- 1Escuela de Kinesiología, Facultad de Salud, Universidad Católica Silva Henríquez, Santiago, Chile
- 2Centro de Investigación en Fisiología del Ejercicio, Facultad de Ciencias, Universidad Mayor, Santiago, Chile
- 3Navarrabiomed, Complejo Hospitalario de Navarra (CHN), Universidad Pública de Navarra, IdiSNA, Pamplona, Spain
- 4Unidad de Estadística, Departamento de Calidad, Clínica Santa María, Santiago, Chile
- 5Laboratory of Human Performance, Quality of Life and Wellness Research Group, Department of Physical Activity Sciences, Universidad de Los Lagos, Osorno, Chile
- 6Institute of Sport Sciences, University of Lausanne, Lausanne, Switzerland
- 7Laboratory of Cardiorespiratory Control, Department of Physiology, Pontificia Universidad Católica de Chile, Santiago, Chile
- 8Centro de Envejecimiento y Regeneración (CARE), Pontificia Universidad Católica de Chile, Santiago, Chile
- 9Centro de Excelencia en Biomedicina de Magallanes (CEBIMA), Universidad de Magallanes, Punta Arenas, Chile
- 10Centro de Fisiología y Medicina de Altura, Facultad de Ciencias de la Salud, Universidad de Antofagasta, Antofagasta, Chile
During an apnea, changes in PaO2 activate peripheral chemoreceptors to increase respiratory drive. Athletes with continuous apnea, such as breath-hold divers, have shown a decrease in hypoxic ventilatory response (HVR), which could explain the long apnea times; however, this has not been studied in swimmers. We hypothesize that the long periods of voluntary apnea in swimmers is related to a decreased HVR. Therefore, we sought to determine the HVR and cardiovascular adjustments during a maximum voluntary apnea in young-trained swimmers. In fifteen trained swimmers and twenty-seven controls we studied minute ventilation (VE), arterial saturation (SpO2), heart rate (HR), and autonomic response [through heart rate variability (HRV) analysis], during acute chemoreflex activation (five inhalations of pure N2) and maximum voluntary apnea test. In apnea tests, the maximum voluntary apnea time and the end-apnea HR were higher in swimmers than in controls (p < 0.05), as well as a higher low frequency component of HRV (p < 0.05), than controls. Swimmers showed lower HVR than controls (p < 0.01) without differences in cardiac hypoxic response (CHR). We conclude that swimmers had a reduced HVR response and greater maximal voluntary apnea duration, probably due to decreased HVR.
Introduction
Water sports are part of Olympic Games and have several modalities, including sailing, apnea dives, swimming, among others (FINA, 2020). Swimmers and apnea divers are characterized by the ability to modulate their breathing to such a point that ventilation can be interrupted by ∼4 min (Heusser et al., 2009). Despite that the end of an apnea is related to the central command of the ventilation (Guyenet and Bayliss, 2015), most of the evidence related to regulatory responses to apnea are focused on cardiovascular responses, which has been linked to the sports performance (Costill et al., 1991; Rodríguez-Zamora et al., 2012; Guimard et al., 2014; Costa et al., 2015; Viana et al., 2019). However, considering that PaO2 decreases and PaCO2 increases during apneas (Muth et al., 2003) and, that respiratory regions, at the brainstem level, could modulate the cardiovascular autonomic responses (Andrade et al., 2018; Díaz et al., 2020), the longer duration of apnea and cardiovascular adjustments to the apnea observed in swimmers, may be related to neural control of breathing, through a desensitization of peripheral chemoreceptors to hypoxia and hypercapnia. However, it has not been studied yet in swimmers.
Most water sports involve immersion periods with both, intermittent (i.e., swimming) and prolonged apneas (i.e., breath-hold dive or speed swimming), and these can last from seconds to minutes depending on discipline (Craig et al., 1985). In fact, one of the most important physiological adaptations, that help improve the athletes’ performances, is the increase of apnea time (Shei, 2018). During a breath-hold there is a decrease in PaO2 and increase in PaCO2, which could stimulate peripheral and central chemoreceptors (Dempsey et al., 2012; Kumar and Prabhakar, 2012; Guyenet and Bayliss, 2015), suggesting that the end of voluntary apnea is related to the activation of these reflex arcs. The principal peripheral chemoreceptors are allocated in the carotid body (CB), which propagates information to the brainstem in response to several stimuli (PaO2, PaCO2, pH, flow, glucose, among others) (Iturriaga and Alcayaga, 2004; Kumar and Prabhakar, 2012; Del Rio et al., 2017). The increase in CB chemoreceptors activity elicits an increase in minute ventilation (VE), sympathetic activity, arterial blood pressure (BP), and heart rate (HR) to cope with metabolic demands (Iturriaga et al., 2016). Therefore, as swimmers are subjected to intermittent apneas during training session, it is possible that the apnea-dependent repetitive hypoxic-hypercapnic stimuli could induce a depressed respiratory neuroplasticity (Vermeulen et al., 2020) and therefore impact on peripheral chemoreflex drive and possibly on apnea time.
Thus, considering that elite apnea divers displayed longer apnea duration (Heusser et al., 2009), it is possible that in swimmers, during a breath-hold the peripheral chemoreceptors could be desensitized to hypoxia and hypercapnia, delaying the end of the apnea, contributing to a prolonged maximum voluntary apnea duration (Lemaître et al., 2009). Thus, we sought to determine the maximum voluntary apnea duration and hypoxic ventilatory response (HVR), on top of the cardiovascular and autonomic adjustments during the breath-hold and during the hypoxic challenge in young-trained swimmers compared to control participants. We hypothesized that sub-aquatic training induces an adaptive phenomenon of peripheral chemoreflex desensitization, decreasing its response in apnea-induced hypoxia/hypercapnia allowing swimmers to have longer apnea times.
Materials and Methods
Ethics Statements
Protocols were approved by the Ethical Committee of the Universidad Mayor (Approval number #169_2019) and were performed according to the standards set by the latest version of the Declaration of Helsinki. Participants were carefully informed about the experimental procedures, and the possible risks and benefits associated with their participation in the study. Thereafter, written informed assent and consent were obtained from the parents of under-age athletes and from adult athletes, respectively.
Subjects
Fifteen young trained regional- to national-level competitive swimmers [eight males and seven females; age, 20.93 ± 5.18 years; height, 169.53 ± 10.44 cm; body mass, 71.5 ± 12.77 kg; body mass index (BMI), 24.7 ± 2.05 kg/m2], with 5–12 years of swimming training, and a mean weekly training volume of ∼4 h per day, five times per week, participated in this study. Twenty-seven controls (22 males and five females; age, 17.22 ± 2.42 years; height, 169.52 ± 8.12 cm; body mass, 63.85 ± 10.3 kg; BMI, 22.12 ± 2.49 kg/m2) also volunteered to participate in this study. All females were assessed (by a female technician) during the early follicular phase of their menstrual cycle. Experiments were conducted between 08:00 and 17:00 h. Forty-eight hours before experiments participants, were asked to avoid consumption of alcohol, cigarettes, caffeine, or drugs that may alter autonomic control. None of the participants were taking any medication or had a personal or family history of any cardiac, ventilatory or endocrine disorder.
Experimental Design
A descriptive cross-sectional study was performed to determine HVR and autonomic response to hypoxia and maximum voluntary apnea in young highly trained swimmers compared to controls. The inclusion criteria were: (i) high-performance swimmers with less than 12 years of training; (ii) from national or university teams, active participants in national or international competitions; and a minimum of 20 h of training per week. Exclusion criteria were: (i) potential medical problems or history of cardiorespiratory diseases; (ii) any cardiovascular or respiratory surgery in the past 2 years; (iii) autonomic control impairment at rest, estimated by heart rate variability (HRV) disturbances (low to high frequency ratio of HRV < 2.3) (Nunan et al., 2010); (iv) being in the course of an acute illness or consumption of any drug or pharmacological ergogenic aid and (v) history of chronic obstructive or restrictive pulmonary diseases and/or altered spirometry on the day of the testing session, including (vi) forced expiratory volume at first second (FEV1)/vital capacity (VC) < 70, (vii) FEV1 < 80% of predicted value, or (viii) VC < 80% of predicted value (see Supplementary Table 1).
On the first day, body mass, height, HR, clinical spirometry [VC; peak expiratory flow (PEF); FEV1; FEV1/VC; forced expiratory flow at 25% (FEF 25), 50% (FEF 50), and 75% (FEF 75) of VC] and resting metabolic ratio were measured (Figure 1). Body mass was estimated to the nearest 0.1 kg using a digital scale (BF-350, Tanita, IL, United States). Height was measured using a wall-mounted stadiometer (HR-200, Tanita, Japan) and recorded to the nearest 0.1 cm. The BMI was calculated as kg/m2.
