- 1Instituto de Bioquímica Médica Leopoldo de Meis, Universidade Federal do Rio de Janeiro, Rio de Janeiro, Brazil
- 2Instituto Nacional de Ciência e Tecnologia em Entomologia Molecular, Rio de Janeiro, Brazil
Blood-feeding arthropods are considered an enormous public health threat. They are vectors of a plethora of infectious agents that cause potentially fatal diseases like Malaria, Dengue fever, Leishmaniasis, and Lyme disease. These vectors shine due to their own physiological idiosyncrasies, but one biological aspect brings them all together: the requirement of blood intake for development and reproduction. It is through blood-feeding that they acquire pathogens and during blood digestion that they summon a collection of multisystemic events critical for vector competence. The literature is focused on how classical immune pathways (Toll, IMD, and JAK/Stat) are elicited throughout the course of vector infection. Still, they are not the sole determinants of host permissiveness. The dramatic changes that are the hallmark of the insect physiology after a blood meal intake are the landscape where a successful infection takes place. Dominant processes that occur in response to a blood meal are not canonical immunological traits yet are critical in establishing vector competence. These include hormonal circuitries and reproductive physiology, midgut permeability barriers, midgut homeostasis, energy metabolism, and proteolytic activity. On the other hand, the parasites themselves have a role in the outcome of these blood triggered physiological events, consistently using them in their favor. Here, to enlighten the knowledge on vector–pathogen interaction beyond the immune pathways, we will explore different aspects of the vector physiology, discussing how they give support to these long-dated host–parasite relationships.
Introduction
For a long time, the traditional thinking on the insect vector–pathogen interaction described this relationship as impinging a low or even no fitness cost to the insect host (Sisterson, 2009; Powell, 2019). However, the discovery of the insect immune system brought to the scene a plethora of immune genes that were frequently modulated by the infection and, in several cases, the upregulation of these genes promptly reduced pathogen burden (Saraiva et al., 2016; Shaw et al., 2018; Telleria et al., 2018; Matetovici et al., 2019; Salcedo-Porras and Lowenberger, 2019). These findings led to a large number of studies on the immune signaling pathways and immune effector genes capable of decreasing or blocking pathogen burden and vector competence, which could lead to the possibility of limiting the disease prevalence under field conditions.
The immunology conceptual framework that has dominated the last century is the idea that the capacity to eliminate the infectious agent is the hallmark of the organism’s reaction against the infection. This so-called pathogen resistance and the path that eventually leads to health are considered synonymous with the elimination of the infectious agent. However, in the last decade, several studies have directed attention to the ability of organisms to reduce deleterious effects of infections without relying on the elimination of the parasite, but rather acting by alternative mechanisms that could restore tissue homeostasis, reducing self-inflicted damage caused by the host immune reaction or by the pathogen directly (Chovatiya and Medzhitov, 2014). Some early studies have termed this disease endurance (Casadevall and Pirofski, 1999; Graham et al., 2005; Ayres et al., 2008). Later these processes directed to reduce fitness loss due to infection were grouped under the concept of disease tolerance (Medzhitov et al., 2012). The tolerance to disease is highlighted here as a way to fight infection. Mechanistic studies show that a wide variety of processes are implicated in disease tolerance, such as stress response, tissue homeostasis, wound repair, and energy metabolism (Shaw et al., 2018). Essentially, this fresh outlook in immunology changes the microbe-killing focus of the immune response to the promotion of host health and survival through a global regulation of physiological responses (Lissner and Schneider, 2018). In insects, these studies were mainly performed with model insects, especially Drosophila (Lissner and Schneider, 2018) and the concept of disease tolerance has not yet been explored in the context of the interaction between insect vectors and the pathogens they transmit, as recently pointed (Oliveira et al., 2020).
Blood-feeding is a central event in the life cycle of both the insect vector and the pathogens they transmit. Typically, few parasites or viruses are taken up by the insect along with a blood meal. The number of pathogens in the blood meal is known as the critical bottleneck that will define the success of the infection. After a meal, the midgut becomes an aggressive environment, quickly populated by digestive enzymes that can potentially attack the pathogen. Moreover, it is colonized by an exuberant indigenous microbiota that may be a competitor for the incoming invader, at a clear numerical disadvantage. In addition, like all epithelial tissues, one of the main functions of the gut epithelium is to be a barrier of protection from the external world and to select what should enter into the organism. For most hematophagous insects, blood is the essential source of amino acids used to make yolk proteins. Therefore, hormonal control of reproduction is usually triggered by blood intake, which is tightly linked to the pace of blood digestion (Hagedorn, 2004). Several reports have shown hormonal effects on parasite infectivity, which, in some cases, have been attributed to a crosstalk between hormone signaling cascades and immune pathways (Nunes et al., 2020). Finally, for most blood-sucking insects, vertebrate hosts might be available more than once in a lifetime, and the effect of multiple blood meals have been shown to affect the life of pathogens by mechanisms that are not fully understood (Serafim et al., 2018). To summarize, the physiology of blood digestion of the hematophagous insect is the actual landscape where pathogens transmitted by them will thrive or not, a concept that was also revised by Nouzova et al. (2019). More importantly, the functioning of these processes ultimately defines the fitness cost of the infection to the insect vector, a major variable that affects vectorial capacity. Here we have addressed just some topics of vector physiology to illustrate the general concept that the performance of host organism integrated basic functions is critical to the success of the vector/pathogen association. As these are multiple and comprehensive topics, our intention is not to exhaust the analysis of the literature related to them. More than that, our goal is to highlight the need of examining the role of these and other non-immune basic aspects of insect physiology, not reviewed here, in determining the vector competence.
Blood Digestion and Metabolic Signaling
Some hematophagous arthropods feed on blood for their whole life cycle such as ticks and triatomine bugs, while in others, like mosquitoes and sandflies, only female adults feed on blood. In the adult stages, blood meals are strictly essential for oogenesis for most species. However, even for those few autogenous insects that use their teneral reserves to make the first batch of eggs, the following cycles of ovarian growth rely on vertebrate blood, and therefore, reproductive success depends on blood-feeding. The ingestion of a blood meal elicits a response in the gut-brain axis known to involve the central nervous system, the enteric nervous system, and the gastrointestinal tract (Hagedorn, 2004). The gut-brain crosstalk not only ensures the proper maintenance of gastrointestinal homeostasis but has multiple effects on insect physiology through neural, endocrine, immune, and humoral links (Gonzalez et al., 1999; Brown et al., 2008; Castillo et al., 2011; Gulia-Nuss et al., 2011). The nutritional intake connects intermediary metabolism to sexual maturation, oogenesis, microbiota colonization, and immune response, the latter being triggered by the encounter of the vector with pathogens.
Strictly speaking, digestion starts with hydrolysis of food by a vast array of digestive enzymes secreted after a meal (proteinases, carbohydrases, and lipases) that, together with nutrient transporting proteins, are needed to process nutrients in the gut (Santiago et al., 2017). However, in the context of hematophagous insects, proteases have received far more attention because blood is composed mainly of proteins (90% of dry weight), leading those insects to translate an arsenal of proteases to support protein digestion (Lehane, 2005; Brackney et al., 2010; Henriques et al., 2017; Sterkel et al., 2017). In most insects, proteolysis is based on trypsin and other serine proteases, in contrast to triatomines and ticks, where aspartic and cysteine proteases drive protein digestion (Sojka et al., 2008; Santiago et al., 2017; Henriques et al., 2021). Initially, protein degradation was described as accomplished by a few enzymes of each type, but genome sequencing in mosquitoes, triatomines, sandflies and ticks showed the existence of multiple copies of enzymes in all classes, revealing extensive gene duplications that probably occurred after the acquisition of the blood-feeding habit, suggesting the need for redundancy or some type of functional differentiation among enzymes of the same family (Sojka et al., 2008; Henriques et al., 2017; Santiago et al., 2017).
The possibility of pathogens being targeted by digestive enzymes and/or the manipulation of the host’s digestion process by the pathogens has been addressed in several studies, but most of them were performed before the genomic expansion of digestive proteases was acknowledged. Insect digestive tracts vary extensively in morphology and physicochemical properties, factors that greatly influence the potential interaction between their also diverse set of transmissible pathogens and digestive milieu. The activity of proteolytic enzymes in the gut of Anopheles mosquitoes does not appear to be affected by Plasmodium infection. In addition, differences in the activity of digestive proteases in general are not observed among mosquitoes with different levels of susceptibility to infection (Feldman et al., 1990; Chege et al., 1996; Jahan et al., 1999). However, proteolytic activities present in the midgut lumen are required to activate a chitinase secreted by these parasites that is essential in the initial event of midgut infection (Shahabuddin et al., 1993). In the Leishmania mexicana-Lutzomyia’s pair, the pathogen promotes a decrease of trypsin activity in the vector’s gut, increasing parasite burden. Trypsin knockdown exerts the same effect (Sant’Anna et al., 2009), probably making the parasite more resistant to the highly degradative habitat of midgut lumen. In contrast, in the triatomine bug Rhodnius prolixus, the activity of cathepsin D-like enzymes increases upon infection by Trypanosoma cruzi (Borges et al., 2006). Still, its inhibition did not affect parasite development in the conditions tested (Garcia and Gilliam, 1980). As we can see, the existing examples concerning proteases in the vector-parasite interaction were based on the analysis of total enzymatic activities, which resulted from the action of multiple enzymes belonging to the same class. These facts might be the answer to the diversity of responses observed among the different groups of insects. It would be interesting to know what are the specific proteases involved in the different events and if they can have the same role in the different vector-parasite pairs.
For enveloped viruses, it is known that the establishment of a successful infection is highly dependent on the fusion of the viral envelope with host cell membranes, where the envelope proteins need to be activated by proteolytic processing by host cell proteases (Klenk and Garten, 1994). As for Aedes aegypti infection by dengue virus, treating the mosquito with a trypsin inhibitor before exposure to the virus decreases the midgut infection, which can be partially rescued when the virus is previously incubated with bovine trypsin (Molina-Cruz et al., 2005). In this case, the authors discuss the possibility of the virus be pre-processed by trypsin before gut epithelia invasion, enhancing its virulence. In contrast, another work published in 2008 showed that silencing the late trypsin 5G1 or the addition of soybean trypsin inhibitor to the infectious blood meals increased midgut infection rates by DENV-2 (Brackney et al., 2008). The latter work was confirmed by a study showing that prior colonization of Ae. aegypti with the fungus Talaromyces sp. induced the downregulation of many digestive enzymes, including several trypsins, resulting in higher susceptibility to dengue infection. Moreover, knockdown of these trypsin genes was able to recapitulate the fungus-induced decrease in viral infection (Angleró-Rodríguez et al., 2017b). Although controversial, the studies made with dengue and Ae. aegypti suggest that blood digestion mediated by trypsins may influence the rate of DENV-2 infection. Although the literature on the subject is scarce, proteases may influence vector infection by viruses. The determination of the precise time course of viral invasion and protease expression together with the repertoire of proteases in the mosquito midgut would allow for a more comprehensive approach of the contribution of each digestive enzyme for mosquito vectorial competence.
There is now a general agreement that the successful infection of an insect vector involves a tripartite interaction between the insect, the pathogens and the intestinal microbiota (Cirimotich et al., 2011; Ramirez et al., 2012), a concept that has been verified for different host/pathogens associations (Castro et al., 2012; Narasimhan and Fikrig, 2015; Romoli and Gendrin, 2018; Telleria et al., 2018). Interestingly, studies performed with mammalian models revealed that proteases both from the host or from the microbiota are important modulators of intestinal homeostasis and are involved in the interaction with pathogens (Buzza et al., 2010; Motta et al., 2019; Edogawa et al., 2020; Kriaa et al., 2020). Therefore, it is tempting to speculate if the influence of proteases on vector infection by pathogens is not at least partially mediated by a role of these enzymes on intestinal microbiota.
In addition to proteins, vertebrate blood is also enriched with lipids. Host lipid usage by pathogens and regulatory changes of lipid metabolism triggered by infection appear as key players in several host-pathogen relationships, being essential determinants for vector competence, as recently revised by O’neal et al. (2020). Digestive lipases, such as TG lipases, are flux generating enzymes for lipid metabolism pathways. The essential process of absorption of digested lipids from the blood meal by midgut cells is followed by an increased lipid transport from the midgut to other tissues, to support development and oogenesis. This lipid transfer is promoted by lipophorin, the main hemolymph lipoprotein, and results in lipid accumulation in the fat body (revised by Gondim et al., 2018). Interestingly, Plasmodium oocysts in the Aedes gut basal lamina hijack mosquito lipophorin to support the parasite development (Atella et al., 2009) and knockdown of lipophorin by RNA interference (RNAi) strongly restricted development of Plasmodium oocysts, reducing their number by 90% (Cheon et al., 2006; Rono et al., 2010). Similarly, induction of lipophorin synthesis is observed in C. quinquefasciatus with the filaria Wuchereria bancrofti (Kumar and Paily, 2011).
Host lipid remodeling is also observed in vertebrate infection by arboviruses to support their replication (Ng et al., 2008; Fernández de Castro et al., 2016; Leier et al., 2020). Similar reprogramming events have already been shown in vector cells (Perera et al., 2012; O’neal et al., 2020). An increase in the number of lipid droplets has been observed in Aedes albopictus C6/36 cells infected with dengue virus (Samsa et al., 2009). Furthermore, lipidomic analyses of the same DENV-infected cells revealed a large number of differentially expressed genes of diverse classes of lipids such as phospholipids and sphingolipids (Perera et al., 2012). On the same way, Ae. aegypti Aag2 cell line showed a regulation in the expression of lipid-related genes upon dengue 2 infection (Barletta et al., 2016). Besides, in vivo lipidomics showed that dengue infection in Ae. aegypti mosquitoes changed the lipid profile, mainly based on the inhibition of acylglycerolphosphate acyltransferase (AGAPT1), leading to an accumulation of phospholipids that support the viral replication (Chotiwan et al., 2018; Vial et al., 2019).
The blood-fed Ae. aegypti gut seems to increase the expression of many lipid-related genes, such as fatty acid synthase and perilipin-like proteins, which boost lipid droplet formation after blood meal (Barletta et al., 2016). Lipid droplets were shown in mammals to serve as a signaling platform involved with the synthesis of bioactive lipids (eicosanoids) (Vallochi et al., 2018). In Aedes mosquitoes, it has been shown that the midgut epithelia synthesize prostaglandins in response to the microbiota expansion in a phospholipase A-dependent manner that tunes the innate immune system against viral infection (Barletta et al., 2020). Similarly, Anopheles gambiae midgut produces prostaglandins in response to microbiota elicitors upon Plasmodium invasion, which triggers a cellular immune response (Barletta et al., 2019). The bug R. prolixus humoral and cellular responses seem to be modulated by eicosanoids as well (Azambuja et al., 2017). Using T. rangeli infection model, it was demonstrated that the insect reduces the arachidonic acid (the eicosanoid precursor) circulating in the hemolymph, leading to an inhibition of hemocytes phagocytic activity in the hemolymph (Garcia et al., 2004b; Figueiredo et al., 2008). Although eicosanoids/prostaglandins are part of the immune molecular arsenal in insects, these arthropods lack the canonical cyclooxygenase (COX), a key enzyme to convert the arachidonic acid into eicosanoids (Varvas et al., 2009). This is intriguing because those insects respond to the treatment with pharmacological COX inhibitors, such as indomethacin and acetylsalicylic acid, impairing the eicosanoid synthesis and modulating the immune response (Garcia et al., 2004a; Barletta et al., 2020). A question that remains open is what are the enzymes that play the role of canonical vertebrate COX in insects. In this sense, a specific peroxinectin named Pxt, that catalyzes the formation of the prostaglandin H2 (PGH2), was identified in the follicle cells of Drosophila (Tootle et al., 2011). Later the same COX-Like activity was identified in the moth Spodoptera exigua (Park et al., 2014). Additionally, Barletta et al. (2019) showed that An. gambiae heme peroxidases 7 and 8 are important enzymes to synthesize the prostaglandin by the mosquito gut epithelia, suggesting possible candidates for alternative enzymes with COX-like activities.
The interplay between the lipid metabolism pathways and the autophagy-related molecular machinery, named as lipophagy, and its role in physiological and pathological processes in mammals has received increased attention in the last few years (Schulze et al., 2017; Kounakis et al., 2019). Recently, it was demonstrated that the Chagas’ disease vector R. prolixus can use lipophagy during starvation to increase life span and locomotor activity (Santos-Araujo et al., 2020). It would be interesting to verify how this lipophagic machinery works under infection by either T. cruzi, a parasite limited to the intestinal environment, or T. rangeli, which is capable to invade the hemocoel and colonize salivary glands. Moreover, in enteric-infected Drosophila, an immune response is assembled by a lipophagy-dependent activation of DUOX (Lee et al., 2018). Thus, the contribution of lipophagy to the success of vectors’ infection by their respective pathogens, remains obscure and deserve to be investigated.
Insects do not synthesize cholesterol de novo, meaning that this lipid has to be absorbed from the diet over their lifetime (Clark and Block, 1959; Zande, 1967). It has been shown in mammalian models that different immune challenges entail cholesterol mobilization (Tall and Yvan-Charvet, 2015). In the case of viruses, the infection interferes in several aspects of cholesterol metabolism, needed for the formation of cell membranes and intimately related to both the entry of viral particles in the cell and their exportation (Osuna-Ramos et al., 2018). Unfortunately, literature on vector biology only tangentially looked at this particular aspect, pointing out some genes involved in cholesterol metabolism and cellular traffic, such as the Niemann Pick 1 protein and the Sterol Carrier Protein 2, as host factors that allow the viral multiplication in the mosquito (Junjhon et al., 2014; Jupatanakul et al., 2014, 2017; Fu et al., 2015; Chotiwan et al., 2018). Dengue infection blocking by Wolbachia in mosquitoes also correlates with changes in cholesterol metabolism, trafficking, and accumulation (Geoghegan et al., 2017). Moreover, even though cholesterol is known as a precursor for the hormone ecdysone (Canavoso et al., 2001), the association between viral infection and hormonal signaling is largely unknown. Nevertheless, definitive evidence showing the contribution of dietary cholesterol to the viral replication in mosquito is still lacking. Additionally, it is also unexplored how serum cholesterol fluctuation in populations from endemic areas could correlate to the viral transmission by mosquitoes.
Some medical relevant parasites such as Apicomplexan and Trypanosomatids, similarly to insects, lack the capacity of de novo cholesterol synthesis (Coppens, 2013; Pereira et al., 2015). In order to differentiate and proliferate in the insect gut, they obtain cholesterol from the vertebrate’s plasma low-density lipoprotein (LDL) (Labaied et al., 2011; De Cicco et al., 2012; Petersen et al., 2017). Lipophorin, mentioned previously to be hijacked from mosquitoes by parasites, might be the lipoprotein responsible for cholesterol import during the parasite insect stage. However, this hypothesis remains to be tested.
