Muscle Microbiopsy to Delineate Stem Cell Involvement in Young Patients: A Novel Approach for Children With Cerebral Palsy
- 1Shirley Ryan AbilityLab, Chicago, IL, United States
- 2Department of Physical Medicine and Rehabilitation, Northwestern University, Chicago, IL, United States
- 3Hines VA Medical Center, Maywood, IL, United States
A Commentary on
Muscle Microbiopsy to Delineate Stem Cell Involvement in Young Patients: A Novel Approach for Children With Cerebral Palsy
by Corvelyn, M., De Beukelaer, N., Duelen, R., Deschrevel, J., Van Campenhout, A., Prinsen, S., et al. (2020). Front. Physiol. 11:945. doi: 10.3389/fphys.2020.00945
Introduction
We appreciate the opportunity to comment on the above-referenced article from our colleagues in Belgium. Our goals are (Domenighetti et al., 2018) to provide important background for this and related studies and (Lieber et al., 2003) to opine regarding apparent differences between this work and our previously published paper on the same topic (Domenighetti et al., 2018) (Figure 1).
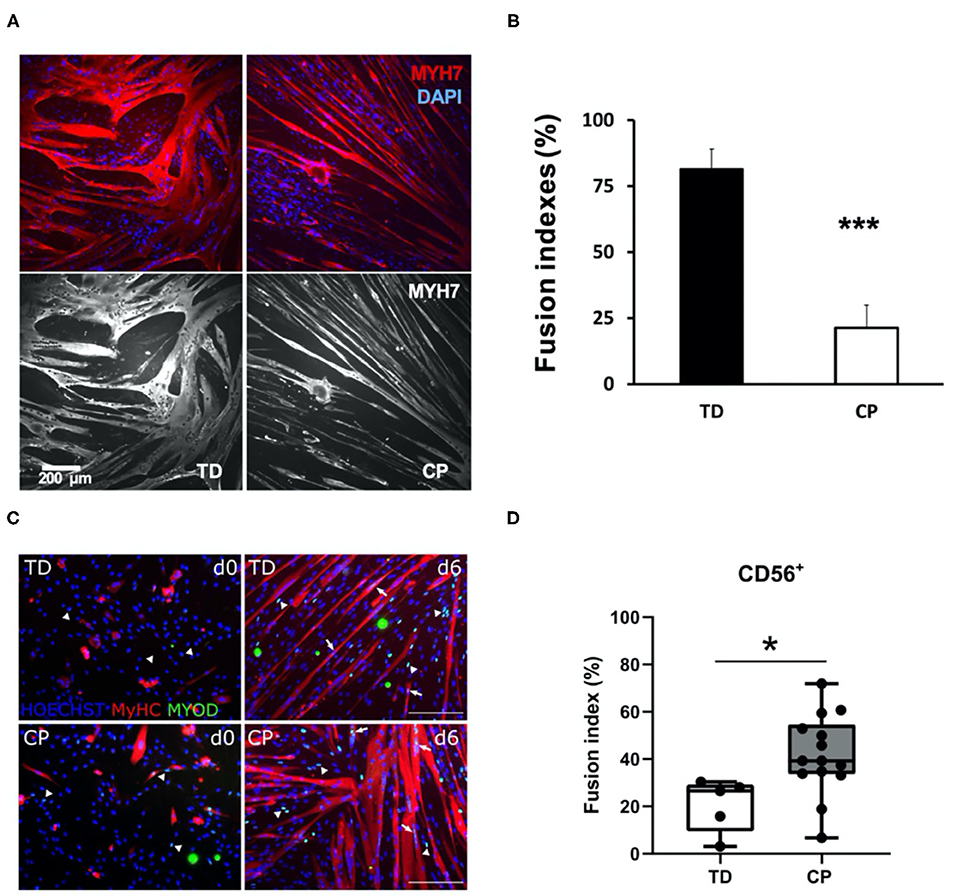
Figure 1. Comparison of immunohistochemical appearance and fusion indices between studies. (A) Myoblasts from CP and TD preparations were differentiated for 42 h and stained for slow myosin heavy chain (MYH7) and a nuclear stain (4-,6-diamidino-2-phenylindole, DAPI). CP myotubes appeared spindly, thin, and with fewer nuclei per myo- tube. Gray scale panels show MYH7 staining as a single channel. (B) Quantification of fusion index for CP and TD myoblasts. Quantification was performed after 42 h of differentiation; ***CP vs. TD, P < 0.001 (n = 8 per group). Figures from Domenighetti et al. (2018). (C) Representative immunofluorescence from satellite cell-derived progenitors of a TD child and a CP patient at days 0 and 6 of myogenic differentiation. MYOD+ nuclei (green) are highlighted by arrows when included into myotubes and by arrowheads if not yet fused; MyHC (red) and nuclei are counterstained by HOECHST (blue). Scale bar: 200 μm. (D) Fusion index (FI) values are represented by boxplots and dots represent individual subjects (TD: n = 5; CP: n = 14; *p < 0.05). Figures from Corvelyn et al. (2020).
