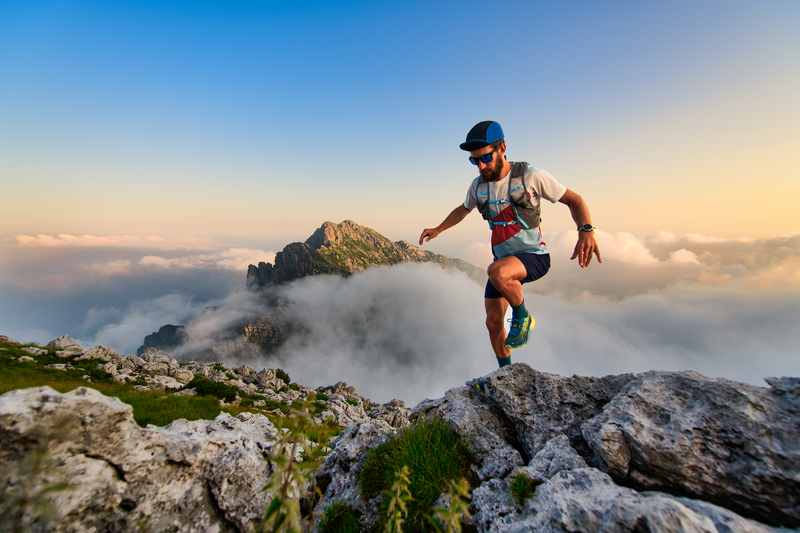
94% of researchers rate our articles as excellent or good
Learn more about the work of our research integrity team to safeguard the quality of each article we publish.
Find out more
ORIGINAL RESEARCH article
Front. Physiol. , 28 January 2021
Sec. Exercise Physiology
Volume 12 - 2021 | https://doi.org/10.3389/fphys.2021.632664
Only a few studies have evaluated changes in mitochondrial function and oxidative stress associated with ultramarathon running. Invasive biopsies are needed to assess mitochondrial function of skeletal muscle, which may not be well tolerated by some individuals. Platelets (PLTs) as a metabolically highly active and homogenous cell population were suggested as a potentially valuable surrogate to investigate mitochondrial function. Thus, this study was aimed to evaluate mitochondrial function of PLTs and its association with individual race performance and markers of oxidative stress, muscle damage and renal dysfunction. Race performance and mitochondrial function (high-resolution respirometry, HRR) of PLTs using different substrates inducing ROUTINE, LEAK, N-pathway control state (Complex I linked oxidative phosphorylation; CI, OXPHOS), NS-pathway control state (CI + II linked OXPHOS and electron transfer pathway; ET), S-pathway control state (CII linked ET) as well as parameters of oxidative stress and antioxidant capacity, and markers of muscle and renal injury were assessed in eight male ultramarathon runners (26–45 years) before, immediately after and 24 h after an ultramarathon race (PRE, POST, and REC). Ultramarathon running induced an increase in LEAK O2 flux of PLT mitochondria and slight, largely non-significant changes in the oxidant/antioxidant balance. Levels of creatine kinase (CK), lactate dehydrogenase (LDH), blood urea nitrogen, and creatinine were all significantly elevated POST and remained high in REC. There were inverse correlations between race time and N-linked substrate state PRE-POST, and changes in CK and LDH levels were significantly related to PLT mitochondrial LEAK and N-linked respiration PRE. Although race-related changes in respirometry parameters of PLT mitochondria were rather small, a somewhat more pronounced increase in the relative N-linked respiration in faster runners might suggest PLT CI as indicator of physical fitness. The higher PLT LEAK PRE and diminished increase of CK during the race may represent a prophylactic preconditioning and the slight but non-significant elevation of the antioxidant potential post-race as a protective consequence of the race-related oxidative stress and potential threat to the kidney. Our findings point toward an interrelationship between mitochondrial function of PLTs, individual fitness levels and extreme physical and metal stresses, which stimulates further research.
Long-distance endurance competitions are physically and mentally challenging events affecting various physiological systems (Knechtle and Nikolaidis, 2018). In contrast to a standard marathon, (patho) physiological responses to an ultramarathon (any race > 42.2 km in distance) have not been evaluated extensively (Knechtle and Nikolaidis, 2018; Hoppel et al., 2019). Whereas markers of inflammation, muscle tissue damage, liver and renal function are among the most characterized (Jastrzębski et al., 2015; Knechtle and Nikolaidis, 2018; Hoppel et al., 2019), only a few studies have assessed mitochondrial function and oxidative stress associated with ultramarathon running (Knez et al., 2006; Fernström et al., 2007; Hattori et al., 2009; Sahlin et al., 2010; de Lucas et al., 2014; Mrakic-Sposta et al., 2015). Skeletal muscle mitochondria are key for cellular energy homeostasis and adaptation to physical activity (Lee and Shao, 2014; Porter et al., 2015; Christensen et al., 2016), but biopsies are needed to assess mitochondrial function, which is an invasive procedure and may not be well tolerated by some individuals. Therefore, platelets as a metabolically highly active and homogenous cell population have been suggested as a potentially valuable surrogate to investigate mitochondrial function (Kramer et al., 2014; Melchinger et al., 2019).
PLTs have been suggested to reflect the systemic effects of exercise on multi-organ systems based on existing data on the release of different cytokines, ions, metabolites and tricarboxylic acid (TCA) cycle intermediates from contracting muscles to the blood stream (primarily span-2 metabolites: succinate, malate, and fumarate), which are further affecting succinate receptor 1 (SUCNR1) positive cells such as PLTs in a dose-dependent manner (Gibala et al., 1998; Lewis et al., 2010; Högberg et al., 2011; Ariza et al., 2012; Knechtle and Nikolaidis, 2018). Moreover, recent studies have demonstrated parallels between oxidative respiration of skeletal muscle mitochondria and those of PLTs (Tyrrell et al., 2016; Braganza et al., 2019; Rose et al., 2019). Thus, the well accepted association between cardiorespiratory fitness and OXPHOS capacity of skeletal muscles (Walsh et al., 2001; Daussin et al., 2008; Pesta et al., 2011; Jacobs and Lundby, 2013; Christensen et al., 2016) might also apply (at least partly) to PLT mitochondrial function (Tyrrell et al., 2016). To the best of our knowledge, only one study has dealt with effects ultra-endurance exercise on PLT metabolism (de Lucas et al., 2014).
