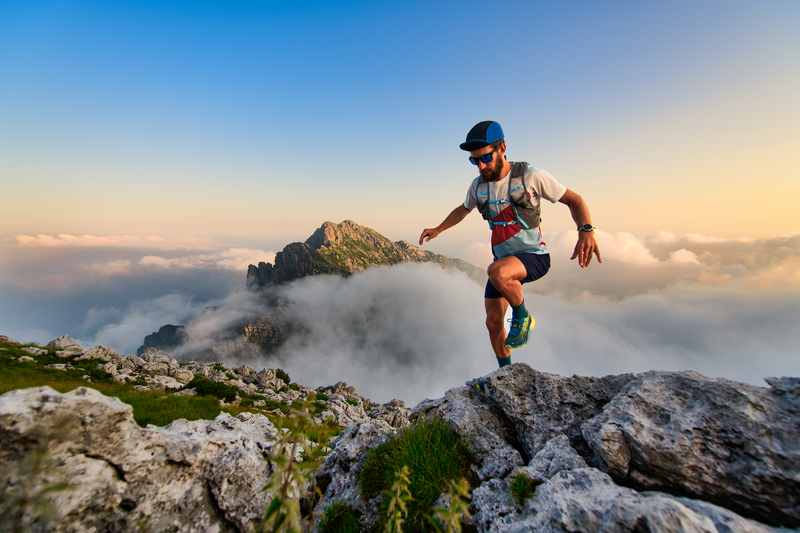
94% of researchers rate our articles as excellent or good
Learn more about the work of our research integrity team to safeguard the quality of each article we publish.
Find out more
ORIGINAL RESEARCH article
Front. Physiol. , 07 April 2021
Sec. Exercise Physiology
Volume 12 - 2021 | https://doi.org/10.3389/fphys.2021.620438
The present study investigated the angiotensin II (Ang II) responses in rat femoral veins taken from 2-kidney-1clip (2K1C) hypertensive rats at 4 weeks after clipping, as well as the effects of exercise on these responses. In this manner, femoral veins taken from 2K1C rats kept at rest or exposed to acute exercise or to exercise training were challenged with Ang II or endothelin-1 (ET-1) in organ bath. Simultaneously, the presence of cyclooxygenase-1 (COX-1) and cyclooxygenase-2 (COX-2) were determined in these preparations by western blotting. In these experiments, femoral veins exhibited subdued Ang II responses. However, after nitric oxide (NO) synthesis blockade, the responses were higher in the femoral veins taken from animals kept at rest [0.137(0.049–0.245); n = 10] than those obtained in trained animals kept at rest [0.008(0.001–0.041); n = 10] or studied after a single bout of exercise [0.001(0.001–0.054); n = 11]. In preparations in which, in addition to NO synthesis, both the local production of prostanoids and the action of ET-1 on type A (ETA) or B (ETB) receptors were inhibited, the differences induced by exercise were no longer observed. In addition, neither ET-1 responses nor the presence of COX-1 and COX-2 in these preparations were modified by the employed exercise protocols. In conclusion, NO maintains Ang II responses reduced in femoral veins of 2K1C animals at rest. However, vasodilator prostanoids as well as other relaxing mechanisms, activated by ETB stimulation, are mobilized by exercise to cooperate with NO in order to maintain controlled Ang II responses in femoral veins.
During exercise, there is a significant increase in metabolic demand, especially in cardiac, and working skeletal muscle (Delp and O’Leary, 2004; Duncker and Bache, 2008). To meet this metabolic demand an extensive blood flow redistribution occurs characterized by increases in blood flow to the heart, diaphragm and working skeletal muscles at the expense of flow to skin, stomach, intestines, pancreas, liver, kidneys, spleen, and non-working skeletal muscles (Armstrong et al., 1987; Musch et al.,1987a,b). It has been proposed that the exercise-induced blood flow redistribution may be facilitated by locally produced substances that modulate the vasomotor effects of angiotensin II (Ang II) in several vascular beds (Kashimura et al., 1995; Chies et al., 2010, 2013, 2017; Park et al., 2012). These studies suggest that local substances are released as a consequence of exercise-induced shear stress.
The effects of exercise upon the modulating mechanisms that regulate the vascular responses to endogenous vasoconstrictors are more clearly delineated in the arterial than in the venous beds. This is an important knowledge gap considering that, at rest, about 60–80% of the blood in mammals is localized in the venous compartment and a significant part of this volume may be shifted to the heart and muscle tissue during exercise (Rothe, 1983; Pang, 2001). Thus, an uncontrolled venoconstriction could result in an increment of resistance to centripetal blood flow, thus impairing the venous return (Rowell, 1993).
Exercise training promotes functional adaptations in rat portal vein that result in stronger Ang II responses whenever the animal is re-exposed to the exercise (Chies et al., 2010). Such greater Ang II responses apparently involve a balance between nitric oxide (NO) and vasodilator prostanoids, prevailing at rest, and endothelin-1 (ET-1) that prevails when the animal is subjected to exercise. Inhibition of NO synthesis increases the Ang II responses in femoral veins taken from sedentary animals kept at rest, but does not change such responses in preparations taken from animals submitted to a single bout of exercise or in those kept in training for 8 weeks. Apparently, mechanisms related to vasodilator prostanoids and/or some other vasodilator mechanism triggered by the action of ET-1 on ETB receptors (ETB) act as backup to NO in animals exposed to exercise, and thus maintain control of the Ang II responses in femoral veins (Chies et al., 2013).
Considering that the local modulating mechanisms described are necessary to keep the Ang II responses in femoral veins under control during exercise, it is important to assess how they are altered in pathophysiological conditions that involve cardiocirculatory modifications and endothelial dysfunction. The 2-kidney-1-clip (2K1C) rat model develops, in parallel to the blood pressure elevation, an oxidative stress-induced endothelial dysfunction (Ceron et al., 2010; Xue et al., 2015; Kumral et al., 2016). This experimental model is particularly interesting during the phase I (up to 4 weeks) because it is also characterized by elevations in the plasma and tissue Ang II levels (Martinez-Maldonado, 1991; Nakada et al., 1996; Xie et al., 2006). However, high levels of Ang II have not been consistently reported (Guan et al., 1992). Accordingly, the aim of the present study was to establish the effects of a single short bout of exercise as well as of regular exercise training on the Ang II responses in femoral veins taken from 2K1C rats.