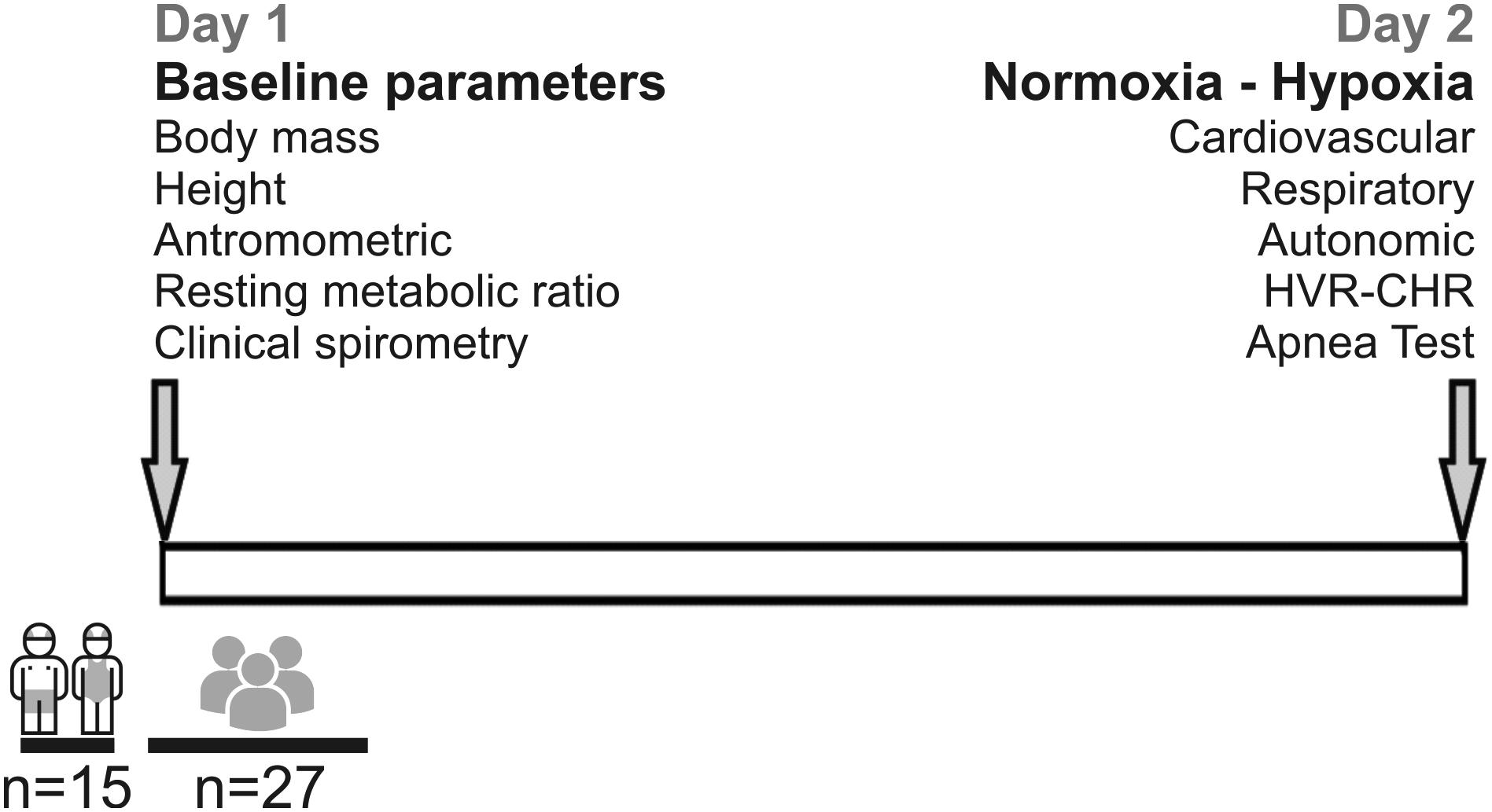
Figure 1. Timeline and design of the experiment. Fifteen young swimmers and twenty-seven control subjects were enrolled to participate in this study. Measurements were performed in 2 days. At day 1, baseline parameters (height, weight, anthropometric, resting metabolic rate, and clinical spirometry were performed. At day 2, respiratory, autonomic, cardiovascular parameters, hypoxic ventilatory response (HVR) and cardiac hypoxic response (CHR), and a maximum apnea duration test were estimated.
On the second day, the participants were instrumented and positioned in the supine position, at an ambient temperature of ∼22°C. Instrumentation includes: 3-leads electrocardiogram (ECG), core temperature regulator, oxygen saturation using a pulse oximeter measured on the index or thumb finger (SpO2) (BK-PO2, BIOBASE, China) and an orofacial mask (Hans Rudolph3, 3700A, Kansas City, MO, United States) connected to a gas mixing chamber to measure airflow and expired gases. From respiratory flow, tidal volume (VT) was calculated (FE141, ADInstruments Inc., New Zealand). The expired fraction of end-tidal carbon dioxide (FECO2), end-tidal oxygen (FEO2) levels, and fraction inspired of O2 (FIO2) were measured with CO2 and O2 gas analyzers (ML206, ADInstruments Inc., New Zealand). After instrumenting the subjects, they were given a 15-min rest period in supine position before the recording started. After this period, baseline parameters were recorded for 20 min. In addition, they were instrumented with eye mask and headphones to reduce external noise (MPA 101, Masprot, Chile) to blunt the effect of manipulation of gases on participants’ arousal. Ventilatory data, pulse oximeter, and gas exchanges were digitized using PowerLab Data Acquisition System (PowerLab, 16SP, ADInstruments Inc., New Zealand) and analyzed with LabChart 8.0 (ADInstruments Inc., New Zealand). All experiments were performed according to the timeline shown in Figure 1.
Hypoxic Ventilatory Response (HVR) and Maximum Voluntary Apnea Test
Hypoxic ventilatory response was evaluated by a poikilocapnic transient hypoxic challenge similar to the one previously described (Pfoh et al., 2016). Participants underwent three consecutive trials (each trial was separate by 5 min) that consisted of five-breaths of 100% N2. N2 was blended into a port on the mask through N2 tubing. After the application of N2, 15 min elapsed until ventilatory parameters returned to baseline levels. Afterward, the subjects changed from a supine to a sitting position, and they were instructed to perform a maximum voluntary apnea (after a maximum inspiration). This experiment was performed with a PowerLab Data Acquisition System (PowerLab, 16SP, ADInstruments Inc., New Zealand).
Cardiac Hypoxic Response (CHR)
Cardiac hypoxic response was assessed by the same stimulus of HVR, similar as in previous experiments (Richalet et al., 2012; Bourdillon et al., 2014). Briefly, three transient tests of five-breaths at 100% N2 were applied with 5 min of rest between trials. The highest HR response from these trials was used. To determine the CHR, 1 min of rest in normoxia and 1 min of maximum HR response in hypoxia were used for the analysis. Thus, CHR was calculated as follows: resting HR – maximum HR/SpO2 in normoxia – SpO2 in hypoxia (= ΔHR/ΔSpO2) (Richalet et al., 2012; Bourdillon et al., 2014).
Electrocardiogram (ECG)
The 3-leads ECG was recorded using a bio-amplifier connected to a digital recording system (PowerLab, 16SP, ADInstruments Inc., New Zealand). The electrodes (3M, Saint Paul, MN, United States) were placed in second derivative (DII) from Einthoven triangle with participants in a supine position. The ECG was recorded continuously, along with breathing gases and ventilation in all experiments considering peripheral chemoreflex test and maximum voluntary apnea test. The sampling frequency was set at 4 kHz and was amplified x100. The ECG analysis was performed with LabChart software v.8 (ADInstruments Inc., New Zealand).
Heart Rate Variability (HRV)
The HRV was assessed as an indirect measure of autonomic balance of the heart (Camm, 1996). From the 3-lead ECG recording, time series were obtained from R-R interval and a time-varying spectrogram was used to obtain the power spectral density (PSD) of HRV (2 s resolution, Tarvainen et al., 2014). Cut-off frequencies were defined as very low frequency [Very low frequency (VLFHRV; 0.00–0.04 Hz), low frequency (LFHRV; 0.04–0.10 Hz), and high frequency (HFHRV; 0.10–0.40 Hz) (Yuda et al., 2018)]. Additionally, we used the LF/HFHRV ratio as an indicator of global autonomic balance of the heart. The LFHRV and HFHRV were expressed as normalized units (n.u.), calculated as follow: LF n.u. = LF power/(total power – VLF); and HF n.u. = HF power/(total power – VLF) (Camm, 1996); thereafter the area under curve (AUC) of the total responses was calculated, as previously described (Andrade et al., 2020). The baseline values and the maximum apnea event were analyzed using Kubios HRV Premium Software v3.1 (Kubios, Kuopio, Finland).
