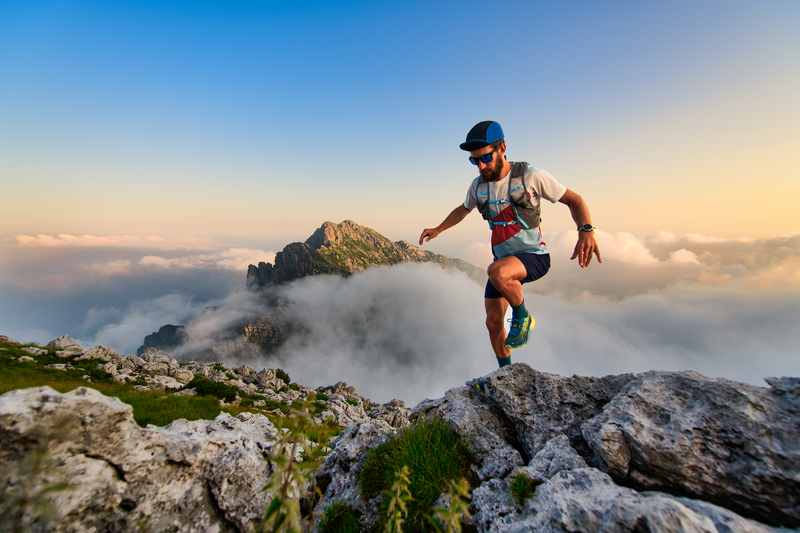
94% of researchers rate our articles as excellent or good
Learn more about the work of our research integrity team to safeguard the quality of each article we publish.
Find out more
REVIEW article
Front. Physiol. , 26 February 2021
Sec. Striated Muscle Physiology
Volume 12 - 2021 | https://doi.org/10.3389/fphys.2021.619710
This article is part of the Research Topic Calcium Homeostasis in Skeletal Muscle Function, Plasticity and Disease View all 14 articles
Skeletal muscle possesses remarkable plasticity that permits functional adaptations to a wide range of signals such as motor input, exercise, and disease. Small animal models have been pivotal in elucidating the molecular mechanisms regulating skeletal muscle adaptation and plasticity. However, these small animal models fail to accurately model human muscle disease resulting in poor clinical success of therapies. Here, we review the potential of in vitro three-dimensional tissue-engineered skeletal muscle models to study muscle function, plasticity, and disease. First, we discuss the generation and function of in vitro skeletal muscle models. We then discuss the genetic, neural, and hormonal factors regulating skeletal muscle fiber-type in vivo and the ability of current in vitro models to study muscle fiber-type regulation. We also evaluate the potential of these systems to be utilized in a patient-specific manner to accurately model and gain novel insights into diseases such as Duchenne muscular dystrophy (DMD) and volumetric muscle loss. We conclude with a discussion on future developments required for tissue-engineered skeletal muscle models to become more mature, biomimetic, and widely utilized for studying muscle physiology, disease, and clinical use.
Skeletal muscle is the largest organ in the body by mass and is essential for respiration, locomotion, posture, and whole-body energy homeostasis. To attain maximal performance and efficiency for these diverse roles, skeletal muscle displays a remarkable level of plasticity. Specifically, multiple isoforms of contractile, calcium-handling, metabolic, and structural proteins have evolved to meet the broad demands placed upon skeletal muscle (Schiaffino and Reggiani, 2011). Skeletal muscle dysfunction due to genetic mutations, aging, volumetric muscle loss, or acquired diseases significantly impair quality of life and can even be lethal. The foundation of our mechanistic understanding of skeletal muscle function, plasticity, and disease is derived predominantly from in vivo animal experiments and two-dimensional (2D) in vitro cell culture studies. Small animal studies, particularly comparative biology and genetic manipulations, have been pivotal in elucidating the molecular mechanisms regulating skeletal muscle function and plasticity (Schiaffino and Reggiani, 2011; Hoppeler, 2016). However, small animal models require additional translational and validation models to increase the successful translation of identified therapies to the clinic (Hay et al., 2014). The in vitro culture of human cells has the potential to generate experimental models with increased translational relevance. However, traditional skeletal muscle cell culture systems have limited longevity due to cellular detachment resulting in developmentally immature tissues with limited translational relevance. Over the past 30 years, three-dimensional (3D) tissue engineered skeletal muscle culture systems have been developed that better mimic the native muscle microenvironment, permit functional testing, and enable prolonged culture durations (Khodabukus et al., 2018; Wang J. et al., 2019). In this review, we discuss methods to generate three-dimensional tissues and factors that regulate their functionality. We then discuss multiple factors regulating skeletal muscle fiber-type in vivo and the ability to study these factors in vitro. Lastly, we discuss further developments regulated for engineered muscle tissues to become more widely utilized and to better model adult skeletal muscle.
Skeletal muscle is comprised of a hierarchical architectural structure that permits efficient force generation. Ultra-structurally, the most basic units of a myofibers are sarcomeres that contain myosin and actin which form overlaps to permits muscle contraction in a calcium-dependent manner. Efficient muscle contraction is coordinated by the transverse tubule system, a branched membrane network that runs along the entire length of the myofiber to the junction of the A and I bands of the sarcomere. The calcium required for contraction is stored in the sarcoplasmic reticulum (SR) which connects to the t-tubule at the specialized terminal cisternae. The SR stores calcium at a significantly higher concentration than seen in the sarcoplasm due to the calcium-binding protein calsequestrin (CSQ) (Murphy et al., 2009; Lamboley et al., 2013). Calcium is released from SR by the ryanodine receptor 1 (RyR1) into the myofibrils and binds to troponin C inducing a conformational change which results in removal of tropomyosin from myosin which enables actin to bind to myosin. Actin binding to myosin results in adenosine triphosphate (ATP) hydrolysis that causes actin to pull along myosin, shortening the sarcomere and generating muscle contraction (Sellers, 2004). Skeletal muscle relaxation is an active process that requires removal of calcium from the myofibrils to reestablish tropomyosin blocking of myosin actin-binding sites. Calcium removal is regulated by the sarcoplasmic-endoplasmic reticulum Ca2+ ATPase pumps (SERCA) that pump calcium back to the SR and the high affinity calcium binding protein parvalbumin found in fast skeletal muscle that quickens relaxation rate (Muntener et al., 1985; Schwaller et al., 1999).
Due to the high metabolic demands of skeletal muscle, long-term in vitro culture of whole skeletal muscles is impossible due to hypoxia and resultant loss of viability. To minimize hypoxic stress, muscles such as the extensor digitorum longus (EDL) and soleus (SOL) can be dissected to generate muscle strips that can be cultured for up to 12 h in highly oxygenated media (Park et al., 2012). These tissues can be utilized to measure contractile function (Brooks and Faulkner, 1988) and assess insulin-stimulated glucose uptake (Hansen et al., 1994). To overcome the short-term culture duration of intact muscle explants, single myofibers can be carefully dissected and isolated from the muscle belly and cultured for up to 7 days (Renzini et al., 2018), though most studies utilize 48–96 h time points. The single myofiber model is the gold-standard model to study satellite cell activation in vitro, due to SCs being retained within their niche and the ability to study SC dynamics with multiple modalities (Pasut et al., 2013; Wang Y. X. et al., 2019). Single fiber studies utilizing transgenic mice have been used to help unravel the transcription factors and molecular mechanisms regulating SC activation (Beauchamp et al., 2000; Kuang et al., 2007), polarity (Le Grand et al., 2009; Dumont et al., 2015), and symmetric divisions (Wang Y. X. et al., 2019). Intact single fiber studies have also enabled role of MyHC isoform on contractile properties (Bottinelli et al., 1996; Harridge et al., 1996) and the factors regulating muscle fatigue to be assessed (Westerblad and Allen, 1991; Westerblad et al., 1993). Single myofibers can also be used to assess multiple aspects of muscle physiology includingfactors regulating excitation-coupling (Allen et al., 1997; Prosser et al., 2010), genetic regulators of calcium-handling and t-tubule stability (Al-Qusairi et al., 2009; Kerr et al., 2013), membrane resealing in response to injury (Bansal et al., 2003; Sreetama et al., 2018), glucose uptake in response to electrical stimulation (Castorena et al., 2015) and insulin (Lanner et al., 2006), and to study mitochondrial function (Schuh et al., 2012). Single fiber studies have been pivotal to increasing our understanding of muscle physiology and permit in vitro testing of muscle fibers with adult ultrastructure and function. Importantly, the described 2D and 3D studies below are yet to replicate the developmental maturation and contractile function of the single fiber system. However, both explant and single fiber preparations are technically challenging, have limited experimental duration, are difficult to scale up for high throughput screening, and require continual new samples – making large-scale experiments in human tissues infeasible.
The alternative method to single fiber culture models is to liberate satellite cells from their niche by enzymatic dissociation or permitting them to “outgrow” from partially minced muscle tissue. This method results in heterogenous cell populations found within skeletal muscles that can be further purified by cell surface marker expression (Maesner et al., 2016; Uezumi et al., 2016) by taking advantage of the faster adhesion kinetics of fibroblasts to enrich for muscle progenitor cells by pre-plating (Gharaibeh et al., 2008). Satellite cells isolated by this method rapidly activate and become myoblasts or muscle precursor cells (MPCs) characterized by increased expression of MyoD, and decreased expression of Pax7 (Ryall et al., 2015). The functional impact of this SC activation is the dramatically impaired ability of these cultured cells to engraft in skeletal muscle upon transplantation, with just 72 h in culture decreasing engraftment efficiency threefold (Montarras et al., 2005). To minimize activation of these cells, small molecules (Charville et al., 2015), culture substratum stiffness (Gilbert et al., 2010), and regulating metabolic fuel source (Ryall et al., 2015) have been described to maintain MPCs in a more stem-like state. Alternatively, a quiescence media and culture system has been developed that can maintain SCs in a quiescent-like state but cannot reverse activated cells into a quiescent state (Quarta et al., 2016).