Eighty-six Male Wistar rats (350–450 g) were housed in plastic cages (50 cm × 40 cm × 20 cm) with four animals per cage. Food and water were available ad libitum. During the exercise protocol, rats were maintained in the training room under a 12 h light-dark cycle, with lights on at 07:00 h. Room temperature was maintained at 25°C. All procedures were performed in accordance with ethical principles (Guide for the Care and Use of Laboratory Animals, 1996), and the study was reviewed and approved by the Research Ethics Committee of the Marília Medical School (Protocol no. 300/14).
Animals were distributed to the experimental groups, taking into account their innate exercise capacity (Melo et al., 2003). 2-month-old rats were trained by daily 10 min treadmill sessions, for 2 weeks (Movement Technology LX 170) without inclination, at speeds of 0.3 to 0.5 km/h. At the end of this period, these animals were submitted to the maximal exercise capacity test on treadmill. In this test, the animals were placed on a treadmill at a speed of 0.3 km/h. Then, every 3 min, the belt speed was increased by 0.3 km/h until exhaustion. The time that the animals continued to run under these conditions reflects their “maximal exercise capacity.” Based on this parameter, the animals were distributed among the groups so that all groups received animals that, on average, presented similar results in the exercise capacity test. The following experimental groups were constituted: RS = resting-sedentary, consisting of sedentary animals studied at rest; ES = exercised-sedentary, consisting of sedentary animals submitted to a 20 min session of exercise with speed of 60% of the average maximum velocity (equivalent to the first session of exercise training) immediately before the organ bath experiments; RT = resting-trained, consisting of trained animals studied at rest; and ET = exercised-trained, consisting of trained animals studied immediately after the last session of exercise, respectively. Based on the maximal exercise capacity test, we also determined the average speed of each group.
The animals designated as “trained” (belonging to RT and ET groups) were exercised 5 days per week for 1 h per day for 8 weeks. The exercise intensity was progressively increased by a combination of time and velocity, attaining 1 h per day at a velocity corresponding to 60% of average speed of each group by the third week. This protocol has been defined as constituting low-intensity exercise training (Melo et al., 2003; Martins et al., 2005).
Rats were anesthetized with tribromoethanol (250 mg/kg, i.p) and subjected to a midline laparotomy to access the left renal artery. A silver clip (0.25 mm) gap was placed on the renal artery. After surgery, animals received a dose of enrofloxacin (Baytril®; 25 mg/kg–1, i.m) and dipyrone (300 mg/kg–1; i.m.). Another dose of dipyrone was given 12 h after surgery. The systolic blood pressures of these animals were measured before, as well as 3 weeks after the clipping surgery using the “tail cuff” method. Hypertensive rats presented systolic pressures greater than 150 mmHg and elevation of blood pressure ≥30 mmHg in comparison to the pre-clipping condition.
The clipping surgery occurred during the exercise training period (4 weeks prior to euthanasia for vascular reactivity experiments). Because the vasoconstriction induced by Ang II is the focus of the present study, the femoral veins were harvested during this time, which corresponds to the end of the acute phase I. This phase is known as the renin-dependent phase and is characterized by elevation in the plasma and tissue Ang II levels (Martinez-Maldonado, 1991; Xie et al., 2006). After this surgery, the rats were not exercised for 4 days, so that they could recover.
For plasma Ang II quantification, some RS normotensive (two kidneys; 2K) animals were used. These animals were submitted to the laparotomy, but without placing the clip on the renal artery.
Animals were killed in a CO2 chamber and blood samples were immediately harvested by caval puncturing with a syringe containing ethylenediaminetetraacetic acid (EDTA). The blood samples were centrifuged at 4°C (3,500 rpm) in order to separate the plasma, followed by addition of a mix of protease inhibitors (cOmplete mini; Roche). The samples were immediately frozen at −80°C for later quantification of Ang II.
The extraction of Ang II was held in Oasis HBL 3cc columns (Waters, Ireland), previously activated with methanol (5 mL), tetrahydrofuran (5 mL), hexane (5 mL), methanol (5 mL), and water (10 mL). After sample introduction, columns were washed with water (10 mL) and peptides of interest were eluted with ethanol, acetic acid, and water (90: 4: 6). The eluted fractions were lyophilized and ressuspended in 500 μL mobile phase A: 5% ACN (50 mL) in 0.1% orthophosphoric acid (1 mL), and filtered with 0.22 μm membrane before analysis. The Ang II was quantified by ELISA, using the “Angiotensin II Enzyme Immunoassay Kit” (SPI-DIO), according to the manufacturer’s instructions.
Immediately after euthanasia and blood sample collection, the femoral veins were harvested, dissected, and divided into rings (3–4 mm) and set up in 2 mL organ baths. In the organ bath, the preparation was placed between two stainless-steel hooks, one of them bound to a stationary support and the other, connected to an isometric force transducer. Preparations were bathed in Krebs–Henseleit solution (composition in mmol/L): NaCl 130; KCl 4.7; CaCl2 1.6; KH2PO4 1.2; MgSO4 1.2; NaHCO3 15; glucose 11.1. The solution was kept at pH 7.4 and 37°C and bubbled continuously with a mixture of 95% O2 and 5% CO2. Tension was monitored continuously and recorded using a Powerlab 8/30 data-acquisition system (ADInstruments, Castle Hill, NSW, Australia). Prior to administering drugs, rings were equilibrated for 60 min at a resting tension of 0.5 g. The time frame from the end of the exercise sessions to the beginning of the Ang II cumulative concentration–response curves was approximately 90 min.