Resting Metabolic Rate
Resting metabolic rate (RMR) was measured by indirect calorimetry as previously described (Speakman and Selman, 2003). All participants were instructed to minimize movement after waking-up and to avoid vigorous exercise before the implementation of the calorimetry (Speakman and Selman, 2003; Compher et al., 2006; Nieman et al., 2006). Participants underwent RMR evaluation between 8:00 and 10:00 am. During RMR measurement participants breathed through an oronasal mask (7450 Series Silicone V2, Hans Rudolph, Kansas City, MO, United States) for expired gas collection and analysis (Quark CPET metabolic cart; COSMED, Rome, Italy). Every three measurements the metabolic cart was re-calibrated with a known calibration gas (O2 15%, CO2 5%, N2 balanced) (Nieman et al., 2013). The RMR measurement was performed in a specially conditioned room isolated from noise, at a temperature of 23°C and 50% of humidity. Before the measurement, the participants rested for 30 min. The subjects were instrumented and placed in a supine position during the 40 min of measurements. From the total recording, the first 5-min were discarded as part of the acclimatization period. The calculation of respiratory quotient (RQ), protein, carbohydrates and lipids oxidation were obtained from the remaining 35 min. Protein, carbohydrates and lipids oxidation, were expressed as kcal/day and as % of total resting metabolic rate. The recording and analysis were performed with OMNIA, Cardiopulmonary Diagnostic Suite v 1.4 (Quark CPET metabolic cart; COSMED, Rome, Italy).
Pulmonary Function
Pulmonary function was assessed according to the American Thoracic Society and the European Respiratory Society Task Force consensus (Miller et al., 2005). Briefly, all participants were asked to exert maximum effort during forced breathing. For VT subjects were asked to perform a maximal inspiration (inspiratory reserve volume), return to tidal volume, and then perform a maximal expiration (expiratory reserve volume). We used the maximal expiratory curve to calculate VC, PEF, PIF, FEV1, FEV1/VC, FEF 25, FEF 50, and FEF 75. Each subject was asked to do three attempts, from which the best maximal expiratory curve was used for analysis (Andrade et al., 2020). The recording and analysis were performed with OMNIA, Cardiopulmonary Diagnostic Suite v 1.4 (Quark CPET metabolic cart; COSMED, Rome, Italy).
Statistical Analysis
Data was expressed as mean ± standard deviation (SD). Normality of the data was assessed using Shapiro Wilk test and the homoscedasticity of the variance was determined by Levene’s test. Differences between groups were assessed using unpaired T-tests for variables with normal distribution and Mann–Whitney test for variables with non-normal distribution. For the analysis between normoxia and hypoxia the data between both groups was analyzed using a two-way ANOVA followed by the Holm–Sidak post hoc test (GraphPad Prism software Inc., version 8.0, La Jolla, CA, United States). Significant differences were set at p < 0.05.
Results
Baseline Cardiorespiratory and Pulmonary Parameters
At baseline, demographic, respiratory, cardiovascular, and metabolic variables were not different between swimmers and controls, with the exception of inspiratory time (Ti) (Table 1 and Supplementary Table 1). Indeed, swimmers showed greater Ti compared to controls (1.97 ± 0.89 vs. 1.73 ± 0.25 s, p < 0.05) (Table 1). Regarding pulmonary function, swimmers and control participants did not show significant differences (Supplementary Table 1).
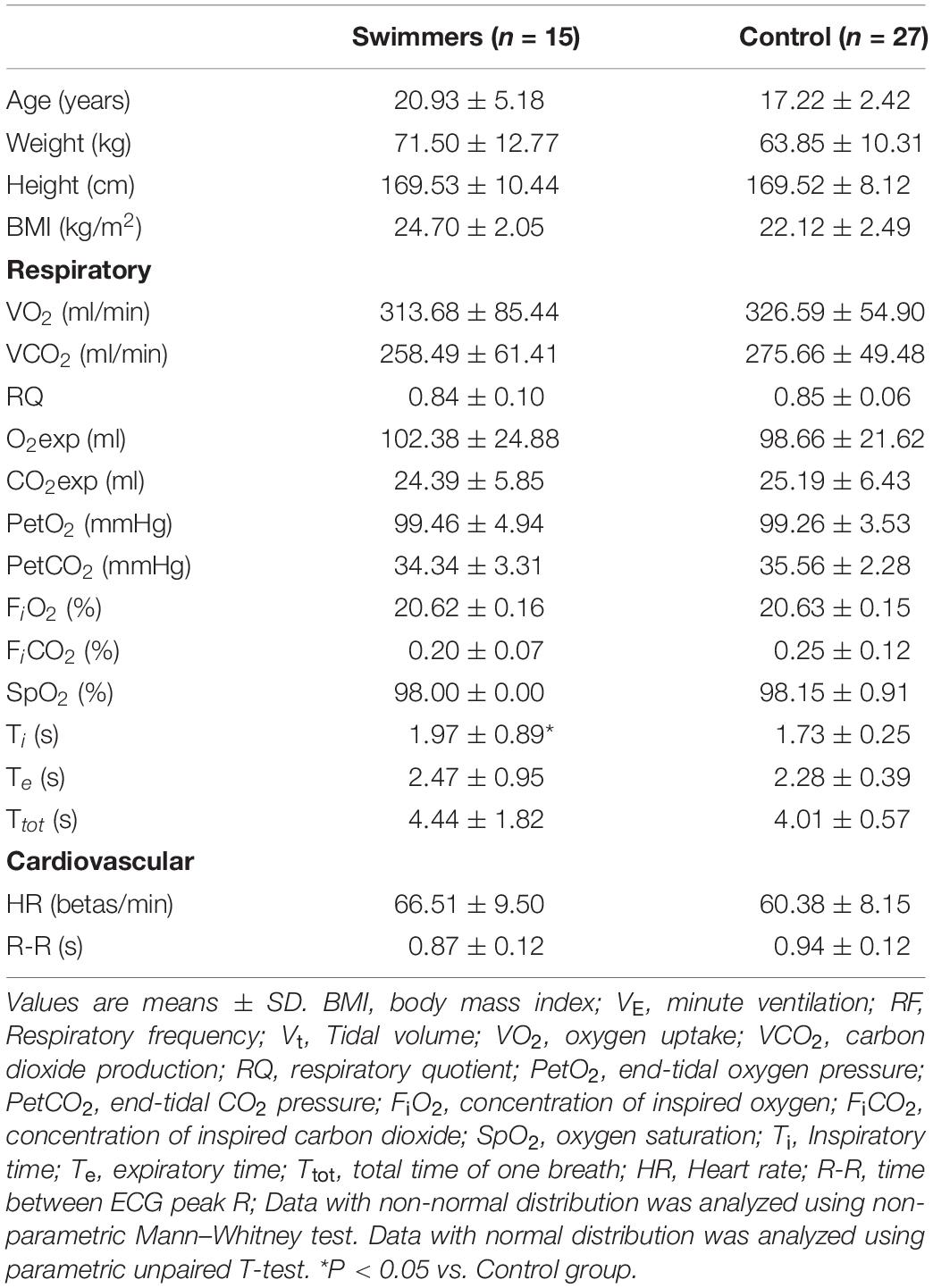
Table 1. Basal anthropometric, respiratory, and cardiovascular characteristics of swimmers compared to control participants at rest condition.
Maximum Voluntary Apnea Time and Cardiovascular Response to Hypoxia and Apnea
The maximum voluntary apnea duration was higher in swimmers than in control participants (83.18 ± 41.43 vs. 55.77 ± 23.71 s) (Figures 2A,B). The HR response during the apnea was higher in swimmers compared to the controls (HR: 71.99 ± 7.67 vs. 63.20 ± 10.07 beats/min) (Figure 2C). In addition, the maximum HR response to voluntary apnea was higher in swimmers compared to controls (ΔHR: 2.94 ± 7.88 vs. −2.20 ± 7.86 Δbeats/min) (Figure 2D). Which showed that the swimmers displayed the classical adaptation to their training (Shei, 2018).