After expansion, MPCs can be induced to differentiate by reduction of serum content that induces cell cycle withdrawal, upregulation of differentiation genes, and ultimately fusion into multi-nucleated myotubes. Due to the rapid rate (48–96 h) of fusion and ease of the system, this model is the most frequently used system to assess the impact of genetic manipulations, growth factors, or small molecules on muscle differentiation and fusion. However, the assessment of longer-term (<7 days) experimental interventions are often prevented due to the detachment of myotubes due to spontaneous contractions of developing myotubes (Cooper et al., 2004). Furthermore, this detachment limits the developmental maturation of the tissue cultures, limiting the translation relevance of experimental findings (Rao et al., 2018). Lastly, the assessment of contractile function, the primary measure for therapeutic efficacy, is not permitted by traditional two-dimensional culture preventing functional assessments.
To overcome the limitations of 2D cell culture, several 3D skeletal muscle culture models have been developed over the past 30 years (Khodabukus et al., 2018; Wang J. et al., 2019). Two culture methods have been predominantly utilized: (1) hydrogel and (2) self-organized/assembled tissues (Figure 1). Both methods aim to create a biomimetic muscle microenvironment that provides cells with the appropriate extracellular matrix (ECM) and the biological and mechanical signals to promote rapid muscle development, maturation, and function.
Figure 1. Methods to engineered functional skeletal muscle. Schematic depicting various skeletal muscle models. (Top) Single fibers with satellite cells retained within their niche are isolated by enzymatic and mechanical dissociation. Single fibers can be cultured for up to 120 h and satellite cell activation assessed by evaluating Pax7 and MyoD expression or resistance to injury susceptibility assessed with laser-induced membrane damage. (Middle) Scaffold engineered muscles are created by embedding muscle progenitor cells at high density in a hydrogel. Cells remodel the hydrogel to generate 3D tissues packed with aligned functional myotubes. (Bottom) Scaffold-free engineered muscles created by seeding a monolayer of cells on laminin coated plates. The cells secrete sufficient ECM and progressive lift off the plate or “delaminate” and roll up to generate small 3D tissues. Both scaffold and scaffold-free tissues can be utilized for multiple assays with multiple functional output for up to 4 weeks.
The majority of engineered tissues are generated from three-dimensional hydrogels derived from natural ECM proteins such as collagen and fibrin. Hydrogels should: (1) provide a high surface area for cell adhesion, (2) provide mechanical support and/or topical guidance to maximize, (3) minimize diffusion distances, and (4) fully degrade once sufficient cell-derived ECM is deposited to generate densely packed muscle tissue supported by its own ECM. Hydrogel based tissues are typically formed from expanded MPCs that are embedded at high density within or on top of these hydrogels. The hydrogels are cast between two fixed anchor points that enable cellular forces to remodel the hydrogel and maintain the tissues under tension that promotes tissue alignment, rapid fusion, and muscle hypertrophy (Vandenburgh et al., 1988; Khodabukus et al., 2018; Wang J. et al., 2019).
The first 3D engineered tissues utilized Type I collagen hydrogels (Vandenburgh et al., 1988; Okano and Matsuda, 1997, 1998; Shansky et al., 1997; Powell et al., 2002), due to Type I collagen being the most abundant ECM protein in skeletal muscle (Kjaer, 2004). Whilst these studies initially utilized the C2C12 cell line, this method has successfully generated tissues from primary rodent (Vandenburgh et al., 2008; Lee and Vandenburgh, 2013) and human (Powell et al., 2002; Brady et al., 2008; Gholobova et al., 2015) cells. While collagen I is the most abundant ECM in skeletal muscle, excessive collagen levels are associated with poor regeneration and function of native muscle (Stearns-Reider et al., 2017). In native muscle, myofibers directly interact with the basal lamina which is rich in collagen IV and laminin but not type I collagen (Thorsteinsdottir et al., 2011). To better model native muscle structure, collagen hydrogels can be combined with MatrigelTM, a commercially available basal lamina extract isolated from murine Engelbreth-Holm-Swarm tumors, at the time of tissue formation. Collagen-matrigel hydrogels improve muscle structure but still generate lower contractile forces than fibrin based tissues and consequently are being used less frequently for studies measuring contractile function (Hinds et al., 2011; Sato et al., 2011).
Fibrin is the major structural component of blood clots that functions to first prevent bleeding and then be completely remodeled, resorbed, and replaced over time making it an ideal substrate for tissue engineering (Ahmed et al., 2008). The main disadvantage of fibrin is the significant lot-to-lot variability in tissue function and gel degradation rate which can be overcome by lot testing and regulation of fibrinolysis with cross-linkers and anti-fibrinolytics, respectively (Khodabukus and Baar, 2009). Tissues engineered from fibrin alone have specific contractile force generation significantly higher than collagen-matrigel tissues (Huang et al., 2005, 2006) and can be further enhanced by the addition of matrigel to generate engineered muscle tissues with the highest reported contractile function (Hinds et al., 2011; Juhas et al., 2014; Madden et al., 2015; Khodabukus et al., 2019). The increase in force generation is due in part to fibrin being several orders of magnitude less stiff than collagen (Collet et al., 2005; Yang et al., 2007) and fibrin gels having muscle-like stiffness (Chiron et al., 2012). Substrate stiffness is a key regulator of tissue dependent gene transcription programs (Engler et al., 2006), with muscle-like stiffness increasing myogenic gene expression and promoting muscle maturation (Engler et al., 2004).
An alternative approach to the use of hydrogels/scaffolds is to allow cells to secrete their own ECM and self-organize into a 3D tissue. The first self-organized engineered muscle used saran wrap substratum upon which MPCs were seeded with fibroblasts to ensure sufficient ECM deposition to enable tissue self-assembly (Strohman et al., 1990; Li et al., 2011). This model was then improved by seeding cells onto a PDMS substratum coated with laminin to support cell adhesion and reduce the number of fibroblasts required to generate sufficient ECM to support tissue formation (Dennis and Kosnik, 2000; Dennis et al., 2001; Kosnik et al., 2001; Larkin et al., 2006a, b). Further improvements to this model by utilizing aligned micropatterned surfaces to both quicken myoblast fusion and myotube alignment resulted in greater muscle differentiation (Lam et al., 2009). More recently, self-organized muscle cell sheets have been generated using the thermoresponsive polymer poly(N-isopropylacrylamide) (Takahashi and Okano, 2015; Takahashi et al., 2018). The cell sheets can be detached from culture plates by lowering temperature and used to engineer multi-layered tissue sheets comprised of muscle, vascular, or neuronal cells (Nagamori et al., 2013; Ngo et al., 2013; Takahashi et al., 2013). The benefits of these self-organized models are the tissues being encased entirely in cell secreted ECM and circumventing the lot variability of commercially available ECM proteins. However, the longer time to tissue formation and the inability of this model to easily be automated have resulted in hydrogel methods being more frequently used. A further limitation of self-assembled tissues is the small tissue size which hinders translational studies, though the ability to stack cell sheets or engraft multiple tissues together may overcome this issue.
Tissue engineered muscles replicate basic muscle contractile physiology such as force-length relationships and positive force-frequency relationships (Dennis and Kosnik, 2000; Huang et al., 2005; Hinds et al., 2011). However, their twitch:tetanus ratio, contractile kinetics (i.e., time to peak tension and half-relaxation time), and specific force generation are more consistent with embryonic and neonatal skeletal muscle values (Close, 1972). The developmentally immature contractile properties reflect the developmentally immature transcriptome and protein isoform expression seen in engineered tissues. While myotubes with engineered tissues have more mature gene expression and greater hypertrophy than myotubes cultured in 2D and undergo progressive hypertrophy in culture (Rao et al., 2018), even after 4 weeks of culture myotubes resemble developmentally immature or long-term denervated fibers (Kern et al., 2004). As discussed below, the incorporation of additional cell types and electro-mechanical stimulation can further increase muscle hypertrophy and contractile function. However, significant advances are required to generate adult-like engineered muscles within the shortened timeframe that would be most attractive to researchers.
Skeletal muscle has a remarkable level of plasticity that enables muscle to adapt to several physiological stressors such as changes in contractile activity, mechanical load, nutritional state, and hypoxia. Studying these processes in isolation in vivo is extremely difficult and confounding factors can hinder interpretation of the results. As discussed below, tissue-engineered skeletal muscle models have the potential to study many of these factors in isolation to not only better understand these mechanistic processes but to further enhance the maturation of engineered muscle tissues.