The responses (g) evoked by cumulatively adding Ang II (10–11 to 10–7 mol/L; Sigma) directly into the organ bath were plotted in Prism® software to obtain concentration–response curves. From these, the area under curve (AUC) was calculated. The AUC, instead pD2 (negative logarithm of the concentration that evoke 50% of the maximal response), was used because not all curves showed a sigmoidal profile. Some preparations challenged with (ET-1; 10–11 to 10–6 mol/L; Sigma) showed a sigmoidal profile, thereby permitting to obtain the pD2. The Rmax (maximum response), which is the highest response evoked by Ang II or ET-1 in each preparation was also determined.
The actions of Ang II were also evaluated in preparations pretreated for 20 min with 10–4 mol/L L-NAME and 10–5 mol/L indomethacin, non-selective NO synthase and cyclooxygenase inhibitors (Sigma), respectively, 10–6 mol/L BQ-123 (antagonist of endothelin receptor type A – ETA; Sigma) or 10–6 mol/L BQ-788 (antagonist of ETB; Sigma), 10–4 mol/L tiron (superoxide anion scavenger; Sigma), and 10–4 mol/L apocynin [a non-selective NAD(P)Hoxidase inhibitor; Sigma]. Some preparations were also studied in presence of PD 123,319, a selective inhibitor of angiotensin receptors subtype 2 (AT2). In parallel, the actions of ET-1 were evaluated in presence of both 10–4 mol/L L-NAME and 10–5 mol/L indomethacin. All drugs were administered directly into the organ bath.
Samples of femoral veins were collected, frozen in liquid nitrogen and stored at −80°C to be analyzed by Western blotting. Frozen samples were homogenized at 4°C in RIPA buffer (BioRad®, United States) with protease inhibitor (Sigma-Aldrich®, United States) in Tureaux type homogenizer for three 5-s cycles. The homogenate material was centrifuged at 15,000 rpm for 20 min at 4°C and the supernatant was collected. The protein quantification was performed as described by Bradford (1976) in ELISA plates with 96 wells, and read in an ELISA reader (595 nm). Aliquots (30 μg protein) were treated with buffer solution to run gel (Laemli Sample Buffer – BioRad®) and β-mercaptoethanol at 95°C for 5 min. The proteins were then separated by vertical electrophoresis (Mini-Protean, BioRad®) 7.5% polyacrylamide gel SDS-PAGE, and after the electrophoresis, transferred to a nitrocellulose membrane in a wet transfer system. Nonspecific protein binding was blocked with 3% skimmed milk in TBST buffer for 1 h at room temperature. The membranes were then incubated overnight with primary antibodies: COX-1 (4841; concentration 1:500; Anti-Rabbit, Cell Signaling Technology®, Danvers, MA, United States) and COX-2 (ab15191, concentration 1:500,Anti-Rabbit, Abcam Inc.®, Cambridge, MA, United States) all of which were diluted in 1% BSA in TBST. Subsequently, the membranes were washed in TBST for three 10-min cycles, incubated for 2 h with specific HRP secondary antibody (clone: ab97051, IgG goat-Anti-Rabbit concentration 1:1000, Abcam Inc.®, Cambridge, MA, United States; clone: a5420) diluted in 1% BSA in TBST, and then washed in TBST for three 10-min cycles. Immunoreactive components were revealed using a luminescence kit (AmershamTM ELC SelectTM Western Blotting Detection Reagent, GE Healthcare®, United Kingdom) and the optical density of each band was measured using Image J® Windows® software, normalized by GAPDH (G3PD, concentration 1:1000, Sigma-Aldrich Co.®, St. Louis, Mo, United States) density.
The data normality was verified by the Kolmogorov–Smirnov test with Lilliefors correction and the homogeneity of the variances by the Levene test. The variables with parametric distribution are presented as mean ± standard error of the mean (±S.E.M.) whereas the variables without parametric distribution are presented as median and interquartile range (25th first quartile; 75th third quartile). When the parametric distribution of the data was verified, Anova-one-way test, followed by the Bonferroni Post-Hoc test, was used for comparisons among independent groups. Blood pressure values were compared by mixed Anova of repeated measures. When the non-parametric distribution of the data was verified, Kruskal–Wallis test, followed by the peer-to-peer comparison by the Mann–Whitney test with the Holm-Sidak Post-Hoc correction, was used for comparisons among independent groups. The significance level adopted was 5% (p-value ≤ 0.05) and the data were analyzed using SPSS software (version 19.0).
It is noteworthy that Rmax and AUC did not present a normal distribution and/or the homogeneity of the variances, therefore, they were analyzed by a non-parametric test and presented in the tables as median and interquartile range. However, the concentration-response curves, from which these parameters were calculated, were built by mean ± S.E.M. in order to facilitate the visualization.
The clip placement around the left renal artery led to a significant increase of systolic blood pressure in all studied groups (Figure 1A). These animals, designated as 2K1C, were demonstrably hypertensive in the day of the organ bath experiments. However, there was no difference in plasma Ang II concentrations between 2K1C and 2K animals when they were not submitted to exercise. In addition, differences in plasma Ang II levels were also not observed between 2K1C rats kept at rest and those submitted to any kind of exercise (Figure 1B).
Figure 1. Systolic blood pressure (A) and plasma angiotensin II concentration (B) determined in 2K1C rats belonging to resting-sedentary (RS), exercised-sedentary (ES), resting-trained (RT), and exercised-trained (ET) groups. (B) also show plasma Ang II concentrations determined in resting-sedentary 2K animals. Values expressed as mean ± SEM; the number of independent determinations is in parentheses. *indicates significant difference (p < 0.001) from baseline in the same group (repeated measures ANOVA, followed by Bonferroni post-test).
