ankara Medipol University, Ankara, Turkey
- 2TUM School of Life Sciences, Technical University Munich, Freising, Germany
CO2 differs from most other odors by being ubiquitously present in the air animals inhale. CO2 levels of the atmosphere, however, are subject to change. Depending on the landscape, temperature, and time of the year, CO2 levels can change even on shortest time scales. In addition, since the 18th century the CO2 baseline keeps increasing due to the intensive fossil fuel usage. However, we do not know whether this change is significant for animals, and if yes whether and how animals adapt to this change. Most insects possess olfactory receptors to detect the gaseous molecule, and CO2 is one of the key odorants for insects such as the vinegar fly Drosophila melanogaster to find food sources and to warn con-specifics. So far, CO2 and its sensory system have been studied in the context of rotting fruit and other CO2-emitting sources to investigate flies’ response to significantly elevated levels of CO2. However, it has not been addressed whether flies detect and potentially react to atmospheric levels of CO2. By using behavioral experiments, here we show that flies can detect atmospheric CO2 concentrations and, if given the choice, prefer air with sub-atmospheric levels of the molecule. Blocking the synaptic release from CO2 receptor neurons abolishes this choice. Based on electrophysiological recordings, we hypothesize that CO2 receptors, similar to ambient temperature receptors, actively sample environmental CO2 concentrations close to atmospheric levels. Based on recent findings and our data, we hypothesize that Gr-dependent CO2 receptors do not primarily serve as a cue detector to find food sources or avoid danger, instead they function as sensors for preferred environmental conditions.
Introduction
CO2 is released into the atmosphere as a by-product of many natural processes such as organic matter decay or animal metabolic activity. Not surprisingly, many insect species show strong responses to changing CO2 stimuli in their environment (Guerenstein and Hildebrand, 2008), and some use elevated levels of CO2 as cues for locating food sources (Thom et al., 2004; Dekker and Cardé, 2011), oviposition sites (Stange, 1999), or a sign of danger (Suh et al., 2004). CO2 has significant importance for Drosophila melanogaster as rotting fruits, the primary food source of this Drosophila species, emit CO2. It has been shown that different activity states may induce attractive or aversive responses to elevated levels of CO2 in Drosophila. While attraction to elevated levels of CO2 is mediated by IR25a receptor neurons (van Breugel et al., 2018) in the active, flying state associated with foraging, aversion is mediated by the Gr63a/Gr21a neurons (Suh et al., 2004; Jones et al., 2007). The reason flies avoid CO2 remains unclear, however, it has been suggested to help flies avoid dangerous situations by avoiding the odor emitted by groups of stressed flies (aka Drosophila stress odor, dSO).
So far, CO2 and its sensory system have been studied in Drosophila with relatively high levels of CO2 (i.e., 1–5%) with few exceptions (Faucher et al., 2006; Andrea Yao and Carlson, 2010; Bräcker et al., 2013). However, CO2 differs from other olfactory cues by being ubiquitously present in the ambient air of the environment of the animal, and its concentration fluctuates throughout the year (Keeling et al., 2005). Moreover, since the 18th century the CO2 base line keeps increasing due to the intensive fossil fuel usage, exceeding the 400 ppm (0.04%) threshold in 2014 and reaching 414 ppm in July 2020 (Keeling et al., 2005; Figure 1A). However, it is not known whether insects can detect these atmospheric level changes or even react to them behaviorally. Therefore, we have asked whether a role, or possibly even one of the main roles of the fly CO2 receptor is to inform the animal of the ambient concentrations of the gas in its environment allowing them to find their preferred location, similar to ectothermic animals navigating environments of different temperatures (Giraldo et al., 2019). To this end, we gave naïve flies the choice between CO2-free air and atmospheric air (400 ppm CO2) using the T-maze assay, a two-choice olfactory maze (Figure 1B). Surprisingly, flies showed a strong avoidance of the current atmospheric air and preferred the CO2-free side (Figure 1C). We next surgically removed the third segment of the antenna, the main olfactory organ (Vosshall and Stocker, 2007) (Figure 1C), and tested these flies for their preference of CO2-free air. Indeed removal of both antennae completely abolished the flies’ choice between atmospheric air and CO2-free air (Figure 1C). As shown in previous studies, avoidance of elevated levels of CO2 requires co-expression of two gustatory receptors, Gr21a and Gr63a (Jones et al., 2007; Kwon et al., 2007) in olfactory receptor neurons (ORNs) located on those segments of the antenna. Therefore, we tested gr63a mutants for their response to CO2-free vs. atmospheric air. As expected, these mutants showed no preference between 1% CO2 and atmospheric CO2 levels compared to controls that strongly avoided it (Figure 1D). In addition to that, gr63a mutants also completely lost their reaction to CO2-free air as compared to controls that clearly avoided atmospheric levels (Figure 1D). This result demonstrates that the same receptors are used to detect elevated and atmospheric concentrations of CO2. Moreover, these results indicate that flies compare differences at even lowest CO2 concentrations and are capable of detecting atmospheric CO2 levels, which, surprisingly, they appear to find repulsive.
