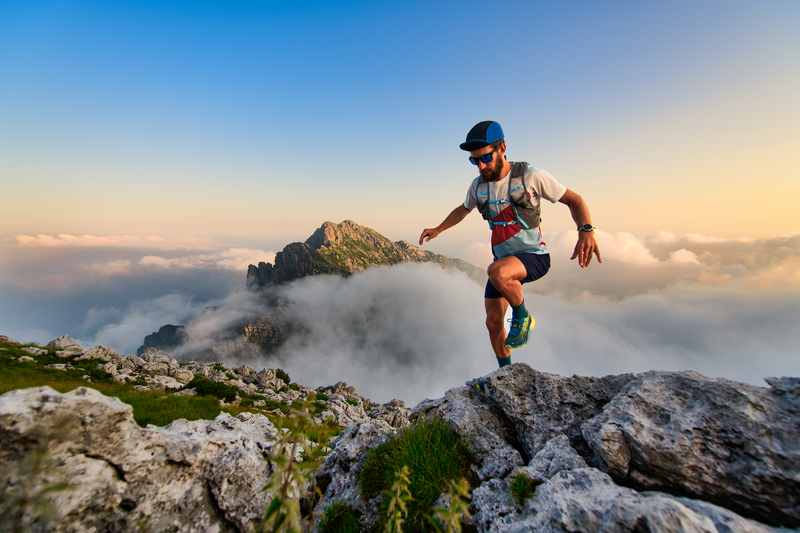
95% of researchers rate our articles as excellent or good
Learn more about the work of our research integrity team to safeguard the quality of each article we publish.
Find out more
ORIGINAL RESEARCH article
Front. Physiol. , 01 February 2021
Sec. Avian Physiology
Volume 12 - 2021 | https://doi.org/10.3389/fphys.2021.637629
A study was conducted to understand the effects of 25-hydroxyvitamin D3 (25OHD) and 1,25-dihydroxyvitamin D3 (1,25OHD) administration on the expression of key genes related to osteogenesis, adipogenesis, myogenesis, and vitamin D3 metabolism in the chicken embryo. A total of 120 fertilized Cobb 500 eggs were used in the current study and were reared under standard incubation conditions. On embryonic day 3 (ED 3), PBS (C), PBS with 40ng 1,25OHD (1,25D-L), 200ng 1,25OHD (1,25D-H), 40ng 25OHD (25D-L), or 200ng 25OHD (25D-H) were injected into the dorsal vein of developing embryos. Whole embryos were harvested at 1, 3, and 6h post-injection for gene expression analyses (n=8). Gene expression for key osteogenesis markers (RUNX2: runt-related transcription factor 2; BMP2: bone morphogenetic protein 2; COL1A2: collagen type I alpha 2 chain; BGLAP: bone gamma-carboxyglutamate protein; SPP1: secreted phosphoprotein 1; and ALP: alkaline phosphatese), adipogenesis markers (PPAR-γ: peroxisome proliferator-activated receptor gamma; FASN: fatty acid synthase; and FABP4: fatty acid binding protein 4), myogenesis markers (MYOG: myogenin; MYOD1: myogenic differentiation 1; and MYF5: myogenic factor 5), and the enzyme responsible for vitamin D3 inactivation (CYP24A1: cytochrome P450 family 24 subfamily A member 1) were measured using real-time quantitative reverse transcription polymerase chain reaction (qRT-PCR). Data were normalized by the ΔΔCT method and analyzed using a one-way ANOVA. Results indicated that at 1h post-injection, no differences were found among treatments. At 3h, the early osteogenesis differentiation marker, ALP, was increased by 1,25D-H and 25D-H, and 25D-H also stimulated the expression of adipogenesis markers (FAPB4 and FASN). In contrast, the expression of myogenesis markers (MYOD1 and MYF5) was suppressed by 25OHD or 1,25OHD treatments, respectively. At 6h, a late osteogenic differentiation marker, SPP1, was increased by 25D-H. MYOD1 and MYF5 were continuously suppressed by 25OHD treatments or 1,25D-H. The evidence of vitamin D3 metabolite retention was assessed by measuring CYP24A1 expression. At 1h, there were no differences in CYP24A1 expression. At 3h, all treatments upregulated CYP24A1 expression relative to control (PBS) embryos. However, at 6h, only the 25D-H group retained higher CYP24A1 expression compared to the other treatments. In conclusion, the results suggested both 1,25OHD and 25OHD induced chicken embryo osteogenesis and adipogenesis, but inhibited myogenesis during early chicken embryo development. The higher dosage of 25OHD showed a possibility of a longer retention time in the embryos.
Vitamin D3 is essential for normal chicken embryo development (Sunde et al., 1978). It is well-established that Vitamin D3 undergoes two biological conversions, first in the liver, to become 25-hydroxyvtamin D3 (25OHD) hydroxylated by 25-hydroxylase (CYP2R1), and then mainly, in the kidney to become its biologically active form, 1,25-dihydroxyvitamin D3 (1,25OHD), which is catalyzed by 1α-hydroxylase (CYP27B1; St-Arnaud, 2008; Christakos et al., 2010). Vitamin D3 is primarily stored in the egg yolk in the form of 25OHD to be used by the developing chicken embryo during development (Ovesen et al., 2003; Vieira, 2007; Fatemi et al., 2020). In the poultry industry, 25OHD has become a commercial feed additive for poultry production due to its higher bioactivity than regular vitamin D3 (Soares et al., 1995; Atencio et al., 2005a). Considerable research has demonstrated beneficial effects of 25OHD on chicken bone quality (Koreleski and Swiatkiewicz, 2005; Wideman et al., 2015; Chen et al., 2020) and muscle development (Michalczuk et al., 2010; Han et al., 2016).