Preparations of femoral veins taken from 2K1C animals presented very small contractile responses to Ang II (Figure 2A). These Ang II responses were equally modest in preparations pre-treated with indomethacin (Figure 2B). On the other hand, L-NAME pretreatment increased the Ang II responses about three times only in femoral veins taken from RS animals (Figure 2C). Thus, the Ang II Rmax and AUC values obtained in these animals were significantly higher in comparison to those obtained in trained animals, either kept at rest or exposed to a single bout of exercise (Tables 1, 2). The co-incubation with L-NAME and indomethacin increased the responses of Ang II in femoral veins taken from both ES and RT animals, but not in veins taken from ET animals. Actually, in this condition, sedentary and trained animals presented similar Ang II responses at rest. However, sedentary animals exhibited higher Ang II responses when submitted to a single bout of exercise while the opposite occurred with trained animals. Notably, the Ang II responses were widely dispersed within the ES group. Thus, no difference of Rmax was observed between groups in presence of both L-NAME and indomethacin (Figure 2D and Table 1). It should be noted that the AUC also did not differ between these groups (Table 2).
Figure 2. Ang II concentration-response curves determined in circular preparations of the femoral veins taken from resting-sedentary (RS), exercised-sedentary (ES), resting-trained (RT), and exercised-trained (ET) 2K1C rats, treated with saline (A), 10– 5 mol/L indomethacin (INDO; B), 10–4 mol/L L-NAME (C), 10–4 mol/L L-NAME plus 10–5 mol/L INDO (D), 10–4 mol/L L-NAME/10–5 mol/L INDO plus 10–6 M BQ-123 (E) or 10–6 M BQ-788 (F). Points represent the mean ± SEM, and the number of independent determinations is in parentheses. Dotted lines indicate groups that presented differences in terms of Rmax and AUC (see Tables 1, 2).
When BQ-123 was added to incubation, together with L-NAME and indomethacin, the Ang II responses obtained in all groups came closer to those obtained in the ET animals. In this manner, the exercise-induced changes of Ang II responses, that occurred in the presence of only L-NAME and indomethacin (Figure 2D and Tables 1, 2), were no longer observed (Figure 2E and Tables 1, 2). In contrast, when the BQ-788 was added in the incubation medium, together with L-NAME and indomethacin, the values of Ang II Rmax were elevated in all groups of animals (Figure 2F). Thus, the exercise-induced changes of Ang II responses, previously observed in the presence of only L-NAME and indomethacin (Figure 2D and Tables 1, 2), were no longer detectable. In addition, neither the exercise training nor the exposition of sedentary or trained animals to single bout of exercise resulted in significant changes of ET-1 responses in preparations pre-treated with both L-NAME and indomethacin. In this manner, no differences of ET-1 Rmax or pD2 were observed among groups (Figure 3).
Figure 3. Endothelin-1 (ET-1) concentration-response curves determined in circular preparations of the femoral veins taken from resting-sedentary (RS), exercised-sedentary (ES), resting-trained (RT), and exercised-trained (ET) 2K1C rats, treated with both 10–4 mol/L L-NAME and 10–5 mol/L indomethacin (INDO). Points represent the mean ± SEM, and the number of independent determinations is in parentheses.
In the presence of PD123,319, femoral veins taken from ES animals exhibited higher responses to Ang II in comparison to the other groups (Figure 4). In line with these modifications of response, Ang II Rmax values were significantly higher in preparations of ES animals when compared to those of RS animals (Table 1). Further, the Ang II responses remained very small in the presence of apocynin (Figure 5A) or tiron (Figure 5B). Moreover, in the presence of these inhibitors, there was no exercise-induced modification of Ang II response among the groups (Figures 5A,B and Tables 1, 2).
Figure 4. Ang II concentration-response curves determined in circular preparations of the femoral veins taken from resting-sedentary (RS), exercised-sedentary (ES), resting-trained (RT), and exercised-trained (ET) 2K1C rats, treated with 10–6 mol/L PD 123,319. Points represent the mean ± SEM, and the number of independent determinations is in parentheses. Dotted lines indicate groups that presented differences in terms of Rmax (see Table 1).
Figure 5. Ang II concentration-response curves determined in circular preparations of the femoral veins taken from resting-sedentary (RS), exercised-sedentary (ES), resting-trained (RT), and exercised-trained (ET) 2K1C rats, treated with 10–4 mol/L apocynin (A) or 10–4 mol/L tiron (B). Points represent the mean ± SEM, and the number of independent determinations is in parentheses.
The presence of COX-1 in femoral veins of 2K1C animals was not significantly different among the groups (Figure 6A). Likewise, there were also no differences in the levels of COX-2 among groups (Figure 6B).
Figure 6. Western blotting and semiquantitative analysis of COX-1 (A) COX-2 (B), estimated by the density of proteins bands (normalized relative to GAPDH), determined in femoral veins taken from resting-sedentary (RS), exercised-sedentary (ES), resting-trained (RT), and exercised-trained (ET) 2K1C rats. Bars indicate mean ± S.E.M of 4–5 samples.
In normotensive animals, mechanisms related to vasodilator prostanoids and/or a vasodilator mechanism mediated by ETB appear to act as backup to NO to keep the Ang II responses in femoral veins markedly reduced during exercise (Chies et al., 2013). This local modulation of Ang II responses may be even more necessary in 2K1C animals, which is a hypertension model that has activation of the renin-angiotensin-aldosterone system due to increased renin secretion by the clipped kidney leading to increases in circulating renin and ANG II levels (Martinez-Maldonado, 1991; Ceron et al., 2010). Previous studies have reported high plasma Ang II levels in 2K1C rats up to 4 weeks post clipping (Martinez-Maldonado, 1991; Nakada et al., 1996). However, Guan et al. (1992) observed an increment in Ang II plasma levels at 7 days, with a return to normal plasma levels around the 25th day after clipping. In the present study, there was no difference in plasma Ang II levels between 2K1C and 2K animals when they are not submitted to exercise. Moreover, neither the acute exercise nor exercise training changed the plasma Ang II levels in 2K1C animals. Although there were no modifications of plasma Ang II levels, the hypertension model was effective since all 2K1C animals had a significant elevation of systolic blood pressure 4 weeks after clipping. These data, however, do not negate the modulatory role of the Ang II response in the femoral veins during exercise, even if the concentration of this peptide is normal in plasma. Actually, during the fourth week of the 2K1C hypertension, when the plasma levels of Ang II trend to return to normal, the tissue levels of Ang II are still elevated (Guan et al., 1992). On the other hand, we also cannot rule out a faster cleavage of Ang II by the angiotensin-2 converting enzyme (ACE-2) into these femoral veins, converting it to Angiotensin 1-7 (Fernandes et al., 2011).