Skeletal muscle can be classified as type 1 slow-twitch (ST) or type 2 fast-twitch (FT) fiber types based on myosin heavy chain (MYH) isoform expression. While ST fibers express type I myosin (MYH7), FT fibers can be further classified into three subtypes, IIa (MYH1), IIx (MYH2), and IIb (MYH4), resulting in four fiber-type classifications. However, Type IIb fibers which possess the fastest contractile phenotype, are absent in human skeletal muscle resulting in humans only possessing three fiber-types (Schiaffino, 2010). During development and muscle regeneration, the embryonic (MYH3) and neonatal (MYH8) MYH isoforms are sequentially expressed before being down regulated and replaced by the adult MYH isoforms (Chargé and Rudnicki, 2004). Importantly, approximately 25% of muscle fibers are “hybrid” and express two or more MYH isoforms that arise to provide a functional continuum for optimal contractile performance or reflect transitionary states during development and regeneration (Medler, 2019). In addition to MYH isoform, slow and fast isoforms of multiple sarcomeric and calcium-handling proteins are found in a graded fashion in fiber-types to regulate speed of contraction. Functionally, slow contractile and calcium-handling isoforms result in slower contractile kinetics and importantly permit more energy efficient contraction by utilizing less ATP to generate equivalent contractile forces than fast muscle fibers (Bottinelli et al., 1994; Stienen et al., 1996). Additionally, ST fibers possess sarcomeric isoforms of titin, nebulin, and myomesin that contribute to the increased width of the z-disk and help to stabilize muscles during muscle contraction (Yamaguchi et al., 1985; Agarkova et al., 2004; Prado et al., 2005) (Figure 2). Functionally, these adaptations result in ST fibers being less prone to contraction-induced muscle damage (Choi and Widrick, 2010) and contribute to the increased disease severity of FT fibers seen in Duchenne muscle dystrophy (Webster et al., 1988). Lastly, type I and IIa fibers are characterized by high mitochondrial content, increased myoglobin levels, and high capillary density to maximize oxygen delivery to support more oxidative metabolism. Importantly, mitochondrial specialization occurs between fiber-types with mitochondria in ST fibers adapted to maximize fatty acid oxidization and reduced mitochondrial transition pore opening to prevent cell death due to the chronically elevated calcium levels in ST fibers (Picard et al., 2012). In contrast, mitochondria in FT fibers have a 10-fold greater ability to oxidize of glycerol-3-phosphate to help maintain a balanced redox state (Picard et al., 2012). ST fibers also have increased reactive oxygen species scavenging capacity due in part to increased antioxidant enzyme levels (Loureiro et al., 2016) and increased NADPH generation due to increased isocitrate dehydrogenase two expression and consequent regulation of the tricarboxylic acid cycle (Murgia et al., 2015).
Figure 2. Structural differences between slow and fast-fiber type muscles. (A) Schematic depicting gross morphological differences between slow and fast skeletal muscles. Note the higher levels of mitochondria and capillary density associated with slow skeletal muscles. (B) Sarcomeric gene specialization and enrichment in slow and fast myofibers.
Over the past 30 years, significant advances in elucidating the complex molecular and transcriptomic regulation of fiber-type have been achieved. A combination of epigenetic imprinting, neuronal activity, oxygen tension, environmental factors, and metabolic and hormonal influences regulate signaling pathway cascades and transcription factor activity (Hoppeler, 2016). A key regulator of fiber-type specification is intracellular calcium concentrations, with slow tonic motor neuron activity promoting sustained elevated calcium levels that activate calcineurin and calmodulin kinase (Meissner et al., 2001; Kubis et al., 2003). This activation then results in increased activity of NFAT (Swoap et al., 2000; Kubis et al., 2002, 2003; Mccullagh et al., 2004; Calabria et al., 2009; Ehlers et al., 2014) and MEF2 (Wu et al., 2000), and decreased class II HDAC activity (Potthoff et al., 2007) to promote slow sarcomeric and oxidative metabolism gene expression. The four isoforms of NFAT contribute to muscle fiber-type with NFATc1-4 expressed in ST fibers, NFATc2-4 expressed in type IIa fibers, and IIb fibers only expressing NFATc4 (Calabria et al., 2009). In addition to regulation by calcineurin, the transcription factor Prox1 increases expression of NFATc1-3 (Kivela et al., 2016), represses multiple members of the fast transcription program (Petchey et al., 2014), and is required for typical slow fiber distribution. Elevated calcium levels also promote mitochondrial biogenesis and oxidative phosphorylation via activation of the transcription factors PGC1α, PGC1β, PPARβ, and PPARδ (Jornayvaz and Shulman, 2010; Phua et al., 2018). Overexpression of PGC1α (Handschin et al., 2007; Rasbach et al., 2010; Summermatter et al., 2012), PPARβ (Schuler et al., 2006), and PPARδ (Wang et al., 2004) result in fast-slow fiber type and alter sarcomeric and calcium-handling gene expression demonstrating the interrelationship between metabolism, calcium-handling, and sarcomeric transcriptome. Additionally, overexpression of TEAD1, a member of the Hippo pathway, induces fast-to-slow fiber type conversions (Tsika et al., 2008). Conversely, a slow-to-fast transition occurs in the presence of thyroid hormone (Zhang et al., 2014) or deletion of VGLL2 (Honda et al., 2017) by disruption of TEAD1. Whilst the molecular transduction pathways leading to fast muscle fiber-types are less well characterized, genetic regulation of fast muscle fiber-type is well established. Fast muscle fiber neural input is linked to increased HIF1α content (Lunde et al., 2011), which is known to increase glycolytic gene expression, and decreased MyoD phosphorylation (Ekmark et al., 2007). MyoD is enriched in fast muscle fibers (Hughes et al., 1997) and repressed by the key slow-fiber type regulator NFATc1 (Ehlers et al., 2014) but its deletion only results in mild fiber-type switching suggesting that, whilst important, MyoD is not a master regulator of fast-fiber transcription. The master regulators of the fast muscle program are the Six1, Six4, Eya1 transcription factor complex which promote fast muscle gene expression (Grifone et al., 2004; Niro et al., 2010) and the transcriptional repressor Sox6 which inhibit slow muscle gene expression and increases fast muscle gene expression (Hagiwara et al., 2005, 2007; An et al., 2011; Quiat et al., 2011; Wang et al., 2011; Sakakibara et al., 2014). In humans, increases in fast muscle fiber-types are seen in donors with polymorphisms in HIF1α that increase HIF1α transcriptional activity (Ahmetov et al., 2008). Similarly, polymorphisms that decrease levels of the fast-fiber specific z-disk protein α-actinin3 are associated with increased calcineurin activity and endurance performance indicating that regulators of muscle fiber type can regulate human muscle performance (Yang et al., 2003; Eynon et al., 2012; Seto et al., 2013).
Complete muscle development and maintenance of adult muscle mass and fiber-type requires functional innervation. A key difference between slow and fast fiber-types is the motor input received with the frequency neural impulses differing between slow (10–30 Hz) and fast (50–100 Hz) muscle fibers (Hennig and Lomo, 1985; Eken et al., 2008). Additionally, slow fibers are active for greater periods of time and receive a far higher number of neural impulses per day than fast muscle fibers. The predominant role of neural input in regulating muscle fiber-type was first described in 1960 (Buller et al., 1960) and detailed in multiple elegant studies in the 1960s and 1970s (Close, 1969; Barany and Close, 1971; Hoh, 1975). Specifically, the contractile properties of slow muscles become faster when reinnervated by a fast nerve and fast muscles become slower when reinnervated by a slow nerve. The second series of studies used implanted electrodes to determine the role of stimulation frequency, work:rest ratio, and contractile impulse numbers in directing contractile kinetics and fiber-type shifts (Salmons and Sreter, 1976; Eberstein and Pachter, 1986; Eken and Gundersen, 1988; Gorza et al., 1988; Westgaard and Lomo, 1988; Windisch et al., 1998). The seminal finding of these studies is that neural input firing pattern is the predominant neural factor regulating muscle fiber-type and not secreted neurotrophic factors (Salmons and Sreter, 1976). Overall, these studies showed that increasing stimulation frequency, decreasing the work:rest ratio, and decreasing the total number of delivered impulses resulted in shifts to a faster-fiber type. Critically, both experimental models revealed that each individual muscle has an “adaptive range”, the degree of plasticity is species dependent, and long time periods (>3 months) are required for more definitive fiber-type changes. This adaptative range can be expanded by thyroid levels, with hypothyroidism and hyperthyroidism promoting greater slow and fast fiber-type shifts respectively (Kirschbaum et al., 1990; Caiozzo et al., 1998, 2000; Di Maso et al., 2000; Zhou et al., 2019). Overall, the typical adaptive ranges of mouse ST fibers permit expression of type I, IIa, and IIx but not IIB fibers, while mouse FT fibers can express Type IIa, IIx, and IIB fibers but not type I fibers.
The role of distinct neural inputs can be studied in culture by field electrical stimulation without potential confounding effects such as regenerative ability, compensatory hypertrophy, and animal locomotion. In 2D cultures, these studies have demonstrated that slow or fast-like stimulation patterns can increase isoform specific sarcomeric and calcium-handling proteins, and induce muscle hypertrophy (Wehrle et al., 1994; Hamalainen and Pette, 1997). The number of impulses and period of contractile activity must be regulated to prevent detachment of contracting myotubes in 2D culture. In contrast, in engineered muscle tissues the 3D environment supports tissue contraction and permits long-term electrical stimulation. However, long-term field electrical stimulation can induce electrochemical damage resulting in tissue damage and ultimately cell death. Electrochemical damage can be minimized by utilizing bipolar impulses and optimizing impulse parameters (i.e., electric field and pulse width) based on tissue excitability to enable adult-like long-term, chronic electrical stimulation without electrochemical damage for at least 2 weeks (Donnelly et al., 2010; Khodabukus and Baar, 2012, 2015c). Clinically, rheobase and chronaxie have been utilized to prevent tissue damaged in chronically denervated patients undergoing neuromuscular electrical stimulation (NMES) therapy (Pieber et al., 2015). The excitability of engineered tissues are similar to that of long-term denervated muscle and thus have potential to be used as an in vitro model to study factors regulating excitability and to screen factors that promote muscle excitability (Dennis and Dow, 2007; Khodabukus and Baar, 2012). In patients, NMES results in increased muscle cross-sectional area and tissue functionality in long-term denervated tissue (Boncompagni et al., 2007; Kern et al., 2010; Kern and Carraro, 2014; Carraro et al., 2015). In engineered muscle tissues, electrical stimulation increases force generation, muscle hypertrophy, and MHC and dystrophin protein levels in engineered tissues (Huang et al., 2006; Khodabukus and Baar, 2012, 2015c; Ito et al., 2014; Khodabukus et al., 2015, 2019). Like native muscle (Baar and Esser, 1999; Terzis et al., 2008; Drummond et al., 2009), these increases in muscle hypertrophy are associated with increased mTORC1 activity and can be inhibited by the mTOR inhibitor rapamycin. These studies also show that electrical stimulation of engineered muscle tissues for 1 or 2 weeks does not induce transformative changes in muscle size and that additional factors and/or time are required to attain adult muscle size.