In addition to utilizing vitamin D3 metabolites in poultry feed, the influence of exogenous 25OHD during embryo development has also been studied. The administration of 25OHD can be achieved by in ovo injection of 25OHD directly to the yolk of chicken embryos at embryonic day 18 (ED 18), which has shown to reduce late embryo mortality (Fatemi et al., 2020). A less invasive alternative for increasing embryo 25OHD level has been accomplished by providing 25OHD in parent breeder diets. Studies have shown that this practice could significantly improve hatchability and embryo livability (Atencio et al., 2005b; Saunders-Blades and Korver, 2014). However, limited research has been conducted to evaluate the role of vitamin D3 metabolites during early development of chicken embryos. Even though considerable cell culture studies have been performed to understand the vitamin D3 signaling pathway in osteogenesis, adipogenesis, and myogenesis (Ding et al., 2012; Girgis et al., 2013; van Driel and van Leeuwen, 2014; Dix et al., 2018), the mono-cell culture model fails to account for interactions with alternate cell types or presence of extracellular factors such as fibroblast growth factors, insulin, and growth factors (Rosen and MacDougald, 2006). Osteoblasts, adipocytes, and myoblasts are all differentiated from embryonic stem cells (Kolf et al., 2007). The factors in favor of one cell fate may be at the cost of others (Kolf et al., 2007). As such, it is critical to study the role of vitamin D3 in embryogenesis, while all different types of cells are present simultaneously.
Thus, the current study was conducted to understand the role of vitamin D3 metabolites on the expression of key osteogenic, adipogenic, myogenic, and vitamin D3 metabolism genes in the chicken embryos. Treatments were injected through the dorsal vein of chicken embryo at ED 3. Embryos were then sampled to examine expression of various genes related to osteogenesis, adipogenesis, and myogenesis. The current study provided an overview of how vitamin D3 metabolites affect embryogenesis and the metabolism of vitamin D3 in the embryo during early developmental stages. The results could also provide insight into the manipulation of nutrients during chicken embryo development and contribute to designing a strategy targeting optimized embryo nutrition.
The study was approved by the Institutional Animal Care and Use Committee at the University of Georgia and conducted at the research facility of the Department of Poultry Science at the University of Georgia. Cobb 500 fertilized eggs were purchased from Cobb Hatchery (Cleveland, GA, United States). A total of 120 Cobb 500 fertilized eggs (8 eggs/treatment×5 treatments×3 time points) were used in the current study. They were incubated inside a bench incubator (GQF 1502, Savannah, GA, United States) at 37.5°C and around 45% relative humidity. The eggs were positioned horizontally without turning to ensure that the position of embryos was appropriate and consistent for injection. At 72h of incubation, fertilized eggs were sprayed with 70% alcohol, and 1.5ml albumen was carefully taken out from the sharp end of the eggs. The needle hole was sealed with glue immediately to avoid contamination. A mini drill was used for opening a window (around 1cm2) on the side of the eggs. Hundred microliter of Penicillin-Streptomycin (10,000U/ml; Thermo Fisher Scientific, MA, United States) was applied to the eggs. Then the eggs were placed under a microscope (Olympus, PA, United States). PBS (C), PBS with 40ng 1,25-dihydroxyvitamin D3 (1,25D-L), 200ng 1,25-dihydroxyvitamin D3 (1,25D-H), 40ng 25-hydroxyvitamin D3 (25D-L), or 200ng 25-hydroxyvitamin D3 (25D-H; MilliporeSigma, MO, United States) were injected into the dorsal vein of embryos (Figure 1). Afterward, glass coverslips were used to seal the windows, and injected eggs were placed back to the incubator. The injected time was recorded for individual eggs. Whole embryos were harvested at 1, 3, and 6h post-injection for gene expression analyses (eight embryos/treatment/time point). The embryos were collected in 1ml RNAlater (Thermo Fisher Scientific, MA, United States) and stored at −80C until analysis.
Figure 1. Diagram showing the procedure of in-ovo injection at ED 3 and the injection location (white arrow).
Total RNA was extracted from whole embryos by using QIAzol Lysis reagents (Qiagen, MD, United States) according to the manufacturer’s protocol. RNA quantity and purity were determined using a Nanodrop 1000 spectrophotometer (Thermo Fisher Scientific, Pittsburgh, PA, United States). For each sample, 2μg of RNA was reverse-transcribed to cDNA using a High-Capacity cDNA Reverse Transcription Kit (Thermo Fisher Scientific, MA, United States) following the manufacturer’s protocol in a 96-well thermal cycler (Thermo Fisher Scientific, MA, United States). cDNA templates were diluted 10-fold prior to analysis. The samples were analyzed in duplicate by real-time quantitative reverse transcription polymerase chain reaction (qRT-PCR) performed on an Applied Biosystems StepOnePlus™ (Thermo Fisher Scientific, Waltham, MA, United States) with iTaq™ Universal SYBR Green Supermix (BioRad, Hercules, CA, United States) using the following conditions for all genes: 95°C for 10min followed by 40cycles of 15s denaturation at 95°C, annealing for 20s, and 15s extension at 72°C, followed by 95°C for 15s and a melt curve stage. The key osteogenesis marker genes (RUNX2: runt-related transcription factor 2; BMP2: bone morphogenetic protein 2; COL1A2: collagen type I alpha 2 chain; BGLAP: bone gamma-carboxyglutamate protein; SPP1: secreted phosphoprotein 1; and ALP: alkaline phosphatese; Chatakun et al., 2014; Adhikari et al., 2019); key adipogenesis marker genes (PPAR-γ: peroxisome proliferator-activated receptor gamma; FASN: fatty acid synthase; and FAPB4: fatty acid binding protein 4; Bhat et al., 2014; Ji et al., 2015); key myogenesis marker genes (MYOG: myogenin; MYOD1: myogenic differentiation 1, and MYF5: myogenic factor 5; Wagatsuma and Sakuma, 2014); and a vitamin D catabolism gene (CYP24A1: cytochrome P450 family 24 subfamily A member 1; Christakos et al., 2010) were investigated (Table 1). GAPDH (glyceraldehyde-3-phosphate dehydrogenase) was used as a housekeeping gene. Samples were normalized and analyzed by the ΔΔCT method (Livak and Schmittgen, 2001).