As observed in normotensive animals (Chies et al., 2013), different local mechanisms appear to act coordinately to mitigate the Ang II responses in femoral veins taken from 2K1C animals. In preparations from this group, Ang II responses were unchanged by exercise. However, during NO synthesis inhibition by L-NAME, the Ang II Rmax and AUC in femoral veins were increased, but only in animals that were not exposed to any exercise. In the presence of both indomethacin and L-NAME, the responses of Ang II were higher in different groups, with the exception of the ET group. These modifications of Ang II responses, however, were not followed by statistically significant differences of Rmax or AUC because the data were widely dispersed within the ES group. These data suggest that, like in normotensive animals (Chies et al., 2013), both acute exercise and exercise training mobilizes vasodilator prostanoids to act as backup to NO (Farouque and Meredith, 2003) in order to keep under control the Ang II responses in femoral veins of 2K1C animals. In ET rats, the femoral vein responses to Ang II remained reduced even in the presence of both indomethacin and L-NAME, which suggests the participation of additional mechanisms, not related to NO or prostanoids.
Exercise can increase the shear stress on the endothelium of veins (Cheng et al., 2003). In endothelial cell cultures, it has been shown that shear stress induces expression of several enzymes, including endothelial nitric oxide synthases (eNOS) and COX-2 (Uematsu et al., 1995; Topper et al., 1996; Xiao et al., 1997; Inoue et al., 2002). Thus, an increased amount of COX-1 and COX-2 supports an enhanced participation of vasodilator prostanoids suppressing the Ang II responses in femoral veins taken from animals submitted to exercise. In the present study, however, there were no statistically significant differences in either the COX-1 or COX-2 expression among the groups. These data suggest that the exercise-induced augmentation in the influence of vasodilator prostanoids on the Ang II responses in femoral veins may be due to an increment in COX(s) activity or to a higher smooth muscle sensitivity to vasodilator prostanoids.
The data obtained in presence of both L-NAME and indomethacin, although dispersed, suggest that the Ang II responses in femoral veins are enhanced whenever sedentary animals are exposed to acute exercise. This acute exercise-evoked potentiation of Ang II responses, however, can only be observed if the synthesis of NO and vasodilator prostanoids are blocked. It was reported that Ang II can release ET-1 from endothelial cells of rat aorta (Oriji, 1999) and bovine carotid artery (Emori et al., 1989) as well as from cultured rat vascular smooth muscle cells (VSMC; Hanehira et al., 1997) by a mechanism that involves AT1 stimulation and activation of kinase C (PKC) protein. Thus, ET-1 production may be increased by exercise, but its vascular effects can also be attenuated by other endothelial mediators in these conditions (Maeda et al., 1997; Rapoport and Merkus, 2017). In this manner, it is reasonable to infer that in femoral veins of 2K1C animals submitted to acute exercise, Ang II releases ET-1 inasmuch as it stimulates AT1 and, as a consequence, activates PKC (Karnik et al., 2015). This released ET-1, in turn, may augment the Ang II responses in these preparations by acting on ETA. Such potentiation of Ang II responses could remain suppressed by NO and vasodilator prostanoids. This hypothesis is supported by findings that the increased Ang II response observed in preparations of ES animals was no longer observed when BQ-123 was added to the incubation.
When BQ-788 was added in the incubation medium, together with L-NAME and indomethacin, the values of Ang II Rmax were elevated in all groups. This augmented Ang II response caused by BQ-788 was particularly evident in preparations taken from ET animals. Preparations taken from ET animals exhibited Ang II responses greater than those observed in the other groups. This suggests that, in femoral veins of ET 2K1C animals, the Ang II has its contractile effects attenuated by relaxing mechanisms, beyond those related to NO or vasodilator prostanoids, but which are activated through ETB stimulation. Supporting this inference, several studies have suggested that activation of ETB releases endothelium-derived relaxing substances that attenuate the vasoconstriction mediated by ETA in the vascular smooth muscle (Just et al., 2005; Maguire and Davenport, 2015). Moreover, Tirapelli et al. (2005) proposed that the ETB-mediated vasodilation in carotid artery involves not only NO and vasodilator prostanoids, but also activation of voltage-dependent K+ channels. Thus, it is likely that Ang II responses in femoral veins of 2K1C animals are also modulated by hyperpolarizing mechanisms activated as a consequence of ETB stimulation. Unfortunately, it was not possible to identify the mediator(s), released by ETB stimulation in these preparations.
The data obtained in presence of both BQ-123 and BQ-788 suggest that exercise mobilizes mechanisms through ETA and ETB receptors to regulate the Ang II responses in femoral veins taken from 2K1C animals. Notably, the participation of these receptors in the modulation of Ang II responses has similarities with that observed in femoral veins of normotensive animals submitted to exercise (Chies et al., 2013). This suggests that 2K1C hypertension does not substantially modify the functioning of local mechanisms that regulate the responses of these veins to Ang II.
Because of the possibility that Ang II responses in femoral veins may be influenced by ET-1, we decided to verify contractile effects of exogenous ET-1 in these preparations. This challenge was performed in the presence of both L-NAME and indomethacin in order to avoid NO and/or prostanoids masking the effects of exercise on the ET-1 responses. In these experiments, however, the exposure to exercise did not modify significantly the ET-1 responses in both sedentary and trained animals. These data suggest that the vascular responsiveness to ET-1 is not modified by exercise. However, it is noteworthy that the challenge with ET-1 was made in the absence of Ang II, which does not definitely exclude modifications of the ET-1 responses.