To begin, we would like to state that we agree with the authors that the study of muscle growth and development (myogenesis) in cerebral palsy (CP) is an important and timely topic and that performing these studies (which requires direct access to human muscle tissue) is extremely challenging. We previously performed a number of these studies (Lieber et al., 2003; Smith et al., 2009, 2011, 2012, 2013; Dayanidhi et al., 2015; Domenighetti et al., 2018), including three relevant investigations on resident muscle stem cell homeostasis in contractured CP muscle (Smith et al., 2013; Dayanidhi et al., 2015; Domenighetti et al., 2018). Our studies were performed in children with spastic CP of an average age of 9, 11, and 13 years respectively, and with motor dysfunction rankings I–V of the Gross Motor Function Classification System (GMFCS). Some limitations of our studies included low sample sizes (n = 6–10 children per group) and age differences between groups in two of these studies, with children with CP being a few years younger than typically developing (TD) control children who donated muscle tissue during ACL reconstruction surgery (average age of ~14 years) (Smith et al., 2013; Domenighetti et al., 2018).
Corvelyn et al. (2020) are to be congratulated for circumventing some of these biases by developing a method to obtain a non-intraoperative but smaller (~10 mg) muscle biopsy by needle (Bergstrom, 1975), from less severely affected (GMFCS I–III) and generally younger children (average ~6 years of age). Unfortunately, their study lacked clarity regarding the actual muscle stem cell population being studied. This is based on the method chosen to extract myogenic cells from the very small biopsy and the need to expand and passage these cells multiple times before FACS analysis. As such, without further characterization of these cells, it is very difficult to interpret their results in light of muscle stem cell biology.
Satellite Cell Homeostasis in Children With CP
While multiple resident and non-resident stem cells can support myogenesis, including fibroadipogenic stem cells (FAPs) (Joe et al., 2010), pericytes (Crisan et al., 2008), and other cell types (Brack and Rando, 2012), the actual primary self-renewing stem cell that proliferates, differentiates and fuses to produce a muscle fiber is the PAX7-expressing satellite cell (SC) (Schultz and McCormick, 1994; Brack and Rando, 2012; Yin et al., 2013). Muscle SCs are absolutely required for post-natal muscle development and growth (Cardasis and Cooper, 1975; White et al., 2010; Delhaas et al., 2013; Duddy et al., 2015; Gattazzo et al., 2020). When resident SC number or rates of myogenesis are decreased in a non-physiological manner during postnatal development, it leads to impaired muscle growth or recovery from contracture (Murach et al., 2017; Bachman et al., 2018; Chang et al., 2019; Dayanidhi et al., 2020). We and others showed that the PAX7-expressing SC pool is significantly reduced in contractured hamstrings and biceps of 3–18 years old children with CP (Smith et al., 2013; Dayanidhi et al., 2015; Von Walden et al., 2018). We also showed that myoblast progenitors derived from the same SCs (enzymatically extracted from contractured hamstring muscles and FACS-sorted as CD34−, CD45−, CD56+ cells) had decreased capacity to fuse and to differentiate into myotubes in vitro (Domenighetti et al., 2018). This result is in apparent contrast to what Corvelyn et al. observed with their mixed CD56+ cell populations (Figure 1).