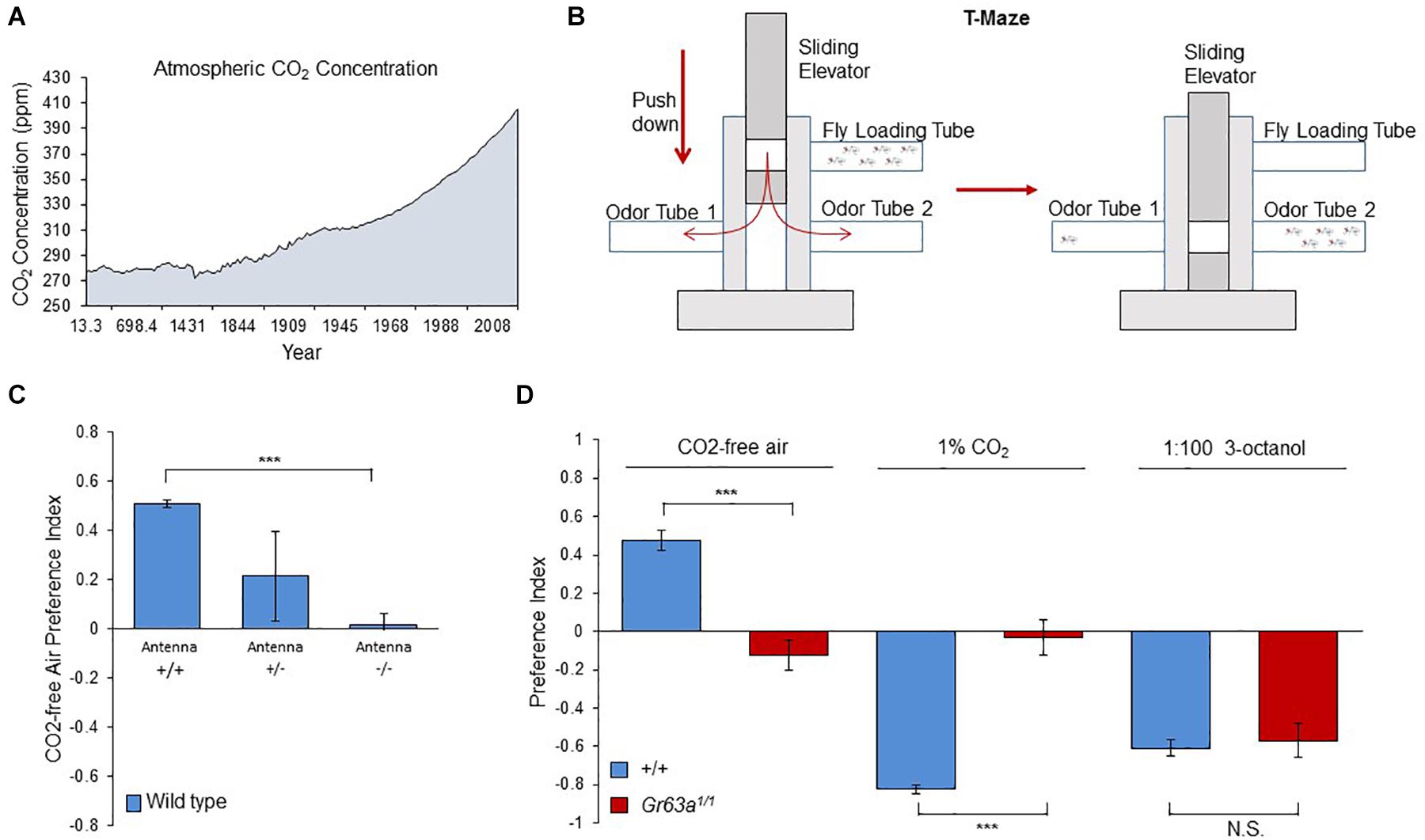
Figure 1. Flies can detect and are attracted to sub-atmospheric CO2 concentrations. (A) The change of atmospheric CO2 concentration as a result of human activities. The graph is drawn by using the merged data of atmospheric CO2 record based on ice core data before 1958 (MacFarling Meure et al., 2006) and yearly averages of direct observations from Mauna Loa and the South Pole after and including 1958 (Keeling et al., 2005). (B) The olfactory T-maze set-up used for olfactory choice behavior. (C) Response of antennaless wild-type flies to CO2-free air over the atmospheric air. Antennaless flies are no longer attracted to CO2-free air (n = 4). (D) Response of gr63a1/1 flies to CO2-free air, 1% CO2 and 3-octanol. gr63a1/1 flies showed no attraction to CO2-free air and no aversion to CO2, while OR-dependent 3-octanol sensitivity is still intact (p = 0.0001, n = 6–8). Significance assessed by T-test (p < 0.001). Error bars represent SEM. (ns > 0.05, *p ≤ 0.05, **p ≤ 0.01, ***p ≤ 0.001).