To date, relatively few studies have determined the fiber-type impacts of different electrical stimulation protocols on engineered tissue function. The first study showed differential functional responses between muscles engineered from cells isolated from fast and slow muscles when electrically stimulated with biomimetic fiber-type neural input protocols (Huang et al., 2006). Specifically, engineered slow tissues increased force generation but did not alter their contractile kinetics in response to slow-fiber mimetic electrical stimulation. In contrast, slow-fiber mimetic stimulation in TA tissues did not increase force generation but did slow contractile kinetics. The fast fiber-type protocol did not change any parameters in either slow or fast engineered tissues, potentially due to the number of contractions being insufficient to induce functional changes or the lack of factors such as T3 which are critical for the fast-fiber type program. Human engineered tissues increase force generation and hypertrophy when subjected to a fast-like periodic intermittent electrical stimulation protocol with slow-like 1 or 10 Hz frequencies (Khodabukus et al., 2019). The 10 Hz but not the non-physiological 1 Hz protocol induced a quickening of half-relaxation time which was associated with increased fast CSQ and SERCA gene expression indicating that initial fiber-type shifts can be studied in human tissues. To date, the greatest fiber-type shift has been shown in C2C12 tissues where the role of contraction duration in inducing a slow fiber-type transition was defined (Khodabukus et al., 2015). When keeping the stimulation frequency, work:rest ratio, and total number of impulses received per day consistent, contraction lengths of greater than 6 s were required to induce more complete fast-to-slow MYH shifts. Fast-to-slow isoform switching of each troponin isoform, CSQ, and SERCA were independent of contraction length changes, suggesting differential regulation of MYH and other contractile proteins. These changes in protein abundance correlated to functional changes, with the slow electrical stimulation paradigm inducing slowing of contractile kinetics and increased fatigue resistance. Overall, these studies demonstrate the ability of engineered muscle tissues to study the fiber-type changes induced by electrical stimulation. However, while generation of slow fiber-types has been demonstrated, further optimization of the culture conditions and electrical stimulation protocols are required to generate fast fiber-type tissues.
While field electrical stimulation can mimic neural input, it does not faithfully recapitulate EC-coupling or model the complex physical and chemical interactions between muscle and nerves. Motor innervation is essential for complete muscle development and maintenance of muscle mass and defects in the neuromuscular junction (NMJ) result in multiple neuromuscular diseases such as myasthenia gravis (MG) and amyotrophic lateral sclerosis (ALS) (Rudolf et al., 2016; Cappello and Francolini, 2017). The first reports of NMJ formation were reported over 40 years in co-cultures of Xenopus myoblasts and embryonic neurons (Anderson and Cohen, 1977; Anderson et al., 1977). Similar studies utilizing rodent cells show markers of muscle maturation such as fetal to neonatal MHC isoform transitions, increased sarcomere structural maturation, and acetylcholine receptor (AchR) clustering (Das et al., 2007, 2010; Guo et al., 2010). However, in these studies AchR clustering does not co-localize with nerve terminals, as seen in embryonic development (Witzemann, 2006), the NMJs fail to replicate mature pretzel-like morphology, and no definitive evidence of electrical transmission provided suggesting incomplete NMJ development and maturation. In the past decade, substantial progress has been made in generating motor neuron-like progenitors from hiPSCs to permit studies utilizing human cells (Patani et al., 2011; Amoroso et al., 2013; Faravelli et al., 2014; Sances et al., 2016). These cells when co-cultured with primary or hiPSC-derived myotubes demonstrate AchR clustering but more importantly show functional NMJ formation (Demestre et al., 2015; Puttonen et al., 2015; Toli et al., 2015). Specifically, motor neuron-induced muscle contraction can be induced by specific motor neuron glutamatergic excitation by glutamate or N-Methyl-D-aspartate or inhibited by tubocurarine, an anti-cholinergic drug that prevents neural transmission (Figure 3E). Alternatively, muscle contraction can be induced optically by transduction of motor neurons with the light-sensitive ion channel channelrhodopsin2 (ChR2) (Steinbeck et al., 2016). Expression of ChR2 permits specific optical activation of motor neurons with blue light and subsequent myotube contraction if functional NMJs are present. Importantly, the use of hiPSCs allows the modeling of multiple human neuromuscular disease such as MG, spinal muscular atrophy (SMA), and ALS which would not be possible due to the post-mitotic state of adult motor neurons (Dimos et al., 2008; Ebert et al., 2009; Sances et al., 2016).
Figure 3. Tissue-engineered neuromuscular junction (NMJ) models. (A) Traditional co-culture NMJ models where single MNs or neurospheres are embedded with muscle cells at the time of tissue formation. (B) Representative image of the co-culture NMJ model (Afshar Bakooshli et al., 2019) with immunofluorescent staining of neurite extensions (SMI32), acetylcholine receptors [α-bungarotoxin (BTX)] and muscle sarcomeres [sarcomeric α-actinin (SAA)]. Scale bar, 200μm. (C) Modular NMJ muscle model where motor neurons (MNs) and engineered muscle tissues are cultured in separate compartments and connected by a collagen gel which supports neurite extension. (D) Representative image of the modular NMJ model (Osaki et al., 2018b) with immunofluorescent staining of neurite extensions (TUJ1) and myotubes [filamentous(f)-actin]. Scale bars, 100 μm. (E) Schematic depicting pharmacological and genetic methods to assess neuromuscular junction (NMJ) functionality in vitro.
The first 3D tissue engineered muscle motor-neuron co-cultures utilized neonatal rat MPCs and embryonic spinal cord explants (Larkin et al., 2006b). Neural projections from the explants extended into the engineered muscle and preserved their structure to enable neural specific stimulation of the resulting tissue. Spinal explants increased force generation, ∼25% of which could be achieved by direct neural stimulation, indicating incomplete innervation of all myotubes and/or the presence of non-functional NMJs. Mixing of rat motor neurons with rat MPCs at time of tissue formation also results in improved contractile force and muscle structure, though the level of functional NMJ generation was not tested (Martin et al., 2015). Incorporation of hiPSC-derived neurospheres, that contain motor neurons and other supporting cell types, into primary human engineered muscle tissues results in successful generation of innervated myotubes as assessed by glutamate stimulation (Afshar Bakooshli et al., 2019) (Figures 3A,B). Neurosphere co-culture also resulted in shifts to more developmentally mature AchR subunit expression and more adult-like AchR structure then that seen in 2D cultures. While motor neuron-muscle co-cultures clearly increase functionality and muscle structure, it is still unclear what effects are due to increased contractile activity and secreted neurotrophic factors. The synaptogenic factor agrin can induce AchR formation, AchR clustering, and increase force generation almost 2-fold (Bian and Bursac, 2012). Similarly, the neurotrophic factor neuregulin-1 induced AchR subunit isoform maturation though its role in regulating muscle function unknown (Afshar Bakooshli et al., 2019). The most recent models to study NMJ formation in 3D tissues utilize neural cells and engineered muscle tissues in two distinct modular compartments (Figures 3C,D). These compartments are then attached by a collagen gel bridge that supports and guides neural cell migration and neurite extension into the engineered muscle tissue. Importantly, specific activation of the neural compartment can induced optogenetically by overexpression of ChR2 and engineered muscle contractile function assessed by pillar deformation (Uzel et al., 2016; Osaki et al., 2018b; Vila et al., 2019). Thus in the presence of functional NMJ formation, optical stimulation of the neural compartment induces contraction of the engineered muscle compartment. These models have been used to successfully model impaired NMJ function in ALS and MG (Osaki et al., 2018b; Afshar Bakooshli et al., 2019; Vila et al., 2019) and to identify and validate prospective clinical drug candidates such as rapamycin and bosutinib for ALS (Osaki et al., 2018b). Overall, significant progress has been made with innervation of engineered muscle tissues over the past 5 years and they possess great potential as more biomimetic models for muscle disease and development. However, significant improvements in NMJ maturation are still required as well as more detailed assessment of NMJ electrophysiology, structure, and function.
A fundamental question underlying muscle plasticity is if the satellite cells that make typically slow (e.g., SOL) or fast (e.g., tibialis anterior) muscle fibers are distinct and predisposed to generate a specific fiber-type. As discussed above, the adaptive ranges of muscle fibers suggest that fiber-type is predetermined. Slow fiber-type muscles contain a higher level of satellite cells than fast fiber type tissues (Gibson and Schultz, 1982; Putman et al., 2001; Mackey et al., 2009). This difference is further exacerbated with aging due to a greater loss of SCs in fast muscle fibers (Verdijk et al., 2007, 2014), which correlates to the greater loss of function seen in fast muscles in sarcopenia (Deschenes et al., 2013; Purves-Smith et al., 2014). Satellite cells isolated from slow-fiber type muscles have increased transplantation efficiency and thus are likely more translationally relevant for future SC transplantation therapies (Collins et al., 2005). The existence of intrinsic differences between satellite cells from fast and slow muscles is further suggested by the finding that electrical stimulation of regenerating slow SOL and fast EDL muscles with the same slow stimulus pattern in the absence of innervation leads to widespread slow MYH expression in regenerated SOL but only limited expression of slow MYH in regenerated EDL (Kalhovde et al., 2005). Additionally, the altered MYH isoform profile of single fibers isolated from long-term denervated slow and fast-fiber type muscles remain distinct and do not converge to similar MYH isoform expression (Patterson et al., 2006). Traditional 2D cell cultures of cells isolated from slow and fast muscles show preferential expression of fiber-type MYH expression and displayed expected adaptive ranges to fiber-like electrical stimulation (Wehrle et al., 1994; Rosenblatt et al., 1996; DiMario and Stockdale, 1997). In 3D culture, muscles engineered from MPCs isolated from rats (Huang et al., 2006) or mice (Khodabukus and Baar, 2015a) retain the contractile and metabolic properties of the muscles from which they were derived. Specifically, expected isoform shifts in multiple sarcomeric, calcium-handling, and metabolic proteins are seen. However, due to the lack of neural input these shifts are less distinct than seen in innervated adult muscle fibers and more closely resemble long-term denervated myofibers. Interestingly, these changes are linked to changes in transcriptional master regulators such as Sox6 which is more abundant in myotubes derived from fast muscles (Khodabukus and Baar, 2015a). Overall, these studies demonstrate that the satellite cells generating fast and slow muscles are distinct and result in long-term differences in metabolism and function both in vitro and in vivo.