Different Experimental Methods to Isolate Myogenic Stem Cells
Based on their very small tissue sizes, Corvelyn et al. were forced to allow cells to grow out of the biopsy onto a culture dish for several days (see their Figure 1), and then expand them in vitro for several passages to obtain sufficient numbers of cells to sort into CD56+ and CD56− populations by FACS. There are two major drawbacks with this approach: First, is lack of control over which cells (and in what percentages) will colonize the plate and continue to expand over several passages, leading to highly variable cultures. Second, without preplating (Yoshioka et al., 2020), growth of primary myogenic cultures over time will result in non-myogenic enriched populations of cells (Rando and Blau, 1994), while SCs will differentiate into myoblasts and rapidly lose their potential to self-renew and contribute to muscle fiber formation (Cosgrove et al., 2009). These limitations necessitated a longitudinal characterization of cell types at the time of plate colonization and then during amplification. While Corvelyn et al. confirmed presence of CD56+ and MYOD+ myogenic cells in vitro (their Supplementary Figure 3A), cell sorting should have included additional negative selection markers and a verification that a sampling of CD56+ sorted cells were also PAX7+. This was not done and the amplified cell type(s) are not known.
Thus, we believe that apparent biological differences between our two studies (Figure 1) are mainly caused by a lower-than-expected myogenic potential of cell cultures in Corvelyn et al. Modest upregulation of myosin heavy chain (MyHC) during 6 days of differentiation is indicative of this phenotype (see their Figure 3A). Furthermore, myogenic potential of their TD CD56+ cultures (~20% fusion index after 6 days of differentiation) is significantly lower than expected for human SC-derived myoblasts (60–80% fusion indices after 24–48 h of differentiation) (Fischer-Lougheed et al., 2001; Cerletti et al., 2006; Agley et al., 2017; Catteau et al., 2020). Thus, our provisional interpretation of their data is not that CP cells were more myogenic than TD but that, for unexplained reasons, the TD cells were grossly underperforming. It is also possible that muscle-specific differences in the differentiation ability of isolated myogenic progenitors could also have contributed for some of the differences observed between our studies. However, since both of these muscles have the same embryonic origin, we think this unlikely (Zammit, 2008).
Discussion
Our goal in this commentary was to provide background insight into the complexity of performing such in vitro experiments from tissue extracted from young children, and the resulting difficulty interpretating results when the cellular identity is not clear. We congratulate the authors on completing a very difficult study and offer this critique in the spirit of improving all of our experiments and with the hope of uncovering new insights into etiology and treatment of cerebral palsy. Specifically, we believe that the optimization of techniques for SC isolation from small muscle biopsies of very young children (e.g., 0–3 years old) will significantly improve our understanding of early/developmental biological mechanisms that lead to motor and muscle impairments (including contracture development) in CP. We welcome continued development of these techniques of SC isolation.
Author Contributions
RL and AD conceived, wrote, edited, and approved this commentary. Both authors contributed to the article and approved the submitted version.
Funding
This work was supported by NIH National Institute of Arthritis and Musculoskeletal and Skin Diseases Grants P30 AR-061303 and R01 AR-057393 and the Shirley Ryan AbilityLab. This work was supported by Research Career Scientist Award (Award Number IK6 RX003351) from the United States (U.S.) Department of Veterans Affairs Rehabilitation R&D (Rehab RD) Service.
Conflict of Interest
The authors declare that the research was conducted in the absence of any commercial or financial relationships that could be construed as a potential conflict of interest.
References