To further understand how flies can distinguish the small concentration differences between atmospheric CO2 (400 ppm) and 0 ppm CO2, and how the response to CO2-free air is processed at the neural level, we next analyzed the requirement of synaptic output from CO2 sensory neurons. All olfactory neurons send axonal projections to the first olfactory processing center of the fly brain, the antennal lobe (AL) (Vosshall and Stocker, 2007). CO2 sensory neurons project to a particular region of the AL, the V glomerulus. There, they synapse with downstream projection neurons (PNs) that transmit the information to two higher brain centers, the mushroom body and the lateral horn. Additionally, inhibitory as well as excitatory interneurons (LNs) connect glomeruli of different types and likely sharpen the olfactory information content (Wilson, 2013; Figure 2A). Blocking synaptic output of CO2 sensory neurons onto downstream neurons has been shown to abolish avoidance of above atmospheric CO2 concentrations (Suh et al., 2004). To block synaptic output of CO2 sensory neurons, we generated flies that expressed a temperature-sensitive, dominant-negative Dynamin (Shibire, shits1) (Kitamoto, 2001) exclusively in CO2 sensory neurons (see Methods). Shits1 blocks synaptic release transiently at temperatures above ∼30°C. We tested Gr63a > shits1 flies and controls for their response to CO2-free air in the T-maze at restrictive (32°C) and permissive (25°C) temperatures. Similar to gr63a mutants, flies with blocked synaptic output of CO2 sensory neurons showed no avoidance of elevated CO2 concentrations (Figure 2B) and no preference for CO2 -free air compared to controls (Figure 2C). Hence, the presence of the CO2 receptor, Gr63a, as well as synaptic output of the CO2 sensory neuron are essential for mediating the choice between sub-atmospheric and atmospheric CO2 levels. This suggests that CO2 perception at atmospheric levels is mediated by the same sensory neuron and the same downstream neurons as used for the detection of much higher CO2 concentrations.
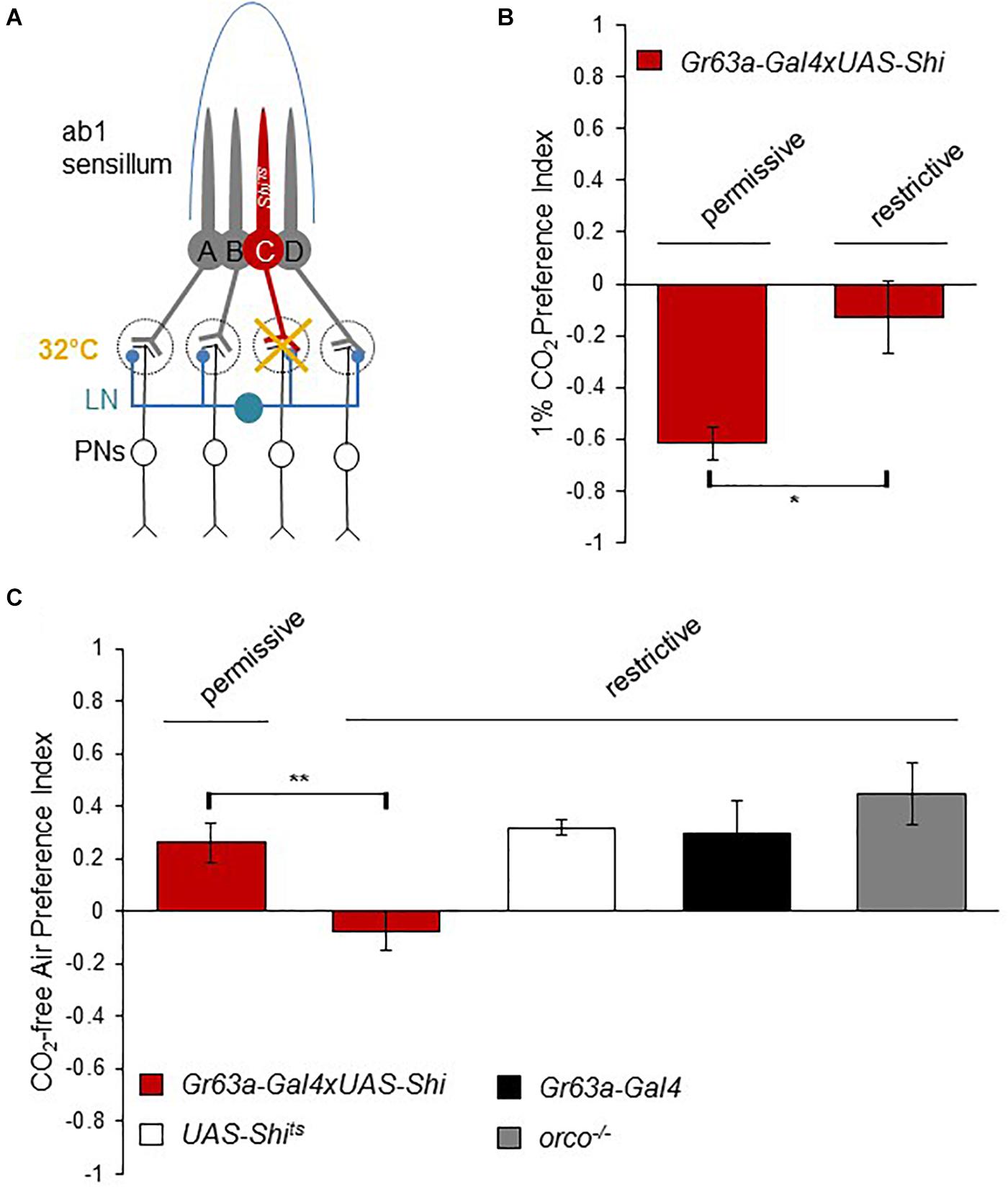
Figure 2. Synaptic release from the CO2 neuron is necessary to detect sub-atmospheric CO2. (A) Neuronal composition of the ab1 sensillum. Neuron C represents the CO2 receptor neuron (ab1C) expressing UAS-shits1 under the control of a Gr63a-Gal4 driver. Synaptic output of ab1C neurons was blocked by shifting flies to 32°C (restrictive) and compared to the behavior of flies tested at 25°C (permissive). (B) Response of Gr63a > shits1 flies to CO2 under permissive and restrictive temperatures. Blocking ab1C neuron output under restrictive temperature reduced CO2 avoidance significantly (p = 0.025, n = 6) compared to permissive controls (n = 4). (C) Response of Gr63a > shits1 flies to CO2.-free air at restrictive and permissive temperature. Similar to their loss of aversion to CO2, flies under restrictive temperature conditions were not attracted to CO2.-free air compared to controls (p = 0.0099, n = 8). Gr63a > shits1 flies under the permissive temperature (n = 6) as well as UAS-shits1 (n = 8), Gr63a-Gal4 (n = 4), and orco1/1 (n = 8) controls showed attraction to CO2.-free air. Significance assessed by T-test. Error bars represent SEM. (ns > 0.05, *p ≤ 0.05, **p ≤ 0.01, ***p ≤ 0.001).