Most articles that compare carbohydrate metabolism of vectors-fed in sugar-rich diets with those fed on blood have focused on the fat body and physiological homeostasis. Sugar metabolism in these animals is controlled at the hormonal level by ILP and juvenile hormone (JH) (Clifton and Noriega, 2011; Hansen et al., 2014; Hou et al., 2015; Roy et al., 2015). It is increasingly clear that parasites can dramatically change the cellular energy metabolism of their arthropod vectors, as recently revised by Samaddar et al. (2020). Still, for mosquito-arbovirus interactions, the knowledge on such metabolic alterations is limited. An in vitro study showed that Zika virus infection in Ae. albopictus drives the glucose metabolism toward the pentose phosphate pathway, differently from human cells that increase flux to the tricarboxylic acid cycle (Thaker et al., 2019). Activation of the pentose pathway provides NADPH for antioxidant pathways, which control the intracellular redox state. Maintaining the redox balance would be beneficial for viruses as it would protect them from oxidative damage. However, the relevance of these changes to the course of viral infection has not been experimentally addressed in the literature yet, despite alterations in expression of metabolic enzymes being regularly observed in transcriptomic analyses of infected vector digestive apparatus (Padrón et al., 2014; Angleró-Rodríguez et al., 2017a; Etebari et al., 2017; Narasimhan et al., 2017; Coutinho-Abreu et al., 2020). A large amount of gene expression data on vector infection has now accumulated, and it could be used to direct studies focusing on the crosstalk between canonical immunity pathways and carbohydrate/energy metabolism, the so-called immunometabolism, and the relevance of them to vector biology (Samaddar et al., 2020).
As in other organisms, in addition to its digestive role, the digestive tract functions as a nutrient sensor. Intestinal signaling is involved in integrative processes that link nutritional availability with behavior and metabolism and the microbial intestinal world, including eventual pathogens that come along with the food. One of the few reports on this subject showed that in Ae. aegypti blood intake triggers nutrient-sensing signaling, such as the Target of Rapamycin (TOR), responsible for translation of early trypsin (Brandon et al., 2008). This is extremely important for the course of digestion, as this initial event coordinates the late digestive phase (Barillas-Mury et al., 1995; Brackney et al., 2010). A broad spectrum of cellular mechanisms depends on a signaling pathway. The routes taken will rest on the pairs of signaling molecules and receptors that trigger the process. In addition to nutrients such as amino acids and heme, which act also as signaling molecules (Hansen et al., 2004; Oliveira et al., 2011a; Bottino-Rojas et al., 2015; Short et al., 2017), a blood meal brings also regulatory peptides that act as neurochemicals and hormones, like vertebrate insulin, insulin like growth factor (IGF1), TGF-β and other cytokines. Furthermore, the presence of parasites in the blood meal can antagonize or potentiate the effects of these vertebrate-borne signaling molecules in the vector organism (Pakpour et al., 2013a). The resulting cellular responses can be beneficial or detrimental to pathogen development. Most of the studies on signaling pathways and vector susceptibility/resistance to infection have focused on their role in the activation of immune pathways. These studies have been extensively discussed previously by others (Pakpour et al., 2013a, 2014; Urbanski and Rosinski, 2018; Sharma et al., 2019; Nunes et al., 2020). Among the best-known signaling pathways in insect vector species is the insulin/insulin-like growth factor signaling pathway (IIS), which is involved in the regulation of growth, longevity, reproduction and immunity. An. stephensi stimulated with human ILPs induces ROS-mediated signaling, without oxidative damage that culminates with NFκB inhibition, allowing the Plasmodium falciparum oocyst development (Surachetpong et al., 2011; Pakpour et al., 2012). On the other hand, dietary insulin showed a negative impact on flavivirus replication in Ae. aegypti and Ae. albopictus cells, and Culex quinquefasciatus adult mosquitoes, in a mechanism dependent on JAK/Stat activation (Ahlers et al., 2019). Furthermore, it was shown that insulin receptor knockdown in C. quinquefasciatus blocks filarial parasite development (Nuss et al., 2018).
The IIS pathway comprehends two branches: the mitogen-activated protein kinase (MAPK) and the phosphatidylinositol 3-kinase (PI3K)/Akt. A series of studies have shown that both branches are modulated by host blood components. Host growth factors/cytokines affect the mosquito-malaria parasite interaction by modulating the MAPK signaling pathway. Ingested human IGF1 reduces phosphorylation of the MAPK ERK signaling protein in An. stephensi midgut and decrease the intensity and prevalence of P. falciparum infection (Drexler et al., 2013). Accordingly, the mammalian host TGF-β-1 induces the expression of nitric oxide synthase and reduces the prevalence of Plasmodium infection in An. stephensi. This effect is inhibited by the activation of ERK (Surachetpong et al., 2009). Interestingly, TGF-β also appears to be critical for the survival of parasites such as T. cruzi and Leishmania amazonensis in mammalian hosts (Barral-Netto et al., 1992; Ming et al., 1995; Omer et al., 2000). However, the impact of host TGF-β on the interaction of these parasites with their vectors has not yet been investigated.
Regarding the PI3K/AKT, Corby-Harris et al. (2010) showed that the overexpression of an activated form of Akt in An. stephensi, a regulator of IIS, shortened the mosquito lifespan and increased resistance to P. falciparum. Lately, the same group showed that the sustained Akt activation in the mosquito midgut resulted in mitochondrial dysfunction coupled to Akt-mediated repression of autophagy and compromised midgut epithelial structure. The perturbation of midgut homeostasis enhanced parasite resistance and decreased mosquito lifespan (Luckhart et al., 2013).
Insulin-like peptides induced by blood-feeding trigger vitellogenesis in the fat body of Ae. aegypti and act as regulators of the blood digestion in the gut (Gulia-Nuss et al., 2011; Roy and Raikhel, 2012). In An. stephensi, ILPs interfere in the mosquitoes intermediary metabolism and nutrient intake (Pietri et al., 2016). Interestingly, P. falciparum soluble products induce the expression of ILPs in An. stephensi, through both the MEK/ERK and PI3K/Akt branches of IIS and inhibiting P. falciparum development in vivo by affecting mosquito immune effector genes (Pietri et al., 2015). This ILP-mediated inhibition of parasite development is somehow contradictory with the previous findings that human insulin could favor the parasite growth, raising the possibility that even being structurally similar, vector and host insulins can elicit distinct gut responses to Plasmodium infection. Moreover, Castillo et al. (2011) showed that the insulin signaling pathway can directly regulate hemocyte proliferation in Ae. aegypti. The same study also highlights an interesting observation regarding blood-feeding: resistance and tolerance to the same bacterial pathogen dramatically change due to blood meal digestion and/or mobilization of resources for reproduction (Castillo et al., 2011).
The PKC pathway is part of this complex signaling network that responds to infection in different vectors. Inhibition of PKC blocks West Nile virus entry in C6/36 cells by inhibiting endosomal sorting (Chu et al., 2006). In Ae. aegypti, activation of PKC by the heme released during the digestion of blood decreases ROS production and allows an increased proliferation of indigenous microbiota (Oliveira et al., 2011a). However, the effect of PKC pathway on viral infection has not been investigated until now. PKCs have also been shown to be expressed in the midgut epithelia of An. gambiae and An. stephensi after a blood meal. As in Ae. aegypti, the An. stephensi PKC activation was also linked to the decrease of midgut epithelial barrier, resulting in the greater development of P. falciparum oocysts (Pakpour et al., 2013b), without modulation of NF-κB-dependent immune factors, thus indicating that the regeneration of the midgut epithelium is essential for infection control, as also suggested by Taracena et al. (2018).
So far, in spite of the advances discussed here, it remains widely unclear how this network of nutritional signaling pathways reflects on the parasite/host interaction. This reinforces the need to build a more holistic view of the interplay between metabolic changes and the canonical immune responses to vector transmitted pathogens that occur after a blood meal, using the integrative conceptual framework of immunometabolism.
Peritrophic Matrix
The mammalian gastrointestinal epithelium is protected by the secretion of a mucus layer, mostly composed of highly glycosylated proteins (mucins) (Johansson et al., 2013; Sicard et al., 2017). The hydrophilic O-linked oligosaccharides that coat these proteins give them the physical and chemical properties that support the mucus protective role. Mucins are secreted by specialized cells, such as goblet cells in the intestine, and have a short half-life, which ensures the constant renewal of the mucus barrier (Deplancke and Gaskins, 2001). This barrier is essential to the digestive tract as it protects the tissue from mechanical damage by food particles, chemical aggression (pH and action of digestive enzymes) and limits direct contact with the microbiota. Although frequently neglected or ignored, insect gut also presents a bonafide mucous layer, with transcriptomic data revealing abundant expression of mucins in the midgut (Terra et al., 2018). The peritrophic matrix (PM) is an extracellular structure found in the intestinal lumen of insects that is ascribed a major role as a protective layer in the midgut, usually described as analogous to the vertebrate mucus. The PM was first described by Lyonet in 1762 and is composed of glycosylated proteins embedded in chitin fibers (for reviews see Lehane, 1997; Terra, 2001, Terra et al., 2018). PMs can be classified into two different types: Type 1 is secreted by all epithelial cells as a continuous gel-like structure that completely packages the food bolus and is formed in response to feeding. The type 2 PM is a membranous structure characterized by its constitutive secretion by a midgut region called cardia and delimits an area between the epithelium and the PM, the ectoperitrophic space. It has a tubular morphology and lines the whole gut epithelium (Shao et al., 2001). Adult mosquitoes such as Ae. aegypti and Anopheles sp. secrete type 1 PM after a blood meal but present the type 2 PM at larval stages. The same is observed in the sandfly L. longipalpis (Secundino et al., 2005). Glossina spp. and Drosophila present the type 2 PM also in the adult stage. Hemipteran insects like the Chagas’ disease vectors are an exception in that they lack the classical PM and the lipidic perimicrovillar membranes are responsible for the PM functions (Terra, 2001; Hegedus et al., 2019). In the case of ticks, the PM is a chitin-containing extracellular layer that covers the digestive cells, displaying a very distinct morphology to that of a typical insect PM (Matsuo et al., 2003; Grigoryeva, 2010; Kotsyfakis et al., 2015), probably reflecting their peculiar tick digestive physiology, based on intracellular digestion of host blood proteins (Lara et al., 2005).
In most insects, the PM functions as a molecular sieve, controlling the traffic of molecules between the intestinal epithelium to the lumen, compartmentalizing the digestion and protecting the epithelium from potentially cytotoxic molecules (Billingsley and Rudin, 1992). However, several of these roles have been scarcely investigated in blood-feeding insects such as mosquitoes. For example, it has been shown that disruption of PM by the action of exogenous chitinases increases the blood digestion rate, an unexpected effect that was attributed to augmented access of intestinal proteases to the blood bolus (Villalon et al., 2003). Also, the Ae. aegypti PM binds heme released during the digestion of hemoglobin, which was hypothesized to reduce its oxidative potential (Pascoa et al., 2002).
In contrast, the PM acting as a barrier that limits exposure to the microbial world has been more thoroughly investigated. In mammals, the relative lack of an exuberant immune response against the intestinal microbiota has been attributed to the mucus acting as a barrier that avoids direct contact between the microbiota and the epithelium and not to the microbiota subverting the host immune response, a phenomenon named as “immunological ignorance” by Hooper (2009). Thus, immune homeostasis is attained largely by the mucus layer limiting the exposure of enterocytes to the microbiota (Li et al., 2018). Similarly, in insects, the secretion of the PM approaches this pivotal immune barrier function, as it compartmentalizes the microbiota and its immune elicitors, avoiding the overexposure of the gut epithelium (Buchon et al., 2009; Kuraishi et al., 2011, 2013; Weiss et al., 2014). In this way, it was demonstrated that An. gambiae mosquitoes express a heme peroxidase, which is essential to crosslink the PM proteins/mucins, supporting the correct assembly of this barrier. Once this peroxidase is knocked down, the PM barrier is compromised and the gut epithelia is exposed to microbial elicitors that over activate the intestinal immune system (Kumar et al., 2010).
When the microbiota and the PM layer barrier function become unbalanced, the intestinal cell homeostasis is rapidly affected. Both in mosquito and Drosophila the gut stem cell populations respond to biotic and abiotic injury and their activation is prevented by the PM presence (Micchelli and Perrimon, 2006; Buchon et al., 2013; Janeh et al., 2017; Taracena et al., 2018). In Ae. aegypti, when the PM structure is compromised by inhibition of chitin synthesis, the epithelial midgut is exposed to the microbiota, which in turn activates the generation of ROS, causing tissue damage and leading to a regenerative response based on mitotic proliferation of progenitor cells (Taracena et al., 2018). Complementary to this mechanism, it was shown in Anopheles mosquitoes that genes related to the synthesis of chitin and peritrophins – that together form the structural backbone of the PM – have their expression stimulated by proliferation of the intestinal microbiota (Rodgers et al., 2017). This effect closely recapitulates the mammalian response of goblet cells that prompt the secretion of stored mucus after exposition to native and pathogenic bacteria (Coconnier et al., 1998; Deplancke and Gaskins, 2001).
In mosquitoes, the intestinal microbiota experiences an explosive expansion after a blood meal. Of course, this microbial blooming is fueled by the sudden increase in the availability of nutrients, jumping from a few thousand bacterial cells in sugar-fed Ae. aegypti to a plateau of a few million cells in a single midgut by 12 h after blood-feeding (Oliveira et al., 2011a). However, this is probably well below the microbial population that could be supported by an unrestrained growth of bacteria in about 2 ml of blood, posing a question that has not yet been properly addressed: how fine-tuning regulation of the microbial growth is attained? At least one of such mechanisms is the production of ROS by the gut epithelium, as already mentioned above (Ha et al., 2005a; Oliveira et al., 2011a). Consistently, it was shown that gut ROS production is also attenuated by the barrier function of the PM (Taracena et al., 2018), highlighting the existence of mechanisms that balance the microbe-killing mucosal response in a way that is neither too detrimental to the host nor to the microbiota. Of interest, this mode of operation can also be relevant during infection by pathogenic microorganisms.
The first encounter between the insect host and a vectored parasite coincides with the blood digestion in the midgut, exactly when the PM is formed, making it plausible that this immune barrier can regulate the infection’s success. Anopheline mosquitoes ingest Plasmodium gametocytes that will fertilize and generate the ookinetes, which will invade the intestinal epithelium by secreting chitinases activated by mosquito digestive enzymes, allowing the parasite to traverse the PM. The invasion will happen around 24 h after feeding, concomitantly with PM formation peak (Huber et al., 1991; Shahabuddin et al., 1993). Moreover, it has been shown that proteins present in the matrix can function as anchors for the parasite, promoting its penetration process (Zhang et al., 2015). Although one would expect the PM might impose a barrier to the parasite infection in the gut, several reports showed instead that the absence of this structure decreases the gut parasitemia (Billingsley and Rudin, 1992; Shahabuddin et al., 1995; Baia-da-Silva et al., 2018). The PM regulation is also essential for Leishmanial infection of sandflies (Coutinho-Abreu et al., 2010). Leishmania parasites do not invade the epithelium but hide in the ectoperitrophic space (between the epithelium and the PM) anchored to epithelial cells, which likely protect them from the action of digestive proteases (Pimenta et al., 1997; Ramalho-Ortigao, 2010). For the Glossina – T. brucei pair, for a long time it was not understood how the parasites crossed the flies’ PM. However, recent evidence was provided that the expression of genes related to the PM formation was influenced by infection. In these studies, the authors show that T. brucei targets the cardia, causing the discontinuation of PM type I secretion and allowing them to invade the ectoperitrophic space (Aksoy, 2019; Rose et al., 2020).
In the Chagas disease parasite replicative stage, the T. cruzi epimastigotes, adhesion to the kissing bug perimicrovillar membranes seems important for their division (Gonzalez et al., 1999). Treatment of the intestinal tissue with antiserum against the perimicrovillar membrane reduces the trypanosomatid development in the vector (Gonzalez et al., 2006). As mentioned above, kissing bugs don’t have a PM but perimicrovillar membranes, which are phospholipid membranes secreted by the gut and that, analogous to the PM, define a perimicrovillar space (Terra, 1988). The T. cruzi epimastigotes are attached to these perimicrovillar membranes through their flagella and membrane glycoinositolphospholipids. Hydrophobic proteins located in their surface and sugar residues present in perimicrovillar membrane glycoproteins appear to be necessary for this interaction (Zingales et al., 1982; Golgher et al., 1993; Pereira-Chioccola et al., 2000; Alves et al., 2007; Nogueira et al., 2007). In R. prolixus, it has been shown that another function of the perimicrovillar membranes is the promotion of the aggregation of heme molecules, forming nucleation sites that convert heme into hemozoin crystals and hence preventing heme toxicity toward both the host and the parasite, that consequently creates a favorable environment for pathogen growth (Oliveira et al., 2000; Stiebler et al., 2014; Ferreira et al., 2018).
The role of the Ae. aegypti matrix in controlling viral infections has not been investigated so far. It is not clear whether the viral particle can get through the pores of the matrix or if the invasion of the epithelium occurs in the first hours after the meal, when the matrix has not yet been completely modeled. The latter hypothesis is the most accepted by the community, although there is a knowledge gap behind this topic (Franz et al., 2015).
In non-hematophagous insects, it is suggested that the peritrophic matrix secretion integrates the hormonal signaling, mediated by ecdysone, to the pathways downstream to nutritional sensors (Merzendorfer and Zimoch, 2003). Feeding mosquitoes with an artificial diet of low nutritional value that promotes distension of the epithelium stimulates the synthesis of a fragile and short-lived matrix, different from the robust structure observed upon blood-feeding (Dinglasan et al., 2009; Whiten et al., 2018). Thus, it is worth assessing whether the matrix synthesis is controlled by nutritional/metabolic sensors (such as the target of rapamycin, TOR and AMP-activated protein kinase, AMPK) regulated upon blood arrival, in addition to the molecules released during digestion, such as heme, hormonal signaling induced by digestion (e.g., ecdysone and ILP) or microbial community expansion.
The understanding of the PM as a barrier that coordinates the insect intestinal immune activation beyond its digestive aspect, leads to new perceptions and insights. For example, maintenance of cellular homeostasis in addition to tissue damage repair may be central to disease tolerance (Oliveira et al., 2020), while current state of literature focuses on infection resistance. Future studies are needed that address how the microbe-associated patterns are presented to the gut epithelia, and consequently how the classical immune system will be tuned and shape the parasite life history.
Intestinal Redox Homeostasis
Historically, research on reactive oxygen species (ROS) was pushed to a central position in biology after the discovery of superoxide dismutase (McCord and Fridovich, 1969). The scene was dominated for about 30 years by the study of the role of ROS in pathologic conditions (Lambeth, 2007; Liu et al., 2018), where oxidative stress was defined as an imbalance between antioxidant mechanisms and production of ROS by several sources, including microbe-killing NADPH oxidases of immune cells. However, the discovery of the signaling role of nitric oxide (NO) in the regulation of diverse aspects of cell physiology, followed by several reports on hydrogen peroxide acting as a second messenger of several hormones and growth factors, led to a change in paradigm, with the introduction of redox signaling and redox homeostasis as novel steering concepts (Reth, 2002; Jones, 2006; Veal et al., 2007; Jones and Sies, 2015).
As already mentioned above, the digestive apparatus of most animals is also home to an abundant and specific microbial community that is now recognized as having a major and pleiotropic impact on the physiology of the metazoan host (Douglas, 2019). However, the mechanisms that control the growth rate of the intestinal microbiota are still not fully understood. Seminal studies in Drosophila revealed that an intestinal Dual oxidase (DUOX) produced H2O2 in response to the presence of microorganisms in the gut lumen (Ha et al., 2005a,b). Importantly, they also showed that the gut epithelium was protected from H2O2 by its dismutation to H2O by a heme-peroxidase that at the time was mistakenly called “Immune regulated catalase.” A DUOX enzyme found in Ae. aegypti mosquitoes has its activities decreased upon ingestion of a blood meal, leading to a marked reduction of ROS levels in the midgut (Oliveira et al., 2011a). Inhibition of the mosquito DUOX caused an increase in the size of the indigenous intestinal microbiota (Oliveira et al., 2011a), revealing a role of ROS metabolism in fine-tuning the symbiotic relationship between the commensal microbial community and the mosquito, in addition to its canonical immune action in the defense against pathogens.