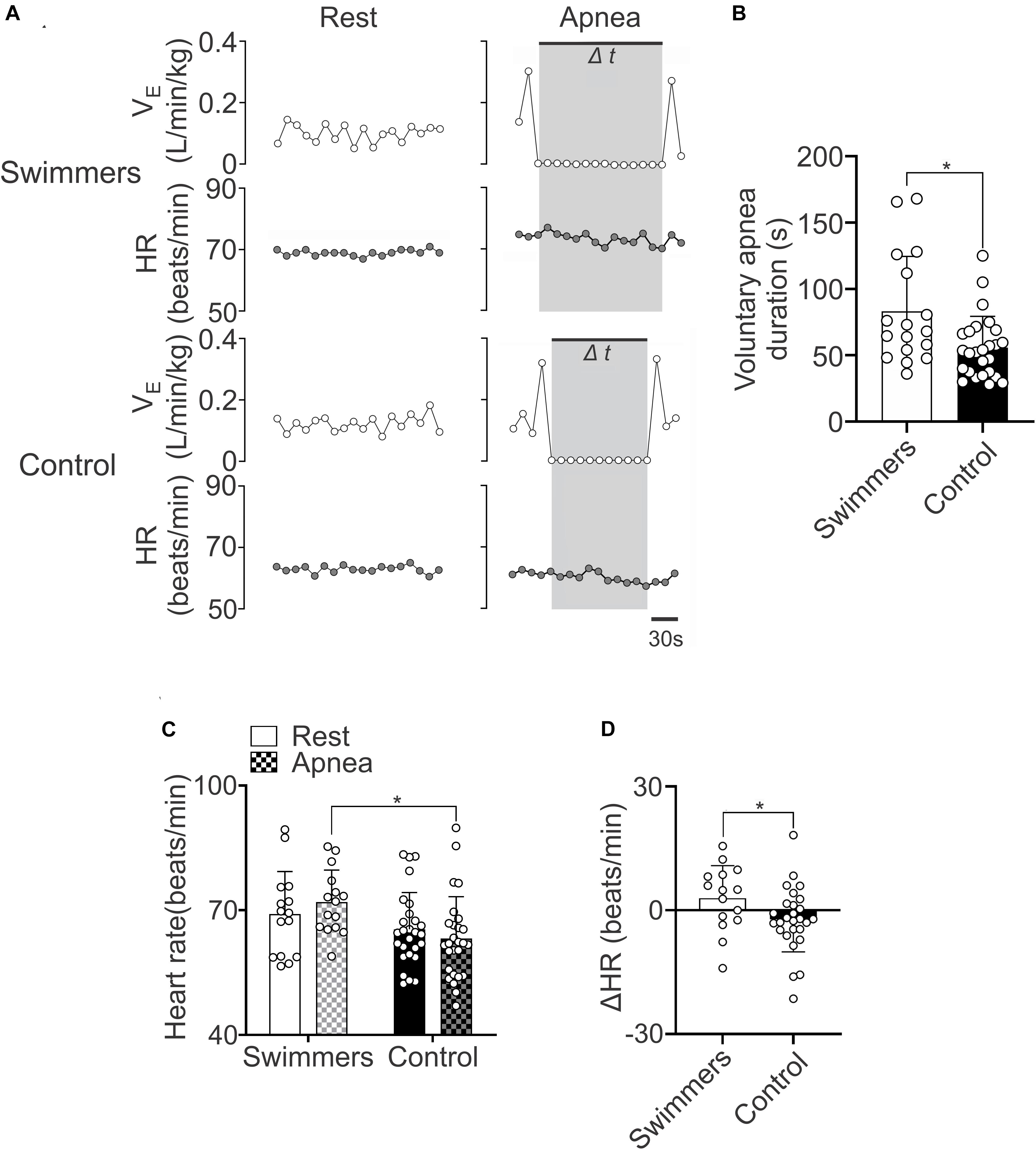
Figure 2. Swimmers are able to maintain longer apnea time and a higher heart rate response during the apnea effort. (A) Representative data of VE and HR of one swimmer and one control participant during rest and maximal voluntary apnea effort. Note that swimmers displayed higher HR responses than controls during apnea test. (B) Summary data of maximal apnea duration. Note that swimmer athletes displayed a marked longer apnea duration compared to control participants. (C,D) Summary results of HR and ΔHR during rest and apnea effort. Note that swimmer athletes displayed a more pronounced positive chronotropic response to a maximum voluntary apnea effort. Two-way ANOVA with repeated measures followed by Holm–Sidak post hoc test for (C) and unpaired T-test for (B,D). Values are mean ± SD, Swimmers n = 15, Control N = 27. *p < 0.05 compared to Swimmers.
Autonomic Control During Maximum Voluntary Apnea Test
During the maximum voluntary apnea test, the LFHRV in swimmers was increased compared to the resting condition (Figures 3A–C). However, the control participants did not show significant changes in LFHRV, between rest and the apnea test (p = 0.424) (Figure 3C). The ΔLFHRV was higher in swimmers than in controls (999.2 ± 1368 vs. 140.4 ± 1194 ΔAUC, p = 0.033) (Figure 3C1). Regarding to HFHRV during apnea, both groups showed a decrease of parasympathetic drive (p < 0.001), without differences with their respective resting values (p = 0.224) (Figures 3A,D,E,E1). Both groups showed an increase in LF/HF from rest to apnea, (swimmers: 0.23 ± 0.18 to 0.54 ± 0.44, p < 0.001; controls: 0.23 ± 0.22 to 0.37 ± 0.41, p = 0.037). The magnitude of the LF/HF increase was not larger in swimmers than in controls (0.31 ± 0.35 vs. 0.12 ± 0.32; p = 0.087) (Figures 3F,G,G1).
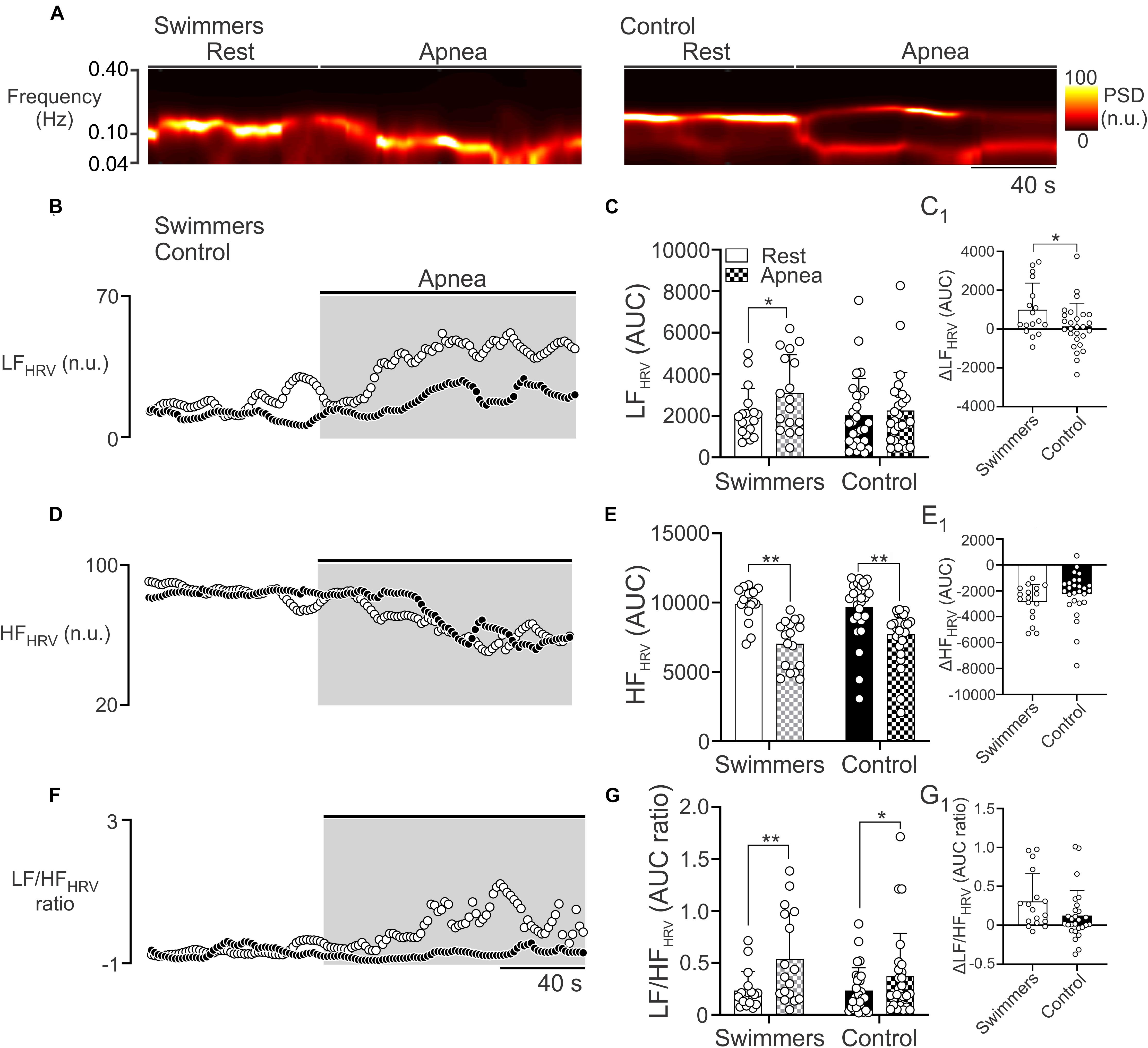
Figure 3. Autonomic control during the maximum voluntary apnea test in swimmers and control participants. (A) Representative time-varying domain spectrum of heart rate variability (HRV) during rest and during the maximal voluntary apnea. Note that swimmers athletes displayed a large increase in the low frequency component of HRV (LFHRV, 0.04–0.10 Hz), concomitant to a decrease of high frequency component of HRV (HFHRV, 0.10–0.40 Hz) following the apnea effort. (B,D,F) Summary data of power spectral density (PSD) of non-stationary analysis of HR with 2-s resolution (time varying domain of HR variability) during the apnea test to LFHRV, HFHRV, and LF/HFHRV ratio, respectively. (C,E,G) Summary data of LFHRV, HFHRV, and LF/HFHRV ratio, respectively, of HRV non-stationary analysis. (C1,E1,G1) Summary data of ΔLFHRV, ΔHFHRV, and ΔLF/HFHRV ratio, respectively. Two-way ANOVA with repeated measures followed by Holm–Sidak post hoc test for (C,E,G); unpaired T-test for (C1,E1,G1). Values are mean ± SD, Swimmers n = 15, Control N = 27. *p < 0.05; **p < 0.01.