To elucidate the cellular mechanism of how flies can distinguish atmospheric from sub-atmospheric concentrations of CO2 through the Gr21a/Gr63a receptor neurons, we measured the spike frequency of the receptor neurons in extra-cellular single sensilla recordings. CO2 receptor, also called ab1C, neurons are housed in sensilla containing four different receptor neurons (ab1A-D, Figure 3A). The other three neurons depend on OR signaling. To isolate the signal of the CO2 sensory neuron, we recorded sensilla responses to atmospheric air, CO2-free air or 1% CO2 in orco mutant flies as the orco mutation prevents the evoked spiking of OR-dependent neurons. As previously shown (Jones et al., 2007), stimulation of CO2 sensory neurons resulted in a significant increase of the number of spikes compared to non-stimulated, presumably spontaneously firing neurons (Figures 3C,E). In contrast to the increase of spiking upon stimulation with CO2, stimulation with CO2-free air resulted in a transient reduction in firing of the sensory neuron during a 1 second stimulation period as compared to atmospheric air or baseline levels (Figures 3D,F). Upon relief of CO2-free air stimulation, spiking immediately returned to baseline levels (Figure 3D). Taken together, we concluded that CO2 receptors are not only sensitive to relatively high concentrations of CO2 above atmospheric air as previously assumed, but also detect CO2 concentration changes at or below current atmospheric levels. While stimulation with elevated CO2 concentrations results in increased spiking and strong avoidance behavior, stimulation with CO2-free air reduces basal spiking in atmospheric air and leads the fly to avoid atmospheric CO2 and instead approach sub-atmospheric CO2 environments (Figure 3G).
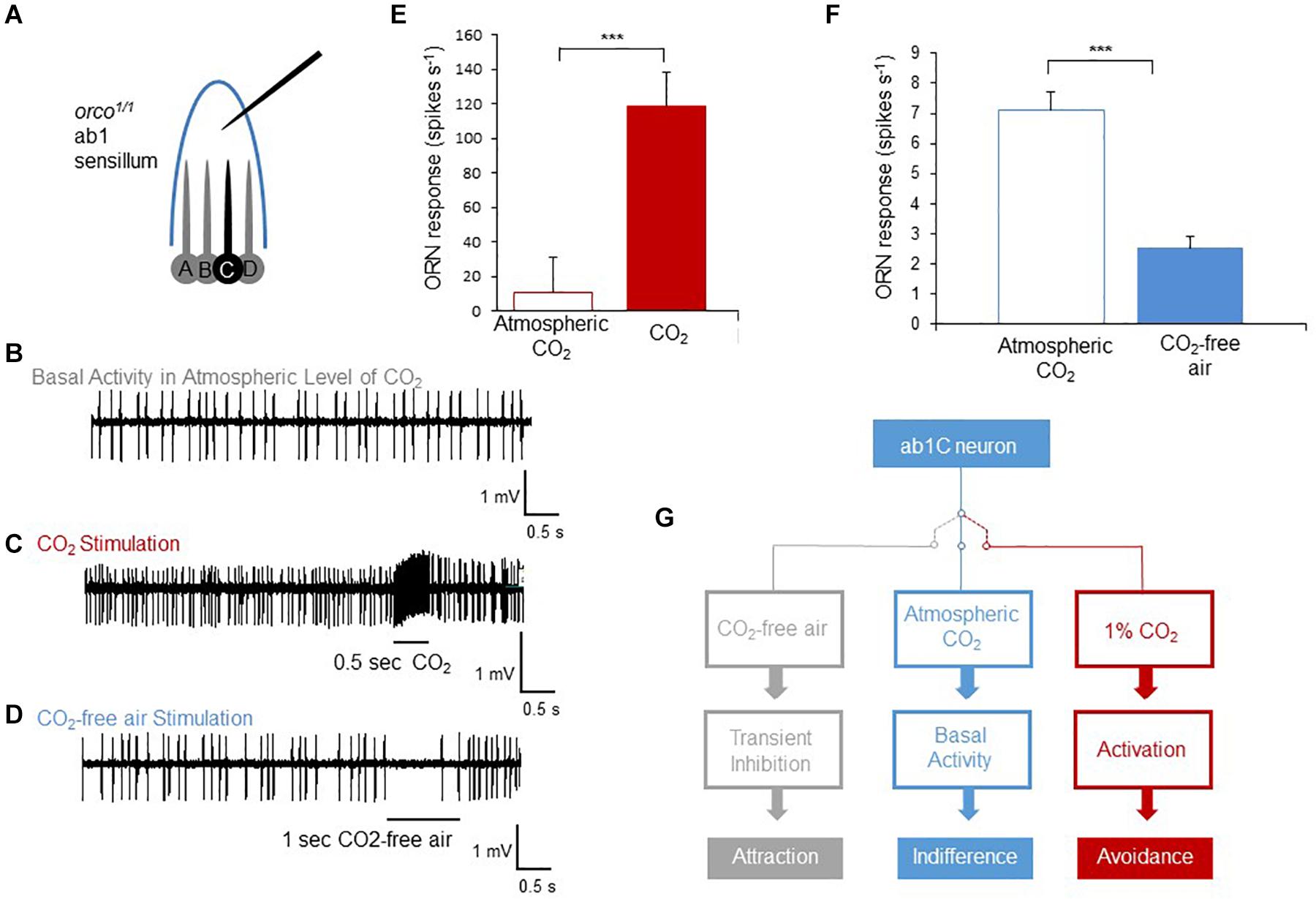
Figure 3. Sub-atmospheric CO2 pulses cause silencing of spontaneous activity in CO2 receptor neurons. (A) Diagram of ab1 single sensillum recording of orco1/1 flies. A,B, and D neurons cannot respond to stimulation due to the orco receptor mutation. (B–D) Representative spike traces of spiking activity of ab1C (CO2) neurons’ spontaneous activity (B), exposed to 1% CO2 (C) and exposed to CO2-free air (D). (E–F) Summary of activity of the ab1C neuron evoked by CO2 (n = 7) and inhibited by CO2-free air (n = 8). The number of generated spikes was significantly reduced when ab1C neurons were exposed to CO2-free air compared to pre-stimulation activity (p < 0.0001, n = 8). Significance assessed by T-test. Error bars represent SEM. (G) Scheme of three activity states of the CO2 neuron and the corresponding behavioral outputs. When the receptor neuron is not exposed to any stimulus, it produces spontaneous spikes resulting in behavioral indifference. Transient inhibition of spontaneous activity by CO2-free air generates attraction. In contrast, activation of the neuron and an increase of spike number cause avoidance behavior as observed for elevated levels of CO2. (ns > 0.05, *p ≤ 0.05, **p ≤ 0.01, ***p ≤ 0.001).