Agley, C. C., Lewis, F. C., Jaka, O., Lazarus, N. R., Velloso, C., Francis-West, P., et al. (2017). Active GSK3beta and an intact beta-catenin TCF complex are essential for the differentiation of human myogenic progenitor cells. Sci. Rep. 7:13189. doi: 10.1038/s41598-017-10731-1
Bachman, J. F., Klose, A., Liu, W., Paris, N. D., Blanc, R. S., Schmalz, M., et al. (2018). Prepubertal skeletal muscle growth requires Pax7-expressing satellite cell-derived myonuclear contribution. Development 145:dev167197. doi: 10.1242/dev.167197
Bergstrom, J. (1975). Percutaneous needle biopsy of skeletal muscle in physiological and clinical research. Scand. J. Clin. Lab. Investigation 35, 609–616. doi: 10.3109/00365517509095787
Brack, A. S., and Rando, T. A. (2012). Tissue-specific stem cells: lessons from the skeletal muscle satellite cell. Cell Stem Cell 10, 504–514. doi: 10.1016/j.stem.2012.04.001
Cardasis, C. A., and Cooper, G. W. (1975). An analysis of nuclear numbers in individual muscle fibers during differentiation and growth: a satellite cell-muscle fiber growth unit. J. Exp. Zool. 191, 347–358. doi: 10.1002/jez.1401910305
Catteau, M., Gouzi, F., Blervaque, L., Passerieux, E., Blaquiere, M., Ayoub, B., et al. (2020). Effects of a human microenvironment on the differentiation of human myoblasts. Biochem. Biophys. Res. Commun. 525, 968–973. doi: 10.1016/j.bbrc.2020.03.020
Cerletti, M., Molloy, M. J., Tomczak, K. K., Yoon, S., Ramoni, M. F., Kho, A. T., et al. (2006). Melanoma cell adhesion molecule is a novel marker for human fetal myogenic cells and affects myoblast fusion. J. Cell Sci. 119(Pt 15), 3117–3127. doi: 10.1242/jcs.03056
Chang, E. I., Rozance, P. J., Wesolowski, S. R., Nguyen, L. M., Shaw, S. C., Sclafani, R. A., et al. (2019). Rates of myogenesis and myofiber numbers are reduced in late gestation IUGR fetal sheep. J. Endocrinol. 244, 339–352. doi: 10.1530/JOE-19-0273
Corvelyn, M., De Beukelaer, N., Duelen, R., Deschrevel, J., Van Campenhout, A., Prinsen, S., et al. (2020). Muscle microbiopsy to delineate stem cell involvement in young patients: a novel approach for children with cerebral palsy. Front. Physiol. 11:945. doi: 10.3389/fphys.2020.00945
Cosgrove, B. D., Sacco, A., Gilbert, P. M., and Blau, H. M. (2009). A home away from home: challenges and opportunities in engineering in vitro muscle satellite cell niches. Differentiation 78, 185–194. doi: 10.1016/j.diff.2009.08.004
Crisan, M., Yap, S., Casteilla, L., Chen, C. W., Corselli, M., Park, T. S., et al. (2008). A perivascular origin for mesenchymal stem cells in multiple human organs. Cell Stem Cell 3, 301–313. doi: 10.1016/j.stem.2008.07.003
Dayanidhi, S., Dykstra, P. B., Lyubasyuk, V., McKay, B. R., Chambers, H. G., and Lieber, R. L. (2015). Reduced satellite cell number in situ in muscular contractures from children with cerebral palsy. J. Orthop. Res. 33, 1039–1045. doi: 10.1002/jor.22860
Dayanidhi, S., Kinney, M. C., Dykstra, P. B., and Lieber, R. L. (2020). Does a reduced number of muscle stem cells impair the addition of sarcomeres and recovery from a skeletal muscle contracture? A transgenic mouse model. Clin. Orthop. Relat. Res. 478, 886–899. doi: 10.1097/CORR.0000000000001134
Delhaas, T., Van der Meer, S. F., Schaart, G., Degens, H., and Drost, M. R. (2013). Steep increase in myonuclear domain size during infancy. Anat. Rec. 296, 192–197. doi: 10.1002/ar.22631
Domenighetti, A. A., Mathewson, M. A., Pichika, R., Sibley, L. A., Zhao, L., Chambers, H. G., et al. (2018). Loss of myogenic potential and fusion capacity of muscle stem cells isolated from contractured muscle in children with cerebral palsy. Am. J. Physiol. Cell Physiol. 315, C247–C257. doi: 10.1152/ajpcell.00351.2017
Duddy, W., Duguez, S., Johnston, H., Cohen, T. V., Phadke, A., Gordish-Dressman, H., et al. (2015). Muscular dystrophy in the mdx mouse is a severe myopathy compounded by hypotrophy, hypertrophy and hyperplasia. Skelet. Muscle 5:16. doi: 10.1186/s13395-015-0041-y
Fischer-Lougheed, J., Liu, J. H., Espinos, E., Mordasini, D., Bader, C. R., Belin, D., et al. (2001). Human myoblast fusion requires expression of functional inward rectifier Kir2.1 channels. J. Cell Biol. 153, 677–686. doi: 10.1083/jcb.153.4.677
Gattazzo, F., Laurent, B., Relaix, F., Rouard, H., and Didier, N. (2020). Distinct phases of postnatal skeletal muscle growth govern the progressive establishment of muscle stem cell quiescence. Stem Cell Rep. 15, P597–611. doi: 10.1016/j.stemcr.2020.07.011