All experimental data were analyzed statistically by one-way ANOVA using SAS software Version 9.3 (SAS Institute, Cary, NC). Variability in the data was expressed as standard error mean (SEM). Differences between means were determined using Duncan’s Multiple Range test. The level of significance was assessed at p≤0.05.
The sequence for CYP27B1 (encoding 1α-hydroxylase) has not yet been identified in chickens (NCBI). 1α-hydroxylase is the critical enzyme that catalyzes 25OHD to 1,25OHD (Christakos et al., 2010). Thus, its expression to indicate the conversion of 25OHD to 1,25OHD could not be examined in the current study. Additionally, the level of 1,25OHD is difficult to measure in the embryo, because the sampling of embryo could not avoid breaking blood vessels, which could convolute analysis. However, the expression of CYP24A1 encoding 24-hydroxylase that is responsible for 1,25OHD inactivation to 1,25,24 OHD (van Driel and van Leeuwen, 2014) could be stimulated by 1,25OHD (Veldurthy et al., 2016). Thus, by investigating CYP24A1 expression level, we could observe the 1,25OHD residues status in the embryos.
Since no differences were observed in BMP2, COL1A2, and BGLAP expression, these data were not shown in this manuscript. At 1h post-injection, there was no difference in the vitamin D3 metabolism marker (CYP24A1) expression (Figure 2A). Meanwhile, no significant differences were observed on osteogenesis (Figures 3A–C), adipogenesis (Figures 4A–C), and myogenesis marker gene expression (Figures 5A–C).
Figure 2. Effect of 25OHD and 1,25OHD on CYP24A1 expression at 1h (A), 3h (B), and 6h (C) post injection. Mean separation was indicated by different letters on the top of bars (value means±SEM, n=8). CYP24A1: cytochrome P450 family 24 subfamily A member 1.
Figure 3. Effect of 25OHD and 1,25OHD on key osteogenesis marker gene expressions. (A–I) showed the expression of RUNX2, ALP and SPP1 at 1h, 3h, and 6h post-injection, respectively. Mean separation was indicated by different letters on the top of bars (value means±SEM, n=8). RUNX2: runt related transcription factor 2; ALP: alkaline phosphatase; SPP1: secreted phosphoprotein 1.
Figure 4. Effect of 25OHD and 1,25OHD on key adipogenesis marker gene expressions. (A–I) showed the expression of FABP4, FASN and PPAR-γ at 1h, 3h, and 6h post-injection, respectively. Mean separation was indicated by different letters on the top of bars (value means±SEM, n=8). FASN: fatty acid synthase; FABP4: fatty acid binding protein 4; PPAR-γ: peroxisome proliferator-activated receptor gamma.
Figure 5. Effect of 25OHD and 1,25OHD on key myogenesis marker gene expressions. (A–I) showed the expression of MYOD1, MYF5 and MYOG at 1h, 3h, and 6h post-injection, respectively. Mean separation was indicated by different letters on the top of bars (value means±SEM, n=8). MYOD1: myogenic differentiation 1; MYF5: myogenic factor 5; MYOG: myogenin.
At 3h post-injection, all treatments showed higher CYP24A1 expression than control (p=0.002; Figure 2B), which indicated the injected 1,25OHD and 25OHD may have activated the catabolism of vitamin D3 in the embryos. At the same time, early osteogenesis differentiation marker (ALP) was increased by high 1,25OHD and high 25OHD injection compared to the control (p=0.0027; Figure 3E). However, no differences were found in RUNX2 (Figure 3D) and SPP1 expression (Figure 3F). With respect to genes involved in adipogenesis, high 25OHD injection induced the expression of FABP4 (p=0.0351; Figure 4D) and FASN (p=0.0339; Figure 4E) compared to the control group, although no differences were detected in the expression of PPAR-γ (Figure 4F). Meanwhile, clear inhibitory effects of vitamin D injection on expression of genes regulating myogenesis were observed. 25OHD treatments significantly decreased MYOD1 expression at 3h (p=0.0224; Figure 5D), and 1,25OHD treatments, and high level 25OHD suppressed the expression of MYF5 (p<0.0001; Figure 5E). No difference in MYOG expression was observed among treatments (Figure 5F).