Ventilatory and Cardiac Response to Hypoxia
The HVR and HCR responses are shown in Figure 4 and Table 2. In normoxia there were no differences between swimmers and controls in SpO2 (98.0 ± 0.0 vs. 98.1 ± 0.9%) (Figures 4B,B1 and Table 2) and VE (0.11 ± 0.04 vs. 0.14 ± 0.04 L/min/kg) (Figures 4C,C1). In addition, before chemoreflex test (during normoxia) RF and VT weren’t different between groups (Table 2).
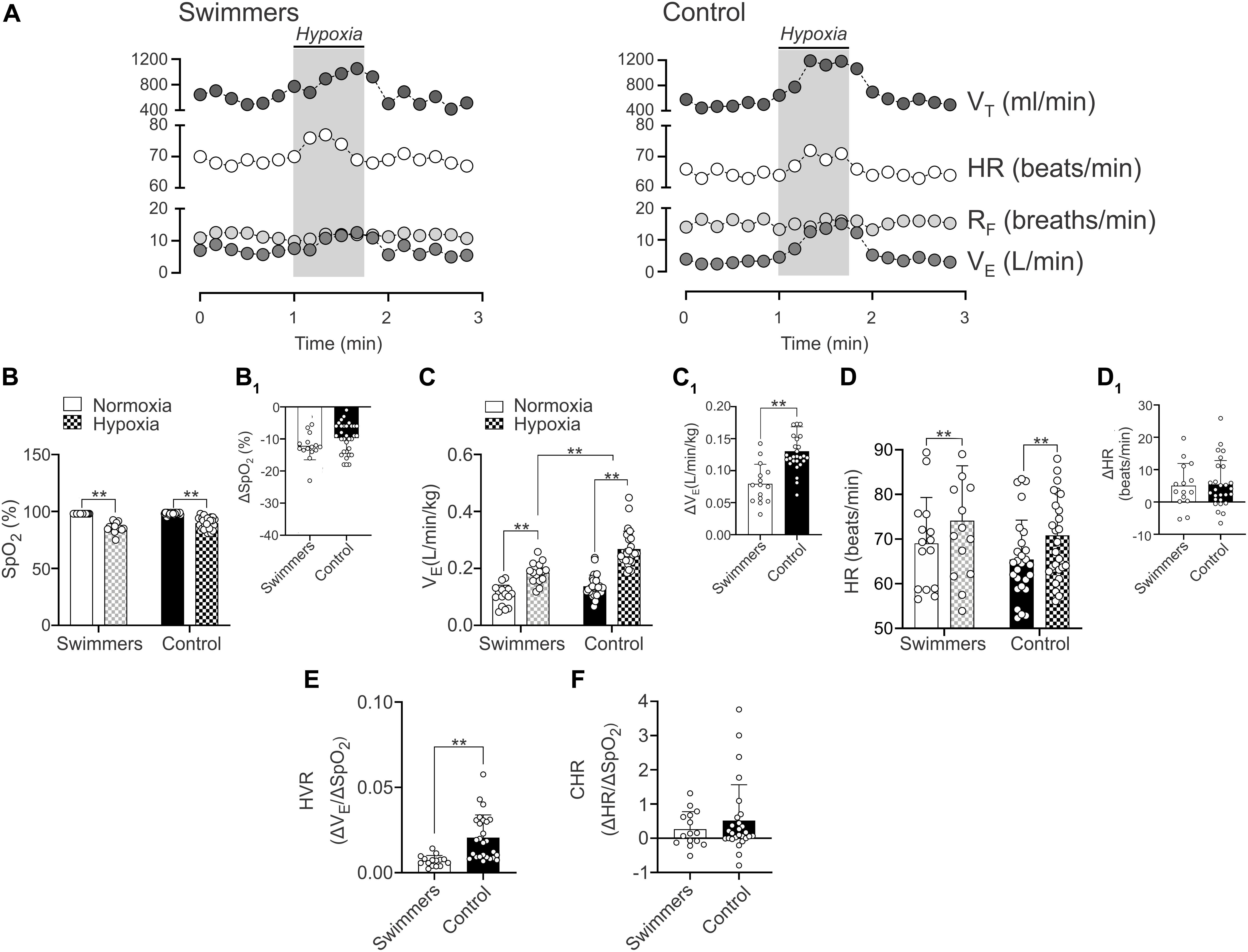
Figure 4. Hypoxic ventilatory (HVR) cardiac (HCR) responses in young trained swimmers. (A,B) Representative data of VT, HR, RF, and VE of one Swimmer and Control participant during a hypoxic challenge (pure N2). Note that swimmers displayed a decrease VE response compared to the control participants. (B,B1) Summary of SpO2 changes in normoxia and hypoxia and summary of ΔSpO2 between normoxia and hypoxia in both groups, respectively. (C) Summary of VE responses to hypoxia of both groups. Note that the breathing response was minor in swimmers compared to the control participants. (C1) Quantification of ΔVE between normoxia and hypoxia in both groups. (D) Summary of HR responses to hypoxia of both groups. (D1) Quantification of ΔHR between normoxia and hypoxia in both groups. (E) Swimmers group showed a significantly lower HVR compared to controls. (F) Summary data of CHR. Two-way ANOVA with repeated measures followed by Holm–Sidak post hoc test for (B–D); unpaired T-test for (C1); and Mann–Whitney test for (D1,E,F). Values are mean ± SD, Swimmers n = 15, Control N = 27. **p < 0.01.