Several other reports addressed the relation between ROS and pathogens in insect vectors. In an elegant work, Liu et al. (2016) showed that RNAi silencing of mosquito DUOX increased dengue virus replication in the midgut. Viral NS1 protein present in the host plasma enhanced susceptibility of the mosquito to DENV infection by reducing expression of DUOX, as well as of NoxM/Nox4-art (a NOX-4 homolog specific of the arthropod lineage, Gandara et al., 2017). Moreover, the iron concentration in the host’s blood was inversely correlated to the prevalence and viral load of mosquito infection by dengue virus (Zhu et al., 2019). The catalase knockdown in Ae. aegypti changed the mosquito’s susceptibility to DENV but had no impact on the Zika virus (ZKV) establishment. On the other hand, the ZKV viral load in the mosquito midgut was decreased by the redox imbalance promoted by down-regulation of NRF2 antioxidant transcription factor, suggesting that other components of the redox homeostasis downstream of NRF2 are involved in the control of ZKV infection (Bottino-Rojas et al., 2018). Among those, there are canonical NRF2 targets, such glutathione S-transferase and cytochrome P450, suggested to be active in maintaining tissue homeostasis during blood digestion in the mosquito (Bottino-Rojas et al., 2018, 2019). The reduction of viral load by ROS has been explained in most cases by assuming it is inflicting direct damage to the viral particle. However, experimental proof for this hypothesis is still lacking. Interestingly, an alternative mechanism emerged from the demonstration that redox imbalance and its associated cellular damage in Ae. aegypti and Ae. albopictus midgut led to increased programmed cell death, triggering a homeostatic response based on tissue-repairing mitotic activity of intestinal stem cells (Janeh et al., 2017; Taracena et al., 2018). The same report showed that this increased cellular turnover in the gut epithelia negatively impacted vector susceptibility to arbovirus infection (Taracena et al., 2018). Notwithstanding, in mosquito strains naturally refractory to viral infection, resistance was dependent on the proper recruitment of stem cells via Delta/Notch signaling pathway (Taracena et al., 2018).
In a way similar to what happens with the mosquito down-regulation of ROS production after a blood meal (Oliveira et al., 2011a), the kissing bug R. prolixus also decreases the intestinal ROS generation after blood-feeding (Gandara et al., 2016). However, this is not triggered by the dietary heme, as it was shown for Ae. aegypti (Oliveira et al., 2011a), but by the nutritional intake, which increases amino acid levels and activates the TOR pathway, impacting negatively the production of mitochondrial ROS by an yet uncharacterized mechanism (Gandara et al., 2016). Nogueira et al. (2015) showed that while high levels of H2O2 reduced growth of the T. cruzi epimastigote stages, low levels increased proliferation. In contrast, antioxidant molecules reduced the proliferation of epimastigotes but increased conversion to the infective trypomastigote form, revealing that ROS levels in the bug digestive apparatus have a complex regulatory role on the life cycle of the parasite.
The phlebotomine L. longipalpis presents an interesting illustration of how redox homeostasis in the normal gut physiology is relevant for the parasite. In the sandfly, there is a decrease in the intestinal ROS generation upon feeding (Diaz-Albiter et al., 2012), similar to Ae. aegypti (Oliveira et al., 2011a) and R. prolixus (Gandara et al., 2016). ROS levels are increased by infection with a Serratia marcescens strain pathogenic for the sandfly, and uric acid (an antioxidant) administration to the sugar meal increased virulence of this bacteria, revealing that the ROS-producing pathways in the gut can be controlled by immune signaling (Diaz-Albiter et al., 2012). In contrast, Leishmania mexicana infection does not increase ROS levels after a blood meal (Diaz-Albiter et al., 2012), but silencing the sandfly catalase or maintaining them fed in sugar meal supplemented with H2O2 decreased the intestinal parasite load. This result revealed that not only is the parasite capable of evading immune activation of ROS, but it is additionally benefited by the down-regulation of ROS levels that is part of the normal physiology of the host. However, this goes beyond simple immune evasion by the parasite, as another report, also from Dillon’s group, showed that Leishmania infection indeed protected flies from death by Serratia co-infection, but without reducing Serratia levels (Sant’Anna et al., 2014), strongly suggesting that the Leishmania is acting by triggering tolerance to disease mechanisms, thereby reducing damage and preventing fitness loss, without killing of the pathogen (in this case, the Serratia bacterium).
A Plasmodium refractory An. gambiae strain was appointed to have intrinsic higher levels of H2O2, which increases even more after an infectious blood meal as part of its antiparasitic defense mediated mainly by hemocytes (Kumar et al., 2003; Molina-Cruz et al., 2008) and this could be attributed to higher mitochondrial ROS production in the refractory mosquitoes (Oliveira et al., 2011b; Gonçalves et al., 2012). The antioxidant defenses induced by blood-feeding in this model seem to be in part under the control of a redox sensor, called OXR1, that regulates both the expression of antioxidant enzymes and the success of parasite infection (Jaramillo-Gutierrez et al., 2010).
Anopheles mosquitoes have a unique redox metabolism upon feeding and infection, described as part of the so-called “time bomb model” where the midgut invasion by the parasites triggers an epithelial response based on protein nitration, activation of peroxidases, and, consequently, apoptosis of those invaded cells (Han et al., 2001; Kumar et al., 2004). This complex intestinal response to the ookinete invasion was molecularly dissected, revealing the role of NOX5, a NADPH oxidase member, as the source of ROS. It works with a heme peroxidase to mediate the parasite nitration (Oliveira et al., 2012). While these studies point to a conventional “immune” function for NOX5, recently, the NOX5 enzymes were shown to regulate muscular function, both in mammalian blood vessel smooth muscle contraction and in the intestinal peristalsis in R. prolixus (Montezano et al., 2018), adding some more complexity to this scenario and bringing about the possibility of the existence of a crosstalk between physiological mechanisms and the microbiota.
The role of reactive nitrogen species (RNS) in the metabolism of hematophagous vectors was initially revealed by the demonstration that increases in NO levels limited the development of P. falciparum in An. stephensi (Luckhart et al., 1998). Subsequent works performed by the same group led to the molecular characterization of nitric oxide synthase (NOS) (Luckhart and Rosenberg, 1999) and the modulation of NOS expression during infection by factors such as the parasite’s hemozoin pigment (Akman-Anderson et al., 2007) and glycosylphosphatidylinositols (Lim et al., 2005). It was also shown that the An. gambiae NOS gene is controlled by Jak/Stat pathway upon infection with P. berghei (Gupta et al., 2009). Nitrogen reactive species have a complex chemistry, acting not only through the canonical effect of NO on cGMP formation, but also via its reaction with superoxide forming peroxynitrite. This highly reactive intermediate modifies amino acid side chains and generates derivatives such as nitrotyrosine or nitrosothiols, which are formed in the mosquito gut and are modulated by infection, having profound effects on cell signaling (Kumar et al., 2004; Gupta et al., 2005; Peterson and Luckhart, 2006; Peterson et al., 2007; Oliveira et al., 2012). While protein nitration was shown to be relevant in triggering an anti-plasmodium response (Oliveira et al., 2012), global and mechanistic analyses of nitrosative signaling on insect physiology are still scarce. More recently, it has been reported that the kissing bug R. prolixus produces NO in response to T. rangeli (Whitten et al., 2007), and NOS inhibition allowed the proliferation of T. cruzi parasites in the insect gut (Batista et al., 2020). Nonetheless, the role of RNS-involved pathways and their oxidative implications to parasites/virus infection of mosquitoes or other vectors is still largely unknown and exposes an important avenue for future investigation.
Another way to positively modulate intestinal ROS in mosquitoes is through native microbiota elicitors (Oliveira et al., 2011a; Xiao et al., 2017), and this can have consequences for pathogens. An Enterobacter strain isolated from the midgut of wild-caught mosquito was shown to decrease P. falciparum infection by inducing ROS generation in the gut. The infection load was restored in the presence of vitamin C (Cirimotich et al., 2011). Accordingly, in An. gambiae, blood digestion increases catalase expression and activity in the midgut epithelium and catalase knockdown turns the mosquito more resistant to P. falciparum infection (Molina-Cruz et al., 2008). In contrast, in An. aquasalis, infection with Plasmodium vivax is increased upon catalase silencing (Bahia et al., 2013). Along with the positive effect of ROS on T. cruzi development in triatomine bugs discussed above (Nogueira et al., 2015), this report on P. vivax and An. aquasalis highlight the complexity of the links between redox homeostasis and parasite/host relationship, which is not explained by a simplistic microbe-killing role of ROS. Even in the several reports mentioned above where ROS levels are inversely correlated with pathogen infection (such as in the Aedes/arbovirus), it is not completely clear how much these ROS are produced under the control of canonical immune signaling pathways and how much is derived from the “regular” physiology, such as handling of heme and iron intake, control of microbiota, muscular activity or reticulum stress.
Reproductive Physiology and Hormonal Regulation
Vertebrate blood-feeding is a decisive evolutionary trait needed to obtain nutrients for egg development. Different species vary dramatically in their reproductive output. Some insects, like mosquitoes, are able to lay hundreds of eggs each time they take a blood meal and this feature impacts deeply their density in endemic areas (Shaw and Catteruccia, 2019). The reproductive fitness of vectors represents a promising target to prevent disease transmission because it interferes directly with the burden caused by large populations. Nonetheless, there is evidence that these organisms balance their energy investment into different life processes, often leading to fitness trade-offs between survival, immunity, and reproduction (Schwenke et al., 2016). Therefore, biological pathways essential for reproductive fitness directly or indirectly influence elements that govern vectorial capacity.
In general, it is considered that activation of immune responses decreases reproductive output in a diverse array of insects. Amongst blood-feeding vectors, parasite-induced fecundity reduction is a strategy that is evident in many vector/parasite associations (Hurd, 2003). In malaria-mosquito systems, a challenge with bacterial components or Plasmodium infection promotes apoptosis of follicle cells and reduces the accumulation of protein in the ovaries, as well as the number of eggs laid (Hogg and Hurd, 1995; Ahmed et al., 2002; Ahmed and Hurd, 2006; Pigeault and Villa, 2018). An immune-mediated arrest of oogenesis was also reported in other disease vectors such as the triatomine bug R. prolixus and tsetse flies (Hu et al., 2008; de Medeiros et al., 2009), suggesting resource allocation toward immunity to achieve recovery from infection. Besides, it is also true that reproductively active insects have reduced resistance to infection (Schwenke et al., 2016). In mosquitoes, it was shown that the same molecular processes involved in delivering blood-acquired nutrients to maturing eggs also favor the development of Plasmodium oocysts in the midgut and diminish the efficiency of parasite killing by the mosquito immune system (Rono et al., 2010). Recently, it was shown that transgenic An. gambiae mosquitoes with reduced reproductive capacity have a significantly higher malaria transmission potential, due to an increase in parasite growth rates (Shaw et al., 2020). Due to the direct implications in currently proposed control strategies (e.g., eggless mosquitoes for population suppression) and the vacancy of descriptions of resource reallocation mechanisms in other insect vector species, this subject deserves a greater deal of attention in the field.
Hormonal control is a critical mechanism for the physiological trade-off between reproduction and immunity. JH and 20-Hydroxyecdysone (20E) are key regulators of metamorphosis and reproduction in all holometabolous insects (as reviewed by Roy et al., 2018). Specifically, the balance between JH and 20E is essential for egg maturation. In most insects, increased JH levels promote egg production and provisioning and, in contrast, high 20E titers result in the resorption of immature vitellogenic eggs (Gruntenko and Rauschenbach, 2008). However, in female mosquitoes, digestion and ovarian development are physiologically integrated through a cascade of ecdysteroid signaling initiated after a blood meal (Hansen et al., 2014). Beyond the induction of synthesis and secretion of yolk protein precursors in the fat body, 20E is shown to regulate a number of additional genes that could impact parasite development in different species. In D. melanogaster, ecdysone triggers a precise signaling pathway shown to modulate expression levels of antimicrobial peptides and interfere with resistance mechanisms in the context of bacterial infections (Flatt et al., 2008; Rus et al., 2013). The chemical inhibition of ecdysone signaling in the blood-feeding triatomine R. prolixus is able to suppress cellular and humoral immune responses, disrupting gut microbial homeostasis (de Azambuja et al., 1991; Vieira et al., 2021).
Anopheline mosquitoes are a unique model for ecdysone studies due to their strict anautogeny and male transfer of 20E to females during a monandrous copulation. The mating-induced increase in oogenesis is mediated by vitellogenic lipid transporters that also facilitate Plasmodium development by reducing the parasite-killing ability (Rono et al., 2010). Additionally, mating affects longevity and induces changes in the midgut that can increase susceptibility to the parasite (Dao et al., 2010; Dahalan et al., 2019). In contrast, the topical application of a 20E agonist shortens lifespan, prevents mating and egg production, and significantly blocks P. falciparum development (Childs et al., 2016). Therefore, 20E exerts a long-range regulation of multiple physiological processes that are highly relevant to the mosquito’s competence to transmit malaria: reproductive success, parasite development, and longevity. Recently, a direct influence of 20E on cellular immune function and antipathogen immunity in mosquitoes was demonstrated. Blood-feeding of An. gambiae females or direct 20E injections increase phagocytic activity and this ecdysone-mediated immune priming reduces bacteria and Plasmodium berghei survival (Reynolds et al., 2020). However, some argue that in natural settings, the coevolution of parasite and vector has led to a less conflicting relationship, in which the immune response is toned down, and the potential cost of infection for invertebrate hosts is minimized (Mitchell and Catteruccia, 2017). Werling et al. (2019) provided evidence of a positive correlation between mosquito and parasite fitness dependent on 20E signaling. By genetic ablation of ovary development and impairment of 20E endogenous production, it was determined that ecdysone signaling is required for P. falciparum development via the production of mosquito host-derived lipids (Werling et al., 2019). This supports a model where the provision of lipid molecules during vitellogenesis is used by the parasite to increase survival and optimize its transmission (Costa et al., 2018). Therefore, the intricate interplay mediated by 20E between insect reproductive physiology and parasite development remains partially unresolved. It is possible that ecdysone signaling has tissue and/or threshold-specific actions, enabling the establishment of infection while boosting anti-parasite responses through distinct mechanisms. To warrant a proper impact in pathogen transmission of future discoveries, further research should ideally consider field/natural conditions and be focused on parasite-vector based combinations that occur in the wild.
Juvenile hormones control almost every aspect of insect’s life. The seminal studies of Wigglesworth, started in the early 1930s, established the existence and major roles of JH in insects, regulating tissue morphogenesis, vitellogenesis and immune response, acting primarily as an ‘inhibitory hormone’ (Wigglesworth, 1965). Additionally, strong evidence across a range of insect taxa endorse the model in which mating increases JH titers and suppresses 20E, promoting egg development and inhibiting immune capability (Schwenke et al., 2016). Much of what we know of the molecular regulation of JH in blood-feeding species comes from studies in Ae. aegypti. Here, the rate of JH synthesis in young stages of mosquitoes is in close correlation with their nutritional status (Noriega, 2004). JH also has essential functions in adult females where it controls post-eclosion maturation, leads to reproductive competency and ability to feed on blood, and regulates gene expression after blood-feeding (Clifton and Noriega, 2011; Roy et al., 2015). Aside from morphological alterations in the ovary, transcriptional JH-induced changes in the fat body render this tissue competent to respond to ecdysone produced by the ovary after blood-feeding (Zou et al., 2013). Moreover, JH is delivered by Ae. aegypti males during mating, which increases egg development by directing nutritional resources toward reproduction (Klowden and Chambers, 1991; Clifton et al., 2014). In Drosophila, a similar mating-induced expression of JH results in a remodeling of the female midgut, leading to cell division, increased organ size and ultimately a higher food intake (Reiff et al., 2015). However, in mosquitoes, a midgut-remodeling process in mated females has not been addressed yet. This event is relevant in disease vectors because it could be aimed to favor nutrient absorption toward egg provisioning, but also benefit pathogen development by deviating resources from immunity and supporting a higher parasite count due to an expanded gut area. JH is transported to target tissues by the hemolymph carrier juvenile hormone-binding protein (JHBP) where it binds to the methoprene-tolerant (Met) receptor and exerts its pleiotropic effects. One of the overall processes affected by Met depletion in mosquitoes is lipid metabolism (Wang et al., 2017), which could have a deep impact on the mounting of immune responses to pathogens and parasite maturation (Cheon et al., 2006). Recently, Kim et al. (2020) described a specific role for JH, through JHBP mutation, in regulating innate immune responses and the development of hemocytes in Ae. aegypti. JHBP-deficient mosquitoes are immunosuppressed at the humoral and cellular levels, and present a severe susceptibility to bacterial infection (Kim et al., 2020). These results call for more detailed studies exploring the role of JH signaling on vector infection by disease pathogens.
Blood-feeding initiates a complex series of physiological events in the gut, fat body and ovary that are integrated by the actions of JH, 20E and peptide hormones. Among the topics that could earn further exploration, the modulating role of sex hormones and their effects on non-reproductive organs are poorly understood in blood-feeding insects. Recently, in Drosophila, it was proven that an ecdysone-dependent signaling from the ovaries to the gut promotes growth of the intestine (Ahmed et al., 2020). In addition, the insect midgut produces certain hormones when it recognizes harmful components or pathogenic bacteria in an ingested meal; concurrently, these hormones regulate other tissues and organs (as reviewed in Wu et al., 2020). Given that blood digestion, parasite development and vitellogenesis require the coordination of molecular events in three different abdominal tissues, these inter-organ relationships have relevance in the context of vector–parasite interactions and deserve further attention. Furthermore, between insects of the different taxa, the diversity in life history traits may lead to distinctive adaptations of these systems (Schwenke et al., 2016) and the study of reproductive processes with respect to species-specific features can help the identification of novel targets for vector control.
Multiple Blood-Feedings
The competence to support pathogen development varies between vectors due to many biological aspects, which include not only immunity but also feeding behavior and nutritional status (Lefèvre et al., 2013). Frequency of feeding is undisputedly an important factor in relation to human infections with insect-transmitted diseases (Kramer and Ebel, 2003; Das et al., 2017). Multiple blood meals can increase vectorial capacity by promoting the contact of the disease-carrying insect with susceptible hosts. In the study of vector biology, it is widely accepted for blood-feeding dipterans that host seeking is halted by a full blood meal (Klowden, 1990). However, a number of observations where gravid females still display host-seeking behavior motivated a reconsideration of this assumption (Scott et al., 1993; Guzman et al., 1994; Beier, 1996; Matthews et al., 2016). As most vectors of diseases need to take additional blood meals after becoming infected to complete the transmission cycle, pathogens may have evolved mechanisms to promote their success, redefining the role of uninfected blood-feedings in the epidemiology of these diseases.
One of the classic entomological parameters used in malaria transmission models rely on the proportion of bites experienced per person and the number of total bites taken by a mosquito per gonotrophic cycle (Tedrow et al., 2019). Due to their low reserves, anophelines frequently seek more than one blood meal at each oviposition cycle (Beier, 1996). This behavioral aspect of Anopheles females appears to increase not only fecundity, but also longevity and resistance to insecticides (Oliver and Brooke, 2017). Interestingly, the development of the malaria parasite seems to equally affect and be affected by frequent blood meals, which accelerate oocyst maturation and sporozoite development (Beier et al., 1989; Ponnudurai et al., 1989), and are induced by pathogen–vector manipulation to further enhance transmission (Cator et al., 2012). Shaw et al. (2020) proved that previously infected An. gambiae females, when provided a second uninfected blood meal, present an increase in oocyst growth rates and faster accumulation of sporozoites in the salivary glands, which can indeed amplify local malaria transmission potential (Shaw et al., 2020). Therefore, this previously overlooked multiple feeding behavior is a justifiable current trend of investigation in vector-borne pathogen transmission.