At 6h post-injection, CYP24A1 expression was significantly higher in 25D-H (p=0.0003; Figure 2C), indicating a higher dosage of 25OHD possibly obtained a longer retention time. Meanwhile, 25D-H has higher SPP1 expression compared to the control (p=0.0061; Figure 3I), but no difference was found in RUNX2 and ALP expression (Figures 3G,H). There were also no differences in adipogenesis gene expression (Figures 4G–I). However, 25OHD treatments down-regulated MYOD1 expression (p=0.0008; Figure 5G), and both high 1,25OHD and 25OHD treatments suppressed MYF5 expression (p=0.0210; Figure 5H). Similar to other time points, no difference in MYOG expression were identified at 6h post-injection (Figure 5I).
In the current study, 25OHD and 1,25OHD showed similar effects on embryogenesis, which indicated the embryo might have acquired the ability to convert 25OHD to 1,25OHD at ED 3. However, to our knowledge, the earliest time that the kidney of chicken embryos can produce 1,25OHD is reported at ED 9 (Bishop and Norman, 1975). Nevertheless, the mesonephros (intermediate kidney) begins to appear at about 55h of incubation, and fully formed at ED 4 (Sturkie, 2012; Bolin and Burggren, 2013). It suggested that the intermediate kidney at this embryo stage could convert 25OHD to 1,25OHD (Kubota et al., 1981). Moreover, CYP27B1 (1α-hydroxylase) has reportedly been expressed in lymph nodes, skin, colon, pancreas, dendritic cells, brain, pulmonary alveolar macrophages, pathological parathyroid glands, prostate cells, and bones in various animal models (Panda et al., 2001; Zehnder et al., 2001; van Driel et al., 2006; Atkins et al., 2007; Adams and Hewison, 2012), suggesting that 25OHD may be converted by tissues outside of the kidney. A previous study has shown that 25OHD could inhibit the proliferation of myogenic cells in a similar manner to 1,25OHD, suggesting that the conversion of 25OHD to 1,25OHD may happen in myoblasts (Srikuea et al., 2012). However, further research needs to be conducted to validate the earliest time that chicken embryos can metabolize vitamin D3.
Both 1,25OHD and 25OHD are metabolized by CYP24A1 (24-hydroxylase), becoming 1,24,25OHD and 24,25OHD, respectively (Norman et al., 1980). 1,25OHD induces CYP24A1 synthesis via several pathways to form a negative feedback loop, avoiding excess 1,25OHD formation (Zierold et al., 1995; DeLuca, 2004). Thus, the expression of CYP24A1 could indicate the catabolism status of vitamin D3 metabolites. Results indicated that the injection had not triggered the enhanced catabolism process at 1h post-injection. However, at 3h post-injection, all the treatments showed higher CYP24A1 expression compared to control group. At 6h post-injection, only the higher level of 25OHD obtained the highest expression of CYP24A1. The catabolism of vitamin D3 in the embryos was surprisingly fast, which may be attributed to the fact that catabolism of vitamin D3 is tightly and rapidly regulated by 1,25OHD (Haussler et al., 2013).
The current study showed the positive effects of vitamin D3 metabolites on ALP (alkaline phosphatase) and SPP1 (osteopontin) expression at 3 and 6h post-injection, respectively. ALP is an early osteogenesis marker and is essential for bone mineralization by enhancing the initial mineral crystal formation (Chatakun et al., 2014). SPP1 codes a highly phosphorylated sialoprotein with strong mineral-binding capacities in the extracellular matrix (Chabas, 2005). The role of vitamin D3 metabolites on osteogenesis has been extensively investigated. However, cell culture studies on the influence of vitamin D3 on osteogenesis have produced varied, and at times conflicting, results (van Driel and van Leeuwen, 2014). In agreement with our findings, 1,25OHD has been shown to stimulate on ALP expression in human osteoblasts (Matsumoto et al., 1991; Siggelkow et al., 1999; Chen et al., 2002; Woeckel et al., 2010). However, 1,25OHD is also reported to down-regulate ALP expression in mouse osteoblasts (Chen et al., 2012; Kim et al., 2016). Meanwhile, expression of Spp1 has been reported to increase in response to 1,25OHD in ROS 17/2.8 cell (rat; Staal et al., 1996). These inconsistent results may reflect different experimental conditions such as species, cell stage, treatment time, and dosages (van Driel and van Leeuwen, 2014). Besides the in vitro studies’ contradictory results, additional vitamin D3 or 25OHD injected to chicken embryos at ED 18 revealed a positive effect on bone quality of hatched chicks (Abbasi et al., 2017; Zamani et al., 2018). It is important to note that the cell culture system has limitations such as missing interactions of different types of cell and lacks consideration of other extracellular factors (van Driel and van Leeuwen, 2014). Indeed, it has been reported that factors, such as phosphate concentration, growth factors, and cytokines, may affect the function of 1,25OHD (van Driel and van Leeuwen, 2014).
The stimulatory effects of vitamin D3 metabolites on adipogenesis at 3h post-injection were observed in the current study. However, in previous studies, the effects of vitamin D3 metabolites on adipocyte differentiation were inconsistent. Stimulatory effects were found in murine studies, but inhibitory effects were frequently shown in the humans (Dix et al., 2018). Other animal studies showed mixed results and are thoroughly reviewed in Dix et al. (2018). In chickens, an in vivo study showed that feeding additional 25OHD did not change the fat pad weight but did increase monounsaturated fatty acids and reduced the polyunsaturated fatty acids (Michalczuk et al., 2010). Even though the vitamin D3 interactions with adipose tissue have been reported in various animal and human models (Ding et al., 2012), limited data are available on vitamin D3 metabolites and chicken adipocytes. The research herein may contribute to our understanding of the fatty liver in old laying hens.