Of course, PLT metabolism is highly involved in a variety of systemic processes such as hemostasis, immunoregulation, inflammation and oxidative stress (de Lucas et al., 2014; Posthuma et al., 2015; Taeb et al., 2017; Melchinger et al., 2019). All of them are known to be affected by prolonged (intensive) exercise (Knechtle and Nikolaidis, 2018; Hoppel et al., 2019) and may also be involved in (muscle) tissue damage (Mastaloudis et al., 2006) and/or dysfunction of various organs (Ratliff et al., 2016). With regard to ultra-endurance exercise, increased PLT activity are of interest because it may provoke thromboembolic events under certain conditions (Posthuma et al., 2015; Taeb et al., 2017).
The mitochondrial respiratory chain represents the main source of oxygen-derived free radicals (ROS) in addition to other intracellular sources (e.g., xanthine oxidase, NADPH oxidase, peroxisomal oxidative enzymes) (Mastaloudis et al., 2004; Sahlin et al., 2010). Oxidant species may activate signaling pathways promoting cellular adaptations to exercise but may also become detrimental in certain cases (Hattori et al., 2009; Mrakic-Sposta et al., 2015; He et al., 2016). ROS rapidly react with cellular components and, following exhaustive exercise, a significant increase in ROS production can overcome antioxidant defense (resulting in oxidative stress) (Mrakic-Sposta et al., 2015; He et al., 2016). Adverse consequences may include damage of lipids, proteins and nucleic acids, perturbed cellular mechanisms or inflammation, potentially aggravated with aging, and pre-existing various diseases (Kohen and Nyska, 2002; Hattori et al., 2009; Nikolaidis et al., 2012; Mrakic-Sposta et al., 2015; He et al., 2016). An increased production of ROS as a consequence of high energy demand during exhaustive exercise like ultramarathon running is a well-established finding (Niemann et al., 2002; Knez et al., 2006; Fernström et al., 2007; Hattori et al., 2009; de Lucas et al., 2014; Mrakic-Sposta et al., 2015; He et al., 2016). This has been demonstrated in skeletal muscle mitochondria (Fernström et al., 2007) but fluctuating ROS generation during varying stress conditions may also play a key role in PLTs (Yamagishi et al., 2001; Lopez and Rosado, 2007; Melchinger et al., 2019).
Muscle damage and renal dysfunction are main adverse consequences of extreme endurance performance such as ultramarathon running and both are closely associated with inflammation (Pedersen and Hoffman-Goetz, 2000; Jastrzębski et al., 2015; Knechtle and Nikolaidis, 2018; Hoppel et al., 2019) and oxidative stress (Meyer et al., 2006; Ma et al., 2016). Based on the growing interest for a putative role of PLTs in sensing metabolic and inflammatory stresses, the primary goal of the present study was the evaluation of alterations in mitochondrial function of PLTs by ultramarathon running (Millet and Millet, 2012) and their association with performance, muscle and kidney injury. We hypothesized that there are significant interrelationships between changes in mitochondrial function of PLTs, individual race performance and markers of oxidative stress, muscle damage and renal dysfunction.
Local runners in the age group of 20–50 were selected from the entry list of all male starters. Since measurements were performed on 3 consecutive days, it was only feasible for locals to participate. The 32 eligible individuals were contacted 4 weeks prior to the event, informed about the procedure of measurements and asked to fill a questionnaire on anthropometric data, training and medical history, and smoking behavior if they consented study participation. Exclusion criteria were any disease associated with an increased risk due to the ultramarathon running event (assessed by an experienced physician), and very high training volumes with the intention to recruit only recreational participants (a recreational athlete was defined as training ≤ 15 h/week). Ten race participants (training volume: 4–15 h, median: 8.5 h) were included after providing written informed consent (Table 1). Due to the hot weather conditions[average ambient air temperature: 20.1°C (17–37°C), relative humidity: 54% (45–61%)], two participants did not complete the race and thus 8 individuals were included into the final analysis. The study was approved by the local ethics review board (University of Innsbruck, Institute of Sport Science).
The competition was performed as a non-stop mountain ultramarathon (67 km; approximately 4,500 m of total ascent), taking place in Gmunden, Upper Austria, Austria. Starting time was chosen individually by the competitors anytime between 3:00 – 5:00 am, cut off for finishing the race was set at 9:00 pm at the same day. Figure 1 provides a course outline of the race. Participants were wearing a heart rate monitor during competition with a recording interval of one second (Suunto Ambit 3; Suunto, Vantaa, Finland) to monitor race times, and average and maximal heart rates during the race (Suunto Movescount). Race time and average running speed were calculated using exact start and finishing times, provided by the data service of the race.
Venous blood sampling was performed on three consecutive days: the day before the competition (baseline condition, PRE), within 10 min after finishing the race (POST) and 24 h after the individual finish (REC) using different blood collection tubes (27 mL K3 EDTA, 6 mL Lithium Heparin, 3 mL clot activator for serum analysis; BD Vacutainer, BD diagnostics, NJ, United States). Height and resting heart rate measurements were performed PRE, body mass was determined PRE and POST. Blood lactate concentrations were only determined POST. See Figure 2 for the timeline of all measurements.