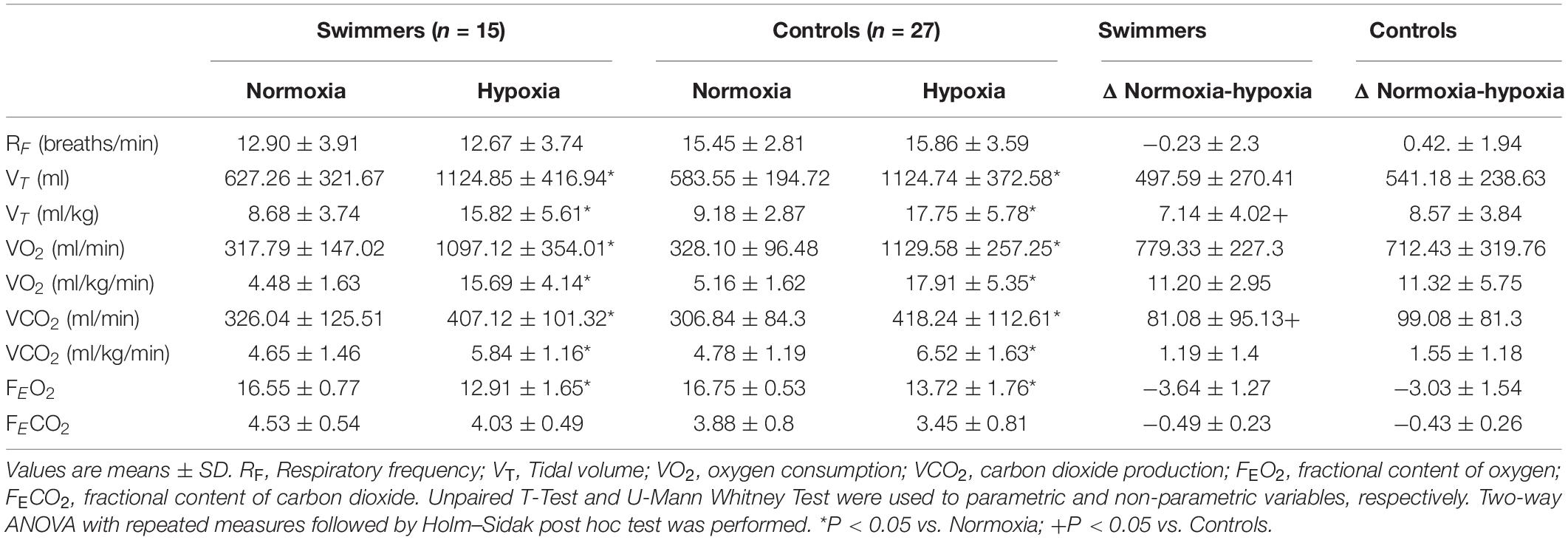
Table 2. Cardiovascular and respiratory responses to severe hypoxic challenge in swimmers and control participants.
Hypoxic Ventilatory Response
Both swimmers and controls showed a significant decrease of SpO2 (p < 0.001), without differences between groups (Figure 4B). Indeed, the ΔSpO2 was not different between groups (p = 0.089) (Figure 4B1). Swimmers showed a lower increase in VE (0.11 ± 0.04 vs. 0.19 ± 0.04 L⋅min⋅kg–1) from normoxic to hypoxic condition than the controls (0.14 ± 0.04 vs. 0.27 ± 0.06 vs. L/min/kg) (Figure 4C). Consequently, the ΔVE was lower in swimmers compared to control participants (5.51 ± 0.49 vs. 8.14 ± 0.34 Δ L⋅min⋅kg–1) (Figure 4C1) and the HVR, expressed as ΔVE/ΔSpO2 was significantly lower in swimmers compared to the control participants (0.007 ± 0.001 vs. 0.016 ± 0.002 ΔVE/ΔSpO2) (Figure 4E).
During the chemoreflex test (hypoxic challenge), swimmers and controls participants did not show significant differences in RF and FECO2, while there were significant increases in absolute and relative VT, absolute and relative VO2, absolute and relative VCO2 and FEO2 in both groups. However, the changes were only different for relative VT and relative VCO2 (Table 2).
Hypoxic Cardiac Response
Both groups showed a significant increase of maximum HR during hypoxic stimulation compared to their normoxic condition (swimmers: 74.12 ± 12.30 vs. 69.06 ± 10.26 beats/min; controls: 70.81 ± 9.97 vs. 65.40 ± 8.86 beats/min, p < 0.001) (Figure 4D). Indeed, cardiac response to hypoxia was similar in swimmers (5.1 ± 6.9 Δbeats/min) compared to controls (5.4 ± 7.4 Δbeats/min) (Figure 4D1). Accordingly, the HCR was similar between swimmers (0.27 ± 0.51 ΔHR/ΔSpO2) and controls (0.52 ± 1.04 ΔHR/ΔSpO2) (Figure 4F). In addition, during hypoxia there were no significant differences between groups in HRV disturbances (Supplementary Table 2).
Discussion
The aim of the study was to determine the maximum voluntary apnea duration and HVR, and the cardiovascular and autonomic adjustments during a breath-hold and during the hypoxic challenge in young-trained swimmers compared to control participants. The main findings of the present study are as follows: when compared to controls, swimmers showed: (i) longer maximum voluntary apnea duration; (ii) markedly decrease of HVR, with similar CHR; (iii) higher cardiac response during the maximum voluntary apnea test characterized by an overall autonomic imbalance. Our results strongly suggest that the lower ventilatory response to hypoxia (determined through a hypoxic challenge) might contribute to longer apnea duration in swimmers. However, the cardiovascular and autonomic adjustments during the breath-hold could be independent of hypoxic chemoreceptor activity and would be attributed to other mechanisms at the central level (i.e., central chemoreceptors).
Hypoxic Ventilatory Response and Apnea Duration in Young Highly Trained Swimmers
Aquatic immersion sports, such as apnea diving, artistic or distance swimming involve severe cardiovascular and autonomic responses to maintain blood flow and oxygen supply to activated tissues during exercise, despite not having pulmonary ventilation for several seconds or minutes (Heusser et al., 2009). During apneas, the decrease and increase of PaO2 and PaCO2 (Muth et al., 2003; Mansukhani et al., 2015), respectively, could stimulate peripheral chemoreceptors (Dempsey et al., 2012; Kumar and Prabhakar, 2012; Guyenet and Bayliss, 2015), and contribute to the end of apnea. Accordingly, considering that swimmers athletes are adapted to extreme hypoxia and hypercapnia during exercise (Heusser et al., 2009; Breskovic et al., 2010), we proposed that HVR could be diminished in young-trained swimmers. Thus, our data showed that swimmers displayed a decrease of HVR response, stimulated by a hypoxic challenge, which could contribute to maintain the apnea duration. We found that swimmers displayed a longer voluntary apnea duration compared to the control participants as shown previously (Heusser et al., 2009). This phenomenon is a typical adaptation in swimmers’ athletes (Schagatay et al., 2000). However, it is important to mention that we observed ∼80-s of maximum apnea duration, a “low” value compared to apnea divers (Heusser et al., 2009). Apnea divers are able to maintain a voluntary apnea up to ∼240-s (Heusser et al., 2009). This marked difference could be related to training status, specific training adaptations or genetics, such as the size of the spleen seen in immersion hunter tribes (Ilardo et al., 2018) or as well as the experience related to maintain longer apnea efforts (Viana et al., 2019). Nevertheless, and contrarily to our data, it has been shown that the breathing responses to hypoxia were not different between apnea divers and control subjects (Dujic et al., 2008; Breskovic et al., 2010). This may be explained by the training adaptations that are specific to swimming. Similarly, Bruce et al. (2021) showed that the maximal voluntary apnea duration is dependent of inspired air prior to apnea, but the HVR was not associated to chemoreflex drive. This finding is contrarily to our data; however, it is important to mention that in the present study we used young-trained swimmers and not healthy individual, which does not reflect the physiological adaptations to underwater training.
In addition, we observed that HCR was similar between swimmers and controls, suggesting that the chronotropic response to hypoxic stimulation is independent to HVR in swimmers. Nevertheless, similar to our results, Dujic et al. (2008) found that repeated episodes of hypoxemia alone are not sufficient to drive an increase in resting sympathetic activity in breath-hold divers. In addition, Breskovic et al. (2010) showed no differences in muscle sympathetic nerve activity during hypoxic challenge between divers and control participants, which could explain partly the HR response. Indeed, contrarily to apnea divers who sustain prolonged apneas, swimmers performed several intermittent apnea efforts during training sessions [between 3 and 20 s depending on the competition (Guimard et al., 2014)] which could be associated to a reduced HVR, but with similar HCR.
During an apnea, the PaCO2 increases and pH decreases can be sensed by the central chemoreceptors located in the brainstem. The most important zone in the brainstem, related to autonomic and breathing responses to CO2, is the retrotrapezoid nucleus (RTN) (Guyenet and Bayliss, 2015; Díaz et al., 2020). Therefore, it is possible that in swimmers the retention of CO2 during the apnea, triggered an RTN-dependent sympathoexcitation, which may have induced a greater tachycardic response. However, controversial evidence has been observed. Indeed, Trembach and Zabolotskikh (2017) showed that peripheral chemosensitivity, through stimulation by transient CO2 inhalation, was associated to the maximum voluntary apnea in healthy individuals; however, Bain et al. (2017) showed that the central respiratory chemoreflex, through stimulation by hyperoxic rebreathe, was not related to maximum breath-hold duration. The divergent evidence depicted by the manuscript of Trembach and Zabolotskikh (2017) compared to Bain et al. (2017) may be partially explained by the characteristics of the participants. While Trembach and Zabolotskikh (2017) recruited healthy participants, Bain et al. (2017) recruited apnea divers. In our study, we included young-trained swimmers’ athletes; hence, it is possible that the sport-training background of the athletes could partially explain the different results reported among studies.