In the phlebotomine sand fly species Lutzomyia, a higher proportion of insects heavily infected can be found after the second blood meal (Moraes et al., 2018). Also, the subsequential feeding induces a faster proliferation of Leishmania parasite infective forms and its rapid migration to the vector proboscis, increasing vectorial competence during the second gonotrophic cycle (Elnaiem et al., 1994; Vivenes et al., 2001). Furthermore, it has been recently proven that the taking of a second uninfected blood meal by Leishmania-infected sand flies triggers a specialized developmental stage of the parasites, the retroleptomonad promastigotes, which is a replicative form and amplifies both the host infection and the infectiveness of the bite (Serafim et al., 2018).
As previously mentioned, blood-feeding triggers intense physiological changes to the gut tissue – including mechanical distention of the midgut, altered cell homeostasis, and changed permeability of the basal lamina – that could aid pathogen dissemination out of the midgut (Okuda et al., 2007; Dong et al., 2017; Taracena et al., 2018). Indeed, in the mosquito Ae. aegypti, stretching of the gut tissue over consecutive blood-feedings seems to be a critical factor causing the midgut basal lamina to become permissive for viral escape (Kantor et al., 2018; Cui et al., 2019). Due to blood-feeding-induced microperforations in the basal lamina, virus-infected individuals fed an additional non-infectious blood meal disseminate and transmit viruses more efficiently than single-fed mosquitoes (Armstrong et al., 2020). It is interesting to note that the previous discussed transfer of male reproductive gland substances during mating in Ae. aegypti can increase blood-feeding frequency, potentially affecting pathogen transmission by female mosquitoes (Villarreal et al., 2018). One could simply postulate that the enhanced or accelerated parasite/pathogen development upon a sequential feeding is due to the higher availability of nutrients. However, other hypotheses can explain this phenomenon, like the aforementioned diversion of energy from the immune response to support oogenesis, and further evolutionary adaptations exploited by the parasite that favor their own development and transmission.
One of the most intriguing propositions in this field is that vector-borne parasites directly manipulate phenotypic traits of their vectors and hosts in ways that increase the contact between them, hence favoring transmission. Major observational examples include Plasmodium, Leishmania, and Trypanosoma spp. manipulating the behavior of mosquitoes, sand flies and kissing bugs, respectively (Hurd, 2003). Frequently studied changes include alterations of biting rates in vectors and increased attractiveness of vertebrate hosts (Lefèvre and Thomas, 2008). Moreover, interesting direct evidence suggests that parasite infection reduces the insect feeding efficiency, prolonging probing time, thus enhancing the likelihood of infecting multiple hosts during a single feeding cycle (Stierhof et al., 1999; van den Abbeele et al., 2010). According to this hypothesis, in malaria-mosquito systems, vector manipulation by the parasites decreases vertebrate host seeking during the pre-infectious phase, lowering the risk of mosquito death during early parasite development. Once the vectors have become infectious, these proceedings are again increased (Schwartz and Koella, 2001). Hence, mosquitoes harboring transmissible sporozoites would be more likely to bite several people per night (Koella et al., 1998). However, most of the evidence of manipulation comes from avian or rodent model systems and is focused on isolated components of mosquito host-feeding process (e.g., host detection, probing, piercing, blood ingestion and terminating the feed) (Friend and Smith, 1977). Therefore, the complexity of human malaria models makes it difficult to characterize how infection affects this multiple set of behaviors (Cator et al., 2012). Furthermore, when long evolutionary association between specific Plasmodium and Anopheles species combinations are tested, distinct or null alterations are shown (Nguyen et al., 2017; Stanczyk et al., 2019). Overall, behavioral manipulations stand as a complex phenomenon that continues to require careful observations with the use of different methods and multidisciplinary approaches (Lefèvre et al., 2006).
Regardless of the mechanism, vector-borne disease transmission depends on the frequency at which the insect vector bites humans. Therefore, fundamental investigations on the impact of complex vector behaviors, and of the ecology and evolution of vector–pathogen interactions, remain key aspects needed to generate better predictions of disease transmission and of the efficacy of control interventions. The varied transmission strategies evolved by pathogens and the vectors’ behavioral changes induced by them can affect how current control tools work. Moreover, biting frequency and how bites are distributed among different people also can have significant epidemiologic effects (Woolhouse et al., 1997; Cooper et al., 2019). High multiple-feeding rates can explain why reducing vector populations alone is difficult for prevention and support the argument for additional studies on feeding behavior (Harrington et al., 2014). Given that multiple blood-feedings directly increase the number of potentially infective encounters, this impact should be considered on model predictions and, accordingly, shape specific vector control strategies. This could mitigate the possibility of underestimating transmission intensity, which could lead to a misunderstanding of the impact of vector control (Tedrow et al., 2019).
Concluding Remarks
The growing impact of vector-borne diseases urgently calls for the development of new entomological interventions. Increasing knowledge on insect biology and insect–pathogen interactions that help unravel the processes that determine vectorial capacity will fuel innovative approaches to stop transmission (Shaw and Catteruccia, 2019). Insect hosts can resist infection or limit/tolerate the deleterious effects caused by the pathogen. Tolerance refers to all host defense mechanisms that limit ‘damage to functions and structures’ during infection, without interfering with pathogen load, as defined more than 60 years ago by plant pathologists (Caldwell et al., 1958). Therefore, resistance has a negative effect on pathogen fitness, whereas tolerance does not. The genetic trade-off between resistance and tolerance can shape the successful evolutionary interactions in a vector-pathogen system (Lambrechts and Saleh, 2019; Oliveira et al., 2020).
Here, we strengthen the argument that the insect vector response to infection does not merely activate immune pathways as a mechanism of resistance. It also encompasses a broad range of adaptive consequences, including metabolic alterations, stress responses, and tissue repair. Many of these are related to blood-feeding and reproduction (Figure 1). These events can lead to improved survival of the insect despite active pathogen replication. The impact of infection on the vector can thus be tuned by the parasite to favor both physiological host homeostasis and completion of the transmission cycle. Moreover, the target for natural selection is seldom one isolated organ or a discrete event, but rather are the multisystemic processes that involve pathogen acquisition and development within the vector. Despite recent advances in the knowledge of physiological mechanisms that can work as non-canonical immunological traits, several elements have yet to be unfolded. Future novel vector control strategies may arise rooted in integrated system biology research to target physiological aspects that act as protective mechanisms and contribute to tolerance to infection. In this way, innovative and effective tools can be used, in an evolutionary considerate manner, to mitigate the great burden imposed on societies by vector-borne diseases.
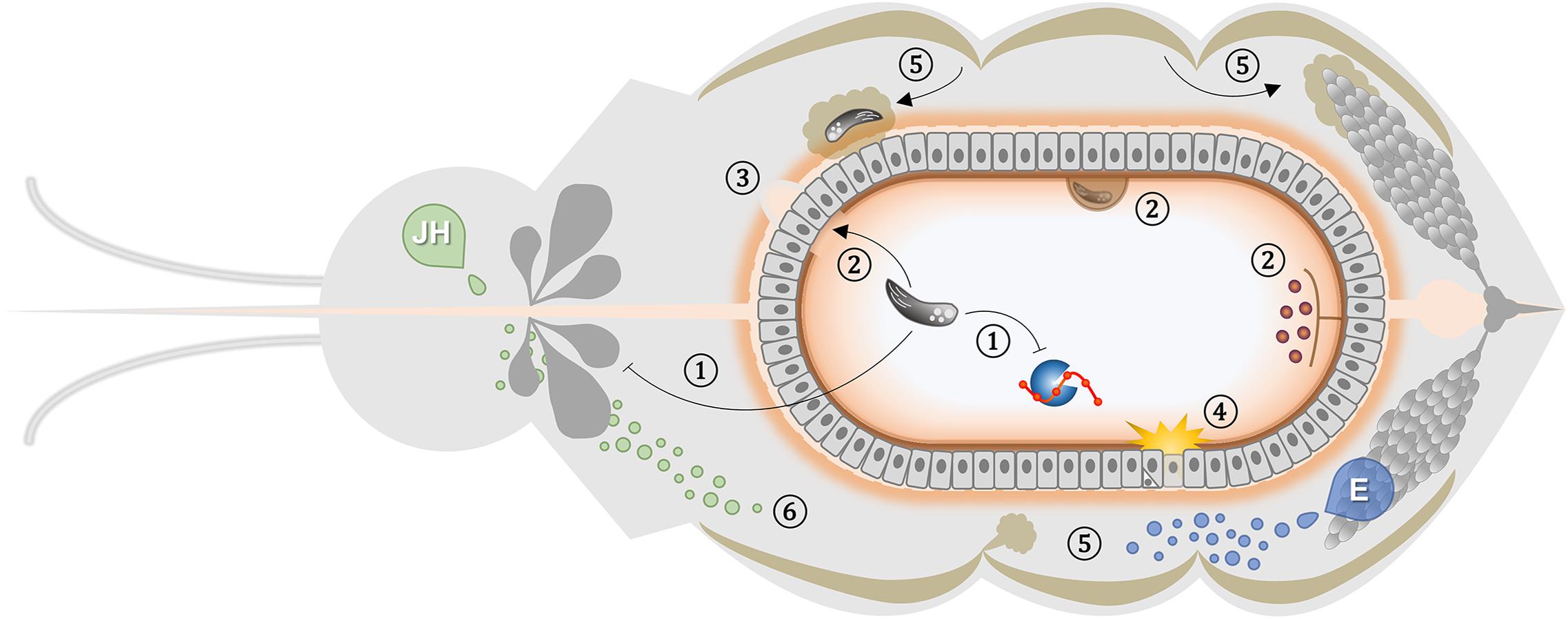
Figure 1. Generic representation of non-immune elements that affect vector-pathogen interaction. The scheme depicts a frontal plane of a blood-feeding insect showing the relative position and interactions of the different non-immune players involved in diverse parasite-vector associations. Organ and cell sizes are not up to scale. (1) Parasites can manipulate feeding rate and digestion activity of the host (Stierhof et al., 1999; Sant’Anna et al., 2009). (2) Peritrophic matrix regulates intestinal infection, requiring parasite escape to establish invasion (Huber et al., 1991; Rose et al., 2020); allowing parasite anchoring to ectoperitrophic space (Pimenta et al., 1997; Zhang et al., 2015); and compartmentalizing microbiota to ensure immune ignorance of the epithelium (Weiss et al., 2014). (3) Blood-feeding-induced microperforations in the basal lamina support pathogen dissemination (Kantor et al., 2018; Armstrong et al., 2020). (4) Rupture of the peritrophic matrix barrier activates ROS generation that triggers an epithelial response to infection (Oliveira et al., 2011a; Taracena et al., 2018). Juvenile Hormone (JH) and Ecdysone (E) are key regulators of the physiological trade-off between reproduction and immunity. (5) Ovary ecdysone production exerts paramount effects such as fat body-induced provision of lipid molecules during vitellogenesis that can reduce the parasite-killing ability and support its development (Rono et al., 2010; Werling et al., 2019). (6) Juvenile hormone influence diverse physiological processes in the insect that can impact pathogen success such as ability to feed on blood, midgut remodeling, reproductive competency, gene expression regulation, lipid metabolism and immune response mounting (Clifton and Noriega, 2011; Zou et al., 2013; Roy et al., 2015; Kim et al., 2020).
Author Contributions
All authors listed have made a substantial, direct and intellectual contribution to the work, and approved it for publication.
Funding
This work was supported by the Conselho Nacional de Desenvolvimento Científico e Tecnológico (CNPq), Coordenação de Aperfeiçoamento de Pessoal de Nível Superior (CAPES), and Fundação de Amparo à Pesquisa do Estado do Rio de Janeiro (FAPERJ).
Conflict of Interest
The authors declare that the research was conducted in the absence of any commercial or financial relationships that could be construed as a potential conflict of interest.
Acknowledgments
The authors would like to thank S. R. Cássia for careful reading of this manuscript.
Abbreviations
TG, triacylglycerol; TGF β, transforming growth factor-beta; ILP, insulin-like peptides; PI3K, phosphoinositide 3-kinase; PKC, protein kinase C; ERK, extracellular signal-regulated kinase; MEK, MAPK/ERK kinase; NF κ B, nuclear factor kappa-light-chain-enhancer of activated B cells; NRF-2, nuclear factor erythroid 2–related factor 2; OXR1, oxidation resistance protein 1; cGMP, cyclic guanosine monophosphate.
References
Ahlers, L. R. H., Trammell, C. E., Carrell, G. F., Mackinnon, S., Torrevillas, B. K., Chow, C. Y., et al. (2019). Insulin potentiates JAK/STAT signaling to broadly inhibit flavivirus replication in insect vectors. Cell Rep. 29, 1946.e5–1960.e5. doi: 10.1016/j.celrep.2019.10.029
Ahmed, A. M., Baggott, S. L., Maingon, R., and Hurd, H. (2002). The costs of mounting an immune response are reflected in the reproductive fitness of the mosquito Anopheles gambiae. Oikos 97, 371–377. doi: 10.1034/j.1600-0706.2002.970307.x
Ahmed, A. M., and Hurd, H. (2006). Immune stimulation and malaria infection impose reproductive costs in Anopheles gambiae via follicular apoptosis. Microbes Infect. 8, 308–315. doi: 10.1016/j.micinf.2005.06.026
Ahmed, S. M. H., Maldera, J. A., Krunic, D., Paiva-Silva, G. O., Pénalva, C., Teleman, A. A., et al. (2020). Fitness trade-offs incurred by ovary-to-gut steroid signalling in Drosophila. Nature 584, 415–419. doi: 10.1038/s41586-020-2462-y
Akman-Anderson, L., Olivier, M., and Luckhart, S. (2007). Induction of nitric oxide synthase and activation of signaling proteins in Anopheles mosquitoes by the malaria pigment, hemozoin. Infect. Immun. 75, 4012–4019. doi: 10.1128/IAI.00645-07
Aksoy, S. (2019). Tsetse peritrophic matrix influences for trypanosome transmission. J. Insect Physiol. 118:103919. doi: 10.1016/j.jinsphys.2019.103919
Alves, C. R., Albuquerque-Cunha, J. M., Mello, C. B., Garcia, E. S., Nogueira, N. F., Bourguingnon, S. C., et al. (2007). Trypanosoma cruzi: Attachment to perimicrovillar membrane glycoproteins of Rhodnius prolixus. Exp. Parasitol. 116, 44–52. doi: 10.1016/j.exppara.2006.11.012
Angleró-Rodríguez, Y. I., MacLeod, H. J., Kang, S., Carlson, J. S., Jupatanakul, N., and Dimopoulos, G. (2017a). Aedes aegypti molecular responses to zika virus: modulation of infection by the toll and Jak/Stat immune pathways and virus host factors. Front. Microbiol. 8:2050. doi: 10.3389/fmicb.2017.02050
Angleró-Rodríguez, Y. I., Talyuli, O. A. C., Blumberg, B. J., Kang, S., Demby, C., Shields, A., et al. (2017b). An Aedes aegypti-associated fungus increases susceptibility to dengue virus by modulating gut trypsin activity. eLife 6, 1–20. doi: 10.7554/eLife.28844
Armstrong, P. M., Ehrlich, H. Y., Magalhaes, T., Miller, M. R., Conway, P. J., Bransfield, A., et al. (2020). Successive blood meals enhance virus dissemination within mosquitoes and increase transmission potential. Nat. Microbiol. 5, 239–247. doi: 10.1038/s41564-019-0619-y
Atella, G. C., Bittencourt-Cunha, P. R., Nunes, R. D., Shahabuddin, M., and Silva-Neto, M. A. C. (2009). The major insect lipoprotein is a lipid source to mosquito stages of malaria parasite. Acta Trop. 109, 159–162. doi: 10.1016/j.actatropica.2008.10.004
Ayres, J. S., Freitag, N., and Schneider, D. S. (2008). Identification of Drosophila mutants altering defense of and endurance to listeria monocytogenes infection. Genetics 178, 1807–1815. doi: 10.1534/genetics.107.083782
Azambuja, P., Garcia, E. S., Waniek, P. J., Vieira, C. S., Figueiredo, M. B., Gonzalez, M. S., et al. (2017). Rhodnius prolixus: from physiology by Wigglesworth to recent studies of immune system modulation by Trypanosoma cruzi and Trypanosoma rangeli. J. Insect Physiol. 97, 45–65. doi: 10.1016/j.jinsphys.2016.11.006
Bahia, A. C., Oliveira, J. H. M., Kubota, M. S., Araújo, H. R. C., Lima, J. B. P., Ríos-Velásquez, C. M., et al. (2013). The role of reactive oxygen species in Anopheles aquasalis response to Plasmodium vivax infection. PLoS One 8:e57014. doi: 10.1371/journal.pone.0057014
Baia-da-Silva, D. C., Alvarez, L. C. S., Lizcano, O. V., Costa, F. T. M., Lopes, S. C. P., Orfanó, A. S., et al. (2018). The role of the peritrophic matrix and red blood cell concentration in Plasmodium vivax infection of Anopheles aquasalis. Parasit. Vectors 11:148. doi: 10.1186/s13071-018-2752-5
Barillas-Mury, C. V., Noriega, F. G., and Wells, M. A. (1995). Early trypsin activity is part of the signal transduction system that activates transcription of the late trypsin gene in the midgut of the mosquito, Aedes aegypti. Insect Biochem. Mol. Biol. 25, 241–246. doi: 10.1016/0965-1748(94)00061-L
Barletta, A. B. F., Alves, L. R., Nascimento Silva, M. C. L., Sim, S., Dimopoulos, G., Liechocki, S., et al. (2016). Emerging role of lipid droplets in Aedes aegypti immune response against bacteria and Dengue virus. Sci. Rep. 6:19928. doi: 10.1038/srep19928
Barletta, A. B. F., Alves e Silva, T. L., Talyuli, O. A. C., Luna-Gomes, T., Sim, S., Angleró-Rodríguez, Y., et al. (2020). Prostaglandins regulate humoral immune responses in Aedes aegypti. PLoS Negl. Trop. Dis. 14:e0008706. doi: 10.1371/journal.pntd.0008706
Barletta, A. B. F., Trisnadi, N., Ramirez, J. L., and Barillas-Mury, C. (2019). Mosquito midgut prostaglandin release establishes systemic immune priming. iScience 19, 54–62. doi: 10.1016/j.isci.2019.07.012