Six OROBOROS Oxygraphs 2k (Oroboros Instruments, Innsbruck, Austria) were used simultaneously to assess PLT mitochondrial function. Data were corrected for instrumental background oxygen flux and Oxygraphs were calibrated before measurement procedure every day in mitochondrial respiration media (MiR05). Each two 9 mL K3EDTA monovettes whole blood were used to extract purified PLTs for subsequent use by two specifically designed centrifugation steps. Respiratory O2 flux (JO2) was traced in various combinations of coupling control states and electron transfer pathways by applying a variation of the Oroboros Substrate-Uncoupler-Inhibitor Titration-8 protocol (SUIT 8) and analyzed using Dat Lab 6.0 analysis software (OROBOROS DatLab 6.0). Chemicals used and mitochondrial pathways, states and fluxes are described/defined in Tables 2, 3. The experimental procedure including sampling, sample preparation, experimental high-resolution respirometry (HRR) performance and data analysis is based on research currently published (Sjoevall et al., 2013; Fasching et al., 2016; Lemieux et al., 2017; Fasching and Gnaiger, 2018; Sumbalová et al., 2018).
Table 2. SUIT protocol, recorded respiratory states and calculated flux control ratios and flux control factors.
For data analysis, flux control ratios (FCR) and flux control factors (FCF) were calculated from data previously corrected for residual oxygen consumption (ROX) according to Gnaiger (2014). FCR are obtained by normalization of O2 fluxes to the respective highest flux of the experiment as reference state (Gnaiger et al., 2019). FCF express the relative change of respiration in a defined coupling state in a step, caused by a specific metabolic control variable (titration of a single substrate), normally being the next higher flux during experimental procedure (Gnaiger et al., 2019; Gnaiger, 2020). In contrast to normalization per cell number or mitochondrial marker, results expressed as FCR and FCF are statistically more reliable (Gnaiger et al., 2019). See Table 2 for details of the performed SUIT protocol and calculated FCR and FCF. For explanation of abbreviations please see Tables 3 and 4.
Nine-mL K3 EDTA, 3 mL Lithium Heparin and 3 mL clot activator blood were analyzed on each sampling day at the local hospital laboratory (Salzkammergut-Klinikum, Gmunden, Austria) regarding indirect muscle, liver and renal damage markers (creatine kinase, CK; lactate dehydrogenase, LDH; serum creatinine, CR; blood urea nitrogen, BUN). With respect to the study protocol, blood was collected in non-fasting conditions. The method has been previously described elsewhere (Hoppel et al., 2019).
EDTA plasma collected from PLT sample preparation for HRR and plasma from heparinized blood (3 mL monovette) were frozen and stored in dry ice for subsequent analysis of plasma oxidative stress and biological antioxidative potential at the University of Innsbruck (Institute of Sports Science, University of Innsbruck, Austria). To evaluate the changes in oxidant species and non-enzymatic scavengers we used the d-ROMs and BAP tests (Diacron, Grosseto, Italy). The principle of d-ROMs is based on a catalytic oxidation of Fe3+ and free radicals to radical alkoxyl and radical peroxyl, oxidizing a chromogen (N, N,-Diethylparap-henylendiamin) to a reddish dye. The measured concentration of hydroperoxides is correlated with intensity of the dye and expressed as Carratelli units (CARR U). Reference values for a normal level of reactive oxygen metabolites are in the range of 250–300 CARR U, consistent with 20 to 24 mg/dl H2O2. BAP test is based on the reduction of Fe3+ ions bound to chromogen Thiocyanat to Fe2+, by adding a biological sample with antioxidative defense capacity. Level of discoloration of the dye correlates with antioxidative potential of the sample and is expressed as μMol/L. Reference for good antioxidative capacity is >2,000 μMol/L (Alberti et al., 2000). To express differences between antioxidant and pro-oxidant potential, the ratio of BAP to d-ROMs has been calculated (BAP/d-ROMs). Outcomes using d-ROMs and BAP tests are robust as long as samples are stored at −20°C or lower as performed in our study (Celi et al., 2010).
Athletes’ anthropometric characteristics and race results are given as mean ± standard deviation (SD). Individual data of HRR measurements (Supplementary Figure 1) and oxidative status parameter (Supplementary Figure 2) are shown in the supplementary material and those of indirect tissue damage parameters in Hoppel et al. (Hoppel et al., 2019).
FCR, FCF and parameters of oxidative status, indirect muscle damage and renal function were analyzed regarding changes PRE-POST-REC using repeated one-way ANOVA with post hoc analysis (Bonferroni correction) for normal distributed data. Wilcoxon signed-rank test and Friedman test were applied for data not meeting ANOVA test requirements. Percentage differences were calculated for PRE-POST and PRE-REC (Tables 5, 6). PRE data were subtracted from POST (ΔPRE-POST) such as from REC (ΔPRE-REC) for FCR, FCF, d-ROMS, BAP and indirect markers of muscle damage and renal function to calculate effective changes of these parameters during the race.
Table 5. Flux control ratios and flux control factors before competition (PRE), immediately after the race (POST) and 24 h after finishing (REC).
Table 6. Oxidative stress parameters and indirect markers of muscular damage and renal dysfunction before competition (PRE), immediately after the race (POST) and 24 h after finishing (REC).
Interactions between HRR data (ΔFCR and ΔFCF PRE-POST), athletes anthropometry and race performance parameters [age, race time, training/week, body mass index (BMI)] were analyzed by a two-tailed Spearman’s rank correlation (r). R was also used to determine interactions between PLT HRR and markers of oxidative stress, tissue damage and renal function. This was done for PRE, POST and ΔPRE-POST data. All statistics were performed using IBM SPSS Statistics 24.0 (SPSS Inc., Chicago, IL, United States). Based on data of de Lucas et al., a power of 80% (regarding changes of PLT mitochondrial function) is expected even with our eight participants (alpha set at 0.05). An effect size (η2) of <0.1 was considered as small effect, 0.1–0.6 as medium effect and >0.6 as large effect (Cohen, 1988).