Autonomic Control During Apnea in Young Highly Trained Swimmers
Acute autonomic response during an apnea effort is critical to maintain the blood flow and oxygen supply to activated muscles during training and/or competitive events in aquatic sports (Heusser et al., 2009; Breskovic et al., 2010). During an apnea, the arterial hypoxemia could explain the positive cardiac inotropic and chronotropic response, mediated by a parasympathetic withdrawal, which could be independent of pulmonary inflation previous to the apnea (inflation reflex) (Siebenmann et al., 2019). However, our data showed that control participants are characterized by a decrease of HFHRV, mainly associated to the parasympathetic tone, while swimmers showed both, a decrease of HFHRV and an increase of LFHRV, which is influenced by sympathetic and parasympathetic system (Goldstein et al., 2011), during the maximum apnea effort. Furthermore, concomitant with the autonomic response, we observed a more pronounced positive chronotropic response in swimmers than in control participants. Therefore, the more severe tachycardic response in swimmers may be a consequence of the autonomic imbalance due to decreased parasympathetic outflow and an increase of sympathetic drive.
The present study is not free of limitations. All our experiments were performed at rest, which is completely different to an exercise condition. Further, the maximum voluntary apnea efforts were performed outside of the water, which could impact on the perception of the subjects (Rodríguez-Zamora et al., 2012). Therefore, further studies should imply in-water immersion so to adequately reproduce the environment swimmers are exposed to. Furthermore, we did not test the central chemoreflex response, which has been seen to be decreased in swimmers (Ohkuwa et al., 1980) through ventilatory response to CO2, which is critical to determine the chronotropic response during the voluntary apnea test, considering that the swimmers showed more retention of CO2, suggesting the participation of central chemoreceptors. In addition, our study does not provide a direct relationship between HVR and apnea duration, which could be determined through pharmacological approaches. Therefore, future studies are required to assess the contribution of both, peripheral and central chemoreceptors on cardiac and autonomic responses induced by an apnea in swimmers athletes, who are subjected to different training regimen compared to other water sports.
Conclusion
Swimmers are characterized by the ability to modulate their breathing and by adaptations to intermittent hypoxemia/hypercapnia. Considering the longer duration of apnea in swimmers and the following cardiovascular adjustments to stop ventilation, we investigated the HVR in trained swimmers. Our data showed that the apnea duration was longer, and that HVR was decreased in swimmers, when compared to control subjects. However, the tachycardic response to apnea was more pronounced in swimmers with more retention of CO2, which suggest that central chemoreceptors could be involved in the autonomic response induced by the apnea.
Data Availability Statement
The raw data supporting the conclusions of this article will be made available by the authors, without undue reservation.
Ethics Statement
Protocols were approved by the Ethical Committee of the Universidad Mayor (Approval number #169_2019) and were performed according to the standards set by the latest version of the Declaration of Helsinki. Written informed consent to participate in this study was provided by the participants’ legal guardian/next of kin.
Author Contributions
DA and AA-Á performed the data collection and analysis, performed interpretation of the data, and contributed to the preparation of the manuscript. DA, AA-Á, MV-M, CV, MI, RR-C, CA, GM, RDR, and MI contributed to the preparation of the manuscript. RR-C, MV-M, CA-L, GPM, RDR, and MI performed interpretation of the data and contributed to the preparation of the manuscript. DA contributed to the concept of the project, performed interpretation of the data, preparation of the manuscript, and undertook in the laboratory of data analysis and interpretation. All authors approved the final version of the manuscript.
Funding
This work was supported by the “Vicerrectoria de Investigación” from Universidad Mayor (PEP I-2019050 and PEP I-2019064) and by Proyecto interdisciplinar Universidad Católica Silva Henríquez (II-2112AAA).
Conflict of Interest
The authors declare that the research was conducted in the absence of any commercial or financial relationships that could be construed as a potential conflict of interest.
Acknowledgments
We thank our volunteers and Paulina Arias for her assistance during all experiments, to the Instituto Nacional de Deportes (IND) and Federacion Chilena de Deportes Acuáticos (FECHIDA). RD was supported by the Fondecyt grant 1180172 and the Basal Center of Excellence in Aging and Regeneration (AFB 170005) and the special grant “Lithium in Health and Disease” from the Sociedad Química y Minera de Chile (SQM).
Supplementary Material
The Supplementary Material for this article can be found online at: https://www.frontiersin.org/articles/10.3389/fphys.2021.632603/full#supplementary-material
References
Andrade, D. C., Arce-Álvarez, A., Parada, F., Uribe, S., Gordillo, P., Dupre, A., et al. (2020). Acute effects of high intensity interval training session and endurance exercise on pulmonary function and cardiorespiratory coupling. Physiol. Rep. 8:e14455.
Andrade, D. C., Arce-Álvarez, A., Toledo, C., Díaz, H. S., Lucero, C., Quintanilla, R. A., et al. (2018). Revisiting the physiological effects of exercise training on autonomic regulation and chemoreflex control in heart failure: does ejection fraction matter? Am. J. Physiol. Heart Circ. Physiol. 314, H464–H474.
Bain, A. R., Barak, O. F., Hoiland, R. L., Drvis, I., Bailey, D. M., Dujic, Z., et al. (2017). Forced vital capacity and not central chemoreflex predicts maximal hyperoxic breath-hold duration in elite apneists. Respir. Physiol. Neurobiol. 242, 8–11. doi: 10.1016/j.resp.2017.02.015
Bourdillon, N., Fan, J. L., and Kayser, B. (2014). Cerebral oxygenation during the Richalet hypoxia sensitivity test and cycling time-trial performance in severe hypoxia. Eur. J. App.l Physiol. 114, 1037–1048. doi: 10.1007/s00421-014-2835-8
Breskovic, T., Ivancev, V., Banic, I., Jordan, J., and Dujic, Z. (2010). Peripheral chemoreflex sensitivity and sympathetic nerve activity are normal in apnea divers during training season. Auton. Neurosci. 154, 42–47. doi: 10.1016/j.autneu.2009.11.001
Bruce, C. D., Vanden Berg, E. R., Pfoh, J. R., Steinback, C. D., and Day, T. A. (2021). Prior oxygenation, but not chemoreflex responsiveness, determines breath-hold duration during voluntary apnea. Physiol. Rep. 9:e14664.
Camm, J. (1996). Heart rate variability: standards of measurement, physiological interpretation and clinical use. Task force of the european society of cardiology and the north american society of pacing and electrophysiology. Circulation 93, 1043–1065. doi: 10.1161/01.cir.93.5.1043
Compher, C., Frankenfield, D., Keim, N., Roth-Yousey, L., and Evidence Analysis Working Group (2006). Review Best practice methods to apply to measurement of resting metabolic rate in adults: a systematic review. J. Am. Diet. Assoc. 106, 881–903. doi: 10.1016/j.jada.2006.02.009
Costa, M. J., Balasekaran, G., Vilas-Boas, J. P., and Barbosa, T. M. (2015). Physiological adaptations to training in competitive swimming: a systematic review. J. Hum. Kinet. 49, 179–194. doi: 10.1515/hukin-2015-0120
Costill, D. L., Thomas, R., Robergs, R. A., Pascoe, D., Lambert, C., Barr, S., et al. (1991). Adaptations to swimming training: influence of training volume. Med. Sci. Sports Exerc. 23, 371–377.
Craig, A. B., Skehan, P. L., Pawelczyk, J. A., and Boomer, W. L. (1985). Velocity, stroke rate, and distance per stroke during elite swimming competition. Med. Sci. Sports Exerc. 17, 625–634. doi: 10.1249/00005768-198512000-00001
Del Rio, R., Andrade, D. C., Toledo, C., Diaz, H. S., Lucero, C., Arce-Álvarez, A., et al. (2017). Carotid body-mediated chemoreflex drive in the setting of low and high output heart failure. Sci. Rep. 7:8035.
Dempsey, J. A., Smith, C. A., Blain, G. M., Xie, A., Gong, Y., and Teodorescu, M. (2012). Role of central/peripheral chemoreceptors and their interdependence in the pathophysiology of sleep apnea. Adv. Exp. Med. Biol. 758, 343–349. doi: 10.1007/978-94-007-4584-1_46
Díaz, H. S., Toledo, C., Andrade, D. C., Marcus, N. J., and Del Rio, R. (2020). Neuroinflammation in heart failure: new insights for an old disease. J. Physiol. 598, 33–59. doi: 10.1113/jp278864
Dujic, Z., Ivancev, V., Heusser, K., Dzamonja, G., Palada, I., Valic, Z., et al. (2008). Central chemoreflex sensitivity and sympathetic neural outflow in elite breath-hold divers. J. Appl. Physiol. 104, 205–211. doi: 10.1152/japplphysiol.00844.2007
FINA (2020). Available online at: http://www.fina.org/ (accessed 01 Feb 2020).