Barral-Netto, M., Barral, A., Brownell, C., Skeiky, Y., Ellingsworth, L., Twardzik, D., et al. (1992). Transforming growth factor-beta in leishmanial infection: a parasite escape mechanism. Science 257, 545–548. doi: 10.1126/science.1636092
Batista, K. K., da, S., Vieira, C. S., Florentino, E. B., Caruso, K. F. B., Teixeira, P. T. P., et al. (2020). Nitric oxide effects on Rhodnius prolixus’s immune responses, gut microbiota and Trypanosoma cruzi development. J. Insect Physiol. 126:104100. doi: 10.1016/j.jinsphys.2020.104100
Beier, J. C. (1996). Frequent blood-feeding and restrictive sugar-feeding behavior enhance the malaria vector potential of Anopheles gambiae s.l. and An. funestus (Diptera: Culicidae) in Western Kenya. J. Med. Entomol. 33, 613–618. doi: 10.1093/jmedent/33.4.613
Beier, J. C., Oster, C. N., Koros, J. K., Onyango, F. K., Githeko, A. K., Rowton, E., et al. (1989). Effect of human circumsporozoite antibodies in Plasmodium-infected Anopheles (Diptera: Culicidae). J. Med. Entomol. 26, 547–553. doi: 10.1093/jmedent/26.6.547
Billingsley, P. F., and Rudin, W. (1992). The role of the mosquito peritrophic membrane in bloodmeal digestion and infectivity of Plasmodium species. J. Parasitol. 78, 430–440. doi: 10.2307/3283640
Borges, E. C., Machado, E. M. M., Garcia, E. S., and Azambuja, P. (2006). Trypanosoma cruzi: effects of infection on cathepsin D activity in the midgut of Rhodnius prolixus. Exp. Parasitol. 112, 130–133. doi: 10.1016/j.exppara.2005.09.008
Bottino-Rojas, V., Pereira, L. O. R., Silva, G., Talyuli, O. A. C., Dunkov, B. C., Oliveira, P. L., et al. (2019). Non-canonical transcriptional regulation of heme oxygenase in Aedes aegypti. Sci. Rep. 9, 1–12. doi: 10.1038/s41598-019-49396-3
Bottino-Rojas, V., Talyuli, O. A. C., Carrara, L., Martins, A. J., James, A. A., Oliveira, P. L., et al. (2018). The redox-sensing gene Nrf2 affects intestinal homeostasis, insecticide resistance, and Zika virus susceptibility in the mosquito Aedes aegypti. J. Biol. Chem. 293, 9053–9063. doi: 10.1074/jbc.RA117.001589
Bottino-Rojas, V., Talyuli, O. A. C., Jupatanakul, N., Sim, S., Dimopoulos, G., Venancio, T. M., et al. (2015). Heme signaling impacts global gene expression, immunity and dengue virus infectivity in Aedes aegypti. PLoS One 10:e0135985. doi: 10.1371/journal.pone.0135985
Brackney, D. E., Isoe, J., Black, W. C., Zamora, J., Foy, B. D., Miesfeld, R. L., et al. (2010). Expression profiling and comparative analyses of seven midgut serine proteases from the yellow fever mosquito, Aedes aegypti. J. Insect Physiol. 56, 736–744. doi: 10.1016/j.jinsphys.2010.01.003
Brackney, D. E., Olson, K. E., and Foy, B. D. (2008). The effects of midgut serine proteases on dengue virus type 2 infectivity of Aedes aegypti. Am. J. Trop. Med. Hyg. 79, 267–274. doi: 10.4269/ajtmh.2008.79.267
Brandon, M. C., Pennington, J. E., Isoe, J., Zamora, J., Schillinger, A.-S., and Miesfeld, R. L. (2008). TOR signaling is required for amino acid stimulation of early trypsin protein synthesis in the midgut of Aedes aegypti mosquitoes. Insect Biochem. Mol. Biol. 38, 916–922. doi: 10.1016/j.ibmb.2008.07.003
Brown, M. R., Clark, K. D., Gulia, M., Zhao, Z., Garczynski, S. F., Crim, J. W., et al. (2008). An insulin-like peptide regulates egg maturation and metabolism in the mosquito Aedes aegypti. Proc. Natl. Acad. Sci. U.S.A. 105, 5716–5721. doi: 10.1073/pnas.0800478105
Buchon, N., Broderick, N. A., and Lemaitre, B. (2013). Gut homeostasis in a microbial world: insights from Drosophila melanogaster. Nat. Rev. Microbiol. 11, 615–626. doi: 10.1038/nrmicro3074
Buchon, N., Broderick, N. A., Poidevin, M., Pradervand, S., and Lemaitre, B. (2009). Drosophila intestinal response to bacterial infection: activation of host defense and stem cell proliferation. Cell Host Microbe 5, 200–211. doi: 10.1016/j.chom.2009.01.003
Buzza, M. S., Netzel-Arnett, S., Shea-Donohue, T., Zhao, A., Lin, C. Y., List, K., et al. (2010). Membrane-anchored serine protease matriptase regulates epithelial barrier formation and permeability in the intestine. Proc. Natl. Acad. Sci. U.S.A. 107, 4200–4205. doi: 10.1073/pnas.0903923107
Caldwell, R. M., Schafer, J. F., Compton, L. E., and Patterson, F. L. (1958). Tolerance to cereal leaf rusts. Science 128, 714–715. doi: 10.1126/science.128.3326.714
Canavoso, L. E., Jouni, Z. E., Karnas, K. J., Pennington, J. E., and Wells, M. A. (2001). Fat metabolism in insects. Annu. Rev. Nutr. 21, 23–46. doi: 10.1146/annurev.nutr.21.1.23
Casadevall, A., and Pirofski, L. (1999). Host-pathogen interactions: redefining the basic concepts of virulence and pathogenicity. Infect. Immun. 67, 3703–3713. doi: 10.1128/IAI.67.8.3703-3713.1999
Castillo, J., Brown, M. R., and Strand, M. R. (2011). Blood feeding and insulin-like peptide 3 stimulate proliferation of hemocytes in the mosquito Aedes aegypti. PLoS Pathog. 7:e1002274. doi: 10.1371/journal.ppat.1002274
Castro, D. P., Moraes, C. S., Gonzalez, M. S., Ratcliffe, N. A., Azambuja, P., and Garcia, E. S. (2012). Trypanosoma cruzi immune response modulation decreases microbiota in Rhodnius prolixus gut and is crucial for parasite survival and development. PLoS One 7:e36591. doi: 10.1371/journal.pone.0036591
Cator, L. J., Lynch, P. A., Read, A. F., and Thomas, M. B. (2012). Do malaria parasites manipulate mosquitoes? Trends Parasitol. 28, 466–470. doi: 10.1016/j.pt.2012.08.004
Chege, G. M. M., Pumpuni, C. B., and Beier, J. C. (1996). Proteolytic enzyme activity and Plasmodium falciparum sporogonic development in three species of Anopheles mosquitoes. J. Parasitol. 82:11. doi: 10.2307/3284108
Cheon, H. M., Sang, W. S., Bian, G., Park, J. H., and Raikhel, A. S. (2006). Regulation of lipid metabolism genes, lipid carrier protein lipophorin, and its receptor during immune challenge in the mosquito Aedes aegypti. J. Biol. Chem. 281, 8426–8435. doi: 10.1074/jbc.M510957200
Childs, L. M., Cai, F. Y., Kakani, E. G., Mitchell, S. N., Paton, D., Gabrieli, P., et al. (2016). Disrupting mosquito reproduction and parasite development for malaria control. PLoS Pathog. 12:e1006060. doi: 10.1371/journal.ppat.1006060
Chotiwan, N., Andre, B. G., Sanchez-Vargas, I., Islam, M. N., Grabowski, J. M., Hopf-Jannasch, A., et al. (2018). Dynamic remodeling of lipids coincides with dengue virus replication in the midgut of Aedes aegypti mosquitoes. PLoS Pathog. 14:e1006853. doi: 10.1371/journal.ppat.1006853
Chovatiya, R., and Medzhitov, R. (2014). Stress, inflammation, and defense of homeostasis. Mol. Cell 54, 281–288. doi: 10.1016/j.molcel.2014.03.030
Chu, J. J. H., Leong, P. W. H., and Ng, M. L. (2006). Analysis of the endocytic pathway mediating the infectious entry of mosquito-borne flavivirus West Nile into Aedes albopictus mosquito (C6/36) cells. Virology 349, 463–475. doi: 10.1016/j.virol.2006.01.022
Cirimotich, C. M., Dong, Y., Clayton, A. M., Sandiford, S. L., Souza-Neto, J. A., Mulenga, M., et al. (2011). Natural microbe-mediated refractoriness to Plasmodium infection in Anopheles gambiae. Science 332, 855–858. doi: 10.1126/science.1201618
Clark, A. J., and Block, K. (1959). The absence of sterol synthesis in insects. J. Biol. Chem. 234, 2578–2582.
Clifton, M. E., Correa, S., Rivera-Perez, C., Nouzova, M., and Noriega, F. G. (2014). Male Aedes aegypti mosquitoes use JH III transferred during copulation to influence previtellogenic ovary physiology and affect the reproductive output of female mosquitoes. J. Insect Physiol. 64, 40–47. doi: 10.1016/j.jinsphys.2014.03.006
Clifton, M. E., and Noriega, F. G. (2011). Nutrient limitation results in juvenile hormone-mediated resorption of previtellogenic ovarian follicles in mosquitoes. J. Insect Physiol. 57, 1274–1281. doi: 10.1016/j.jinsphys.2011.06.002
Coconnier, M. H., Dlissi, E., Robard, M., Laboisse, C. L., Gaillard, J. L., and Servin, A. L. (1998). Listeria monocytogenes stimulates mucus exocytosis in cultured human polarized mucosecreting intestinal cells through action of listeriolysin O. Infect. Immun. 66, 3673–3681.
Cooper, L., Kang, S. Y., Bisanzio, D., Maxwell, K., Rodriguez-Barraquer, I., Greenhouse, B., et al. (2019). Pareto rules for malaria super-spreaders and super-spreading. Nat. Commun. 10, 1–9. doi: 10.1038/s41467-019-11861-y
Coppens, I. (2013). Targeting lipid biosynthesis and salvage in apicomplexan parasites for improved chemotherapies. Nat. Rev. Microbiol. 11, 823–835. doi: 10.1038/nrmicro3139
Corby-Harris, V., Drexler, A., Watkins de Jong, L., Antonova, Y., Pakpour, N., Ziegler, R., et al. (2010). Activation of Akt signaling reduces the prevalence and intensity of Malaria Parasite infection and lifespan in Anopheles stephensi Mosquitoes. PLoS Pathog 6:e1001003. doi: 10.1371/journal.ppat.1001003
Costa, G., Gildenhard, M., Eldering, M., Lindquist, R. L., Hauser, A. E., Sauerwein, R., et al. (2018). Non-competitive resource exploitation within mosquito shapes within-host malaria infectivity and virulence. Nat. Commun. 9, 1–11. doi: 10.1038/s41467-018-05893-z
Coutinho-Abreu, I. V., Serafim, T. D., Meneses, C., Kamhawi, S., Oliveira, F., and Valenzuela, J. G. (2020). Leishmania infection induces a limited differential gene expression in the sand fly midgut. BMC Genomics 21:608. doi: 10.1186/s12864-020-07025-8
Coutinho-Abreu, I. V., Sharma, N. K., Robles-Murguia, M., and Ramalho-Ortigao, M. (2010). Targeting the midgut secreted PpChit1 reduces leishmania major development in its natural vector, the sand fly Phlebotomus papatasi. PLoS Negl. Trop. Dis. 4:e901. doi: 10.1371/journal.pntd.0000901
Cui, Y., Grant, D. G., Lin, J., Yu, X., and Franz, A. W. E. (2019). Zika virus dissemination from the midgut of Aedes aegypti is facilitated by bloodmeal-mediated structural modification of the midgut basal lamina. Viruses 11:1056. doi: 10.3390/v11111056
Dahalan, F. A., Churcher, T. S., Windbichler, N., and Lawniczak, M. K. N. (2019). The male mosquito contribution towards malaria transmission: Mating influences the Anopheles female midgut transcriptome and increases female susceptibility to human malaria parasites. PLoS Pathog. 15:e1008063. doi: 10.1371/journal.ppat.1008063
Dao, A., Kassogue, Y., Adamou, A., Diallo, M., Yaro, A. S., Traore, S. F., et al. (2010). Reproduction-longevity trade-off in Anopheles gambiae (Diptera: Culicidae). J. Med. Entomol. 47, 769–777. doi: 10.1603/ME10052
Das, S., Muleba, M., Stevenson, J. C., Pringle, J. C., and Norris, D. E. (2017). Beyond the entomological inoculation rate: characterizing multiple blood-feeding behavior and Plasmodium falciparum multiplicity of infection in Anopheles mosquitoes in northern Zambia. Parasit. Vectors 10, 1–13. doi: 10.1186/s13071-017-1993-z
de Azambuja, P., Garcia, E. S., Ratcliffe, N. A., and David Warthen, J. (1991). Immune-depression in Rhodnius prolixus induced by the growth inhibitor, azadirachtin. J. Insect Physiol. 37, 771–777. doi: 10.1016/0022-1910(91)90112-D
De Cicco, N. N. T., Pereira, M. G., Corrêa, J. R., Andrade-Neto, V. V., Saraiva, F. B., Chagas-Lima, A. C., et al. (2012). LDL uptake by Leishmania amazonensis: involvement of membrane lipid microdomains. Exp. Parasitol. 130, 330–340. doi: 10.1016/j.exppara.2012.02.014
de Medeiros, M. N., Belmonte, R., Soares, B. C. C., de Medeiros, L. N., Canetti, C., Freire-de-Lima, C. G., et al. (2009). Arrest of oogenesis in the bug Rhodnius prolixus challenged with the fungus Aspergillus niger is mediated by immune response-derived PGE2. J. Insect Physiol. 55, 151–158. doi: 10.1016/j.jinsphys.2008.10.019
Deplancke, B., and Gaskins, H. R. (2001). Microbial modulation of innate defense: Goblet cells and the intestinal mucus layer. Am. J. Clin. Nutr. 73, 1131S–1141S.
Diaz-Albiter, H., Sant’Anna, M. R. V., Genta, F. A., and Dillon, R. J. (2012). Reactive oxygen species-mediated immunity against Leishmania mexicana and Serratia marcescens in the phlebotomine sand fly Lutzomyia longipalpis. J. Biol. Chem. 287, 23995–24003. doi: 10.1074/jbc.M112.376095
Dinglasan, R. R., Devenport, M., Florens, L., Johnson, J. R., McHugh, C. A., Donnelly-Doman, M., et al. (2009). The Anopheles gambiae adult midgut peritrophic matrix proteome. Insect Biochem. Mol. Biol. 39, 125–134. doi: 10.1016/j.ibmb.2008.10.010
Dong, S., Balaraman, V., Kantor, A. M., Lin, J., Grant, D. A. G., Held, N. L., et al. (2017). Chikungunya virus dissemination from the midgut of Aedes aegypti is associated with temporal basal lamina degradation during bloodmeal digestion. PLoS Negl. Trop. Dis. 11:e0005976. doi: 10.1371/journal.pntd.0005976
Douglas, A. E. (2019). Simple animal models for microbiome research. Nat. Rev. Microbiol. 17, 764–775. doi: 10.1038/s41579-019-0242-1
Drexler, A., Nuss, A., Hauck, E., Glennon, E., Cheung, K., Brown, M., et al. (2013). Human IGF1 extends lifespan and enhances resistance to Plasmodium falciparum infection in the malaria vector Anopheles stephensi. J. Exp. Biol. 216, 208–217. doi: 10.1242/jeb.078873
Edogawa, S., Edwinson, A. L., Peters, S. A., Chikkamenahalli, L. L., Sundt, W., Graves, S., et al. (2020). Serine proteases as luminal mediators of intestinal barrier dysfunction and symptom severity in IBS. Gut 69, 62–73. doi: 10.1136/gutjnl-2018-317416
Elnaiem, D. A., Ward, R. D., and Young, P. E. (1994). Development of Leishmania chagasi (Kinetoplastida: Trypanosomatidae) in the second blood-meal of its vector Lutzomyia longipalpis (Diptera: Psychodidae). Parasitol. Res. 80, 414–419. doi: 10.1007/BF00932379
Etebari, K., Hegde, S., Saldaña, M. A., Widen, S. G., Wood, T. G., Asgari, S., et al. (2017). Global transcriptome analysis of Aedes aegypti mosquitoes in response to Zika virus infection. mSphere 2, 1648–1659. doi: 10.1128/mSphere.00456-17
Feldman, A. M., Billingsley, P. F., and Savelkoul, E. (1990). Bloodmeal digestion by strains of Anopheles stephensi Liston (Diptera: Culicidae) of differing susceptibility to Plasmodium falciparum. Parasitology 101, 193–200. doi: 10.1017/S003118200006323X
Fernández de Castro, I., Tenorio, R., and Risco, C. (2016). Virus assembly factories in a lipid world. Curr. Opin. Virol. 18, 20–26. doi: 10.1016/j.coviro.2016.02.009
Ferreira, C. M., Stiebler, R., Saraiva, F. M., Lechuga, G. C., Walter-Nuno, A. B., Bourguignon, S. C., et al. (2018). Heme crystallization in a Chagas disease vector acts as a redox-protective mechanism to allow insect reproduction and parasite infection. PLoS Negl. Trop. Dis. 12:e0006661. doi: 10.1371/journal.pntd.0006661
Figueiredo, M. B., Genta, F. A., Garcia, E. S., and Azambuja, P. (2008). Lipid mediators and vector infection: Trypanosoma rangeli inhibits Rhodnius prolixus hemocyte phagocytosis by modulation of phospholipase A2 and PAF-acetylhydrolase activities. J. Insect Physiol. 54, 1528–1537. doi: 10.1016/j.jinsphys.2008.08.013
Flatt, T., Heyland, A., Rus, F., Porpiglia, E., Sherlock, C., Yamamoto, R., et al. (2008). Hormonal regulation of the humoral innate immune response in Drosophila melanogaster. J. Exp. Biol. 211, 2712–2724. doi: 10.1242/jeb.014878
Franz, A., Kantor, A., Passarelli, A., and Clem, R. (2015). Tissue barriers to arbovirus infection in mosquitoes. Viruses 7, 3741–3767. doi: 10.3390/v7072795
Friend, W. G., and Smith, J. J. B. (1977). Factors affecting feeding by bloodsucking insects. Annu. Rev. Entomol. 22, 309–331. doi: 10.1146/annurev.en.22.010177.001521
Fu, Q., Inankur, B., Yin, J., Striker, R., and Lan, Q. (2015). Sterol carrier protein 2, a critical host factor for dengue virus infection, alters the cholesterol distribution in mosquito Aag2 cells. J. Med. Entomol. 52, 1124–1134. doi: 10.1093/jme/tjv101
Gandara, A. C. P., Oliveira, J. H. M., Nunes, R. D., Goncalves, R. L. S., Dias, F. A., Hecht, F., et al. (2016). Amino acids trigger down-regulation of superoxide via TORC pathway in the midgut of Rhodnius prolixus. Biosci. Rep. 36, 916–922. doi: 10.1042/BSR20160061
Gandara, A. C. P., Torres, A., Bahia, A. C., Oliveira, P. L., and Schama, R. (2017). Evolutionary origin and function of NOX4-art, an arthropod specific NADPH oxidase. BMC Evol. Biol. 17:92. doi: 10.1186/s12862-017-0940-0
Garcia, E. S., and Gilliam, F. C. (1980). Trypanosoma cruzi development is independent of protein digestion in the gut of Rhodnius prolixus. J. Parasitol. 66, 1052–1053.