Selected participant characteristics and race performance data are shown in Table 7. Most drop-outs during the race, including the two of the present study, occurred due to hot weather conditions (up to 37°C; average relative humidity 54%) (Hoppel et al., 2019). No serious health consequences occurred during or after the race in study participants, despite significant changes in leucocyte counts and indirect markers of potential muscular and renal damage (Hoppel et al., 2019). Race time was only correlated with BMI of the athletes (r = 0.862), while age, training volume and body mass change during the race (−0.2%) was not associated with running performance (Hoppel et al., 2019).
Calculated FCR and FCF PRE, POST and REC are presented in Table 5. Only PML (+28.4%) and 1-PML/PMP (−19.2%) changed significantly from PRE to POST. ΔPGMP PRE-POST was inversely correlated to race time (r = −0.829) but positively to BMI PRE (r = 0.826) (Figure 3).
Figure 3. Regression (95% confidence intervals) of ΔPGMP PRE-POST to race performance. Changes of FCRs of the CI OXPHOS state (substrates: PGM) PRE-POST to race time of the participants.
Markers of oxidative stress and those of potential muscular damage and renal injury are presented in Table 6. CK, LDH, BUN, and CR increased significantly from PRE to POST and remained elevated from PRE to REC. BAP/d-ROMS decreased significantly PRE-REC. Correlations between HRR parameters and markers of oxidative stress, muscle and kidney injury are presented in Figure 4. PML PRE was inversely correlated to ΔCK PRE-POST (r = −0.829) and PGMP PRE to ΔLDH PRE-POST (r = −0.841). In addition, ΔBAP/d-ROMs PRE-POST was positively related to ΔCR PRE-POST (r = 0.829).
Figure 4. Regression (95% confidence intervals) of HRR parameters to changes in indirect markers for tissue damage. (A) FCRs of the LEAK state (PML) PRE to changes of CK PRE-POST. (B) FCRs of the CI OXPHOS state (substrates: PGM) to changes of LDH (ΔLDH) PRE-POST. (C) Changes of oxidative stress markers (ΔBAP/d-ROMs) PRE-POST to changes of CR (ΔCR) PRE-POST. Drop-outs are marked as cross and are not included in statistics.
Our study shows that ultramarathon running induces an increase in LEAK O2 flux of PLT mitochondria and slight, largely non-significant changes in the oxidant/antioxidant balance. In addition, correlation analyses indicate a potential association between HRR parameters and those of skeletal muscle and kidney injury due to ultramarathon running.
Both FCR PML and FCF 1-PML/PMP express the relative amount of O2 being consumed in the LEAK state in relation to fully stimulated and physiologically coupled (PGMSP) or partially stimulated and coupled OXPHOS respiration (PMP) (Gnaiger et al., 2019; Gnaiger, 2020). Both positive ΔPML PRE-POST and negative Δ1-PML/PMP PRE-POST are highlighting increased H+ leakage across the PLT mitochondrial inner membrane (intrinsic uncoupling), being associated with diminished respiratory efficiency (P»/O2 flux ratio) but increased formation of ROS (Brookes, 2005; Gnaiger et al., 2019). Basically, PLTs play a pivotal role in hemostasis, due to their accumulation at the site of injury. Whereas PLTs in a resting state are powered by glycolysis and oxidative phosphorylation, OXPHOS may be more important in activated platelets (Aibibula et al., 2018), favoring thrombus formation (Zharikov and Shiva, 2013). A previous study has shown that ultra-endurance performance is increasing PLT O2 consumption (de Lucas et al., 2014), which in turn triggers PLT aggregation (Melchinger et al., 2019), and hereby potentially elevating the risk of thromboembolic events in some athletes (Zaleski et al., 2016; Taeb et al., 2017). The increased intrinsic uncoupling shown in our study might therefore be interpreted as protection against thromboembolism formation (Fernström et al., 2007; Gnaiger et al., 2019).
In PLT OXPHOS, CI contributes to about 2/3 of total respiration (CI + II) at convergent electron input into the Q-junction, thus being a highly sensitive marker for a variety of diseases such as Parkinson’s or Huntington’s disease (Sjoevall et al., 2013; Zharikov and Shiva, 2013; Melchinger et al., 2019). In skeletal muscle, CI is a main source of superoxide anion radicals (O2–), but also known as a marker of cardiorespiratory fitness (Dröse and Brandt, 2008; Votion et al., 2012). If this significance of skeletal muscle CI also holds true in PLTs, the relative decrease of CI and/or increase of CII activity in slower (less fit) runners could be related to increased ROS formation, while faster (more fit) runners increasing their relative CI activity might be less affected by oxidative stress (Zharikov and Shiva, 2013). Thus, the observed relation between ΔPGMP PRE-POST and race time may indicate that PLT activation and associated risk of thrombosis change with race performance. However, despite existing evidence of PLT mitochondrial parameters paralleling those of skeletal muscle (Tyrrell et al., 2016; Braganza et al., 2019; Rose et al., 2019), in data interpretation one should consider basal differences of PLT and muscle metabolic functional involvements (Lanza and Sreekumaran Nair, 2010; Melchinger et al., 2019). Further research is needed to strengthen these suggestions.
Systemic oxidative stress characterized by excessive ROS production and diminished antioxidative capacity is a well-known consequence of ultramarathon running (Hattori et al., 2009; de Lucas et al., 2014). Besides oxidative damage of carbohydrates, lipids and proteins, oxidative stress is associated with inflammation but also with the risk of muscular and renal injury (Ratliff et al., 2016; Liguori et al., 2018). Based on the relation between higher PLT mitochondrial LEAK PRE and less increase of CK during the race, we speculated that the larger LEAK PRE might represent a kind of prophylactic preconditioning.