Goldstein, D. S., Bentho, O., Park, M. Y., and Sharabi, Y. (2011). Low-frequency power of heart rate variability is not a measure of cardiac sympathetic tone but may be a measure of modulation of cardiac autonomic outflows by baroreflexes. Exp. Physiol. 96, 1255–1261. doi: 10.1113/expphysiol.2010.056259
Guimard, A., Prieur, F., Zorgati, H., Morin, D., Lasne, F., and Collomp, K. (2014). Acute apnea swimming: metabolic responses and performance. J. Strength Cond. Res. 28, 958–963. doi: 10.1519/jsc.0000000000000254
Guyenet, P. G., and Bayliss, D. A. (2015). Neural control of breathing and CO2 homeostasis. Neuron 87, 946–961. doi: 10.1016/j.neuron.2015.08.001
Heusser, K., Dzamonja, G., Tank, J., Palada, I., Valic, Z., Bakovic, D., et al. (2009). Cardiovascular regulation during apnea in elite divers. Hypertension 53, 719–724. doi: 10.1161/hypertensionaha.108.127530
Ilardo, M. A., Moltke, I., Korneliussen, T. S., Cheng, J., Stern, A. J., Racimo, F., et al. (2018). Physiological and genetic adaptations to diving in sea nomads. Cell 173, 569–580. doi: 10.1016/j.cell.2018.03.054
Iturriaga, R., and Alcayaga, J. (2004). Neurotransmission in the carotid body: transmitters and modulators between glomus cells and petrosal ganglion nerve terminals. Brain Res. Rev. 47, 46–53. doi: 10.1016/j.brainresrev.2004.05.007
Iturriaga, R., Del Rio, R., Idiaquez, J., and Somers, V. K. (2016). Carotid body chemoreceptors, sympathetic neural activation, and cardiometabolic disease. Biol. Res. 49:13.
Kumar, P., and Prabhakar, N. R. (2012). Peripheral chemoreceptors: function and plasticity of the carotid body. Compr. Physiol. 2, 141–219.
Lemaître, F., Seifert, L., Polin, D., Juge, J., Tourny-Chollet, C., and Chollet, D. (2009). Apnea training effects on swimming coordination. J. Strength Cond. Res. 23, 1909–1914. doi: 10.1519/jsc.0b013e3181b073a8
Mansukhani, M. P., Wang, S., and Somers, V. K. (2015). Chemoreflex physiology and implications for sleep apnea: insights from studies in humans. Exp. Physiol. 100, 130–135. doi: 10.1113/expphysiol.2014.082826
Miller, M. R., Crapo, R., Hankinson, J., Brusasco, V., Burgos, F., Casaburi, R., et al. (2005). ATS/ERS Task Force. General considerations for lung function testing. Eur. Respir. J. 26, 153–161. doi: 10.1183/09031936.05.00034505
Muth, C. M., Radermacher, P., Pittner, A., Steinacker, J., Schabana, R., Hamich, S., et al. (2003). Arterial blood gases during diving in elite apnea divers. Int. J. Sports Med. 24, 104–107. doi: 10.1055/s-2003-38401
Nieman, D. C., Austin, M. D., Benezra, L., Pearce, S., McInnis, T., Unick, J., et al. (2006). Validation of Cosmed’s FitMate in measuring oxygen consumption and estimating resting metabolic rate. Res. Sports Med. 14, 89–96. doi: 10.1080/15438620600651512
Nieman, D. C., Austin, M. D., Dew, D., and Utter, A. C. (2013). Validity of COSMED’s quark CPET mixing chamber system in evaluating energy metabolism during aerobic exercise in healthy male adults. Res. Sports Med. 21, 136–145. doi: 10.1080/15438627.2012.757227
Nunan, D., Sandercock, G. R., and Brodie, D. A. (2010). A quantitative systematic review of normal values for short-term heart rate variability in healthy adults. Pacing Clin. Electrophysiol. 33, 1407–1417. doi: 10.1111/j.1540-8159.2010.02841.x
Ohkuwa, T., Fujitsuka, N., Utsuno, T., and Miyamura, M. (1980). Ventilatory response to hypercapnia in sprint and long-distance swimmers. Eur. J. Appl. Physiol. Occup. Physiol. 43, 235–241. doi: 10.1007/bf00421837
Pfoh, J. R., Tymko, M. M., Abrosimova, M., Boulet, L. M., Foster, G. E., Bain, A. R., et al. (2016). Comparing and characterizing transient and steady-state tests of the peripheral chemoreflex in humans. Exp. Physiol. 101, 432–447. doi: 10.1113/ep085498
Richalet, J. P., Larmignat, P., Poitrine, E., Letournel, M., and Canouï-Poitrine, F. (2012). Physiological risk factors for severe high-altitude illness: a prospective cohort study. Am. J. Respir. Crit. Care Med. 185, 192–198. doi: 10.1164/rccm.201108-1396oc
Rodríguez-Zamora, L., Iglesias, X., Barrero, A., Chaverri, D., Erola, P., and Rodríguez, F. A. (2012). Physiological responses in relation to performance during competition in elite synchronized swimmers. PLoS One 7:e49098. doi: 10.1371/journal.pone.0049098
Schagatay, E., Van Kampen, M., Emanuelsson, S., and Holm, B. (2000). Effects of physical and apnea training on apneic time and the diving response in humans. Eur. J. Appl. Physiol. 82, 161–169. doi: 10.1007/s004210050668
Shei, R. J. (2018). Respiratory muscle training and aquatic sports performance. Sports Sci. Med. 17, 161–162.
Siebenmann, C., Ryrsø, C. K., Oberholzer, L., Fisher, J. P., Hilsted, L. M., Rasmussen, P., et al. (2019). Hypoxia-induced vagal withdrawal is independent of the hypoxic ventilatory response in men. J. Appl. Physiol. 126, 124–131. doi: 10.1152/japplphysiol.00701.2018
Speakman, J. R., and Selman, C. (2003). Physical activity and resting metabolic rate. Proc. Nutr. Soc. 62, 621–634. doi: 10.1079/pns2003282
Tarvainen, M. P., Niskanen, J. P., Lipponen, J. A., Ranta-Aho, P. O., and Karjalainen, P. A. (2014). Kubios HRV–heart rate variability analysis software. Comput. Methods Programs Biomed. 113, 210–220.
Trembach, N., and Zabolotskikh, I. (2017). Breath-holding test in evaluation of peripheral chemoreflex sensitivity in healthy subjects. Respir. Physiol. Neurobiol. 235, 79–82. doi: 10.1016/j.resp.2016.10.005
Vermeulen, T. D., Benbaruj, J., Brown, C. V., Shafer, B. M., Floras, J. S., and Foster, G. E. (2020). Peripheral chemoreflex contribution to ventilatory long-term facilitation induced by acute intermittent hypercapnic hypoxia in males and females. J. Physiol. 598, 4713–4730. doi: 10.1113/JP280458
Viana, E., Bentley, D. J., and Logan-Sprenger, H. M. (2019). A physiological overview of the demands, characteristics, and adaptations of highly trained artistic swimmers: a literature review. Sports Med. Open 5:16.
Keywords: apnea, autonomic nervous system, chemosensitivity, hypoxia, swimming
Citation: Arce-Álvarez A, Veliz C, Vazquez-Muñoz M, von Igel M, Alvares C, Ramirez-Campillo R, Izquierdo M, Millet GP, Del Rio R and Andrade DC (2021) Hypoxic Respiratory Chemoreflex Control in Young Trained Swimmers. Front. Physiol. 12:632603. doi: 10.3389/fphys.2021.632603
Received: 23 November 2020; Accepted: 08 February 2021;
Published: 26 February 2021.
Edited by:
Trevor A. Day, Mount Royal University, CanadaReviewed by:
Enrico M. Camporesi, USF Health, United StatesAlessandro Marroni, DAN Europe Foundation, Malta
Copyright © 2021 Arce-Álvarez, Veliz, Vazquez-Muñoz, von Igel, Alvares, Ramirez-Campillo, Izquierdo, Millet, Del Rio and Andrade. This is an open-access article distributed under the terms of the Creative Commons Attribution License (CC BY). The use, distribution or reproduction in other forums is permitted, provided the original author(s) and the copyright owner(s) are credited and that the original publication in this journal is cited, in accordance with accepted academic practice. No use, distribution or reproduction is permitted which does not comply with these terms.
*Correspondence: David C. Andrade, ZGNhbmRyYWRlQHVjLmNs; ZGF2aWQuYW5kcmFkZUB1YW50b2YuY2w=