Skeletal muscle function and size is dependent upon the load placed upon it as evidenced by loss of muscle mass and strength following immobilization, bed rest, spaceflight (Haddad et al., 2006; Baldwin et al., 2013), and gain of muscle mass and function with resistance exercise (Hughes et al., 2018; Jorgenson et al., 2020). Mechanical load is modeled in vitro by the application of stretch to tissue cultures using deformable membranes (Sim et al., 2007; Pavesi et al., 2015) or custom-made bioreactors (Dennis et al., 2009; Rangarajan et al., 2014) to induce programmable levels of stretch in custom-made regimes. Most studies utilize custom-made systems and unique stimulation protocols that hinder comparisons between studies. To date, in vitro mechanical stimulations of engineered muscle have aimed to replicate: (1) the continual increase in muscle length seen during development (ramp stretch/stimulation); and; (2) muscle strain that occurs during locomotion and exercise (cyclic stretch/stimulation). Ramp stretch results in concomitant proliferation and differentiation of MPCs (Vandenburgh and Karlisch, 1989) which may be due in part to the secretion of the IGF-1 splice variant mechanogrowth factor (Cheema et al., 2005). Application of ramp stretch for only 45 h induces significant muscle hypertrophy and increases in contractile force generation in C2C12 engineered tissues (Aguilar-Agon et al., 2019). Applying both ramp and cyclic stretch induced significant myotube hypertrophy in engineered human tissues (Powell et al., 2002) and increased glucose uptake in avian tissues (Hatfaludy et al., 1989). The seminal in vitro work of Vandenburgh showed that cyclical stretch induces muscle hypertrophy and increases protein and DNA content (Vandenburgh and Kaufman, 1979, 1981; Vandenburgh, 1982, 1988; Vandenburgh et al., 1989, 1991). Like native muscle following resistance exercise or altered mechanical load (Baar and Esser, 1999; Terzis et al., 2008; Marabita et al., 2016), mechanical stimulation induced hypertrophy of in vitro cultures is associated with mTORC1 activation (Baar et al., 2000; Khodabukus et al., 2007; Aguilar-Agon et al., 2019). Conversely, muscle atrophy can be modeled by decreasing engineered tissue length to induce decreases in myotube size and loss of contractile force (Lee and Vandenburgh, 2013). Together, these studies demonstrate that mechanical loading/unloading can model aspects of muscle hypertrophy/atrophy and be used a model system to study these processes. Similar to electrical stimulation studies, no transformative breakthroughs in generating engineered tissues with native-like muscle size or strength have been reported with use of mechanical stimulation alone or in combination with electrical stimulation (Liao et al., 2008).
A key difference between fiber-types is the greater reliance of fast-fiber type muscles on glycolysis and slow-fiber-type muscle on oxidative phosphorylation to meet energy needs. Muscles engineered from fast twitch muscle fibers have increased levels of glycolytic enzymes and fatigue at a greater rate than tissues generated from slow twitch muscle fibers (Khodabukus and Baar, 2015a). Engineered C2C12 tissues cultured with supraphysiological glucose levels promotes a fast fiber-type contractile and metabolic phenotype, permits detectable levels of the fast-fiber specific protein parvalbumin, and increased levels of glycolytic proteins (Khodabukus and Baar, 2015b). Whilst promising, accurate modeling and studying of oxidative metabolism in vitro is hindered by the Crabtree effect where in vitro cultured cells typically utilize glycolysis to generate ATP despite the presence of abundant oxygen and functional mitochondria (Crabtree, 1929; Marroquin et al., 2007). The Crabtree effect can be circumvented by replacing glucose in the cell culture media with galactose which forces cells to rely on oxidative phosphorylation to meet their energy demands due to galactose requiring 2 molecules of ATP to generate pyruvate and thus producing 0 net ATP from anaerobic glycolysis (Marroquin et al., 2007; Ryall et al., 2015). Galactose culture of primary human myotubes increases mitochondrial enzyme levels, increases basal and maximal oxygen consumption, and increased phosphorylation of AMPK (Aguer et al., 2011) – which when chronically activated results in a fast-to-slow fiber-type transition (Ljubicic et al., 2011). Importantly, these adaptations to galactose culture conditions were not seen in myotubes derived from type II diabetics suggesting that true assessment of metabolic dysfunction in diabetic myotubes may require novel culture conditions (Aguer et al., 2011). In contrast, C2C12 cells do not respond to galactose culture conditions indicating that modeling metabolism in immortalized cell lines should be treated with caution (Elkalaf et al., 2013). Alternatively, supplementing culture media with fatty acids has the potential to promote oxidative metabolism and potentially promote a slow fiber-type phenotype. However, in vitro cultures are prone to lipotoxicity due to the low reliance on oxidative phosphorylation and β-oxidation to meet energy demands. In agreement with this, the saturated fatty acid palmitate is known to induce atrophy (Bryner et al., 2012), insulin resistance (Yuzefovych et al., 2010), and cell death (Patkova et al., 2014) in myotubes. Addition of polyunsaturated fatty acids (PUFAs) such as oleic, linoleic, docosahexaenoic, and eicosapentaenoic acid can reduce or prevent these negative cellular effects of palmitate (Bryner et al., 2012; Yuzefovych et al., 2012; Lee et al., 2017; Woodworth-Hobbs et al., 2017). Recently, oleic acid alone was shown to increase MYH7 protein levels, mitochondrial mass, and increase maximal respiration rate in human myotubes supporting the potential role of fatty acids in promoting fiber-type shifts (Watanabe et al., 2020). In contrast, promoting a fast fiber-type in vitro has proven to be more difficult due in part to the fact that fast MYH expression occurs in more mature muscles cultures (Cooper et al., 2004). Lactate can increase fast MYH (MYH 1 and 4) gene expression (Tsukamoto et al., 2018) and thyroid hormone upregulates fast SERCA isoform expression (Thelen et al., 1997). Overall, these studies show that fiber-type shifts can be studied in vitro by modulation of fuel source and hormone availability but advances in generating fast fiber-type changes are still required.
Native skeletal muscle is highly vascularized to provide the oxygen and nutrient supply required to support the high metabolic demands induced by muscle contraction. Hypoxia and impaired cell survival typically occur at a diffusion distance of 150–200μm from blood vessels or in culture media and limits tissue-engineered muscle size (Gholobova et al., 2020a). Satellite cell fate and muscle regeneration is also regulated by vasculature (Abou-Khalil et al., 2009, 2010; Fu et al., 2015), with 50-80% of satellite cells are in near or direct contact with capillaries (Verma et al., 2018). Therefore, vascularized tissue-engineered skeletal muscles are required for accurate modeling of native skeletal muscle in vitro and effective cellular therapies in vivo.
Vascularization of tissue-engineered skeletal muscles has typically been performed by the incorporation of vascular cells at the time of tissue formation. A key technical limitation with vascularized engineered muscle tissues are the incompatible media requirements of muscle and vascular cells (Gholobova et al., 2015). This has led to compromised media conditions that result in suboptimal differentiation of muscle and/or vascular cells than if cultured in isolation. A second technical limitation of co-culturing muscle and vascular cells is the potential for vascular cells to impede myoblast fusion. This issue can be overcome by 3D bioprinting techniques (Choi et al., 2019), the stacking of muscle-only and vascular-only cell sheets (Nagamori et al., 2013), culturing vascular and muscle cells in distinct compartments (Osaki et al., 2018a), or the coating of vascular cells to differentiated mature muscle tissues (Gholobova et al., 2020b). The formation of stable vasculature requires the inclusion of supporting cell types such as fibroblasts, pericytes, and/or smooth muscle cells (Levenberg et al., 2005; Koffler et al., 2011; Perry et al., 2017). Implantation of pre-vascularized engineered muscle tissues with these supporting cell types accelerates vascular anastomosis, increases blood vessel density, and tissue survival compared to co-cultures alone. While the benefit of forming vascular networks for implantation survival is well established, to date no studies have shown increased muscle function, increased muscle maturation, or enhanced SC quiescence in co-cultured tissues in vitro. Additionally, the ability of these vascular networks to enhance nutrient delivery and increase engineered muscle size in vitro have yet to be shown.