Discussion
Evaluation of ambient CO2 level is crucial for insects as it may signal various cues useful for survival. In this study, we have shown that (i) Drosophila flies avoid current atmospheric levels of CO2 and (ii) CO2 receptors might be useful for other purposes than finding food sources or communicating with conspecifics (i.e., dSO). Thus, our results suggest a so-far not appreciated, novel ethological function for CO2 receptors in the insect olfactory system. Our results show that CO2 receptors in the antennae detect CO2 concentration at or below current atmospheric CO2 levels. We propose that similar to temperature receptors (Barbagallo and Garrity, 2015), CO2 receptors actively sample a relative concentration change of an environmentally ubiquitous cue. Thus, both positive and negative deviations from the existing atmospheric concentration are represented by the activity of the CO2 receptor neuron. While lower than atmospheric CO2 concentrations appear to reduce receptor neuron activity, higher CO2 concentrations lead to increased spiking. Accordingly, a relative decrease in firing explains the observed preference for sub-atmospheric CO2 levels.
At this point, we do not know whether the (basal) firing rate of the CO2 receptor neurons in atmospheric air represents spontaneous or evoked activity. Different from other OSNs, Gr-dependent CO2 receptor neurons undergo constant receptor-ligand interaction due to the atmospheric/ambient existence of CO2, making it challenging to differentiate stimulation-evoked from spontaneous activity. Spontaneous activity appears to be a characteristic of olfactory receptor neurons in Drosophila (Wilson, 2013; Wicher and Miazzi, 2021) and various internal (Andrea Yao and Carlson, 2010) and external (Joseph et al., 2012; Cao et al., 2017) factors can change the rate. For instance, increases in temperature increase the basal firing rate, while decreases in temperature decrease it baseline spiking in insect ORNs (Joseph et al., 2012; Cao et al., 2017). It has also been shown that overexpression of a constitutively active G-protein Gαq counteracts and persistently inhibits both the basal and evoked activity of CO2 receptor neurons (Andrea Yao and Carlson, 2010). Similarly, certain odorants can transiently inhibit basal CO2 receptor neuron activity driving an opposite behavior than if the receptor neuron is depolarized (Cao et al., 2017). On the other hand, antagonists of CO2 and other ORNs have been described which can inhibit activity lastingly and thereby suppress behavior rather than evoking it (Turner and Ray, 2009; Turner et al., 2011). While we cannot pinpoint the exact mechanism at this point, the data presented here suggests that a reduction of the CO2 receptor neuron activity observed at atmospheric air is a salient change for the animal sufficient to promote a preference for sub-atmospheric CO2 concentrations.