High serum CR and acute kidney injury (AKI) are known adverse consequences of prolonged intense endurance exercise (Hodgson et al., 2017; Hoppel et al., 2019). Elevated ROS production is closely related to pathophysiological processes as it plays a key role in reducing the permeability of the glomerular basement and the vasoreactivity in renal vessels both reducing renal filtration rate (Ratliff et al., 2016). The kidney is normally protected by an upregulation of antioxidant activity, thus the slight (non-significant) increase of ΔBAP/d-ROMs PRE-POST may be considered a protective consequence to the race-related oxidative stress and potential threat to the kidney (Ratliff et al., 2016).
This study has some important limitations. Unfortunately, we did not have the possibility to freeze PLT samples for later analysis without impacting quality of experimental outcomes. Due to the necessity to use of fresh samples and two drop-outs during the race, the sample size of our study is small (N = 8). However, this is the first study evaluating PLT mitochondrial function together with markers of oxidative stress, muscle and kidney injury, thus likely stimulating further research. We were not able to determine PLT counts, protein assay or citrate synthase activity of subsamples to normalize respirometry data per cell concentration or per mitochondrial-specific marker. Although normalization of data per specific mitochondrial flux (FCR, FCF) is common and statistically precise, general limitations of ratios have to be considered (Gnaiger et al., 2019). Moreover, we did not evaluate consequences of changes in PLT mitochondrial function on hemostasis and a potential risk of thrombosis. We refer to the fact that data on indirect markers of potential tissue damage (CK, LDH, BUN and CR) have already been reported in our previous paper (Hoppel et al., 2019). However, as this study focused on alterations in PLT mitochondrial function, we believed it would be valuable to include those data for evaluation of possible associations.
Our data reflect changes in respirometry parameters of PLT mitochondria as a consequence of ultramarathon running. A somewhat more pronounced increase in the relative CI ratio in faster runners may possibly represent PLT CI as an indicator of physical fitness. The higher PLT mitochondrial LEAK PRE and subsequent diminished increase of CK during the race might be interpreted (with caution) as a prophylactic preconditioning and the elevation of the antioxidant potential post-race as a protective consequence of the race-related oxidative stress and potential impact on the kidney. These findings point toward a potential interrelationship between mitochondrial function of PLTs, individual fitness levels and extreme physical and mental stresses. However, since our study population is too small to draw firm conclusions, our results should be merely considered as a valuable perspective for future investigations. Hence, further studies are needed to replicate results in a larger sample of (ultra)marathon runners of both sexes and more homogenous populations in age, BMI and training status to elucidate their clinical/practical implications.
The raw data supporting the conclusions of this article will be made available by the authors, without undue reservation.
Ethical approval was not provided for this study on human participants because for minimal invasive blood sampling the approval by the local ethics review board (University of Innsbruck, Institute of Sport Science) was sufficient. The patients/participants provided their written informed consent to participate in this study.
FH, EC, EG, MB, and WK-R: planning of the project. FH, EC, WK-R, and DP: data acquisition. FH, MB, EC, and DP: drafting of the manuscript. EG, MB, WK-R, EC: project supervision and providing resources. All authors contributed to manuscript revision, read and approved the submitted version.
This study was supported by the project MitoFit funded by the Land Tirol within the program K-Regio of Standortagentur Tirol, and by COST Action CA15203 MitoEAGLE funded by the Horizon 2020 Framework Program of the European Union. University Internationalization Program edition 2015 funded by the University of Verona.
FH was temporarily employed during the project by the company Oroboros Instruments, Innsbruck, Austria. EG is the founder and CEO of Oroboros Instruments.
The remaining authors declare that the research was conducted in the absence of any commercial or financial relationships that could be construed as a potential conflict of interest.
We thank the team of Oroboros Instruments for the support in high-resolution respirometry and the organizers of the Bergmarathon Gmunden for the cooperation.
The Supplementary Material for this article can be found online at: https://www.frontiersin.org/articles/10.3389/fphys.2021.632664/full#supplementary-material
Supplementary Figure 1 | Individual and averaged data of FCR and FCF PRE, POST and REC. Calculated flux control ratios (A) and flux control factors (B), given as single datapoints of each participant and in average. Drop-outs are marked as cross and are not included in statistics. ∗p of post hoc test < 0.05, xadjusted p of ANOVA < 0.05.
Supplementary Figure 2 | Individual data on d-ROMS, BAP, and BAP/d-ROMs before competition (PRE), immediately after the race (POST) and 24 h after finishing (REC). Changes of d-ROMs (A), BAP (B), and BAP/d-ROMs (C). Drop-outs marked as cross. ∗p < 0.05.