Over the last century, small animal models have been the predominant experimental platform to study disease by genetic manipulations, surgical procedures, or other interventions such as changes made to diet and aging. These studies have given us the majority of the insight we have into the underlying mechanisms of disease and for identifying and pre-clinical validation of novel therapeutics. When assessing all drugs entering clinical trials for all diseases, the successful clinical translation is alarmingly low – with less than 1% of identified drugs making it to the clinic resulting in an estimated cost of $1 billion dollars per drug (Hay et al., 2014). The underlying reasons for this low level of success are multifactorial but include species-specific differences in drug metabolism and toxicity (Dykens and Will, 2007; Shen et al., 2011), animal models not fully recapitulating disease severity in humans (Yucel et al., 2018), animals not accurately modeling human pharmacogenomics (Weinshilboum and Wang, 2017), and epigenetic regulation of disease severity (Lamar and Mcnally, 2014). The ability to generate functional human tissue-engineered organs has potential to address some of the limitations of animal studies and provide complementary methods to improve successful drug clinical translation.
Duchenne muscular dystrophy (DMD) is a fatal X-linked recessive disorder that affects approximately 1 in 5000 male births due to mutations in the dystrophin gene. Dystrophin is part of the dystrophin-glycoprotein complex (DGC) that functions to efficiently transmit contractile force to the ECM and stabilize the sarcolemmal membrane during contraction to prevent muscle damage. The increased susceptibility to muscle injury due to compromised membrane stability and impaired regenerative response of satellite cells results in constant cycles of muscle degeneration and regeneration. Ultimately, this leads to progressive muscle weakness, loss of ambulation, and fatal respiratory failure by the age of 20. Currently, there is no curative treatment with standard-of-care corticosteroid (prednisone and deflazacort) therapy extending life expectancy up to the fourth decade by delaying disease progression (Bushby et al., 2010).
The most widespread animal model of DMD, the mdx mouse model, arose due to spontaneous mutation in exon 23 on the dystrophin gene in C57/BL10 mice. Like DMD patients, mdx muscles undergo consistent rounds of degeneration and regeneration, display increased susceptibility to eccentric contractions, and display abnormalities in SC function. However, these mice do not show more severe disease phenotypes such as severe muscle weakness, early mortality, cardiac dysfunction and only display significant fat and fibrotic replacement at old age (20–24 months) (Yucel et al., 2018; Aartsma-Rus and Van Putten, 2019). In the past two decades, additional dystrophin-deficient mice have been generated on the C57/BL6 (e.g., mdxcv2–5) and on the DBA/2J backgrounds (Yucel et al., 2018; Aartsma-Rus and Van Putten, 2019). The mdxcv2–5 mice have mutations in a range of exons but still demonstrate the lack of functional weakness seen in traditional mdx model. In contrast, the hDMDdel45D2/mdx mouse displays a stronger dystrophic phenotype and displays contractile weakness potentially improving the efficacy of pharmacological therapeutic validation studies (Coley et al., 2016; Young et al., 2017; Van Putten et al., 2019). Mouse models that more accurately replicate human disease severity have required knockout of both dystrophin and utrophin (Deconinck et al., 1997; Grady et al., 1997). These double knockout mice show severe functional and regenerative defects in skeletal muscle, cardiac, and bone tissues. Alternatively, knocking out the telomerase gene in mdx mice (mdx/mTR) induces a more severe muscle (Sacco et al., 2010) and cardiac (Mourkioti et al., 2013) disease phenotype. Together, these double knockout mice provide more severe disease models with which to study dystrophin deficiency and the efficacy of novel therapeutics. Advances in genome editing have permitted the generation of transgenic mice that model disease mutations in human hotspots and allow the testing of gene therapies in vivo. However, these mice do not replicate the significant role that disease modifiers play in disease severity for DMD. Multiple genetic and epigenetic modifiers have been shown to significantly influence disease severity and corticosteroid efficacy (Pegoraro et al., 2011; Flanigan et al., 2013; Lamar and Mcnally, 2014; Bello et al., 2015). For example, latent TGF-β binding protein 4 (LTBP4) and osteopontin, which modulate TGF-β signaling, significantly impact disease progression and efficacy of corticosteroid treatment (Bello et al., 2015), and inhibition of TGF-β signaling reversing disease phenotypes in vitro and in vivo (Choi et al., 2016). Therefore, high throughput personalized in vitro muscle platforms that accurately model pharmacogenomic responses will be required to generate high fidelity and clinically predictive drug screens. The mdx mouse model was used as the preclinical system to validate the current standard of care drugs prednisolone (Hudecki et al., 1993) and deflazacort (Anderson et al., 1996). More recently, these models have been used to validate the antisense oligonucleotide treatments eteplirsen (Mendell et al., 2013) which was approved by the FDA in 2016 (Mendell et al., 2013; Stein, 2016; Khan et al., 2019). The use of more severe mdx models in combination with high fidelity human in vitro models may lead to more successful clinical translation and drug discovery efforts.
To date, two 3D in vitro skeletal muscle models of DMD have been reported using primary cells. The first utilized immortalized dystrophic mouse myoblasts and identified 11 compounds that increased contractile force generation (Vandenburgh et al., 2008). More recently, engineered muscle sheets derived from human primary DMD myoblasts demonstrated decreased fusion ability, decreased myotube size, and produced less force compared to healthy controls after 6 days in differentiation media (Nesmith et al., 2016). Whilst promising, these studies did not describe more mature disease markers such as evidence of degeneration/regeneration, fibrosis or fat replacement or increased susceptibility to contraction-induced injury that will be critical to study DMD pathology and treatment efficacy in vitro. Widespread in vitro personalized medicine models of DMD or any other myopathy will require the use of hiPSC-derived muscle due to the ethical considerations and proliferative limitations of taking muscle biopsies from myopathic patients. To date, the majority of hiPSC studies of DMD have been performed in 2D cell culture. These studies have demonstrated disease phenotypes such as calcium overload (Shoji et al., 2015), fusion deficits, increased creatine kinase release (Young et al., 2016), and elevated BMP/TGF-β signaling (Choi et al., 2016). Interestingly, fusion deficits are not always seen in hiPSC cultures and appear to be dependent upon media conditions or cell surface marker selection that potentially prevent fusion deficits (Hicks et al., 2018). Recently, a high throughput drug screen in 2D iPSC-derived myotubes identified ginsenoside and fenofibrate as factors that improved hiPSC myoblast fusion in vitro and improved mdx morphology and function in vitro (Sun et al., 2020). In addition to pharmaceutical screening, engineered tissues can be used to optimize guide RNA design for highly efficient and maximal functional return for CRISPR-Cas9 mediated exon skipping in engineered DMD hiPSC-derived cardiac tissues (Long et al., 2018). A further advantage of hiPSC cell models is the ability to generate increasingly complex disease models by the addition of multiple cell types. In particular, the NMJ pathophysiology in DMD is poorly studied and has the potential to be studied in hiPSC-derived tissues. The generation of multi-cellular 3D muscle tissues holds promise for studying complex tissue interactions in healthy and diseased states (Maffioletti et al., 2018; Mazaleyrat et al., 2020). Overall, the iPSC-derived disease models have significant potential for generating personalized medicine platforms, disease specific drug screening, and studying pathogenic cellular or organ-organ crosstalk in a modular fashion.
Skeletal muscle regenerative capacity can be overwhelmed by extensive muscle loss seen with trauma, blast injuries, and surgical resection. In animal models, this is typically modeled by the surgical removal of 20-40% of muscle mass (Sicari et al., 2014; Corona et al., 2017; Quarta et al., 2017). This level of muscle loss results in irrecoverable loss of muscle function and mass with fibrotic replacement of the lost muscle tissue (Corona et al., 2016). In contrast, other injury models such as snake venom, ischemia-reperfusion, crush, and repeated eccentric contractions in healthy skeletal muscle typically result in return of function 1–2 months post injury. The incomplete muscle regeneration in VML injuries is likely due to the ablation of muscle fibers and their associated SCs, the loss of ECM and associated biochemical and mechanical guidance cues, and the extensive and prolonged inflammatory response to VML injury (Greising et al., 2017; Aguilar et al., 2018; Corona et al., 2018; Larouche et al., 2018). The only current clinical option for VML, autologous tissue transfer, is ineffective due to high donor site morbidity and graft failure. Recent clinical trials utilizing decellularized ECM, which promote vascularization and an anti-inflammatory healing response, have shown limited therapeutic efficacy due to the lack of significant de novo muscle formation necessitating novel therapeutic approaches (Sicari et al., 2014).
Injection of satellite cells alone into VML injury models does not support muscle regeneration, highlighting the need for a satellite cell niche and myofiber guidance for successful regenerative outcomes (Quarta et al., 2017). In non-VML injury models, successful transplantation of SCs is increased by delivering SCs within a native-like niche such as native myofiber fragments (Marg et al., 2014) or tissue engineered muscle constructs (Juhas et al., 2014). In VML injury models, injection of satellite cells in combination with muscle resident cells (which include endothelial cells and mesenchymal progenitors) attached to artificially engineered muscle fiber scaffold results in retention of SCs and functional regeneration of the injured muscle (Quarta et al., 2017). Incorporating additional cell types such as endothelial (Levenberg et al., 2005; Koffler et al., 2011; Perry et al., 2017; Choi et al., 2019), neuronal (Das et al., 2020; Kim et al., 2020), and immune (Juhas et al., 2018) cells improves the survival of transplanted 3D engineered tissues due a combination of cellular recruitment and paracrine signaling to promote vascular and neural integration. The key limitation in the clinical use of the engineered tissue replacements is neural integration with the host. Implantation of engineered muscle tissues and incorporation of the host nerve into the implant increases implant contractile function and maturation compared to time-matched in vitro controls (Borschel et al., 2006; Dhawan et al., 2007; Williams et al., 2013; VanDusen et al., 2014; Adams et al., 2017). In these studies, AchR clustering and primitive NMJ synaptogenesis can occur in as little as 7 days post-implantation but functional integration with the host system was only seen 3 months post-implantation (Urbanchek et al., 2016). Encouragingly, muscle innervation after VML injury treated with satellite cell-containing construct was significantly enhanced with exercise (Quarta et al., 2017), suggesting a possibility that engineered muscle innervation could be optimized through physical therapy. Importantly, the incorporation of exercise following implantation of these tissues promotes improved muscle recovery and host innervation of the resulting muscle fibers (Quarta et al., 2017) – the key limitation in translating engineered muscle to the clinic. Transplantation of engineered muscle tissues results in their initial degeneration due to the loss of nutrients and hypoxia. Generation of tissues that regenerate in vitro not only enables the study of muscle regeneration in controlled conditions but also correlates to tissue survival upon implantation (Juhas et al., 2014, 2018). Tissues generated from adult rat MPCs fail to regenerate in vitro but the addition of macrophages supports in vitro regeneration and increased regenerative ability and functionality upon implantation in vivo (Juhas et al., 2018).