Our data further shows that the attraction to CO2-free air, as well as the reduction of atmospheric CO2 receptor neuron activity was unaffected in orco or ato mutants (Supplementary Figure 1) suggesting that evoked activity of other chemosensory neurons is dispensable. Therefore, lateral inhibition, dependent on the activity of neighboring OR neurons within the same sensillum described in a previous Drosophila study (Su et al., 2012), is likely not involved.
We also showed that silencing the synaptic output of CO2 sensory neurons abolishes the preference for CO2-free air over atmospheric air. This argues that synaptic transmission of PNs and/or LNs downstream is essential. Due to the non-linear activation of PNs by sensory neurons, PNs are more sensitive to small changes in presynaptic input when receptor neurons fire at a low rate (Kazama and Wilson, 2008). Thus, at the PN level, a reduction of basal activity by a few spikes could have as strong an effect as the several-fold increase of spiking of the receptor neuron.
It has been shown in a previous study that flies are attracted to CO2 in an IR25a dependent manner. How can an odor be both attractive and aversive for the same animal? In this study we have shown that Gr63a/Gr21a CO2 detecting neurons mediate preference for CO2-free air through a transient reduction of Gr63a/Gr21a ORN firing. Altogether, we propose that flies distribute the CO2 detection task into two channels. In the first channel, they use the IR25a ORNs to find food sources and these neurons mediate an attraction when stimulated. On the other hand, in the second channel, Drosophila use Gr63a/Gr21a ORNs to find a habitable place, preferably low in its CO2 content. Up or down oscillation in the activity of these neurons generate aversion or attraction, respectively. However, how the animal switches between these two channels depending on changing needs (looking for food versus habitation area) still remains unclear. Understanding the circuit mechanism of the Gr-dependent CO2 channel and conditional switch to the IR-dependent CO2 channel may provide a novel perspective of ethologically relevant decision-making mechanisms.
From the ecological perspective, understanding the CO2 detection mechanism of Drosophila and its particular preference for low-atmospheric concentrations may provide an entry point for studies on differences between the habitat selections of other Drosophila species. Moreover, further research can increase our understanding of whether the atmospheric CO2 concentrations have a role in the distribution of both Drosophila and other insect species, particularly disease transmitting ones, and may even provide tools to predict the impacts of climate change on the habitat selection of these insects.
Methods
Fly Genetics
D. melanogaster flies were raised on standard corn meal fly food at controlled light and temperature conditions. The following genotypes were used: Figure 1B: (1) Gr63a1/1; Figure 2C and Supplementary Figure 1: (2) orco1/1; Figures 3B,C: (2) Gr63a-Gal4 (3) UAS-shits1 (4) Gr63a-Gal4/UAS-shits1. atonal mosaic mutant and mosaic control flies (eyflp; FRT82B CL/FRT82B atow and eyflp; FRT82B CL/FRT82B) were generated by crossing flies carrying eyflp; FRT82B CL to FRT82B atow or FRT82B flies, respectively. The promoter of the eyeless gene drives the expression of FLP recombinase selectively in the eye-antennal disc.
Behavior
Flies (D. melanogaster) were maintained at 25°C with 60% humidity under a 12 h light:12 h dark cycle except for shibire experiments. In shibire experiments flies were reared at 18°C and transferred to 25°C after eclosion. CantonS strain was used as wild type. In all behavioral experiments, animals were food-deprived 30 h prior to the experiment and were kept on humidified tissue paper. 6–8-day-old animals were tested in groups of 40–60 in a standard non-aspirated T-maze in red light at the same time of the day. CO2 stimulus tubes were prepared by mixing air and pure CO2 (Westfalen Gas) through mass flow controllers (Natec sensors). Atmospheric air contained 400 ppm or 0.04% CO2. CO2-free stimulus tubes were prepared by directly filling them from gas bottles with CO2-free air (Westfalen Gas). 3-octanol was diluted in paraffin oil and applied onto filter paper (40 μl) in the test tube. The preference index was calculated by subtracting the number of flies on the air side from the odor side and dividing the result by the total number of flies. All data was analyzed using students T-test and GraphPad Prism software.