Joe, A. W., Yi, L., Natarajan, A., Le Grand, F., So, L., Wang, J., et al. (2010). Muscle injury activates resident fibro/adipogenic progenitors that facilitate myogenesis. Nat. Cell. Biol. 12, 153–163. doi: 10.1038/ncb2015
Lieber, R. L., Runesson, E., Einarsson, F., and Fridén, J. (2003). Inferior mechanical properties of spastic muscle bundles due to hypertrophic but compromised extracellular matrix material. Muscle Nerve 28, 464–471. doi: 10.1002/mus.10446
Murach, K. A., White, S. H., Wen, Y., Ho, A., Dupont-Versteegden, E. E., McCarthy, J. J., et al. (2017). Differential requirement for satellite cells during overload-induced muscle hypertrophy in growing versus mature mice. Skelet. Muscle 7:14. doi: 10.1186/s13395-017-0132-z
Rando, T. A., and Blau, H. M. (1994). Primary mouse myoblast purification, characterization, and transplantation for cell-mediated gene therapy. J. Cell. Biol. 125, 1275–1287. doi: 10.1083/jcb.125.6.1275
Schultz, E., and McCormick, K. M. (1994). Skeletal muscle satellite cells. Rev. Physiol. Biochem. Pharmacol. 123, 214–257. doi: 10.1007/BFb0030904
Smith, L. R., Chambers, H. G., and Lieber, R. L. (2013). Reduced satellite cell population may lead to contractures in children with cerebral palsy. Dev. Med. Child Neurol. 55, 264–270. doi: 10.1111/dmcn.12027
Smith, L. R., Chambers, H. G., Subramaniam, S., and Lieber, R. L. (2012). Transcriptional abnormalities of hamstring muscle contractures in children with cerebral palsy. PLoS ONE 7:e40686. doi: 10.1371/journal.pone.0040686
Smith, L. R., Lee, K. S., Ward, S. R., Chambers, H. G., and Lieber, R. L. (2011). Hamstring contractures in children with spastic cerebral palsy result from a stiffer extracellular matrix and increased in vivo sarcomere length. J. Physiol. 589(Pt 10), 2625–2639. doi: 10.1113/jphysiol.2010.203364
Smith, L. R., Ponten, E., Hedstrom, Y., Ward, S. R., Chambers, H. G., Subramaniam, S., et al. (2009). Novel transcriptional profile in wrist muscles from cerebral palsy patients. BMC Med. Genomics 2:44. doi: 10.1186/1755-8794-2-44
Von Walden, F., Gantelius, S., Liu, C., Borgstrom, H., Bjork, L., Gremark, O., et al. (2018). Muscle contractures in patients with cerebral palsy and acquired brain injury are associated with extracellular matrix expansion, pro-inflammatory gene expression, and reduced rRNA synthesis. Muscle Nerve 58, 277–285. doi: 10.1002/mus.26130
White, R. B., Bierinx, A. S., Gnocchi, V. F., and Zammit, P. S. (2010). Dynamics of muscle fibre growth during postnatal mouse development. BMC Dev. Biol. 10:21. doi: 10.1186/1471-213X-10-21
Yin, H., Price, F., and Rudnicki, M. A. (2013). Satellite cells and the muscle stem cell niche. Physiol. Rev. 93, 23–67. doi: 10.1152/physrev.00043.2011
Yoshioka, K., Kitajima, Y., Okazaki, N., Chiba, K., Yonekura, A., and Ono, Y. (2020). A modified pre-plating method for high-yield and high-purity muscle stem cell isolation from human/mouse skeletal muscle tissues. Front. Cell. Dev. Biol. 8:793. doi: 10.3389/fcell.2020.00793
Keywords: cerebral palsy, skeletal muscle, satellite cell, cell cultural, stem cell
Citation: Lieber RL and Domenighetti AA (2021) Commentary: Muscle Microbiopsy to Delineate Stem Cell Involvement in Young Patients: A Novel Approach for Children With Cerebral Palsy. Front. Physiol. 12:642366. doi: 10.3389/fphys.2021.642366
Received: 15 December 2020; Accepted: 14 January 2021;
Published: 05 February 2021.
Edited by:
Stefano Biressi, University of Trento, ItalyReviewed by:
Ferdinand Von Walden, Karolinska Institutet (KI), SwedenChristopher S. Fry, University of Kentucky, United States
Copyright © 2021 Lieber and Domenighetti. This is an open-access article distributed under the terms of the Creative Commons Attribution License (CC BY). The use, distribution or reproduction in other forums is permitted, provided the original author(s) and the copyright owner(s) are credited and that the original publication in this journal is cited, in accordance with accepted academic practice. No use, distribution or reproduction is permitted which does not comply with these terms.
*Correspondence: Richard L. Lieber, rlieber@sralab.org