Both osteoblasts and adipocytes are differentiated from embryonic stem cells and mesenchymal stem cells (Kolf et al., 2007). A study has shown that PPAR-γ, the master regulator of adipogenesis (Kawai and Rosen, 2010), could alter mesenchymal stem cells (MSCs) fate by suppressing osteogenic transcription factors, such as homeobox protein DLX5, RUNX2, and Osterix (Kawai and Rosen, 2010), indicating a reciprocal relationship between osteogenic and adipogenic differentiation. Similar results were found in the previous study, where 1,25OHD increased adipogenic differentiation but inhibited osteoblastic cell proliferation and differentiation in rat bone marrow stromal cells (Atmani et al., 2003). However, in the current study, vitamin D3 metabolites stimulated osteogenesis and adipogenesis simultaneously, evidenced by increased expression of FAPB4 and FASN (adipogenesis markers), and ALP (osteogenesis marker) at 3h post-injection. This may reflect that the chicken embryo contains a number of extracellular factors, such as fibroblast growth factors, insulin, and growth factors, contributing to the complexity of vitamin D3 metabolism on adipogenesis (Rosen and MacDougald, 2006).
In the current study, the inhibitory effects of 1,25OHD on myogenic differentiation were shown as a decrease of MYOD1 and MYF5 expression at both 3 and 6h post-injection. MYF5 and MYOD1 are families of Helix-Loop-Helix transcription factors that are expressed during myoblast proliferation and myotube differentiation (Bismuth and Relaix, 2010; Braun and Gautel, 2011). In agreement with the current study, VDR (vitamin D receptor) knockout mice had higher Myf5 and MyoG expression compared to the normal mice (Endo et al., 2003), which indicated the regulatory effects of vitamin D3 on myoblast differentiation. On the contrary, it has been reported that 1,25OHD has a stimulatory effect on the proliferation and differentiation of embryonic chick myoblasts in culture, suggested by an increase in both cell density and fusion after 1,25OHD treatment (Giuliani and Boland, 1984). Likewise, in mice, treatment of C2C12 (mouse myoblast) cells with 1,25OHD increased the MyoD1 and MyoG expression (Garcia et al., 2011). The current paper is contributing to the understanding of these species/cell specific data as it has its own set of unique variables and context. However, the specific pathways involved in such an effect in chicken embryo need to be investigated further.
In summary, 25OHD and 1,25OHD administration to embryos elicited similar responses, suggesting that the embryo may be able to convert 25OHD to 1,25OHD; however, further research is necessary to determine the specific tissue location of the conversion. The catabolism of injected vitamin D3 metabolites appeared to be remarkably fast based on the expression of a key vitamin D3 catabolism-related gene (CYP24A1). The higher dosage of 25OHD showed a possibility of a longer retention time in the embryo. Additionally, both 1,25OHD and 25OHD increased the expression of osteogenesis and adipogenesis-related genes but inhibited myogenesis-related gene expressions during early embryo development in this study. However, the detailed pathways involved in these effects need further studies. Our findings provide an overview of the role of vitamin D3 metabolites in early chicken embryogenesis and the potential basis of practical strategies of early nutrient supplementation in chicken embryos.
The original contributions presented in the study are included in the article/supplementary material, further inquiries can be directed to the corresponding author.
The animal study was reviewed and approved by the Institutional Animal Care and Use Committee at the University of Georgia.
CC and DW: investigation. WK and CC: validation and data curation. CC: formal analysis, writing – original draft preparation, and visualization. WK, BM, and CC: writing – review and editing. WK: supervision, project administration, and funding acquisition. All authors contributed to the article and approved the submitted version.
The authors declare that the research was conducted in the absence of any commercial or financial relationships that could be construed as a potential conflict of interest.
Abbasi, T., Shakeri, M., Zaghari, M., and Kohram, H. (2017). Growth performance parameters, bone calcification and immune response of in ovo injection of 25-hydroxycholecalciferol and vitamin K3 in male ross 308 broilers. Theriogenology 90, 260–265. doi: 10.1016/j.theriogenology.2016.12.016
Adams, J., and Hewison, M. (2012). Extrarenal expression of the 25-hydroxyvitamin D-1-hydroxylase. Arch. Biochem. Biophys. 523, 95–102. doi: 10.1016/j.abb.2012.02.016