Single Sensillum Recordings
Extracellular recordings of Drosophila olfactory sensilla were carried out as described (Hartl et al., 2011). Female flies were recorded at 6–8 days after eclosion. All recordings were performed at the same time of the day. A fly was trapped in a truncated pipette tip with its antenna protruding and mounted on a glass slide. For recording, the antenna was trapped on a coverslip with a glass micropipette. A constant flow of humidified air was provided to the head area of the fly. The reference electrode was placed into the eye. The recording electrode was inserted into the antennal basiconic sensilla containing CO2-responsive ab1C neurons. Both the reference and the recording electrodes were filled with 0.01 M KCl. CO2-free air and 1% CO2 stimulations were carried out by a custom-made odor delivery system (Smartec, Martinsried). Each sensillum was stimulated first with CO2-free air for 1 s, and afterward with 1% CO2 to confirm neuronal identity with an inter-stimulus interval of at least 60 s. Spontaneous and odor-evoked/inhibited extracellular spikes were recorded using a CV-7B headstage and MultiClamp 700B amplifier (Molecular Devices). The recordings were sampled at 10 kHz, digitized and fed into a computer via Digidata 1440A. The action potential spikes were recorded with Clampex 10.2 software and spike sorting and analysis were done manually with Clampfit 10.2 and MS Excel software, respectively. For the calculation of spikes, spontaneous activity was not subtracted from the odor induced/inhibited spikes. One sensillum was sampled from each animal mounted.
Data Availability Statement
The raw data supporting the conclusions of this article will be made available by the authors, without undue reservation.
Author Contributions
HÜ carried out all experiments in this study. HÜ and IG conceived the study, interpreted the results, and wrote the manuscript. Both authors contributed to the article and approved the submitted version.
Funding
This work was supported by Max Planck Society, Boehringer Ingelheim Exploration Grant to IG and ERC starting grant FlyContext to IG.
Conflict of Interest
The authors declare that the research was conducted in the absence of any commercial or financial relationships that could be construed as a potential conflict of interest.
Supplementary Material
The Supplementary Material for this article can be found online at: https://www.frontiersin.org/articles/10.3389/fphys.2021.646401/full#supplementary-material
Supplementary Figure 1 | (A) Response of ato-/- flies to CO2-free air. IR mutant ato-/- flies did not differ in their response to CO2-free air over the atmospheric air (p = 0.6701, n = 8). (B) Response of orco1/1 flies to CO2-free air versus atmospheric air, 1:20 3-octanol and 1:100 3-octanol. While losing their sensitivity to 3-octanol, orco1/1 flies did not differ in their response to CO2-free air over atmospheric air (p = 0.2227, n = 10). Significance assessed by Student’s t test. Error bars represent SEM.
References
Andrea Yao, C., and Carlson, J. R. (2010). Role of G-proteins in odor-sensing and CO2-sensing neurons in Drosophila. J. Neurosci. 30, 4562–4572. doi: 10.1523/JNEUROSCI.6357-09.2010
Barbagallo, B., and Garrity, P. A. (2015). Temperature sensation in Drosophila. Curr. Opin. Neurobiol. 34, 8–13. doi: 10.1016/j.conb.2015.01.002
Bräcker, L. B., Siju, K. P., Varela, N., Aso, Y., Zhang, M., Hein, I., et al. (2013). Essential role of the mushroom body in context-dependent CO2 avoidance in drosophila. Curr. Biol. 23, 1228–1234. doi: 10.1016/j.cub.2013.05.029
Cao, L. H., Yang, D., Wu, W., Zeng, X., Jing, B. Y., Li, M. T., et al. (2017). Odor-evoked inhibition of olfactory sensory neurons drives olfactory perception in Drosophila. Nat. Commun. 8:1357. doi: 10.1038/s41467-017-01185-0
Dekker, T., and Cardé, R. T. (2011). Moment-to-moment flight manoeuvres of the female yellow fever mosquito (Aedes aegypti L.) in response to plumes of carbon dioxide and human skin odour. J. Exp. Biol. 214, 3480–3494. doi: 10.1242/jeb.055186
Faucher, C., Forstreuter, M., Hilker, M., and De Bruyne, M. (2006). Behavioral responses of Drosophila to biogenic levels of carbon dioxide depend on life-stage, sex and olfactory context. J. Exp. Biol. 209, 2739–2748. doi: 10.1242/jeb.02297
Giraldo, D., Adden, A., Kuhlemann, I., Gras, H., and Geurten, B. R. H. (2019). Correcting locomotion dependent observation biases in thermal preference of Drosophila. Sci. Rep. 9:3974. doi: 10.1038/s41598-019-40459-z
Guerenstein, P. G., and Hildebrand, J. G. (2008). Roles and effects of environmental carbon dioxide in insect life. Annu. Rev. Entomol. 53, 161–178. doi: 10.1146/annurev.ento.53.103106.093402