Aibibula, M., Naseem, K., and Sturmey, R. (2018). Glucose metabolism and metabolic flexibility in blood platelets. J. Thromb. Haemost. 16, 2300–2314. doi: 10.1111/jth.14274
Alberti, A., Bolognini, L., Macciantelli, D., and Carratelli, M. (2000). The radical caion of N, N-diethyl-para-phenylendiamine: a possible indicator of oxidative stress in biological samples. Res. Chem. Intermed. 26, 253–267. doi: 10.1163/156856700X00769
Ariza, A., Deen, P., and Robben, J. (2012). The succinate receptor as a novel therapeutic target for oxidative and metabolic stress-related conditions. Front. Endocrinol. 3:22. doi: 10.3389/fendo.2012.00022
Braganza, A., Corey, C. G., Santanasto, A. J., Distefano, G., Coen, P. M., Glynn, N. W., et al. (2019). Platelet bioenergetics correlate with muscle energetics and are altered in older adults. JCI Insight. 5:e128248. doi: 10.1172/jci.insight.128248
Brookes, P. (2005). Mitochondrial H(+) leak and ROS generation: an odd couple. Free. Radic. Biol. Med. 38, 12–23. doi: 10.1016/j.freeradbiomed.2004.10.016
Celi, P., Sullivan, M., and Evans, D. (2010). The stability of the reactive oxygen metabolites (d-ROMs) and biological antioxidant potential (BAP) tests on stored horse blood. Vet. J. 183, 217–218. doi: 10.1016/j.tvjl.2008.09.018
Christensen, P., Jacobs, R., Bonne, T., Flück, D., Bangsbo, J., and Lundby, C. (2016). A short period of high-intensity interval training improves skeletal muscle mitochondrial function and pulmonary oxygen uptake kinetics. J. Appl. Physiol. 120, 1319–1327. doi: 10.1152/japplphysiol.00115.2015
Cohen, J. (1988). Statistical Power Analysis for the Behavioral Sciences (Bd. 2nd edition). USA. Lawrence: Erlbaum Assiciates.
Daussin, F., Zoll, J., Ponsot, E., Dufour, S., Doutrele, S., Lonsdorfer, E., et al. (2008). Training at high exercise intensity promotes qualitative adaptations of mitochondrial function in human skeletal muscle. J. Appl. Physiol. 104, 1436–1441. doi: 10.1152/japplphysiol.01135.2007
de Lucas, R., Caputo, F., Mendes, de Souza, K., Sigwalt, A., Ghisoni, K., et al. (2014). Increased platelet oxidative metabolism, blood oxidative stress and neopterin levels after ultra-endurance exercise. J. Sports. Sci. 32, 22–30. doi: 10.1080/02640414.2013.797098
Dröse, S., and Brandt, U. (2008). The Mechanism of Mitochondrial Superoxide Production bythe Cytochrome bc1 Complex. J. Biol. Chem. 283, 21649–21654. doi: 10.1074/jbc.M803236200
Fasching, M., Fontana-Ayoub, M., and Gnaiger, E. (2016). Mitochondrial Respiration Medium - MiR06. Mitochondr. Physiol. Net. 14.13, 1–4.
Fasching, M., and Gnaiger, E. (2018). O2k Quality Control 2: Instrumental oxygen background correction and accuracy of oxygen flux. Mitochondr. Physiol. Network 14.06, 1–16.
Fernström, M., Bakkman, L., Tonkonogi, M., Shabalina, I., Rozhdestvenskaya, Z., Mattsson, C., et al. (2007). Reduced efficiency, but increased fat oxidation, in mitochondria from human skeletal muscle after 24-h ultraendurance exercise. J. Appl. Physiol. 102, 1844–1849. doi: 10.1152/japplphysiol.01173.2006
Gibala, M., MacLean, D., Graham, T., and Saltin, B. (1998). Tricarboxylic acid cycle intermediate pool size and estimated cycle flux in human muscle during exercise. Am. J. Physiol. 272, 235–242. doi: 10.1152/ajpendo.1998.275.2.E235
Gnaiger, E. (2014). “Mitochondrial pathways and respiratory control,” in An Introduction to OXPHOS Analysis. 4th Edn. (Mitochondr Physiol Network), 19.12. Oroboros MiPNet Publications.
Gnaiger, E. (2020). Mitochondrial pathways and respiratory control. An introduction to OXPHOS analysis. Bioenerg. Commun. 2020:0002. doi: 10.26124/bec:2020-0002
Gnaiger, E., Aasander, F. E., Abdul, K. N., Abdel-Rahman, E. A., Abumrad, N. A, Acuna-Castroviejo, D, Adiele, R. C., et al. (2019). Mitochondrial respiratory states and rates. MitoFit Preprint Arch. 2019, 1–40. doi: 10.26124/mitofit:190001.v5
Hattori, N., Hayashi, T., Nakachi, K., Ichikawa, H., Goto, C., Tokudome, Y., et al. (2009). Changes of ROS during a Two-day Ultra-marathon Race. Int. J. Sports. Med. 30, 426–429. doi: 10.1055/s-0028-1112144
He, F., Li, J., Liu, Z., Chuang, C., Yang, W., and Zuo, L. (2016). Redox Mechanism of Reactive Oxygen Species in Exercise. Front. Physiol. 7:486. doi: 10.3389/fphys.2016.00486
Hodgson, L., Walter, E., Venn, R., Galloway, R., and Pitsiladis, V. S. (2017). Acute kidney injury associated with endurance events-is it a cause for concern? A systematic review. BMJ Open Sport Exerc. Med. 3:1. doi: 10.1136/bmjsem-2015-000093
Högberg, C., Gidlöf, O., Tan, C., Svensson, S., Nilsson-Öhman, J., Erlinge, D., et al. (2011). Succinate independently stimulates full platelet activation via cAMP and phosphoinositide 3-kinase-β signaling. J. Thromb. Haemost. 9, 361–372. doi: 10.1111/j.1538-7836.2010.04158.x
Hoppel, F., Calabria, E., Pesta, D., Kantner-Rumplmair, W., Gnaiger, E., and Burtscher, M. (2019). Physiological and Pathophysiological Responses to Ultramarathon Running in Non-elite Runners. Front. Physiol. 10:1300. doi: 10.3389/fphys.2019.01300