Adhikari, R., Chen, C., Waters, E., West, F. D., and Kim, W. K. (2019). Isolation and differentiation of mesenchymal stem cells from broiler chicken compact bones. Front. Physiol. 9:1892. doi: 10.3389/fphys.2018.01892
Atencio, A., Edwards, H. M. Jr., and Pesti, G. M. (2005a). Effect of the level of cholecalciferol supplementation of broiler breeder hen diets on the performance and bone abnormalities of the progeny fed diets containing various levels of calcium or 25-hydroxycholecalciferol. Poult. Sci. 84, 1593–1603. doi: 10.1093/ps/84.10.1593
Atencio, A., Pesti, G., and Edwards, H. Jr. (2005b). Twenty-five hydroxycholecalciferol as a cholecalciferol substitute in broiler breeder hen diets and its effect on the performance and general health of the progeny. Poult. Sci. 84, 1277–1285. doi: 10.1093/ps/84.8.1277
Atkins, G. J., Anderson, P. H., Findlay, D. M., Welldon, K. J., Vincent, C., Zannettino, A. C., et al. (2007). Metabolism of vitamin D3 in human osteoblasts: evidence for autocrine and paracrine activities of 1α, 25-dihydroxyvitamin D3. Bone 40, 1517–1528. doi: 10.1016/j.bone.2007.02.024
Atmani, H., Chappard, D., and Basle, M. F. (2003). Proliferation and differentiation of osteoblasts and adipocytes in rat bone marrow stromal cell cultures: effects of dexamethasone and calcitriol. J. Cell. Biochem. 89, 364–372. doi: 10.1002/jcb.10507
Bhat, M., Noolu, B., Qadri, S. S., and Ismail, A. (2014). Vitamin D deficiency decreases adiposity in rats and causes altered expression of uncoupling proteins and steroid receptor coactivator3. J. Steroid Biochem. Mol. Biol. 144, 304–312. doi: 10.1016/j.jsbmb.2014.08.005
Bishop, J. E., and Norman, A. W. (1975). Studies on calciferol metabolism: metabolism of 25-hydroxy-vitamin D3 by the chicken embryo. Arch. Biochem. Biophys. 167, 769–773. doi: 10.1016/0003-9861(75)90523-8
Bismuth, K., and Relaix, F. (2010). Genetic regulation of skeletal muscle development. Exp. Cell Res. 316, 3081–3086. doi: 10.1016/j.yexcr.2010.08.018
Bolin, G., and Burggren, W. W. (2013). Metanephric kidney development in the chicken embryo: glomerular numbers, characteristics and perfusion. Comp. Biochem. Physiol. A Mol. Integr. Physiol. 166, 343–350. doi: 10.1016/j.cbpa.2013.07.011
Braun, T., and Gautel, M. (2011). Transcriptional mechanisms regulating skeletal muscle differentiation, growth and homeostasis. Nat. Rev. Mol. Cell Biol. 12, 349–361. doi: 10.1038/nrm3118
Chabas, D. (2005). Osteopontin, a multi-faceted molecule. Med. Sci. 21, 832–838. doi: 10.1051/medsci/20052110832
Chatakun, P., Nunez-Toldra, R., Diaz Lopez, E. J., Gil-Recio, C., Martinez-Sarra, E., Hernandez-Alfaro, F., et al. (2014). The effect of five proteins on stem cells used for osteoblast differentiation and proliferation: a current review of the literature. Cell. Mol. Life Sci. 71, 113–142. doi: 10.1007/s00018-013-1326-0
Chen, F. -P., Lee, N., Wang, K. -C., Soong, Y. -K., and Huang, K. -E. (2002). Effect of estrogen and 1α, 25 (OH) 2-vitamin D3 on the activity and growth of human primary osteoblast-like cells in vitro. Fertil. Steril. 77, 1038–1043. doi: 10.1016/s0015-0282(02)03065-0
Chen, Y. C., Ninomiya, T., Hosoya, A., Hiraga, T., Miyazawa, H., and Nakamura, H. (2012). 1alpha,25-Dihydroxyvitamin D3 inhibits osteoblastic differentiation of mouse periodontal fibroblasts. Arch. Oral Biol. 57, 453–459. doi: 10.1016/j.archoralbio.2011.10.005
Chen, C., Turner, B., Applegate, T., Litta, G., and Kim, W. (2020). Role of long-term supplementation of 25-hydroxyvitamin D3 on laying hen bone 3-dimensional structural development. Poult. Sci. 99, 5771–5782. doi: 10.1016/j.psj.2020.06.080
Christakos, S., Ajibade, D. V., Dhawan, P., Fechner, A. J., and Mady, L. J. (2010). Vitamin D: metabolism. Endocrinol. Metab. Clin. N. Am. 39, 243–253. doi: 10.1016/j.ecl.2010.02.002
DeLuca, H. F. (2004). Overview of general physiologic features and functions of vitamin D. Am. J. Clin. Nutr. 80, 1689S–1696S. doi: 10.1093/ajcn/80.6.1689S
Ding, C., Gao, D., Wilding, J., Trayhurn, P., and Bing, C. (2012). Vitamin D signalling in adipose tissue. Br. J. Nutr. 108, 1915–1923. doi: 10.1017/S0007114512003285
Dix, C. F., Barcley, J. L., and Wright, O. R. L. (2018). The role of vitamin D in adipogenesis. Nutr. Rev. 76, 47–59. doi: 10.1093/nutrit/nux056
Endo, I., Inoue, D., Mitsui, T., Umaki, Y., Akaike, M., Yoshizawa, T., et al. (2003). Deletion of vitamin D receptor gene in mice results in abnormal skeletal muscle development with deregulated expression of myoregulatory transcription factors. Endocrinology 144, 5138–5144. doi: 10.1210/en.2003-0502
Fatemi, S. A., Elliott, K. E. C., Bello, A., Durojaye, O. A., Zhang, H. J., and Peebles, E. D. (2020). The effects of in ovo injected vitamin D3 sources on the eggshell temperature and early posthatch performance of Ross 708 broilers. Poult. Sci. 99, 1357–1362. doi: 10.1016/j.psj.2019.10.055