Interestingly, the presence of PD123,319 caused greater Ang II Rmax in ES animals, in comparison to the RS animals. In parallel, the opposite occurred in trained animals, which exhibited reduced Ang II Rmax immediately after a session of exercise. This pattern of response is similar to that observed in presence of both L-NAME and indomethacin, suggesting that the Ang II releases NO and prostanoids in femoral veins of animals exposed to the acute exercise through AT2 stimulation. It is worth mentioning, however, that elevations of Ang II responses in presence of PD123,319 were not observed in femoral veins of normotensive animals submitted to acute exercise (unpublished own data). This indicates that, in 2K1C animals, AT2-mediated mechanisms become essential to modulate Ang II responses in acute exercise situations.
The Ang II responses appear to be under constant modulation in the studied femoral veins, despite the high level of oxidative stress in 2K1C animals (Ceron et al., 2010; Campagnaro et al., 2013; Xue et al., 2015; Kumral et al., 2016). These data were unexpected because the NO, that appears to be essential in the modulation of Ang II responses in femoral veins of animals not exposed to exercise, may have its bioavailability reduced by the oxidative stress (Santilli et al., 2015; Korsager Larsen and Matchkov, 2016). In addition, Ang II responses were not affected either by tiron, a free radical scavenger, or apocynin, a NAD(P)H oxidase inhibitor. These data reinforce the notion that the 2K1C-related oxidative stress is not sufficient to impair significantly the modulation exerted by NO upon the Ang II responses in femoral veins.
In conclusion, the present study indicates that, in a similar manner as occurs in normotensive animals, a complex interaction of local modulating mechanisms keep the Ang II responses subdued in femoral veins of the 2K1C animals. NO-related mechanisms mitigate the Ang II responses in femoral veins of animals kept at rest. On the other hand, vasodilator prostanoids as well as other relaxing mechanisms related to ET-1 are mobilized by exercise to cooperate with NO in order to control Ang II responses in femoral veins of these animals (Figure 7).
Figure 7. Summary graph. Modulations of angiotensin II-induced vasoconstriction in femoral veins of exercised 2-kidney-1-clip hypertensive rats (2K1C). The main vasodilator endothelial mechanism, which modulates the angiotensin II-induced vasoconstrictor effects is nitric oxide (NO) formed by the enzymes nitric oxide synthases (NOS) from L-arginine (L-Arg). Under training, vasodilator prostanoids formed by cyclooxygenase enzymes (COX1 / 2) from arachidonic acid (AA), as well as vasodilator mediators not yet identified that are released via the angiotensin II – Endothelin-1 (ET-1) – ETB receptors (ETB), are mobilized to act cooperatively to nitric oxide.
The original contributions presented in the study are included in the article/Supplementary Material, further inquiries can be directed to the corresponding author/s.
The animal study was reviewed and approved by Research Ethics Committee of the Marília Medical School (Protocol no. 300/14).
AC, MS, DC, and LN contributed to design the experiments in the study. RD, PO, TM, RM, and CR took part in the experiments. AB and AS contributed to analyze the data and writing the manuscript. DC and LN helped to perform the analysis with constructive discussions as well as reviewing the writing. All authors contributed to the article and approved the submitted version.
This study was supported by Fundação de Amparo à Pesquisa do Estado de São Paulo (FAPESP; Nos. 2013/22655-9 and 2021/02532-6).
The authors declare that the research was conducted in the absence of any commercial or financial relationships that could be construed as a potential conflict of interest.
The authors thank Dale M. Seth (Department of Physiology, Tulane Hypertension and Renal Center of Excellence, Tulane University School of Medicine, New Orleans, Louisiana) by the aid in the plasma Ang II quantification.
The Supplementary Material for this article can be found online at: https://www.frontiersin.org/articles/10.3389/fphys.2021.620438/full#supplementary-material
Armstrong, R. B., Delp, M. D., Goljan, E. F., and Laughlin, M. H. (1987). Distribution of blood flow in muscles of miniature swine during exercise. J. Appl. Physiol. 62, 1285–1298. doi: 10.1152/jappl.1987.62.3.1285
Bradford, M. M. (1976). A rapid and sensitive method for the quantitation of microgram quantities of protein utilizing the principle of protein-dye binding. Anal. Biochem. 72, 248–254. doi: 10.1016/0003-2697(76)90527-3
Campagnaro, B. P., Tonini, C. L., Nogueira, B. V., Casarini, D. E., Vasquez, E. C., and Meyrelles, S. S. (2013). DNA damage and augmented oxidative stress in bone marrow mononuclear cells from Angiotensin-dependent hypertensive mice. Int. J. Hypertens. 2013:305202.
Ceron, C. S., Castro, M. M., Rizzi, E., Montenegro, M. F., Fontana, V., Salgado, M. C., et al. (2010). Spironolactone and hydrochlorothiazide exert antioxidant effects and reduce vascular matrix metalloproteinase-2 activity and expression in a model of renovascular hypertension. Br. J. Pharmacol. 160, 77–87. doi: 10.1111/j.1476-5381.2010.00678.x
Cheng, C. P., Herfkens, R. J., and Taylor, C. A. (2003). Inferior vena caval hemodynamics quantified in vivo at rest and during cycling exercise using magnetic resonance imaging. Am. J. Physiol. Heart Circ. Physiol. 284, H1161–H1167.