Jacobs, R., and Lundby, C. (2013). Mitochondria express enhanced quality as well as quantity in association with aerobic fitness across recreationally active individuals up to elite athletes. J. Appl. Physiol. 114, 344–350. doi: 10.1152/japplphysiol.01081.2012
Jastrzębski, Z., Żychowska, M., Radzimiński, Ł, Konieczna, A., and Kortas, J. (2015). Damage to Liver and Skeletal Muscles in Marathon Runners During a 100 km Run With Regard to Age and Running Speed. J. Hum. Kinet. 45, 93–102. doi: 10.1515/hukin-2015-0010
Knechtle, B., and Nikolaidis, P. (2018). Physiology and Pathophysiology in Ultra-Marathon running. Front. Physiol. 9:634. doi: 10.3389/fphys.2018.00634
Knez, W., Coombes, J., and Jenkins, D. (2006). Ultra-Endurance Exercise and Oxidative Damage. Sports Med. 36, 429–441. doi: 10.2165/00007256-200636050-00005
Kohen, R., and Nyska, A. (2002). Oxidation of biological systems: oxidative stress phenomena, antioxidants, redox reactions, and methods for their quantification. Toxicol. Pathol. 30, 620–650. doi: 10.1080/01926230290166724
Kramer, P., Ravi, S., Chacko, B., Johnson, M., and Darley-Usmar, V. (2014). A review of the mitochondrial and glycolytic metabolism in human platelets and leukocytes: implications for their use as bioenergetic biomarkers. Redox. Biol. 2, 206–210. doi: 10.1016/j.redox.2013.12.026
Lanza, I. R., and Sreekumaran Nair, K. (2010). Regulation of skeletal muscle mitochondrial function: genes to proteins. Acta Physiol. 199, 529–547. doi: 10.1111/j.1748-1716.2010.02124.x
Lee, B., and Shao, J. (2014). Adiponectin and energy homeostasis. Rev. Endocr. Metab. Disord. 15, 149–156. doi: 10.1007/s11154-013-9283-3
Lemieux, H., Blier, P. U., and Gnaiger, E. (2017). Remodeling pathway control of mitochondrial respiratory capacity by temperature in mouse heart: electron flow through the Q-junction in permeabilized fibers. Sci. Rep. 7:2840. doi: 10.1038/s41598-017-02789-8
Lewis, G., Farrell, L., Wood, M., Martinovic, M., Arany, Z., Rowe, G. C., et al. (2010). Metabolic signatures of exercise in human plasma. Sci. Transl. Med. 2, 33–37. doi: 10.1126/scitranslmed.3001006
Liguori, I., Russo, G., Curcio, F., Bulli, G., Aran, L., Della-Morte, D., et al. (2018). Oxidative stress, aging, and diseases. Clin. Interv. Aging. 13, 757–772. doi: 10.2147/CIA.S158513
Lopez, J. S.-A., and Rosado, J. P. (2007). Thrombin induces apoptotic events through the generation of reactive oxygen species in human platelets. J. Thromb. Haemost. 5, 1283–1291. doi: 10.1111/j.1538-7836.2007.02505.x
Ma, R., Chaudhari, S., and Li, W. (2016). Canonical Transient Receptor Potential 6 Channel: A New Target of Reactive Oxygen Species in Renal Physiology and Pathology. Antioxid. Redox. Signal. 25, 732–748. doi: 10.1089/ars.2016.6661
Mastaloudis, A., Morrow, J., Hopkins, D., Devaraj, S., and Traber, M. (2004). Antioxidant supplementation prevents exercise-induced lipid peroxidation, but not inflammation, in ultramarathon runners. Free Radic. Biol. Med 36, 1329–1341. doi: 10.1016/j.freeradbiomed.2004.02.069
Mastaloudis, A., Traber, M. G., Carstensen, K., and Widrick, J. J. (2006). Antioxidants did not prevent muscle damage in response to an ultramarathon run. Med. Sci. Sports Exerc. 38, 72–80. doi: 10.1249/01.mss.0000188579.36272.f6
Melchinger, H., Jain, K., Tyagi, T., and Hwa, J. (2019). Role of Platelet Mitochondria: Life in in a Nucleus-Free Zone. Front. Cardiovasc. Med. 6:153. doi: 10.3389/fcvm.2019.00153
Meyer, L., Machado, L., Santiago, A., da-Silva, W., De Felice, F., Holub, O., et al. (2006). Mitochondrial creatine kinase activity prevents reactive oxygen species generation antioxidant role of mitochondrial kinase-dependent ADP re-cycling activity. J. Biol. Chem. 281, 37361–37371. doi: 10.1074/jbc.M604123200
Millet, G., and Millet, G. (2012). Ultramarathon is an outstanding model for the study of adaptive responses to extreme load and stress. BMC Med. 10:77. doi: 10.1186/1741-7015-10-77
Mrakic-Sposta, S., Gussoni, M., Moretti, S., Pratali, L., Giardini, G., Tacchini, P., et al. (2015). Effects of Mountain Ultra-Marathon Running on ROS Production and Oxidative Damage by Micro-Invasive Analytic Techniques. PLoS One. 10:11. doi: 10.1371/journal.pone.0141780
Niemann, D. H., Mc Anulty, S., McAnulty, L., Swick, N., Utter, A., Vinci, D. M., et al. (2002). Influence of vitamin C supplementation on oxidative and immune changes after an ultramarathon. J. Appl. Physiol. 92, 1970–1977. doi: 10.1152/japplphysiol.00961.2001
Nikolaidis, M., Kyparos, A., Spanou, C. P., Theodorou, A., and Vrabas, I. (2012). Redox biology of exercise: an integrative and comparative consideration of some overlooked issues. J. Exp. Biol. 215, 1615–1625. doi: 10.1242/jeb.067470
Pedersen, B., and Hoffman-Goetz, L. (2000). Exercise and the immune system: regulation, integration, and adaptation. Physiol. Rev. 80, 1055–1081. doi: 10.1152/physrev.2000.80.3.1055
Pesta, D., Hoppel, F., Macek, C., Messner, H., Faulhaber, M., Kobel, C., et al. (2011). Similar qualitative and quantitative changes of mitochondrial respiration following strength and endurance training in normoxia and hypoxia in sedentary humans. Am. J. Physiol. Regul. Integr. Comp. Physiol. 301, 1078–1087. doi: 10.1152/ajpregu.00285.2011