Garcia, L. A., King, K. K., Ferrini, M. G., Norris, K. C., and Artaza, J. N. (2011). 1,25(OH)2vitamin D3 stimulates myogenic differentiation by inhibiting cell proliferation and modulating the expression of promyogenic growth factors and myostatin in C2C12 skeletal muscle cells. Endocrinology 152, 2976–2986. doi: 10.1210/en.2011-0159
Girgis, C. M., Clifton-Bligh, R. J., Hamrick, M. W., Holick, M. F., and Gunton, J. E. (2013). The roles of vitamin D in skeletal muscle: form, function, and metabolism. Endocr. Rev. 34, 33–83. doi: 10.1210/er.2012-1012
Giuliani, D. L., and Boland, R. L. (1984). Effects of vitamin D 3 metabolites on calcium fluxes in intact chicken skeletal muscle and myoblasts culturedin vitro. Calcif. Tissue Int. 36, 200–205. doi: 10.1007/BF02405318
Han, J. C., Chen, G. H., Wang, J. G., Zhang, J. L., Qu, H. X., Zhang, C. M., et al. (2016). Evaluation of relative bioavailability of 25-hydroxycholecalciferol to cholecalciferol for broiler chickens. Asian-Australas. J. Anim. Sci. 29, 1145–1151. doi: 10.5713/ajas.15.0553
Haussler, M. R., Whitfield, G. K., Kaneko, I., Haussler, C. A., Hsieh, D., Hsieh, J. C., et al. (2013). Molecular mechanisms of vitamin D action. Calcif. Tissue Int. 92, 77–98. doi: 10.1007/s00223-012-9619-0
Ji, S., Doumit, M. E., and Hill, R. A. (2015). Regulation of adipogenesis and key adipogenic gene expression by 1, 25-dihydroxyvitamin D in 3T3-L1 cells. PLoS One 10:e0126142. doi: 10.1371/journal.pone.0126142
Kawai, M., and Rosen, C. J. (2010). PPARγ: a circadian transcription factor in adipogenesis and osteogenesis. Nat. Rev. Endocrinol. 6, 629–636. doi: 10.1038/nrendo.2010.155
Kim, J. H., Seong, S., Kim, K., Kim, I., Jeong, B. C., and Kim, N. (2016). Downregulation of Runx2 by 1,25-dihydroxyvitamin D(3) induces the transdifferentiation of osteoblasts to adipocytes. Int. J. Mol. Sci. 17:770. doi: 10.3390/ijms17050770
Kolf, C. M., Cho, E., and Tuan, R. S. (2007). Mesenchymal stromal cells. Biology of adult mesenchymal stem cells: regulation of niche, self-renewal and differentiation. Arthritis Res. Ther. 9:204. doi: 10.1186/ar2116
Koreleski, J., and Swiatkiewicz, S. (2005). Efficacy of different limestone particle size and 25-hydroxycholecalciferol in broiler diets. J. Anim. Feed Sci. 14, 705–714. doi: 10.22358/jafs/67161/2005
Kubota, M., Abe, E., Shinki, T., and Suda, T. (1981). Vitamin D metabolism and its possible role in the developing chick embryo. Biochem. J. 194, 103–109. doi: 10.1042/bj1940103
Livak, K. J., and Schmittgen, T. D. (2001). Analysis of relative gene expression data using real-time quantitative PCR and the 2−ΔΔCT method. Methods 25, 402–408. doi: 10.1006/meth.2001.1262
Matsumoto, T., Igarashi, C., Takeuchi, Y., Harada, S., Kikuchi, T., Yamato, H., et al. (1991). Stimulation by 1, 25-dihydroxyvitamin D3 of in vitro mineralization induced by osteoblast-like MC3T3-E1 cells. Bone 12, 27–32. doi: 10.1016/8756-3282(91)90051-j
Michalczuk, M., Pietrzak, D., Niemiec, J., and Mroczek, J. (2010). Effectiveness of vitamin D3 and calcidiol (25-OH-D3) application in feeding broiler chickens-production performance and meat quality. Polish J. Food Nutr. Sci. 60, 121–126.
Norman, A. W., Henry, H. L., and Malluche, H. H. (1980). 24R, 25-Dihydroxyvitamin D3 and 1α, 25-dihydroxyvitamin D3 are both indispensable for calcium and phosphorus homeostasis. Life Sci. 27, 229–237. doi: 10.1016/0024-3205(80)90142-3
Ovesen, L., Brot, C., and Jakobsen, J. (2003). Food contents and biological activity of 25-hydroxyvitamin D: a vitamin D metabolite to be reckoned with? Ann. Nutr. Metab. 47, 107–113. doi: 10.1159/000070031
Panda, D. K., Miao, D., Tremblay, M. L., Sirois, J., Farookhi, R., Hendy, G. N., et al. (2001). Targeted ablation of the 25-hydroxyvitamin D 1alpha -hydroxylase enzyme: evidence for skeletal, reproductive, and immune dysfunction. Proc. Natl. Acad. Sci. U. S. A. 98, 7498–7503. doi: 10.1073/pnas.131029498
Rosen, E. D., and MacDougald, O. A. (2006). Adipocyte differentiation from the inside out. Nat. Rev. Mol. Cell Biol. 7, 885–896. doi: 10.1038/nrm2066
Saunders-Blades, J. L., and Korver, D. R. (2014). The effect of maternal vitamin D source on broiler hatching egg quality, hatchability, and progeny bone mineral density and performance. J. Appl. Poult. Res. 23, 773–783. doi: 10.3382/japr.2014-01006
Siggelkow, H., Schulz, H., Kaesler, S., Benzler, K., Atkinson, M., and Hüfner, M. (1999). 1, 25 Dihydroxyvitamin-D3 attenuates the confluence-dependent differences in the osteoblast characteristic proteins alkaline phosphatase, procollagen I peptide, and osteocalcin. Calcif. Tissue Int. 64, 414–421.