Garcia, E. S., Machado, E. M. M., and Azambuja, P. (2004a). Effects of eicosanoid biosynthesis inhibitors on the prophenoloxidase-activating system and microaggregation reactions in the hemolymph of Rhodnius prolixus infected with Trypanosoma rangeli. J. Insect Physiol. 50, 157–165. doi: 10.1016/j.jinsphys.2003.11.002
Garcia, E. S., Machado, E. M. M., and Azambuja, P. (2004b). Inhibition of hemocyte microaggregation reactions in Rhodnius prolixus larvae orally infected with Trypanosoma rangeli. Exp. Parasitol. 107, 31–38. doi: 10.1016/j.exppara.2004.03.015
Geoghegan, V., Stainton, K., Rainey, S. M., Ant, T. H., Dowle, A. A., Larson, T., et al. (2017). Perturbed cholesterol and vesicular trafficking associated with dengue blocking in Wolbachia-infected Aedes aegypti cells. Nat. Commun. 8:526. doi: 10.1038/s41467-017-00610-8
Golgher, D. B., Colli, W., Souto-Padrón, T., and Zingales, B. (1993). Galactofuranose-containing glycoconjugates of epimastigote and trypomastigote forms of Trypanosoma cruzi. Mol. Biochem. Parasitol. 60, 249–264. doi: 10.1016/0166-6851(93)90136-L
Gonçalves, R. L. S., Oliveira, J. H. M., Oliveira, G. A., Andersen, J. F., Oliveira, M. F., Oliveira, P. L., et al. (2012). Mitochondrial reactive oxygen species modulate mosquito susceptibility to Plasmodium infection. PLoS One 7:e41083. doi: 10.1371/journal.pone.0041083
Gondim, K. C., Atella, G. C., Pontes, E. G., and Majerowicz, D. (2018). Lipid metabolism in insect disease vectors. Insect Biochem. Mol. Biol. 101, 108–123. doi: 10.1016/j.ibmb.2018.08.005
Gonzalez, M. S., Hamedi, A., Albuquerque-Cunha, J. M., Nogueira, N. F. S., De Souza, W., Ratcliffe, N. A., et al. (2006). Antiserum against perimicrovillar membranes and midgut tissue reduces the development of Trypanosoma cruzi in the insect vector, Rhodnius prolixus. Exp. Parasitol. 114, 297–304. doi: 10.1016/j.exppara.2006.04.009
Gonzalez, M. S., Nogueira, N. F. S., Mello, C. B., De Souza, W., Schaub, G. A., Azambuja, P., et al. (1999). Influence of brain and azadirachtin on Trypanosoma cruzi development in the vector, Rhodnius prolixus. Exp. Parasitol. 92, 100–108. doi: 10.1006/expr.1998.4387
Graham, A. L., Allen, J. E., and Read, A. F. (2005). Evolutionary causes and consequences of immunopathology. Annu. Rev. Ecol. Evol. Syst. 36, 373–397. doi: 10.1146/annurev.ecolsys.36.102003.152622
Grigoryeva, L. A. (2010). Morpho-functional changes in the midgut of ixodid ticks (Acari: Ixodidae) during the life cycle. Entomol. Rev. 90, 405–409. doi: 10.1134/S0013873810030073
Gruntenko, N. E., and Rauschenbach, I. Y. (2008). Interplay of JH, 20E and biogenic amines under normal and stress conditions and its effect on reproduction. J. Insect Physiol. 54, 902–908. doi: 10.1016/j.jinsphys.2008.04.004
Gulia-Nuss, M., Robertson, A. E., Brown, M. R., and Strand, M. R. (2011). Insulin-like peptides and the target of rapamycin pathway coordinately regulate blood digestion and egg maturation in the mosquito Aedes aegypti. PLoS One 6:e0020401. doi: 10.1371/journal.pone.0020401
Gupta, L., Kumar, S., Yeon, S. H., Pimenta, P. F. P., and Barillas-Mury, C. (2005). Midgut epithelial responses of different mosquito-Plasmodium combinations: The actin cone zipper repair mechanism in Aedes aegypti. Proc. Natl. Acad. Sci. U.S.A. 102, 4010–4015. doi: 10.1073/pnas.0409642102
Gupta, L., Molina-Cruz, A., Kumar, S., Rodrigues, J., Dixit, R., Zamora, R. E., et al. (2009). The STAT pathway mediates late-phase immunity against Plasmodium in the mosquito Anopheles gambiae. Cell Host Microbe 5, 498–507. doi: 10.1016/j.chom.2009.04.003
Guzman, H., Walters, L. L., and Tesh, R. B. (1994). Histologic detection of multiple blood meals in Phlebotomus duboscqi (Diptera: Psychodidae). J. Med. Entomol. 31, 890–897. doi: 10.1093/jmedent/31.6.890
Ha, E.-M., Oh, C.-T., Bae, Y. S., and Lee, W.-J. (2005a). A direct role for dual oxidase in Drosophila gut immunity. Science 310, 847–850. doi: 10.1126/science.1117311
Ha, E.-M., Oh, C.-T., Ryu, J.-H., Bae, Y.-S., Kang, S.-W., Jang, I.-H., et al. (2005b). An antioxidant system required for host protection against gut infection in Drosophila. Dev. Cell 8, 125–132. doi: 10.1016/j.devcel.2004.11.007
Hagedorn, H. (2004). “Mosquito endocrinology,” in Biology of Disease Vectors, 2nd Edn, ed. W. Marquardt (Amsterdam: Elsevier).
Han, Y. S., Thompson, J., Kafatos, F. C., and Barillas-Mury, C. (2001). Molecular interactions between Anopheles stephensi midgut cells and Plasmodium berghei: the time bomb theory of ookinete invasion of mosquitoes. EMBO J. 20, 1483–1483. doi: 10.1038/sj.emboj.7593651b
Hansen, I. A., Attardo, G. M., Park, J.-H., Peng, Q., and Raikhel, A. S. (2004). Target of rapamycin-mediated amino acid signaling in mosquito anautogeny. Proc. Natl. Acad. Sci. U.S.A. 101, 10626–10631. doi: 10.1073/pnas.0403460101
Hansen, I. A., Attardo, G. M., Rodriguez, S. D., and Drake, L. L. (2014). Four-way regulation of mosquito yolk protein precursor genes by juvenile hormone-, ecdysone-, nutrient-, and insulin-like peptide signaling pathways. Front. Physiol. 5:103. doi: 10.3389/fphys.2014.00103
Harrington, L. C., Fleisher, A., Ruiz-Moreno, D., Vermeylen, F., Wa, C. V., Poulson, R. L., et al. (2014). Heterogeneous feeding patterns of the dengue vector, Aedes aegypti, on individual human hosts in Rural Thailand. PLoS Negl. Trop. Dis. 8:e0003048. doi: 10.1371/journal.pntd.0003048
Hegedus, D. D., Toprak, U., and Erlandson, M. (2019). Peritrophic matrix formation. J. Insect Physiol. 117:103898. doi: 10.1016/j.jinsphys.2019.103898
Henriques, B. S., Gomes, B., da Costa, S. G., da Moraes, C. S., Mesquita, R. D., Dillon, V. M., et al. (2017). Genome wide mapping of peptidases in Rhodnius prolixus: identification of protease gene duplications, horizontally transferred proteases and analysis of peptidase A1 structures, with considerations on their role in the evolution of hematophagy in Triatomi. Front. Physiol. 8:1051. doi: 10.3389/fphys.2017.01051
Henriques, B. S., Gomes, B., Oliveira, P. L., Garcia, E., de, S., Azambuja, P., et al. (2021). Characterization of the temporal pattern of blood protein digestion in Rhodnius prolixus: first description of early and late gut cathepsins. Front. Physiol. 11:509310. doi: 10.3389/fphys.2020.509310
Hogg, J. C., and Hurd, H. (1995). Plasmodium yoelii nigeriensis : the effect of high and low intensity of infection upon the egg production and bloodmeal size of Anopheles stephensi during three gonotrophic cycles. Parasitology 111, 555–562. doi: 10.1017/S0031182000077027
Hooper, L. V. (2009). Do symbiotic bacteria subvert host immunity? Nat. Rev. Microbiol. 7, 367–374. doi: 10.1038/nrmicro2114
Hou, Y., Wang, X. L., Saha, T. T., Roy, S., Zhao, B., Raikhel, A. S., et al. (2015). Temporal coordination of carbohydrate metabolism during mosquito reproduction. PLoS Genet. 11:e1005309. doi: 10.1371/journal.pgen.1005309
Hu, C., Rio, R. V. M., Medlock, J., Haines, L. R., Nayduch, D., Savage, A. F., et al. (2008). Infections with immunogenic trypanosomes reduce tsetse reproductive fitness: potential impact of different parasite strains on vector population structure. PLoS Negl. Trop. Dis. 2:e0000192. doi: 10.1371/journal.pntd.0000192
Huber, M., Cabib, E., and Miller, L. H. (1991). Malaria parasite chitinase and penetration of the mosquito peritrophic membrane. Proc. Natl. Acad. Sci. U.S.A. 88, 2807–2810. doi: 10.1073/pnas.88.7.2807
Hurd, H. (2003). Manipulation of medically important insect vectors by their parasites. Annu. Rev. Entomol. 48, 141–161. doi: 10.1146/annurev.ento.48.091801.112722
Jahan, N., Docherty, P. T., Billingsley, P. F., and Hurd, H. (1999). Blood digestion in the mosquito, Anopheles stephensi: the effects of Plasmodium yoelii nigeriensis on midgut enzyme activities. Parasitology 119, 535–541. doi: 10.1017/S0031182099005090
Janeh, M., Osman, D., and Kambris, Z. (2017). Damage-induced cell regeneration in the midgut of Aedes albopictus mosquitoes. Sci. Rep. 7:44594. doi: 10.1038/srep44594
Jaramillo-Gutierrez, G., Molina-Cruz, A., Kumar, S., and Barillas-Mury, C. (2010). The Anopheles gambiae oxidation resistance 1 (OXR1) gene regulates expression of enzymes that detoxify reactive oxygen species. PLoS One 5:e11168. doi: 10.1371/journal.pone.0011168
Johansson, M. E. V., Sjövall, H., and Hansson, G. C. (2013). The gastrointestinal mucus system in health and disease. Nat. Rev. Gastroenterol. Hepatol. 10, 352–361. doi: 10.1038/nrgastro.2013.35
Jones, D. P. (2006). Redefining oxidative stress. Antioxid. Redox Signal. 8, 1865–1879. doi: 10.1089/ars.2006.8.1865
Jones, D. P., and Sies, H. (2015). The redox code. Antioxid. Redox Signal. 23, 734–746. doi: 10.1089/ars.2015.6247
Junjhon, J., Pennington, J. G., Edwards, T. J., Perera, R., Lanman, J., and Kuhn, R. J. (2014). Ultrastructural characterization and three-dimensional architecture of replication sites in dengue virus-infected mosquito cells. J. Virol. 88, 4687–4697. doi: 10.1128/jvi.00118-14
Jupatanakul, N., Sim, S., Angleró-Rodríguez, Y. I., Souza-Neto, J., Das, S., Poti, K. E., et al. (2017). Engineered Aedes aegypti JAK/STAT pathway-mediated immunity to dengue virus. PLoS Negl. Trop. Dis. 11:e0005187. doi: 10.1371/journal.pntd.0005187
Jupatanakul, N., Sim, S., and Dimopoulos, G. (2014). Aedes aegypti ML and Niemann-Pick type C family members are agonists of dengue virus infection. Dev. Comp. Immunol. 43, 1–9. doi: 10.1016/j.dci.2013.10.002
Kantor, A. M., Grant, D. G., Balaraman, V., White, T. A., and Franz, A. W. E. (2018). Ultrastructural analysis of chikungunya virus dissemination from the midgut of the yellow fever mosquito, Aedes aegypti. Viruses 10:571. doi: 10.3390/v10100571
Kim, I. H., Castillo, J. C., Aryan, A., Martin-Martin, I., Nouzova, M., Noriega, F. G., et al. (2020). A mosquito juvenile hormone binding protein (mJHBP) regulates the activation of innate immune defenses and hemocyte development. PLoS Pathog. 16:e1008288. doi: 10.1371/journal.ppat.1008288
Klenk, H. D., and Garten, W. (1994). Host cell proteases controlling virus pathogenicity. Trends Microbiol. 2, 39–43. doi: 10.1016/0966-842X(94)90123-6
Klowden, M. J. (1990). The endogenous regulation of mosquito reproductive behavior. Experientia 46, 660–670. doi: 10.1007/BF01939928
Klowden, M. J., and Chambers, G. M. (1991). Male accessory gland substances activate egg development in nutritionally stressed Aedes aegypti mosquitoes. J. Insect Physiol. 37, 721–726. doi: 10.1016/0022-1910(91)90105-9
Koella, J. C., Sorensen, F. L., and Anderson, R. A. (1998). The malaria parasite, Plasmodium falciparum, increases the frequency of multiple feeding of its mosquito vector, Anopheles gambiae. Proc. R. Soc. B Biol. Sci. 265, 763–768. doi: 10.1098/rspb.1998.0358
Kotsyfakis, M., Schwarz, A., Erhart, J., and Ribeiro, J. M. C. (2015). Tissue- and time-dependent transcription in Ixodes ricinus salivary glands and midguts when blood-feeding on the vertebrate host. Sci. Rep. 5, 1–10. doi: 10.1038/srep09103
Kounakis, K., Chaniotakis, M., Markaki, M., and Tavernarakis, N. (2019). Emerging roles of lipophagy in health and disease. Front. Cell Dev. Biol. 7:185. doi: 10.3389/fcell.2019.00185
Kramer, L. D., and Ebel, G. D. (2003). Dynamics of flavivirus infection in mosquitoes. Adv. Virus Res. 60, 187–232. doi: 10.1016/S0065-3527(03)60006-0
Kriaa, A., Jablaoui, A., Mkaouar, H., Akermi, N., Maguin, E., and Rhimi, M. (2020). Serine proteases at the cutting edge of IBD: Focus on gastrointestinal inflammation. FASEB J. 34, 7270–7282. doi: 10.1096/fj.202000031RR
Kumar, B. A., and Paily, K. P. (2011). Up-regulation of lipophorin (Lp) and lipophorin receptor (LpR) gene in the mosquito, Culex quinquefasciatus (Diptera: Culicidae), infected with the filarial parasite, Wuchereria bancrofti (Spirurida: Onchocercidae). Parasitol. Res. 108, 377–381. doi: 10.1007/s00436-010-2075-8
Kumar, S., Christophides, G. K., Cantera, R., Charles, B., Han, Y. S., Meister, S., et al. (2003). The role of reactive oxygen species on Plasmodium melanotic encapsulation in Anopheles gambiae. Proc. Natl. Acad. Sci. U.S.A. 100, 14139–14144. doi: 10.1073/pnas.2036262100
Kumar, S., Gupta, L., Yeon, S. H., and Barillas-Mury, C. (2004). Inducible peroxidases mediate nitration of Anopheles midgut cells undergoing apoptosis in response to Plasmodium invasion. J. Biol. Chem. 279, 53475–53482. doi: 10.1074/jbc.M409905200
Kumar, S., Molina-Cruz, A., Gupta, L., Rodrigues, J., and Barillas-Mury, C. (2010). A peroxidase/dual oxidase system modulates midgut epithelial immunity in Anopheles gambiae. Science 327, 1644–1648. doi: 10.1126/science.1184008
Kuraishi, T., Binggeli, O., Opota, O., Buchon, N., and Lemaitre, B. (2011). Genetic evidence for a protective role of the peritrophic matrix against intestinal bacterial infection in Drosophila melanogaster. Proc. Natl. Acad. Sci. U.S.A. 108, 15966–15971. doi: 10.1073/pnas.1105994108
Kuraishi, T., Hori, A., and Kurata, S. (2013). Host-microbe interactions in the gut of Drosophila melanogaster. Front. Physiol. 4:375. doi: 10.3389/fphys.2013.00375
Labaied, M., Jayabalasingham, B., Bano, N., Cha, S. J., Sandoval, J., Guan, G., et al. (2011). Plasmodium salvages cholesterol internalized by LDL and synthesized de novo in the liver. Cell. Microbiol. 13, 569–586. doi: 10.1111/j.1462-5822.2010.01555.x
Lambeth, J. D. (2007). Nox enzymes, ROS, and chronic disease: an example of antagonistic pleiotropy. Free Radic. Biol. Med. 43, 332–347. doi: 10.1016/j.freeradbiomed.2007.03.027
Lambrechts, L., and Saleh, M. C. (2019). Manipulating mosquito tolerance for arbovirus control. Cell Host Microbe 26, 309–313. doi: 10.1016/j.chom.2019.08.005
Lara, F. A., Lins, U., Bechara, G. H., and Oliveira, P. L. (2005). Tracing heme in a living cell: hemoglobin degradation and heme traffic in digest cells of the cattle tick Boophilus microplus. J. Exp. Biol. 208, 3093–3101. doi: 10.1242/jeb.01749
Lee, K.-A., Cho, K.-C., Kim, B., Jang, I.-H., Nam, K., Kwon, Y. E., et al. (2018). Inflammation-modulated metabolic reprogramming is required for DUOX-dependent gut immunity in Drosophila. Cell Host Microbe 23, 338.e5–352.e5. doi: 10.1016/j.chom.2018.01.011
Lefèvre, T., Koella, J. C., Renaud, F., Hurd, H., Biron, D. G., and Thomas, F. (2006). New prospects for research on manipulation of insect vectors by pathogens. PLoS Pathog. 2:e0020072. doi: 10.1371/journal.ppat.0020072
Lefèvre, T., and Thomas, F. (2008). Behind the scene, something else is pulling the strings: Emphasizing parasitic manipulation in vector-borne diseases. Infect. Genet. Evol. 8, 504–519. doi: 10.1016/j.meegid.2007.05.008
Lefèvre, T., Vantaux, A., Dabiré, K. R., Mouline, K., and Cohuet, A. (2013). Non-genetic determinants of mosquito competence for malaria parasites. PLoS Pathog. 9:e1003365. doi: 10.1371/journal.ppat.1003365
Lehane, M. (2005). The Biology of Blood-Sucking in Insects, 2nd Edn. Cambridge, MA: Cambridge University Press.
Lehane, M. J. (1997). Peritrophic matrix structure and function. Annu. Rev. Entomol. 42, 525–550. doi: 10.1146/annurev.ento.42.1.525
Leier, H. C., Weinstein, J. B., Kyle, J. E., Lee, J.-Y., Bramer, L. M., Stratton, K. G., et al. (2020). A global lipid map defines a network essential for Zika virus replication. Nat. Commun. 11:3652. doi: 10.1038/s41467-020-17433-9
Li, Z., Quan, G., Jiang, X., Yang, Y., Ding, X., Zhang, D., et al. (2018). Effects of metabolites derived from gut microbiota and hosts on pathogens. Front. Cell. Infect. Microbiol. 8:314. doi: 10.3389/fcimb.2018.00314
Lim, J., Gowda, D. C., Krishnegowda, G., and Luckhart, S. (2005). Induction of nitric oxide synthase in Anopheles stephensi by Plasmodium falciparum: mechanism of signaling and the role of parasite glycosylphosphatidylinositols. Infect. Immun. 73, 2778–2789. doi: 10.1128/IAI.73.5.2778-2789.2005
Lissner, M. M., and Schneider, D. S. (2018). The physiological basis of disease tolerance in insects. Curr. Opin. Insect Sci. 29, 133–136. doi: 10.1016/j.cois.2018.09.004
Liu, J., Liu, Y., Nie, K., Du, S., Qiu, J., Pang, X., et al. (2016). Flavivirus NS1 protein in infected host sera enhances viral acquisition by mosquitoes. Nat. Microbiol. 1:16087. doi: 10.1038/nmicrobiol.2016.87
Liu, Z., Ren, Z., Zhang, J., Chuang, C. C., Kandaswamy, E., Zhou, T., et al. (2018). Role of ROS and nutritional antioxidants in human diseases. Front. Physiol. 9:477. doi: 10.3389/fphys.2018.00477
Luckhart, S., Giulivi, C., Drexler, A. L., Antonova-Koch, Y., Sakaguchi, D., Napoli, E., et al. (2013). Sustained activation of akt elicits mitochondrial dysfunction to block Plasmodium falciparum infection in the mosquito host. PLoS Pathog. 9:e1003180. doi: 10.1371/journal.ppat.1003180
Luckhart, S., and Rosenberg, R. (1999). Gene structure and polymorphism of an invertebrate nitric oxide synthase gene. Gene 232, 25–34. doi: 10.1016/S0378-1119(99)00121-3
Luckhart, S., Vodovotz, Y., Ciu, L., and Rosenberg, R. (1998). The mosquito Anopheles stephensi limits malaria parasite development with inducible synthesis of nitric oxide. Proc. Natl. Acad. Sci. U.S.A. 95, 5700–5705. doi: 10.1073/pnas.95.10.5700
Matetovici, I., De Vooght, L., and Van Den Abbeele, J. (2019). Innate immunity in the tsetse fly (Glossina), vector of African trypanosomes. Dev. Comp. Immunol. 98, 181–188. doi: 10.1016/j.dci.2019.05.003
Matsuo, T., Sato, M., Inoue, N., Yokoyama, N., Taylor, D., and Fujisaki, K. (2003). Morphological studies on the extracellular structure of the midgut of a tick, Haemaphysalis longicornis (Acari: Ixodidae). Parasitol. Res. 90, 243–248. doi: 10.1007/s00436-003-0833-6
Matthews, B. J., McBride, C. S., DeGennaro, M., Despo, O., and Vosshall, L. B. (2016). The neurotranscriptome of the Aedes aegypti mosquito. BMC Genomics 17:32. doi: 10.1186/s12864-015-2239-0
McCord, J. M., and Fridovich, I. (1969). Superoxide dismutase. An enzymic function for erythrocuprein (hemocuprein). J. Biol. Chem. 244, 6049–6055.