Porter, C., Reidy, P., Bhattarai, N., Sidossis, L., and Rasmussen, B. (2015). Resistance Exercise Training Alters Mitochondrial Function in Human Skeletal Muscle. Med. Sci. Sports. Exerc. 47, 1922–1931. doi: 10.1249/MSS.0000000000000605
Posthuma, J. J., van der Meijden, P. E. J., Ten Cate, H., and Spronk, H. M. H. (2015). Short- and Long-term exercise induced alterations in haemostasis: a review of the literature. Blood Rev. 29, 171–178. doi: 10.1016/j.blre.2014.10.005
Ratliff, B., Abdulmahdi, W., Pawar, R., and Wolin, M. S. (2016). Oxidant Mechanisms in Renal Injury and Disease. Antioxid. Redox. Signal. 25, 119–146. doi: 10.1089/ars.2016.6665
Rose, S., Carvalho, E., Diaz, E. C., Cotter, M., Bennuri, S. C., Azhar, G., et al. (2019). A comparative study of mitochondrial respiration in circulating blood cells and skeletal muscle fibers in women. Am. J. Physiol. Endocrinol. Metab. 317, E503–E512. doi: 10.1152/ajpendo.00084.2019
Sahlin, K., Shabalina, I., Mattsson, C., Bakkman, L., Fernström, M., Rozhdestvenskaya, Z., et al. (2010). Ultraendurance exercise increases the production of reactive oxygen species in isolated mitochondria from human skeletal muscle. J. Appl. Physiol. 108, 780–787. doi: 10.1152/japplphysiol.00966.2009
Sjoevall, F., Ehinger, J., Marelsson, S., Morota, S., Asander-Frostner, E., Uchino, H., et al. (2013). Mitochondrial respiration in human viable platelets—Methodology and influence of gender, age and storage. Mitochondrion 13, 7–14. doi: 10.1016/j.mito.2012.11.001
Sumbalová, Z., Garcia-Souza, L. F., Veliká, B., Volani, C., and Gnaiger, E. (2018). “Analysis of mitochondrial function in human blood cells,” in Recent advances in mitochondrial medicine and Coenzyme Q10, eds A. Gvozdjáková, G. Cornelissen, and R. B. Singh (New York, NY: Nova Sciences), 255–260.
Taeb, A., Regn, D., and Sill, J. M. (2017). Running Ourselves to Death: Does Long Distance Running Increase the Risk of Venous Thromboembolism? Am. J. Respir. Crit. Care Med. 195:A6189.
Tyrrell, D., Bharadwaj, M., Jorgensen, M., Register, T., and Molina, A. (2016). Blood cell respirometry is associated with skeletal and cardiac muscle bioenergetics: Implications for a minimally invasive biomarker of mitochondrial health. Redox Biol. 10, 65–77. doi: 10.1016/j.redox.2016.09.009
Votion, D., Gnaiger, E., Lemieux, H., Mouithys-Mickalad, A., and Serteyn, D. (2012). Physical Fitness and Mitochondrial Respiratory Capacity in Horse Skeletal Muscle. PLoS One 7:4. doi: 10.1371/journal.pone.0034890
Walsh, B., Tonkonogi, M., and Sahlin, K. (2001). Effect of endurance training on oxidative and antioxidative function in human permeabilized muscle fibres. Pflugers Arch. 442, 420–425. doi: 10.1007/s004240100538
Yamagishi, S., Edelstein, D., Du, X., and Brownlee, M. (2001). Hyperglycemia potentiates collagen-induced platelet activation through mitochondrial superoxide overproduction. Diabetes 506, 1491–1494. doi: 10.2337/diabetes.50.6.1491
Zaleski, A., Taylor, B., Pescatello, L., and Thompson, P. (2016). Marathon Maladies: Venous Thromboembolism Risk Associated with Marathon Running. J. Clin. Exerc. Physiol. 5, 1–5. doi: 10.31189/2165-6193-5.1.1
Keywords: mitochondrial function, platelets, ultramarathon running, acute kidney injury, muscle damage
Citation: Hoppel F, Calabria E, Pesta DH, Kantner-Rumplmair W, Gnaiger E and Burtscher M (2021) Effects of Ultramarathon Running on Mitochondrial Function of Platelets and Oxidative Stress Parameters: A Pilot Study. Front. Physiol. 12:632664. doi: 10.3389/fphys.2021.632664
Received: 24 November 2020; Accepted: 05 January 2021;
Published: 28 January 2021.
Edited by:
Vincent Pialoux, Université Claude Bernard Lyon 1, FranceReviewed by:
Carlos Hernando Domingo, University of Jaume I, SpainCopyright © 2021 Hoppel, Calabria, Pesta, Kantner-Rumplmair, Gnaiger and Burtscher. This is an open-access article distributed under the terms of the Creative Commons Attribution License (CC BY). The use, distribution or reproduction in other forums is permitted, provided the original author(s) and the copyright owner(s) are credited and that the original publication in this journal is cited, in accordance with accepted academic practice. No use, distribution or reproduction is permitted which does not comply with these terms.
*Correspondence: Florian Hoppel, RmxvLmhvcHBlbEBnbWFpbC5jb20=; Martin Burtscher, TWFydGluLmJ1cnRzY2hlckB1aWJrLmFjLmF0
Disclaimer: All claims expressed in this article are solely those of the authors and do not necessarily represent those of their affiliated organizations, or those of the publisher, the editors and the reviewers. Any product that may be evaluated in this article or claim that may be made by its manufacturer is not guaranteed or endorsed by the publisher.
Research integrity at Frontiers
Learn more about the work of our research integrity team to safeguard the quality of each article we publish.