Chies, A. B., de Oliveira, P. B., Rossignoli, P. S., Baptista, R. F., de Labio, R. W., and Payao, S. L. (2017). Prostanoids counterbalance the synergism between endothelin-1 and angiotensin II in mesenteric veins of trained rats. Peptides 88, 67–73. doi: 10.1016/j.peptides.2016.12.013
Chies, A. B., de Souza Rossignoli, P., and Daniel, E. F. (2010). Exercise increases the angiotensin II effects in isolated portal vein of trained rats. Peptides 31, 883–888. doi: 10.1016/j.peptides.2010.02.011
Chies, A. B., Rossignoli, P. D. S., Baptista, R. D. F., de Lábio, R. W., and Payão, S. L. (2013). Exercise reduces angiotensin II responses in rat femoral veins. Peptides 44, 47–54. doi: 10.1016/j.peptides.2013.01.020
Delp, M. D., and O’Leary, D. S. (2004). Integrative control of the skeletal muscle microcirculation in the maintenance of arterial pressure during exercise. J. Appl. Physiol. 97, 1112–1118. doi: 10.1152/japplphysiol.00147.2003
Duncker, D. J., and Bache, R. J. (2008). Regulation of coronary blood flow during exercise. Physiol. Rev. 88, 1009–1086. doi: 10.1152/physrev.00045.2006
Emori, T., Hirata, Y., Ohta, K., Shichiri, M., and Marumo, F. (1989). Secretory mechanism of immunoreactive endothelin in cultured bovine endothelial cells. Biochem. Biophys. Res. Commun. 160, 93–100. doi: 10.1016/0006-291x(89)91625-2
Farouque, H. M., and Meredith, I. T. (2003). Relative contribution of vasodilator prostanoids, NO, and KATP channels to human forearm metabolic vasodilation. Am. J. Physiol. Heart Circ. Physiol. 284, H2405–H2411.
Fernandes, T., Hashimoto, N. Y., Magalhães, F. C., Fernandes, F. B., Casarini, D. E., Carmona, A. K., et al. (2011). Aerobic exercise training-induced left ventricular hypertrophy involves regulatory MicroRNAs, decreased angiotensin-converting enzyme-angiotensin ii, and synergistic regulation of angiotensin-converting enzyme 2-angiotensin (1-7). Hypertension 58, 182–189. doi: 10.1161/hypertensionaha.110.168252
Guan, S., Fox, J., Mitchell, K. D., and Navar, L. G. (1992). Angiotensin and angiotensin converting enzyme tissue levels in two-kidney, one clip hypertensive rats. Hypertension 20, 763–767. doi: 10.1161/01.hyp.20.6.763
Hanehira, T., Kohno, M., and Yoshikawa, J. (1997). Endothelin production in cultured vascular smooth muscle cells–modulation by the atrial, brain, and C-type natriuretic peptide system. Metabolism 46, 487–493. doi: 10.1016/s0026-0495(97)90182-7
Inoue, H., Taba, Y., Miwa, Y., Yokota, C., Miyagi, M., and Sasaguri, T. (2002). Transcriptional and posttranscriptional regulation of cyclooxygenase-2 expression by fluid shear stress in vascular endothelial cells. Arterioscler. Thromb. Vasc. Biol. 22, 1415–1420. doi: 10.1161/01.atv.0000028816.13582.13
Just, A., Olson, A. J., Falck, J. R., and Arendshorst, W. J. (2005). NO and NO-independent mechanisms mediate ETB receptor buffering of ET-1-induced renal vasoconstriction in the rat. Am. J. Physiol. Regul. Integr. Comp. Physiol. 288, R1168–R1177.
Karnik, S. S., Unal, H., Kemp, J. R., Tirupula, K. C., Eguchi, S., Vanderheyden, P. M., et al. (2015). International union of basic and clinical pharmacology. XCIX. Angiotensin receptors: interpreters of pathophysiological angiotensinergic stimuli [corrected]. Pharmacol. Rev. 67, 754–819. doi: 10.1124/pr.114.010454
Kashimura, O., Sakai, A., and Yanagidaira, Y. (1995). Effects of exercise-training on hypoxia and angiotensin II-induced pulmonary vasoconstrictions. Acta Physiol. Scand. 155, 291–295. doi: 10.1111/j.1748-1716.1995.tb09976.x
Korsager Larsen, M., and Matchkov, V. V. (2016). Hypertension and physical exercise: the role of oxidative stress. Medicina (Kaunas) 52, 19–27. doi: 10.1016/j.medici.2016.01.005
Kumral, Z. N., Sener, G., Ozgur, S., Koc, M., Suleymanoglu, S., Hurdag, C., et al. (2016). Regular exercise alleviates renovascular hypertension-induced cardiac/endothelial dysfunction and oxidative injury in rats. J. Physiol. Pharmacol. 67, 45–55.