Medzhitov, R., Schneider, D. S., and Soares, M. P. (2012). Disease tolerance as a defense strategy. Science 335, 936–941. doi: 10.1126/science.1214935
Merzendorfer, H., and Zimoch, L. (2003). Chitin metabolism in insects: structure, function and regulation of chitin synthases and chitinases. J. Exp. Biol. 206, 4393–4412. doi: 10.1242/jeb.00709
Micchelli, C. A., and Perrimon, N. (2006). Evidence that stem cells reside in the adult Drosophila midgut epithelium. Nature 439, 475–479. doi: 10.1038/nature04371
Ming, M., Ewen, M. E., and Pereira, M. E. A. (1995). Trypanosome invasion of mammalian cells requires activation of the TGFβ signaling pathway. Cell 82, 287–296. doi: 10.1016/0092-8674(95)90316-X
Mitchell, S. N., and Catteruccia, F. (2017). Anopheline reproductive biology: Impacts on vectorial capacity and potential avenues for malaria control. Cold Spring Harb. Perspect. Med. 7:14. doi: 10.1101/cshperspect.a025593
Molina-Cruz, A., DeJong, R. J., Charles, B., Gupta, L., Kumar, S., Jaramillo-Gutierrez, G., et al. (2008). Reactive oxygen species modulate Anopheles gambiae immunity against bacteria and Plasmodium. J. Biol. Chem. 283, 3217–3223. doi: 10.1074/jbc.M705873200
Molina-Cruz, A., Gupta, L., Richardson, J., Bennett, K., Black, W., and Barillas-Mury, C. (2005). Effect of mosquito midgut trypsin activity on dengue-2 virus infection and dissemination in Aedes aegypti. Am. J. Trop. Med. Hyg. 72, 631–637. doi: 10.4269/ajtmh.2005.72.631
Montezano, A. C., Camargo, L. D. L., Persson, P., Rios, F. J., Harvey, A. P., Anagnostopoulou, A., et al. (2018). NADPH Oxidase 5 Is a pro-contractile nox isoform and a point of cross-talk for calcium and redox signaling-implications in vascular function. J. Am. Heart Assoc. 7, 1–15. doi: 10.1161/JAHA.118.009388
Moraes, C. S., Aguiar-Martins, K., Costa, S. G., Bates, P. A., Dillon, R. J., and Genta, F. A. (2018). Second blood meal by female Lutzomyia longipalpis?: enhancement by oviposition and its effects on digestion, longevity, and leishmania infection. Biomed. Res. Int. 2018, 1–10. doi: 10.1155/2018/2472508
Motta, J. P., Denadai-Souza, A., Sagnat, D., Guiraud, L., Edir, A., Bonnart, C., et al. (2019). Active thrombin produced by the intestinal epithelium controls mucosal biofilms. Nat. Commun. 10, 1–12. doi: 10.1038/s41467-019-11140-w
Narasimhan, S., and Fikrig, E. (2015). Tick microbiome: the force within. Trends Parasitol. 31, 315–323. doi: 10.1016/j.pt.2015.03.010
Narasimhan, S., Schuijt, T. J., Abraham, N. M., Rajeevan, N., Coumou, J., Graham, M., et al. (2017). Modulation of the tick gut milieu by a secreted tick protein favors Borrelia burgdorferi colonization. Nat. Commun. 8, 1–16. doi: 10.1038/s41467-017-00208-0
Ng, C. G., Coppens, I., Govindarajan, D., Pisciotta, J., Shulaev, V., and Griffin, D. E. (2008). Effect of host cell lipid metabolism on alphavirus replication, virion morphogenesis, and infectivity. Proc. Natl. Acad. Sci. U.S.A. 105, 16326–16331. doi: 10.1073/pnas.0808720105
Nguyen, P. L., Vantaux, A., Hien, D. F. S., Dabiré, K. R., Yameogo, B. K., Gouagna, L. C., et al. (2017). No evidence for manipulation of Anopheles gambiae, An. coluzzii and An. arabiensis host preference by Plasmodium falciparum. Sci. Rep. 7, 1–11. doi: 10.1038/s41598-017-09821-x
Nogueira, N. F. S., Gonzalez, M. S., Gomes, J. E., de Souza, W., Garcia, E. S., Azambuja, P., et al. (2007). Trypanosoma cruzi: Involvement of glycoinositolphospholipids in the attachment to the luminal midgut surface of Rhodnius prolixus. Exp. Parasitol. 116, 120–128. doi: 10.1016/j.exppara.2006.12.014
Nogueira, N. P., Saraiva, F. M. S., Sultano, P. E., Cunha, P. R. B. B., Laranja, G. A. T., Justo, G. A., et al. (2015). Proliferation and differentiation of Trypanosoma cruzi inside its vector have a new trigger: Redox status. PLoS One 10:e0116712. doi: 10.1371/journal.pone.0116712
Noriega, F. G. (2004). Nutritional regulation of JH synthesis: A mechanism to control reproductive maturation in mosquitoes? Insect Biochem. Mol. Biol. 34, 687–693. doi: 10.1016/j.ibmb.2004.03.021
Nouzova, M., Clifton, M. E., and Noriega, F. G. (2019). Mosquito adaptations to hematophagia impact pathogen transmission. Curr. Opin. Insect Sci. 34, 21–26. doi: 10.1016/j.cois.2019.02.002
Nunes, C., and Sucena, É, and Koyama, T. (2020). Endocrine regulation of immunity in insects. FEBS J. 2020:15581. doi: 10.1111/febs.15581
Nuss, A. B., Brown, M. R., Murty, U. S., and Gulia-Nuss, M. (2018). Insulin receptor knockdown blocks filarial parasite development and alters egg production in the southern house mosquito, Culex quinquefasciatus. PLoS Negl. Trop. Dis. 12:e0006413. doi: 10.1371/journal.pntd.0006413
Okuda, K., de Almeida, F., Mortara, R. A., Krieger, H., Marinotti, O., and Tania Bijovsky, A. (2007). Cell death and regeneration in the midgut of the mosquito, Culex quinquefasciatus. J. Insect Physiol. 53, 1307–1315. doi: 10.1016/j.jinsphys.2007.07.005
Oliveira, G. D. A., Lieberman, J., and Barillas-Mury, C. (2012). Epithelial nitration by a peroxidase/NOX5 system mediates mosquito antiplasmodial immunity. Science 335, 856–859. doi: 10.1126/science.1209678
Oliveira, J. H., Bahia, A. C., and Vale, P. F. (2020). How are arbovirus vectors able to tolerate infection? Dev. Comp. Immunol. 103:103514. doi: 10.1016/j.dci.2019.103514
Oliveira, J. H. M., Gonçalves, R. L. S., Lara, F. A., Dias, F. A., Gandara, A. C. P., Menna-Barreto, R. F. S., et al. (2011a). Blood meal-derived heme decreases ROS levels in the midgut of Aedes aegypti and allows proliferation of intestinal microbiota. PLoS Pathog. 7:e1001320. doi: 10.1371/journal.ppat.1001320
Oliveira, J. H. M., Gonçalves, R. L. S., Oliveira, G. A., Oliveira, P. L., Oliveira, M. F., and Barillas-Mury, C. (2011b). Energy metabolism affects susceptibility of Anopheles gambiae mosquitoes to Plasmodium infection. Insect Biochem. Mol. Biol. 41, 349–355. doi: 10.1016/j.ibmb.2011.02.001
Oliveira, M. F., Silva, J. R., Dansa-Petretski, M., De Souza, W., Braga, C. M. S., Masuda, H., et al. (2000). Haemozoin formation in the midgut of the blood-sucking insect Rhodnius prolixus. FEBS Lett. 477, 95–98. doi: 10.1016/S0014-5793(00)01786-5
Oliver, S. V., and Brooke, B. D. (2017). The effects of ingestion of hormonal host factors on the longevity and insecticide resistance phenotype of the major malaria vector Anopheles arabiensis (Diptera: Culicidae). PLoS One 12:e0180909. doi: 10.1371/journal.pone.0180909
Omer, F. M., Kurtzhals, J. A., and Riley, E. M. (2000). Maintaining the immunological balance in parasitic infections: a role for TGF-β? Parasitol. Today 16, 18–23. doi: 10.1016/S0169-4758(99)01562-8
O’neal, A. J., Butler, L. R., Rolandelli, A., Gilk, S. D., and Pedra, J. H. F. (2020). Lipid hijacking: A unifying theme in vector-borne diseases. eLife 9:e61675. doi: 10.7554/eLife.61675
Osuna-Ramos, J. F., Reyes-Ruiz, J. M., and Del Ángel, R. M. (2018). The role of host cholesterol during flavivirus infection. Front. Cell. Infect. Microbiol. 8:388. doi: 10.3389/fcimb.2018.00388
Padrón, A., Molina-Cruz, A., Quinones, M., Ribeiro, J. M., Ramphul, U., Rodrigues, J., et al. (2014). In depth annotation of the Anopheles gambiae mosquito midgut transcriptome. BMC Genomics 15:636. doi: 10.1186/1471-2164-15-636
Pakpour, N., Akman-Anderson, L., Vodovotz, Y., and Luckhart, S. (2013a). The effects of ingested mammalian blood factors on vector arthropod immunity and physiology. Microbes Infect. 15, 243–254. doi: 10.1016/j.micinf.2013.01.003
Pakpour, N., Camp, L., Smithers, H. M., Wang, B., Tu, Z., Nadler, S. A., et al. (2013b). Protein kinase C-dependent signaling controls the midgut epithelial barrier to malaria parasite infection in anopheline mosquitoes. PLoS One 8:e76535. doi: 10.1371/journal.pone.0076535
Pakpour, N., Corby-Harris, V., Green, G. P., Smithers, H. M., Cheung, K. W., Riehle, M. A., et al. (2012). Ingested human insulin inhibits the mosquito NF-κB-dependent immune response to Plasmodium falciparum. Infect. Immun. 80, 2141–2149. doi: 10.1128/IAI.00024-12
Pakpour, N., Riehle, M. A., and Luckhart, S. (2014). Effects of ingested vertebrate-derived factors on insect immune responses. Curr. Opin. Insect Sci. 3, 1–5. doi: 10.1016/j.cois.2014.07.001
Park, J., Stanley, D., and Kim, Y. (2014). Roles of peroxinectin in PGE2-mediated cellular immunity in Spodoptera exigua. PLoS One 9:e105717. doi: 10.1371/journal.pone.0105717
Pascoa, V., Oliveira, P. L., Dansa-Petretski, M., Silva, J. R., Alvarenga, P. H., Jacobs-Lorena, M., et al. (2002). Aedes aegypti peritrophic matrix and its interaction with heme during blood digestion. Insect Biochem. Mol. Biol. 32, 517–523. doi: 10.1016/S0965-1748(01)00130-8
Pereira, M. G., Visbal, G., Salgado, L. T., Vidal, J. C., Godinho, J. L. P., De Cicco, N. N. T., et al. (2015). Trypanosoma cruzi epimastigotes are able to manage internal cholesterol levels under nutritional lipid stress conditions. PLoS One 10:e0128949. doi: 10.1371/journal.pone.0128949
Pereira-Chioccola, V. L., Acosta-Serrano, A., Correia de Almeida, I., Ferguson, M. A. J., Souto-Padron, T., Rodrigues, M. M., et al. (2000). Mucin-like molecules form a negatively charged coat that protects Trypanosoma cruzi trypomastigotes from killing by human anti-alpha-galactosyl antibodies. J. Cell Sci. 113(Pt 7), 1299–1307.
Perera, R., Riley, C., Isaac, G., Hopf-Jannasch, A. S., Moore, R. J., Weitz, K. W., et al. (2012). Dengue virus infection perturbs lipid homeostasis in infected mosquito cells. PLoS Pathog. 8:e1002584. doi: 10.1371/journal.ppat.1002584
Petersen, W., Stenzel, W., Silvie, O., Blanz, J., Saftig, P., Matuschewski, K., et al. (2017). Sequestration of cholesterol within the host late endocytic pathway restricts liver-stage Plasmodium development. Mol. Biol. Cell 28, 726–735. doi: 10.1091/mbc.E16-07-0531
Peterson, T. M. L., Gow, A. J., and Luckhart, S. (2007). Nitric oxide metabolites induced in Anopheles stephensi control malaria parasite infection. Free Radic. Biol. Med. 42, 132–142. doi: 10.1016/j.freeradbiomed.2006.10.037
Peterson, T. M. L., and Luckhart, S. (2006). A mosquito 2-Cys peroxiredoxin protects against nitrosative and oxidative stresses associated with malaria parasite infection. Free Radic. Biol. Med. 40, 1067–1082. doi: 10.1016/j.freeradbiomed.2005.10.059
Pietri, J. E., Pakpour, N., Napoli, E., Song, G., Pietri, E., Potts, R., et al. (2016). Two insulin-like peptides differentially regulate malaria parasite infection in the mosquito through effects on intermediary metabolism. Biochem. J. 473, 3487–3503. doi: 10.1042/BCJ20160271
Pietri, J. E., Pietri, E. J., Potts, R., Riehle, M. A., and Luckhart, S. (2015). Plasmodium falciparum suppresses the host immune response by inducing the synthesis of insulin-like peptides (ILPs) in the mosquito Anopheles stephensi. Dev. Comp. Immunol. 53, 134–144. doi: 10.1016/j.dci.2015.06.012
Pigeault, R., and Villa, M. (2018). Long-term pathogenic response to Plasmodium relictum infection in culex pipiens mosquito. PLoS One 13:e0192315. doi: 10.1371/journal.pone.0192315
Pimenta, P. F. P., Modi, G. B., Pereira, S. T., Shahabuddin, M., and Sacks, D. L. (1997). A novel role for the peritrophic matrix in protecting Leishmania from the hydrolytic activities of the sand fly midgut. Parasitology 115, 359–369. doi: 10.1017/S0031182097001510
Ponnudurai, T., Lensen, A. H. W., Van Gemert, G. J. A., Bensink, M. P. E., Bolmer, M., and Meuwissen, J. H. E. T. (1989). Sporozoite load of mosquitoes infected with Plasmodium falciparum. Trans. R. Soc. Trop. Med. Hyg. 83, 67–70. doi: 10.1016/0035-9203(89)90708-6
Powell, J. R. (2019). An evolutionary perspective on vector-borne diseases. Front. Genet. 10:1266. doi: 10.3389/fgene.2019.01266
Ramalho-Ortigao, M. (2010). Sand Fly-leishmania interactions: long relationships are not necessarily easy. Open Parasitol. J. 4, 195–204. doi: 10.2174/1874421401004010195
Ramirez, J. L., Souza-Neto, J., Torres Cosme, R., Rovira, J., Ortiz, A., Pascale, J. M., et al. (2012). Reciprocal tripartite interactions between the Aedes aegypti midgut microbiota, innate immune system and dengue virus influences vector competence. PLoS Negl. Trop. Dis. 6:e1561. doi: 10.1371/journal.pntd.0001561
Reiff, T., Jacobson, J., Cognigni, P., Antonello, Z., Ballesta, E., Tan, K. J., et al. (2015). Endocrine remodelling of the adult intestine sustains reproduction in Drosophila. eLife 4:e06930. doi: 10.7554/eLife.06930
Reth, M. (2002). Hydrogen peroxide as second messenger in lymphocyte activation. Nat. Immunol. 3, 1129–1134. doi: 10.1038/ni1202-1129
Reynolds, R. A., Kwon, H., and Smith, R. C. (2020). 20-hydroxyecdysone primes innate immune responses that limit bacterial and malarial parasite survival in Anopheles gambiae. mSphere 5:e00983-19. doi: 10.1128/mSphere.00983-19
Rodgers, F. H., Gendrin, M., Wyer, C. A. S., Christophides, G. K., Vilo, J., Yarza, P., et al. (2017). Microbiota-induced peritrophic matrix regulates midgut homeostasis and prevents systemic infection of malaria vector mosquitoes. PLoS Pathog. 13:e1006391. doi: 10.1371/journal.ppat.1006391
Romoli, O., and Gendrin, M. (2018). The tripartite interactions between the mosquito, its microbiota and Plasmodium. Parasit. Vectors 11:200. doi: 10.1186/s13071-018-2784-x
Rono, M. K., Whitten, M. M. A., Oulad-Abdelghani, M., Levashina, E. A., and Marois, E. (2010). The major yolk protein vitellogenin interferes with the anti-Plasmodium response in the malaria mosquito Anopheles gambiae. PLoS Biol. 8:e1000434. doi: 10.1371/journal.pbio.1000434
Rose, C., Casas-Sánchez, A., Dyer, N. A., Solórzano, C., Beckett, A. J., Middlehurst, B., et al. (2020). Trypanosoma brucei colonizes the tsetse gut via an immature peritrophic matrix in the proventriculus. Nat. Microbiol. 5, 909–916. doi: 10.1038/s41564-020-0707-z
Roy, S. G., and Raikhel, A. S. (2012). Nutritional and hormonal regulation of the TOR effector 4E-binding protein (4E-BP) in the mosquito Aedes aegypti. FASEB J. 26, 1334–1342. doi: 10.1096/fj.11-189969
Roy, S., Saha, T. T., Johnson, L., Zhao, B., Ha, J., White, K. P., et al. (2015). Regulation of gene expression patterns in mosquito reproduction. PLoS Genet. 11:1005450. doi: 10.1371/journal.pgen.1005450
Roy, S., Saha, T. T., Zou, Z., and Raikhel, A. S. (2018). Regulatory pathways controlling female insect reproduction. Annu. Rev. Entomol. 63, 489–511. doi: 10.1146/annurev-ento-020117-043258
Rus, F., Flatt, T., Tong, M., Aggarwal, K., Okuda, K., Kleino, A., et al. (2013). Ecdysone triggered PGRP-LC expression controls Drosophila innate immunity. EMBO J. 32, 1626–1638. doi: 10.1038/emboj.2013.100
Salcedo-Porras, N., and Lowenberger, C. (2019). The innate immune system of kissing bugs, vectors of chagas disease. Dev. Comp. Immunol. 98, 119–128. doi: 10.1016/j.dci.2019.04.007
Samaddar, S., Marnin, L., Butler, L. R., and Pedra, J. H. F. (2020). Immunometabolism in arthropod vectors: redefining interspecies relationships. Trends Parasitol. 36, 807–815. doi: 10.1016/j.pt.2020.07.010
Samsa, M. M., Mondotte, J. A., Iglesias, N. G., Assunção-Miranda, I., Barbosa-Lima, G., Da Poian, A. T., et al. (2009). Dengue virus capsid protein usurps lipid droplets for viral particle formation. PLoS Pathog. 5:e1000632. doi: 10.1371/journal.ppat.1000632
Sant’Anna, M. R., Diaz-Albiter, H., Aguiar-Martins, K., Al Salem, W. S., Cavalcante, R. R., Dillon, V. M., et al. (2014). Colonisation resistance in the sand fly gut: Leishmania protects Lutzomyia longipalpis from bacterial infection. Parasit. Vectors 7:329. doi: 10.1186/1756-3305-7-329