The recent advances and progress made in tissue engineering more biomimetic muscle tissues has provided researchers a novel model to complement traditional 2D cell culture and animal models. Here, we have discussed the utility of these engineered tissues to study muscle physiology, regeneration, exercise, and disease. However, the highest functioning engineered muscle tissues have the functionality of neonatal muscle tissue and do not possess the developmental maturity of adult skeletal muscle (Juhas et al., 2014; Khodabukus et al., 2019). Given that full mouse and human muscle maturation requires 3 months and 18 years respectively, methods to rapidly mature engineered muscle tissues are required. Supraphysiological electrical stimulation of engineered hiPSC cardiac tissues accelerates development to achieve adult-like transcriptomic signatures and mitochondrial levels in 4 weeks (Ronaldson-Bouchard et al., 2018). However, the resulting contractile function was inferior to the highest reported in the field demonstrating incomplete tissue maturation and that paradoxically high tissue function does not always equate to greater maturation. For skeletal muscle, the combined use of electrical and mechanical stimulation, functional innervation, small molecules, and appropriately timed biochemical signals will be required to generate more developmentally mature tissues.
For engineered muscle tissues to be more widely utilized, a shift to serum-free culture conditions is also required to increase reproducibility and permit clinical translation of cellular therapies. This is highlighted by the fact that geographical origin of serum can impact the contractile kinetics and isoform expression of calcium-handling proteins in engineered tissues (Khodabukus and Baar, 2014). Furthermore, batch variations in serum, matrigel, and fibrinogen induce significant functional variations, further adding to reproducibility issues in and between laboratories (Khodabukus and Baar, 2009). The use of serum-free differentiation media utilizing commercially available serum-free supplements such as Ultroser-G (Gawlitta et al., 2007; Fujita et al., 2010) or N-2 (Rao et al., 2018; Khodabukus et al., 2020) have demonstrated the muscle function and structure can be maintained or even improved with serum-free supplements. Further advances will also be required in the generation of more physiologically relevant basal media to provide physiologically relevant levels of saccharides, TCA derivatives, metabolites, and hormones (Cantor et al., 2017). Similarly, the use of synthetic or recombinant extracellular matrices will also be required to improve reproducibility, improve toxicity studies, and for successful clinical translation of cellular or engineered tissue therapies (Nguyen et al., 2017). Lastly, the combinatorial use of small molecules can also be used to enhance muscle function (Selvaraj et al., 2019) but the ability to use these tissues for additional drug screening may be limited due to toxicity issues related to high concentrations organic solvents often used as solvent vehicles.
The majority of studies on engineered muscle tissue function are derived of cultures only containing muscle and contaminating or added fibroblasts. While this enables the ability to isolate muscle-specific effects, native muscle tissue is comprised of multiple cell types that are required for complete muscle development and function. The incorporation of motor neurons (Osaki et al., 2018b; Afshar Bakooshli et al., 2019; Vila et al., 2019), sensory neuron (Colon et al., 2017; Guo et al., 2017), vascular (Levenberg et al., 2005; Perry et al., 2017), immune (Juhas et al., 2018), and other supporting cell types will be required to generate more biomimetic engineered muscles. Furthermore, the ability to generate multiple tissue types and to couple them together will enable researchers to study organ-organ crosstalk in a highly controlled and regulated environment. These multi-organ or human-on-a-chip systems are required for more physiologically relevant drug screens and the identification of unexpected drug toxicity (Maschmeyer et al., 2015; Oleaga et al., 2016; Skardal et al., 2017; Vernetti et al., 2017). For drug screening purposes, more high throughput systems such as 96 well plate platforms using pillar deflection methods to record force generation are required (Vandenburgh et al., 2008; Mills et al., 2019). Additionally, identifying culture conditions that prevent the Crabtree effect and promote the use of oxidative phosphorylation to meet energy demands are required to improve tissue maturation and better assay mitochondrial toxicity – the most common factor for drug toxicity (Dykens and Will, 2007; Marroquin et al., 2007). Lastly, generation of clinically relevant muscle tissues for VML injuries will require the combination of all factors discussed above and novel approaches to promote rapid neuronal and vascular host integration. Specifically, the use of small molecules (Ko et al., 2013; Quarta et al., 2016), innovative engineering and/or surgical techniques (Borschel et al., 2006; Kim et al., 2015; Al-Himdani et al., 2017), and physical rehabilitation therapy (Quarta et al., 2017) in conjunction with novel methods to generate viable human size tissues are essential for VML therapies.
Overall, tissue engineered muscle systems are a powerful tool to study skeletal muscle function, development, plasticity, and disease. These systems can be used for stand-alone studies, add functional translational components, or be used to supplement in vivo studies as 2D systems have been for decades. Future advances in tissue maturation, generation of more complex heterocellular tissues, and coupling to other organ systems will generate improved models to study muscle disease and increase the chance of identifying novel biological and pharmaceutical therapeutics.
AK wrote, edited, and generated figures for the entire manuscript.
This work was supported by the NIH Grants AR055226 and AR065873 from National Institute of Arthritis and Musculoskeletal and Skin Disease, grant UG3TR002142 from the NIH Common Fund for the Microphysiological Systems Initiative, the Jain foundation, and Duke COPE funding.
The author declares that the research was conducted in the absence of any commercial or financial relationships that could be construed as a potential conflict of interest.
Figures were created with Biorender.com.
Aartsma-Rus, A., and Van Putten, M. (2019). The use of genetically humanized animal models for personalized medicine approaches. Dis. Model Mech. 13:dmm041673. doi: 10.1242/dmm.041673
Abou-Khalil, R., Le Grand, F., Pallafacchina, G., Valable, S., Authier, F. J., Rudnicki, M. A., et al. (2009). Autocrine and paracrine angiopoietin 1/Tie-2 signaling promotes muscle satellite cell self-renewal. Cell Stem. Cell 5, 298–309. doi: 10.1016/j.stem.2009.06.001
Abou-Khalil, R., Mounier, R., and Chazaud, B. (2010). Regulation of myogenic stem cell behavior by vessel cells: the “menage a trois” of satellite cells, periendothelial cells and endothelial cells. Cell Cycle 9, 892–896. doi: 10.4161/cc.9.5.10851
Adams, A. M., Vandusen, K. W., Kostrominova, T. Y., Mertens, J. P., and Larkin, L. M. (2017). Scaffoldless tissue-engineered nerve conduit promotes peripheral nerve regeneration and functional recovery after tibial nerve injury in rats. Neural. Regen. Res. 12, 1529–1537. doi: 10.4103/1673-5374.215265
Afshar Bakooshli, M., Lippmann, E. S., Mulcahy, B., Iyer, N., Nguyen, C. T., Tung, K., et al. (2019). A 3D culture model of innervated human skeletal muscle enables studies of the adult neuromuscular junction. Elife 8:e44530.
Agarkova, I., Schoenauer, R., Ehler, E., Carlsson, L., Carlsson, E., Thornell, L. E., et al. (2004). The molecular composition of the sarcomeric M-band correlates with muscle fiber type. Eur. J. Cell Biol. 83, 193–204. doi: 10.1078/0171-9335-00383
Aguer, C., Gambarotta, D., Mailloux, R. J., Moffat, C., Dent, R., Mcpherson, R., et al. (2011). Galactose enhances oxidative metabolism and reveals mitochondrial dysfunction in human primary muscle cells. PLoS One 6:e28536. doi: 10.1371/journal.pone.0028536
Aguilar, C. A., Greising, S. M., Watts, A., Goldman, S. M., Peragallo, C., Zook, C., et al. (2018). Multiscale analysis of a regenerative therapy for treatment of volumetric muscle loss injury. Cell Death Discov. 4:33.
Aguilar-Agon, K. W., Capel, A. J., Martin, N. R. W., Player, D. J., and Lewis, M. P. (2019). Mechanical loading stimulates hypertrophy in tissue-engineered skeletal muscle: Molecular and phenotypic responses. J. Cell Physiol. 234, 23547–23558. doi: 10.1002/jcp.28923
Ahmed, T. A., Dare, E. V., and Hincke, M. (2008). Fibrin: a versatile scaffold for tissue engineering applications. Tissue Eng. Part B. Rev. 14, 199–215. doi: 10.1089/ten.teb.2007.0435
Ahmetov, I. I., Hakimullina, A. M., Lyubaeva, E. V., Vinogradova, O. L., and Rogozkin, V. A. (2008). Effect of HIF1A gene polymorphism on human muscle performance. Bull. Exp. Biol. Med. 146, 351–353. doi: 10.1007/s10517-008-0291-3
Al-Himdani, S., Jessop, Z. M., Al-Sabah, A., Combellack, E., Ibrahim, A., Doak, S. H., et al. (2017). Tissue-engineered solutions in plastic and reconstructive surgery: principles and practice. Front. Surg. 4:4.