Maeda, S., Miyauchi, T., Sakane, M., Saito, M., Maki, S., Goto, K., et al. (1997). Does endothelin-1 participate in the exercise-induced changes of blood flow distribution of muscles in humans? J. Appl. Physiol. 82, 1107–1111. doi: 10.1152/jappl.1997.82.4.1107
Maguire, J. J., and Davenport, A. P. (2015). Endothelin receptors and their antagonists. Semin. Nephrol. 35, 125–136. doi: 10.1016/j.semnephrol.2015.02.002
Martinez-Maldonado, M. (1991). Pathophysiology of renovascular hypertension. Hypertension 17, 707–719. doi: 10.1161/01.hyp.17.5.707
Martins, A. S., Crescenzi, A., Stern, J. E., Bordin, S., and Michelini, L. C. (2005). Hypertension and exercise training differentially affect oxytocin and oxytocin receptor expression in the brain. Hypertension 46, 1004–1009. doi: 10.1161/01.hyp.0000175812.03322.59
Melo, R. M., Martinho, E. Jr., and Michelini, L. C. (2003). Training-induced, pressure-lowering effect in SHR: wide effects on circulatory profile of exercised and nonexercised muscles. Hypertension 42, 851–857. doi: 10.1161/01.hyp.0000086201.27420.33
Musch, T. I., Friedman, D. B., Pitetti, K. H., Haidet, G. C., Stray-Gundersen, J., Mitchell, J. H., et al. (1987a). Regional distribution of blood flow of dogs during graded dynamic exercise. J. Appl. Physiol. 63, 2269–2277. doi: 10.1152/jappl.1987.63.6.2269
Musch, T. I., Haidet, G. C., Ordway, G. A., Longhurst, J. C., and Mitchell, J. H. (1987b). Training effects on regional blood flow response to maximal exercise in foxhounds. J. Appl. Physiol. 62, 1724–1732. doi: 10.1152/jappl.1987.62.4.1724
Nakada, T., Iijima, Y., Kubota, Y., Watanabe, M., Ishigooka, M., and Suzuki, H. (1996). Increased vascular collagen and noncollagenous protein synthesis contributes to sustain chronic phase of two-kidney, one-clip renovascular hypertension. J. Urol. 156, 1180–1185. doi: 10.1016/s0022-5347(01)65746-x
Oriji, G. K. (1999). Angiotensin II-induced ET and PGI2 release in rat aortic endothelial cells is mediated by PKC. Prostaglandins Leukot. Essent. Fatty Acids 61, 113–117. doi: 10.1054/plef.1999.0079
Pang, C. C. (2001). Autonomic control of the venous system in health and disease: effects of drugs. Pharmacol. Ther. 90, 179–230. doi: 10.1016/s0163-7258(01)00138-3
Park, Y., Prisby, R. D., Behnke, B. J., Dominguez, J. M. II, Lesniewski, L. A., Donato, A. J., et al. (2012). Effects of aging, TNF-alpha, and exercise training on angiotensin II-induced vasoconstriction of rat skeletal muscle arterioles. J. Appl. Physiol. 113, 1091–1100. doi: 10.1152/japplphysiol.00292.2012
Rapoport, R. M., and Merkus, D. (2017). Endothelin-1 regulation of exercise-induced changes in flow: dynamic regulation of vascular tone. Front. Pharmacol. 8:517. doi: 10.3389/fphar.2017.00517
Rothe, C. F. (1983). “Venous system: physiology of the capacitance vessels,” in Handbook of Physiology: The Cardiovascular System, eds J. T. Sherpherd and F. M. Abboud (Washington, DC: Wiley-Blackwell), 397–452.
Santilli, F., D’Ardes, D., and Davi, G. (2015). Oxidative stress in chronic vascular disease: from prediction to prevention. Vascul. Pharmacol. 74, 23–37. doi: 10.1016/j.vph.2015.09.003
Tirapelli, C. R., Casolari, D. A., Yogi, A., Montezano, A. C., Tostes, R. C., Legros, E., et al. (2005). Functional characterization and expression of endothelin receptors in rat carotid artery: involvement of nitric oxide, a vasodilator prostanoid and the opening of K+ channels in ETB-induced relaxation. Br. J. Pharmacol. 146, 903–912. doi: 10.1038/sj.bjp.0706388
Topper, J. N., Cai, J., Falb, D., and Gimbrone, M. A. Jr. (1996). Identification of vascular endothelial genes differentially responsive to fluid mechanical stimuli: cyclooxygenase-2, manganese superoxide dismutase, and endothelial cell nitric oxide synthase are selectively up-regulated by steady laminar shear stress. Proc. Natl. Acad. Sci. U.S.A. 93, 10417–10422. doi: 10.1073/pnas.93.19.10417
Uematsu, M., Ohara, Y., Navas, J. P., Nishida, K., Murphy, T. J., Alexander, R. W., et al. (1995). Regulation of endothelial cell nitric oxide synthase mRNA expression by shear stress. Am. J. Physiol. 269(6 Pt 1), C1371–C1378.
Xiao, Z., Zhang, Z., Ranjan, V., and Diamond, S. L. (1997). Shear stress induction of the endothelial nitric oxide synthase gene is calcium-dependent but not calcium-activated. J. Cell. Physiol. 171, 205–211. doi: 10.1002/(sici)1097-4652(199705)171:2<205::aid-jcp11>3.0.co;2-c
Xie, Q. Y., Sun, M., Yang, T. L., and Sun, Z. L. (2006). Losartan reduces monocyte chemoattractant protein-1 expression in aortic tissues of 2K1C hypertensive rats. Int. J. Cardiol. 110, 60–66. doi: 10.1016/j.ijcard.2005.07.046
Keywords: angiotensin II, endothelin-1, exercise, femoral vein, 2K1C hypertension, nitric oxide, prostanoids
Citation: Chies AB, Spadella MA, de Oliveira PR, Domeniconi RF, de Mello Santos T, Moreira RP, Rosales CB, Casarini DE and Navar LG (2021) Exercise-Induced Modulation of Angiotensin II Responses in Femoral Veins From 2-Kidney-1-Clip Hypertensive Rats. Front. Physiol. 12:620438. doi: 10.3389/fphys.2021.620438
Received: 22 October 2020; Accepted: 15 March 2021;
Published: 07 April 2021.
Edited by:
Jianhua Li, Pennsylvania State University College of Medicine, United StatesReviewed by:
Lu Qin, Penn State Milton S. Hershey Medical Center, United StatesCopyright © 2021 Chies, Spadella, de Oliveira, Domeniconi, de Mello Santos, Moreira, Rosales, Casarini and Navar. This is an open-access article distributed under the terms of the Creative Commons Attribution License (CC BY). The use, distribution or reproduction in other forums is permitted, provided the original author(s) and the copyright owner(s) are credited and that the original publication in this journal is cited, in accordance with accepted academic practice. No use, distribution or reproduction is permitted which does not comply with these terms.
*Correspondence: Agnaldo Bruno Chies, YWduYWxkb2NoaWVzQGhvdG1haWwuY29t
†ORCID: Agnaldo Bruno Chies, orcid.org/0000-0001-9263-2475; Luis Gabriel Navar, orcid.org/0000-0002-3777-7564
Disclaimer: All claims expressed in this article are solely those of the authors and do not necessarily represent those of their affiliated organizations, or those of the publisher, the editors and the reviewers. Any product that may be evaluated in this article or claim that may be made by its manufacturer is not guaranteed or endorsed by the publisher.
Research integrity at Frontiers
Learn more about the work of our research integrity team to safeguard the quality of each article we publish.