Soares, J. Jr., Kerr, J., and Gray, R. (1995). 25-hydroxycholecalciferol in poultry nutrition. Poult. Sci. 74, 1919–1934. doi: 10.3382/ps.0741919
Srikuea, R., Zhang, X., Park-Sarge, O. -K., and Esser, K. A. (2012). VDR and CYP27B1 are expressed in C2C12 cells and regenerating skeletal muscle: potential role in suppression of myoblast proliferation. Am. J. Phys. 303, C396–C405. doi: 10.1152/ajpcell.00014.2012
Staal, A., Van Wijnen, A. J., Desai, R. K., Pols, H., Birkenhäger, J., DeLuca, H. F., et al. (1996). Antagonistic effects of transforming growth factor-beta on vitamin D3 enhancement of osteocalcin and osteopontin transcription: reduced interactions of vitamin D receptor/retinoid X receptor complexes with vitamin E response elements. Endocrinology 137, 2001–2011. doi: 10.1210/endo.137.5.8612541
St-Arnaud, R. (2008). The direct role of vitamin D on bone homeostasis. Arch. Biochem. Biophys. 473, 225–230. doi: 10.1016/j.abb.2008.03.038
Sunde, M., Turk, C., and DeLuca, H. (1978). The essentiality of vitamin D metabolites for embryonic chick development. Science 200, 1067–1069. doi: 10.1126/science.206963
van Driel, M., Koedam, M., Buurman, C., Hewison, M., Chiba, H., Uitterlinden, A., et al. (2006). Evidence for auto/paracrine actions of vitamin D in bone: 1α-hydroxylase expression and activity in human bone cells. FASEB J. 20, 2417–2419. doi: 10.1096/fj.06-6374fje
van Driel, M., and van Leeuwen, J. P. (2014). Vitamin D endocrine system and osteoblasts. Bonekey Rep. 3:493. doi: 10.1038/bonekey.2013.227
Veldurthy, V., Wei, R., Campbell, M., Lupicki, K., Dhawan, P., and Christakos, S. (2016). 25-Hydroxyvitamin D3 24-hydroxylase: a key regulator of 1, 25 (OH) 2D3 catabolism and calcium homeostasis. Vitam. Horm. 100, 137–150. doi: 10.1016/bs.vh.2015.10.005
Vieira, S. L. (2007). Chicken embryo utilization of egg micronutrients. Rev. Bras. Cienc. Avic. 9, 1–8. doi: 10.1590/S1516-635X2007000100001
Wagatsuma, A., and Sakuma, K. (2014). Vitamin D signaling in myogenesis: potential for treatment of sarcopenia. Biomed. Res. Int. 2014:121254. doi: 10.1155/2014/121254
Wideman, R. Jr., Blankenship, J., Pevzner, I., and Turner, B. (2015). Efficacy of 25-OH vitamin D3 prophylactic administration for reducing lameness in broilers grown on wire flooring. Poult. Sci. 94, 1821–1827. doi: 10.3382/ps/pev160
Woeckel, V., Alves, R., Swagemakers, S., Eijken, M., Chiba, H., Van Der Eerden, B., et al. (2010). 1α, 25-(OH) 2D3 acts in the early phase of osteoblast differentiation to enhance mineralization via accelerated production of mature matrix vesicles. J. Cell. Physiol. 225, 593–600. doi: 10.1002/jcp.22244
Zamani, A., Shariatmadari, F., Rahimi, S., and Karimi Torshizi, M. A. (2018). Effects of in ovo injection of carbohydrates, β-hydroxy-β-methylbutyrate, and vitamins on ostrich organ weight, bone characteristics, and small intestinal morphology. Can. J. Anim. Sci. 99, 116–122. doi: 10.1139/cjas-2017-0167
Zehnder, D., Bland, R., Williams, M. C., McNinch, R. W., Howie, A. J., Stewart, P. M., et al. (2001). Extrarenal expression of 25-hydroxyvitamin D3-1α-hydroxylase. J. Clin. Endocrinol. Metab. 86, 888–894. doi: 10.1210/jcem.86.2.7220
Keywords: vitamin D3, chicken embryo, osteogenesis, adipogenesis, myogenesis, metabolism, gene expression
Citation: Chen C, White DL, Marshall B and Kim WK (2021) Role of 25-Hydroxyvitamin D3 and 1,25-Dihydroxyvitamin D3 in Chicken Embryo Osteogenesis, Adipogenesis, Myogenesis, and Vitamin D3 Metabolism. Front. Physiol. 12:637629. doi: 10.3389/fphys.2021.637629
Received: 04 December 2020; Accepted: 05 January 2021;
Published: 01 February 2021.
Edited by:
Massimiliano Petracci, University of Bologna, ItalyReviewed by:
Martina Zappaterra, University of Bologna, ItalyCopyright © 2021 Chen, White, Marshall and Kim. This is an open-access article distributed under the terms of the Creative Commons Attribution License (CC BY). The use, distribution or reproduction in other forums is permitted, provided the original author(s) and the copyright owner(s) are credited and that the original publication in this journal is cited, in accordance with accepted academic practice. No use, distribution or reproduction is permitted which does not comply with these terms.
*Correspondence: Woo Kyun Kim, d2traW1AdWdhLmVkdQ==
Disclaimer: All claims expressed in this article are solely those of the authors and do not necessarily represent those of their affiliated organizations, or those of the publisher, the editors and the reviewers. Any product that may be evaluated in this article or claim that may be made by its manufacturer is not guaranteed or endorsed by the publisher.
Research integrity at Frontiers
Learn more about the work of our research integrity team to safeguard the quality of each article we publish.