Hartl, M., Loschek, L. F., Stephan, D., Siju, K. P., Knappmeyer, C., and Kadow, I. C. G. (2011). A new prospero and microRNA-279 pathway restricts CO2 receptor neuron formation. J. Neurosci. 31, 15660–15673. doi: 10.1523/JNEUROSCI.2592-11.2011
Jones, W. D., Cayirlioglu, P., Grunwald Kadow, I., and Vosshall, L. B. (2007). Two chemosensory receptors together mediate carbon dioxide detection in Drosophila. Nature 445, 86–90. doi: 10.1038/nature05466
Joseph, J., Dunn, F. A., and Stopfer, M. (2012). Spontaneous olfactory receptor neuron activity determines follower cell response properties. J. Neurosci. 32, 2900–2910. doi: 10.1523/JNEUROSCI.4207-11.2012
Kazama, H., and Wilson, R. I. (2008). Homeostatic matching and nonlinear amplification at identified central synapses. Neuron 58, 401–413. doi: 10.1016/j.neuron.2008.02.030
Keeling, C. D., Piper, S. C., Bacastow, R. B., Wahlen, M., WhorfMartin, T. P., Meijer, H. A., et al. (2005). “Atmospheric CO2 and 13CO2 exchange with the terrestrial biosphere and oceans from 1978 to 2000: observations and carbon cycle implications,” in A History of Atmospheric CO2 and its Effects on Plants, Animals, and Ecosystems, eds I. T. Baldwin, M. M. Caldwell, G. Heldmaier, et al. (New York, NY: Springer New York), 83–113. doi: 10.1007/0-387-27048-5_5
Kitamoto, T. (2001). Conditional modification of behavior in Drosophila by targeted expression of a temperature-sensitive shibire allele in defined neurons. J. Neurobiol. 47, 81–92. doi: 10.1002/neu.1018
Kwon, J. Y., Dahanukar, A., Weiss, L. A., and Carlson, J. R. (2007). The molecular basis of CO2 reception in Drosophila. Proc. Natl. Acad. Sci. U.S.A. 104, 3574–3578. doi: 10.1073/pnas.0700079104
MacFarling Meure, C., Etheridge, D., Trudinger, C., Steele, P., Langenfelds, R., van Ommen, T., et al. (2006). Law dome CO2, CH4 and N2O ice core records extended to 2000 years BP. Geophys. Res. Lett. 33:L14810. doi: 10.1029/2006GL026152
Stange, G. (1999). Carbon dioxide is a close-range oviposition attractant in the Queensland fruit fly Bactrocera tryoni. Naturwissenschaften 86, 190–192. doi: 10.1007/s001140050595
Su, C.-Y., Menuz, K., Reisert, J., and Carlson, J. R. (2012). Non-synaptic inhibition between grouped neurons in an olfactory circuit. Nature 492, 66–71. doi: 10.1038/nature11712
Suh, G. S. B., Wong, A. M., Hergarden, A. C., Wang, J. W., Simon, A. F., Benzer, S., et al. (2004). A single population of olfactory sensory neurons mediates an innate avoidance behaviour in Drosophila. Nature 431, 854–859. doi: 10.1038/nature02980
Thom, C., Guerenstein, P. G., Mechaber, W. L., and Hildebrand, J. G. (2004). Floral CO2 reveals flower profitability to moths. J. Chem. Ecol. 30, 1285–1288. doi: 10.1023/B:JOEC.0000030298.77377.7d
Turner, S. L., and Ray, A. (2009). Modification of CO2 avoidance behaviour in Drosophila by inhibitory odorants. Nature 461, 277–281. doi: 10.1038/nature08295
Turner, S. L., Li, N., Guda, T., Githure, J., Cardé, R. T., and Ray, A. (2011). Ultra-prolonged activation of CO2-sensing neurons disorients mosquitoes. Nature 474, 87–91. doi: 10.1038/nature10081
van Breugel, F., Huda, A., and Dickinson, M. H. (2018). Distinct activity-gated pathways mediate attraction and aversion to CO2 in Drosophila. Nature 564, 420–424. doi: 10.1038/s41586-018-0732-8
Vosshall, L. B., and Stocker, R. F. (2007). Molecular architecture of smell and taste in Drosophila. Annu. Rev. Neurosci. 30, 505–533. doi: 10.1146/annurev.neuro.30.051606.094306
Wicher, D., and Miazzi, F. (2021). Functional properties of insect olfactory receptors: ionotropic receptors and odorant receptors. Cell Tissue Res. 383, 7–19. doi: 10.1007/s00441-020-03363-x
Keywords: Drosophila, olfactory system, Gr21a, carbon dioxide, odors, insect
Citation: Üçpunar HK and Grunwald Kadow IC (2021) Flies Avoid Current Atmospheric CO2 Concentrations. Front. Physiol. 12:646401. doi: 10.3389/fphys.2021.646401
Received: 04 January 2021; Accepted: 22 March 2021;
Published: 13 April 2021.
Edited by:
Eoin P. Cummins, University College Dublin, IrelandReviewed by:
Carlotta Martelli, Johannes Gutenberg University Mainz, GermanyThomas Baker, Pennsylvania State University (PSU), United States
Copyright © 2021 Üçpunar and Grunwald Kadow. This is an open-access article distributed under the terms of the Creative Commons Attribution License (CC BY). The use, distribution or reproduction in other forums is permitted, provided the original author(s) and the copyright owner(s) are credited and that the original publication in this journal is cited, in accordance with accepted academic practice. No use, distribution or reproduction is permitted which does not comply with these terms.
*Correspondence: Ilona C. Grunwald Kadow, aWxvbmEuZ3J1bndhbGRAdHVtLmRl; Habibe K. Üçpunar, aGFiaWJlLnVjcHVuYXJAYW5rYXJhbWVkaXBvbC5lZHUudHI=