Sant’Anna, M. R., Diaz-Albiter, H., Mubaraki, M., Dillon, R. J., and Bates, P. A. (2009). Inhibition of trypsin expression in Lutzomyia longipalpis using RNAi enhances the survival of Leishmania. Parasit. Vectors 2, 1–10. doi: 10.1186/1756-3305-2-62
Santiago, P. B., de Araújo, C. N., Motta, F. N., Praça, Y. R., Charneau, S., Bastos, I. M. D., et al. (2017). Proteases of haematophagous arthropod vectors are involved in blood-feeding, yolk formation and immunity - a review. Parasit. Vectors 10:79. doi: 10.1186/s13071-017-2005-z
Santos-Araujo, S., Bomfim, L., Araripe, L. O., Bruno, R., Ramos, I., and Gondim, K. C. (2020). Silencing of ATG6 and ATG8 promotes increased levels of triacylglycerol (TAG) in the fat body during prolonged starvation periods in the Chagas disease vector Rhodnius prolixus. Insect Biochem. Mol. Biol. 127:103484. doi: 10.1016/j.ibmb.2020.103484
Saraiva, R. G., Kang, S., Simões, M. L., Angleró-Rodríguez, Y. I., and Dimopoulos, G. (2016). Mosquito gut antiparasitic and antiviral immunity. Dev. Comp. Immunol. 64, 53–64. doi: 10.1016/j.dci.2016.01.015
Schulze, R. J., Sathyanarayan, A., and Mashek, D. G. (2017). Breaking fat: the regulation and mechanisms of lipophagy. Biochim. Biophys. Acta Mol. Cell Biol. Lipids 1862, 1178–1187. doi: 10.1016/j.bbalip.2017.06.008
Schwartz, A., and Koella, J. C. (2001). Trade-offs, conflicts of interest and manipulation in Plasmodium-mosquito interactions. Trends Parasitol. 17, 189–194. doi: 10.1016/S1471-4922(00)01945-0
Schwenke, R. A., Lazzaro, B. P., and Wolfner, M. F. (2016). Reproduction–immunity trade-offs in insects. Annu. Rev. Entomol. 61, 239–256. doi: 10.1146/annurev-ento-010715-023924
Scott, T. W., Clark, G. G., Lorenz, L. H., Amerasinghe, P. H., Reiter, P., and Edman, J. D. (1993). Detection of multiple blood-feeding in Aedes aegypti (Diptera: Culicidae) during a single gonotrophic cycle using a histologic technique. J. Med. Entomol. 30, 94–99. doi: 10.1093/jmedent/30.1.94
Secundino, N. F. C., Eger-Mangrich, I., Braga, E. M., Santoro, M. M., and Pimenta, P. F. P. (2005). Lutzomyia longipalpis peritrophic matrix: Formation, structure, and chemical composition. J. Med. Entomol. 42, 928–938. doi: 10.1093/jmedent/42.6.928
Serafim, T. D., Coutinho-Abreu, I. V., Oliveira, F., Meneses, C., Kamhawi, S., and Valenzuela, J. G. (2018). Sequential blood meals promote Leishmania replication and reverse metacyclogenesis augmenting vector infectivity. Nat. Microbiol. 3, 548–555. doi: 10.1038/s41564-018-0125-7
Shahabuddin, M., Kaidoh, T., Aikawa, M., and Kaslow, D. C. (1995). Plasmodium gallinaceum: mosquito peritrophic matrix and the parasite-vector compatibility. Exp. Parasitol. 81, 386–393. doi: 10.1006/expr.1995.1129
Shahabuddin, M., Toyoshima, T., Aikawa, M., and Kaslow, D. C. (1993). Transmission-blocking activity of a chitinase inhibitor and activation of malarial parasite chitinase by mosquito protease. Proc. Natl. Acad. Sci. U.S.A. 90, 4266–4270. doi: 10.1073/pnas.90.9.4266
Shao, L., Devenport, M., and Jacobs-lorena, M. (2001). The peritrophic matrix of hematophagous insects. Arch. Insect Biochem. Physiol. 47, 119–125. doi: 10.1002/arch.1042
Sharma, A., Nuss, A. B., and Gulia-Nuss, M. (2019). Insulin-like peptide signaling in mosquitoes: the road behind and the road ahead. Front. Endocrinol. 10:166. doi: 10.3389/fendo.2019.00166
Shaw, D. K., Tate, A. T., Schneider, D. S., Levashina, E. A., Kagan, J. C., Pal, U., et al. (2018). Vector immunity and evolutionary ecology: the harmonious dissonance. Trends Immunol. 39, 862–873. doi: 10.1016/j.it.2018.09.003
Shaw, W. R., and Catteruccia, F. (2019). Vector biology meets disease control: using basic research to fight vector-borne diseases. Nat. Microbiol. 4, 20–34. doi: 10.1038/s41564-018-0214-7
Shaw, W. R., Holmdahl, I. E., Itoe, M. A., Werling, K., Marquette, M., Paton, D. G., et al. (2020). Multiple blood feeding in mosquitoes shortens the Plasmodium falciparum incubation period and increases malaria transmission potential. PLoS Pathog. 16:e1009131. doi: 10.1371/journal.ppat.1009131
Short, S. M., Mongodin, E. F., MacLeod, H. J., Talyuli, O. A. C., and Dimopoulos, G. (2017). Amino acid metabolic signaling influences Aedes aegypti midgut microbiome variability. PLoS Negl. Trop. Dis. 11:e0005677. doi: 10.1371/journal.pntd.0005677
Sicard, J. F., Le Bihan, G., Vogeleer, P., Jacques, M., and Harel, J. (2017). Interactions of intestinal bacteria with components of the intestinal mucus. Front. Cell. Infect. Microbiol. 7:387. doi: 10.3389/fcimb.2017.00387
Sisterson, M. S. (2009). Transmission of insect-vectored pathogens: effects of vector fitness as a function of infectivity status. Environ. Entomol. 38, 345–355. doi: 10.1603/022.038.0206
Sojka, D., Franta, Z., Horn, M., Hajdušek, O., Caffrey, C. R., Mareš, M., et al. (2008). Profiling of proteolytic enzymes in the gut of the tick Ixodes ricinus reveals an evolutionarily conserved network of aspartic and cysteine peptidases. Parasit. Vectors 1:7. doi: 10.1186/1756-3305-1-7
Stanczyk, N. M., Brugman, V. A., Austin, V., Sanchez-Roman Teran, F., Gezan, S. A., Emery, M., et al. (2019). Species-specific alterations in Anopheles mosquito olfactory responses caused by Plasmodium infection. Sci. Rep. 9, 1–9. doi: 10.1038/s41598-019-40074-y
Sterkel, M., Oliveira, J. H. M., Bottino-Rojas, V., Paiva-Silva, G. O., and Oliveira, P. L. (2017). The dose makes the poison: nutritional overload determines the life traits of blood-feeding arthropods. Trends Parasitol. 20, 1–12. doi: 10.1016/j.pt.2017.04.008
Stiebler, R., Majerowicz, D., Knudsen, J., Gondim, K. C., Wright, D. W., Egan, T. J., et al. (2014). Unsaturated glycerophospholipids mediate heme crystallization: Biological implications for hemozoin formation in the kissing bug Rhodnius prolixus. PLoS One 9:e0088976. doi: 10.1371/journal.pone.0088976
Stierhof, Y. D., Bates, P. A., Jacobson, R. L., Rogers, M. E., Schlein, Y., Handman, E., et al. (1999). Filamentous proteophosphoglycan secreted by Leishmania promastigotes forms gel like three-dimensional networks that obstruct the digestive tract of infected sandfly vectors. Eur. J. Cell Biol. 78, 675–689. doi: 10.1016/S0171-9335(99)80036-3
Surachetpong, W., Pakpour, N., Cheung, K. W., and Luckhart, S. (2011). Reactive oxygen species-dependent cell signaling regulates the mosquito immune response to Plasmodium falciparum. Antioxid. Redox Signal. 14, 943–955. doi: 10.1089/ars.2010.3401
Surachetpong, W., Singh, N., Cheung, K. W., and Luckhart, S. (2009). MAPK ERK signaling regulates the TGF-β1-dependent mosquito response to Plasmodium falciparum. PLoS Pathog 5:e1000366. doi: 10.1371/journal.ppat.1000366
Tall, A. R., and Yvan-Charvet, L. (2015). Cholesterol, inflammation and innate immunity. Nat. Rev. Immunol. 15, 104–116. doi: 10.1038/nri3793
Taracena, M. L., Bottino-Rojas, V., Talyuli, O. A. C., Walter-Nuno, A. B., Oliveira, J. H. M., Angleró-Rodriguez, Y. I., et al. (2018). Regulation of midgut cell proliferation impacts Aedes aegypti susceptibility to dengue virus. PLoS Negl. Trop. Dis. 12:e0006498. doi: 10.1371/journal.pntd.0006498
Tedrow, R. E., Zimmerman, P. A., and Abbott, K. C. (2019). Multiple blood-feeding: a force multiplier for transmission. Trends Parasitol. 35, 949–952. doi: 10.1016/j.pt.2019.08.004
Telleria, E. L., Martins-Da-Silva, A., Tempone, A. J., and Traub-Cseko, Y. M. (2018). Leishmania, microbiota and sand fly immunity. Parasitology 145, 1336–1353. doi: 10.1017/S0031182018001014
Terra, W. R. (1988). Physiology and biochemistry of insect digestion: an evolutionary perspective. Brazilian J. Med. Biol. Res. 21, 675–734.
Terra, W. R. (2001). The origin and functions of the insect peritrophic membrane and peritrophic gel. Arch. Insect Biochem. Physiol. 47, 47–61. doi: 10.1002/arch.1036
Terra, W. R., Dias, R. O., Oliveira, P. L., Ferreira, C., and Venancio, T. M. (2018). Transcriptomic analyses uncover emerging roles of mucins, lysosome/secretory addressing and detoxification pathways in insect midguts. Curr. Opin. Insect Sci. 29, 34–40. doi: 10.1016/j.cois.2018.05.015
Thaker, S. K., Chapa, T., Garcia, G., Gong, D., Schmid, E. W., Arumugaswami, V., et al. (2019). Differential metabolic reprogramming by zika virus promotes cell death in human versus mosquito cells. Cell Metab. 29, 1206.e4–1216.e4. doi: 10.1016/j.cmet.2019.01.024
Tootle, T. L., Williams, D., Hubb, A., Frederick, R., and Spradling, A. (2011). Drosophila eggshell production: identification of new genes and coordination by Pxt. PLoS One 6:e19943. doi: 10.1371/journal.pone.0019943
Urbanski, A., and Rosinski, G. (2018). Role of neuropeptides in the regulation of the insect immune system – current knowledge and perspectives. Curr. Protein Pept. Sci. 19, 1201–1213. doi: 10.2174/1389203719666180809113706
Vallochi, A. L., Teixeira, L., Oliveira, K., da, S., Maya-Monteiro, C. M., and Bozza, P. T. (2018). Lipid droplet, a key player in host-parasite interactions. Front. Immunol. 9:1022. doi: 10.3389/fimmu.2018.01022
van den Abbeele, J., Caljon, G., de Ridder, K., de Baetselier, P., and Coosemans, M. (2010). Trypanosoma brucei modifies the tsetse salivary composition, altering the fly feeding behavior that favors parasite transmission. PLoS Pathog. 6:e1000926. doi: 10.1371/journal.ppat.1000926
Varvas, K., Kurg, R., Hansen, K., Järving, R., Järving, I., Valmsen, K., et al. (2009). Direct evidence of the cyclooxygenase pathway of prostaglandin synthesis in arthropods: Genetic and biochemical characterization of two crustacean cyclooxygenases. Insect Biochem. Mol. Biol. 39, 851–860. doi: 10.1016/j.ibmb.2009.10.002
Veal, E. A., Day, A. M., and Morgan, B. A. (2007). Hydrogen peroxide sensing and signaling. Mol. Cell 26, 1–14. doi: 10.1016/j.molcel.2007.03.016
Vial, T., Tan, W. L., Xiang, B. W. W., Missé, D., Deharo, E., Marti, G., et al. (2019). Dengue virus reduces AGPAT1 expression to alter phospholipids and enhance infection in Aedes aegypti. PLoS Pathog. 15:e1008199. doi: 10.1371/journal.ppat.1008199
Vieira, C. S., Figueiredo, M. B., Moraes, C., da, S., Pereira, S. B., Dyson, P., et al. (2021). Azadirachtin interferes with basal immunity and microbial homeostasis in the Rhodnius prolixus midgut. Dev. Comp. Immunol. 114:103864. doi: 10.1016/j.dci.2020.103864
Villalon, J. M., Ghosh, A., and Jacobs-Lorena, M. (2003). The peritrophic matrix limits the rate of digestion in adult Anopheles stephensi and Aedes aegypti mosquitoes. J. Insect Physiol. 49, 891–895. doi: 10.1016/S0022-1910(03)00135-5
Villarreal, S. M., Pitcher, S., Helinski, M. E. H., Johnson, L., Wolfner, M. F., and Harrington, L. C. (2018). Male contributions during mating increase female survival in the disease vector mosquito Aedes aegypti. J. Insect Physiol. 108, 1–9. doi: 10.1016/j.jinsphys.2018.05.001
Vivenes, A., Oviedo, M., Márquez, J. C., and Montoya-Lerma, J. (2001). Effect of a Second Bloodmeal on the Oesophagus Colonization by Leishmania mexicana Complex in Lutzomyia evansi (Diptera: Psychodidae). Mem. Inst. Oswaldo Cruz 96, 281–283. doi: 10.1590/S0074-02762001000300001
Wang, X., Hou, Y., Saha, T. T., Pei, G., Raikhel, A. S., and Zou, Z. (2017). Hormone and receptor interplay in the regulation of mosquito lipid metabolism. Proc. Natl. Acad. Sci. U.S.A. 114, E2709–E2718. doi: 10.1073/pnas.1619326114
Weiss, B. L., Savage, A. F., Griffith, B. C., Wu, Y., and Aksoy, S. (2014). The peritrophic matrix mediates differential infection outcomes in the tsetse fly gut following challenge with commensal, pathogenic, and parasitic microbes. J. Immunol. 193, 773–782. doi: 10.4049/jimmunol.1400163
Werling, K., Shaw, W. R., Itoe, M. A., Westervelt, K. A., Marcenac, P., Paton, D. G., et al. (2019). Steroid hormone function controls non-competitive Plasmodium development in Anopheles. Cell 177, 315.e14–325.e14. doi: 10.1016/j.cell.2019.02.036
Whiten, S. R., Keith Ray, W., Helm, R. F., and Adelman, Z. N. (2018). Characterization of the adult Aedes aegypti early midgut peritrophic matrix proteome using LC-MS. PLoS One 13:e0194734. doi: 10.1371/journal.pone.0194734
Whitten, M., Sun, F., Tew, I., Schaub, G., Soukou, C., Nappi, A., et al. (2007). Differential modulation of Rhodnius prolixus nitric oxide activities following challenge with Trypanosoma rangeli, T. cruzi and bacterial cell wall components. Insect Biochem. Mol. Biol. 37, 440–452. doi: 10.1016/j.ibmb.2007.02.001
Woolhouse, M. E. J., Dye, C., Etard, J.-F., Smith, T., Charlwood, J. D., Garnett, G. P., et al. (1997). Heterogeneities in the transmission of infectious agents: Implications for the design of control programs. Proc. Natl. Acad. Sci. U.S.A. 94, 338–342. doi: 10.1073/pnas.94.1.338
Wu, K., Li, S., Wang, J., Ni, Y., Huang, W., Liu, Q., et al. (2020). Peptide hormones in the insect midgut. Front. Physiol. 11:191. doi: 10.3389/fphys.2020.00191
Xiao, X., Yang, L., Pang, X., Zhang, R., Zhu, Y., Wang, P., et al. (2017). A Mesh-Duox pathway regulates homeostasis in the insect gut. Nat. Microbiol. 2:17020. doi: 10.1038/nmicrobiol.2017.20
Zande, D. (1967). Absence of cholesterol synthesis as contrasted with the presence of fatty acid synthesis in some arthropods. Comp. Biochem. Physiol. 20, 811–822. doi: 10.1016/0010-406X(67)90055-2
Zhang, G., Niu, G., Franca, C. M., Dong, Y., Wang, X., Butler, N. S., et al. (2015). Anopheles midgut FREP1 mediates Plasmodium invasion. J. Biol. Chem. 290, 16490–16501. doi: 10.1074/jbc.M114.623165
Zhu, Y., Tong, L., Nie, K., Wiwatanaratanabutr, I., Sun, P., Li, Q., et al. (2019). Host serum iron modulates dengue virus acquisition by mosquitoes. Nat. Microbiol. 4, 2405–2415. doi: 10.1038/s41564-019-0555-x
Zingales, B., Martin, N. F., De Lederkremer, R. M., and Colli, W. (1982). Endogenous and surface labeling of glycoconjugates from the three differentiation stages of Trypanosoma cruzi. FEBS Lett. 142, 238–242. doi: 10.1016/0014-5793(82)80143-9
Keywords: tolerance, vector competence, blood-feeding, immunity, pathogens, parasite–vector interaction, insect physiology, midgut homeostasis
Citation: Talyuli OAC, Bottino-Rojas V, Polycarpo CR, Oliveira PL and Paiva-Silva GO (2021) Non-immune Traits Triggered by Blood Intake Impact Vectorial Competence. Front. Physiol. 12:638033. doi: 10.3389/fphys.2021.638033
Received: 04 December 2020; Accepted: 08 February 2021;
Published: 02 March 2021.
Edited by:
Aram Megighian, University of Padua, ItalyReviewed by:
Nazzy Pakpour, California State University, East Bay, United StatesDaniele Pereira Castro, Oswaldo Cruz Foundation (Fiocruz), Brazil
Copyright © 2021 Talyuli, Bottino-Rojas, Polycarpo, Oliveira and Paiva-Silva. This is an open-access article distributed under the terms of the Creative Commons Attribution License (CC BY). The use, distribution or reproduction in other forums is permitted, provided the original author(s) and the copyright owner(s) are credited and that the original publication in this journal is cited, in accordance with accepted academic practice. No use, distribution or reproduction is permitted which does not comply with these terms.
*Correspondence: Gabriela O. Paiva-Silva, Z29zaWx2YUBiaW9xbWVkLnVmcmouYnI=
†These authors have contributed equally to this work
‡Present address: Vanessa Bottino-Rojas, Department of Microbiology and Molecular Genetics, University of California, Irvine, Irvine, CA, United States