Allen, D. G., Lannergren, J., and Westerblad, H. (1997). The role of ATP in the regulation of intracellular Ca2+ release in single fibres of mouse skeletal muscle. J. Physiol. 498(Pt 3), 587–600. doi: 10.1113/jphysiol.1997.sp021885
Al-Qusairi, L., Weiss, N., Toussaint, A., Berbey, C., Messaddeq, N., Kretz, C., et al. (2009). T-tubule disorganization and defective excitation-contraction coupling in muscle fibers lacking myotubularin lipid phosphatase. Proc. Natl. Acad. Sci. U.S.A. 106, 18763–18768. doi: 10.1073/pnas.0900705106
Amoroso, M. W., Croft, G. F., Williams, D. J., Keeffe, S., Carrasco, M. A., Davis, A. R., et al. (2013). Accelerated high-yield generation of limb-innervating motor neurons from human stem cells. J. Neurosci. 33:574. doi: 10.1523/jneurosci.0906-12.2013
An, C. I., Dong, Y., and Hagiwara, N. (2011). Genome-wide mapping of Sox6 binding sites in skeletal muscle reveals both direct and indirect regulation of muscle terminal differentiation by Sox6. BMC Dev. Biol. 11:59. doi: 10.1186/1471-213x-11-59
Anderson, J. E., Mcintosh, L. M., and Poettcker, R. (1996). Deflazacort but not prednisone improves both muscle repair and fiber growth in diaphragm and limb muscle in vivo in the mdx dystrophic mouse. Muscle Nerve 19, 1576–1585. doi: 10.1002/(sici)1097-4598(199612)19:12<1576::aid-mus7>3.0.co;2-7
Anderson, M. J., and Cohen, M. W. (1977). Nerve-induced and spontaneous redistribution of acetylcholine receptors on cultured muscle cells. J. Physiol. 268, 757–773. doi: 10.1113/jphysiol.1977.sp011880
Anderson, M. J., Cohen, M. W., and Zorychta, E. (1977). Effects of innervation on the distribution of acetylcholine receptors on cultured muscle cells. J. Physiol. 268, 731–756. doi: 10.1113/jphysiol.1977.sp011879
Baar, K., and Esser, K. (1999). Phosphorylation of p70(S6k) correlates with increased skeletal muscle mass following resistance exercise. Am. J. Physiol. 276, C120–C127.
Baar, K., Torgan, C. E., Kraus, W. E., and Esser, K. (2000). Autocrine phosphorylation of p70(S6k) in response to acute stretch in myotubes. Mol. Cell Biol. Res. Commun. 4, 76–80. doi: 10.1006/mcbr.2000.0257
Baldwin, K. M., Haddad, F., Pandorf, C. E., Roy, R. R., and Edgerton, V. R. (2013). Alterations in muscle mass and contractile phenotype in response to unloading models: role of transcriptional/pretranslational mechanisms. Front. Physiol. 4:284.
Bansal, D., Miyake, K., Vogel, S. S., Groh, S., Chen, C. C., Williamson, R., et al. (2003). Defective membrane repair in dysferlin-deficient muscular dystrophy. Nature 423, 168–172. doi: 10.1038/nature01573
Barany, M., and Close, R. I. (1971). The transformation of myosin in cross-innervated rat muscles. J. Physiol. 213, 455–474. doi: 10.1113/jphysiol.1971.sp009393
Beauchamp, J. R., Heslop, L., Yu, D. S., Tajbakhsh, S., Kelly, R. G., Wernig, A., et al. (2000). Expression of CD34 and Myf5 defines the majority of quiescent adult skeletal muscle satellite cells. J. Cell Biol. 151, 1221–1234. doi: 10.1083/jcb.151.6.1221
Bello, L., Kesari, A., Gordish-Dressman, H., Cnaan, A., Morgenroth, L. P., Punetha, J., et al. (2015). Genetic modifiers of ambulation in the cooperative international neuromuscular research group duchenne natural history study. Ann. Neurol. 77, 684–696. doi: 10.1002/ana.24370
Bian, W., and Bursac, N. (2012). Soluble miniagrin enhances contractile function of engineered skeletal muscle. FASEB J. 26, 955–965. doi: 10.1096/fj.11-187575
Boncompagni, S., Kern, H., Rossini, K., Hofer, C., Mayr, W., Carraro, U., et al. (2007). Structural differentiation of skeletal muscle fibers in the absence of innervation in humans. Proc. Natl. Acad. Sci. U.S.A. 104, 19339–19344. doi: 10.1073/pnas.0709061104
Borschel, G. H., Dow, D. E., Dennis, R. G., and Brown, D. L. (2006). Tissue-engineered axially vascularized contractile skeletal muscle. Plast. Reconstr. Surg. 117, 2235–2242. doi: 10.1097/01.prs.0000224295.54073.49
Bottinelli, R., Canepari, M., Pellegrino, M. A., and Reggiani, C. (1996). Force-velocity properties of human skeletal muscle fibres: myosin heavy chain isoform and temperature dependence. J. Physiol. 495, 573–586. doi: 10.1113/jphysiol.1996.sp021617
Bottinelli, R., Canepari, M., Reggiani, C., and Stienen, G. J. (1994). Myofibrillar ATPase activity during isometric contraction and isomyosin composition in rat single skinned muscle fibres. J. Physiol. 481(Pt 3), 663–675. doi: 10.1113/jphysiol.1994.sp020472
Brady, M. A., Lewis, M. P., and Mudera, V. (2008). Synergy between myogenic and non-myogenic cells in a 3D tissue-engineered craniofacial skeletal muscle construct. J. Tissue Eng. Regen. Med. 2, 408–417. doi: 10.1002/term.112
Brooks, S. V., and Faulkner, J. A. (1988). Contractile properties of skeletal muscles from young, adult and aged mice. J. Physiol. 404, 71–82. doi: 10.1113/jphysiol.1988.sp017279
Bryner, R. W., Woodworth-Hobbs, M. E., Williamson, D. L., and Alway, S. E. (2012). Docosahexaenoic acid protects muscle cells from palmitate-induced atrophy. ISRN Obes 2012:647348.
Buller, A. J., Eccles, J. C., and Eccles, R. M. (1960). Interactions between motoneurones and muscles in respect of the characteristic speeds of their responses. J. Physiol. 150, 417–439. doi: 10.1113/jphysiol.1960.sp006395
Bushby, K., Finkel, R., Birnkrant, D. J., Case, L. E., Clemens, P. R., Cripe, L., et al. (2010). Diagnosis and management of Duchenne muscular dystrophy, part 1: diagnosis, and pharmacological and psychosocial management. Lancet Neurol. 9, 77–93. doi: 10.1016/s1474-4422(09)70271-6
Caiozzo, V. J., Baker, M. J., and Baldwin, K. M. (1998). Novel transitions in MHC isoforms: separate and combined effects of thyroid hormone and mechanical unloading. J. Appl. Physiol. (1985) 85, 2237–2248. doi: 10.1152/jappl.1998.85.6.2237
Caiozzo, V. J., Haddad, F., Baker, M., Mccue, S., and Baldwin, K. M. (2000). MHC polymorphism in rodent plantaris muscle: effects of mechanical overload and hypothyroidism. Am. J. Physiol. Cell Physiol. 278, C709–C717.
Calabria, E., Ciciliot, S., Moretti, I., Garcia, M., Picard, A., Dyar, K. A., et al. (2009). NFAT isoforms control activity-dependent muscle fiber type specification. Proc. Natl. Acad. Sci. U.S.A. 106, 13335–13340. doi: 10.1073/pnas.0812911106
Cantor, J. R., Abu-Remaileh, M., Kanarek, N., Freinkman, E., Gao, X., and Louissaint, A. Jr., et al. (2017). Physiologic medium rewires cellular metabolism and reveals uric acid as an endogenous inhibitor of UMP synthase. Cell 169:e217.
Cappello, V., and Francolini, M. (2017). Neuromuscular junction dismantling in amyotrophic lateral sclerosis. Int. J. Mol. Sci. 18:2092. doi: 10.3390/ijms18102092
Carraro, U., Boncompagni, S., Gobbo, V., Rossini, K., Zampieri, S., Mosole, S., et al. (2015). Persistent muscle fiber regeneration in long term denervation. Past, Present, Future. Eur. J. Transl. Myol. 25:4832.
Castorena, C. M., Arias, E. B., Sharma, N., Bogan, J. S., and Cartee, G. D. (2015). Fiber type effects on contraction-stimulated glucose uptake and GLUT4 abundance in single fibers from rat skeletal muscle. Am. J. Physiol. Endocrinol. Metab. 308, E223–E230.
Chargé, S. B. P., and Rudnicki, M. A. (2004). Cellular and molecular regulation of muscle regeneration. Physiol. Rev. 84, 209–238. doi: 10.1152/physrev.00019.2003
Charville, G. W., Cheung, T. H., Yoo, B., Santos, P. J., Lee, G. K., Shrager, J. B., et al. (2015). Ex vivo expansion and in vivo self-renewal of human muscle stem cells. Stem. Cell Rep. 5, 621–632. doi: 10.1016/j.stemcr.2015.08.004
Cheema, U., Brown, R., Mudera, V., Yang, S. Y., Mcgrouther, G., and Goldspink, G. (2005). Mechanical signals and IGF-I gene splicing in vitro in relation to development of skeletal muscle. J. Cell Physiol. 202, 67–75. doi: 10.1002/jcp.20107
Chiron, S., Tomczak, C., Duperray, A., Laine, J., Bonne, G., Eder, A., et al. (2012). Complex interactions between human myoblasts and the surrounding 3D fibrin-based matrix. PLoS One 7:e36173. doi: 10.1371/journal.pone.0036173
Choi, I. Y., Lim, H., Estrellas, K., Mula, J., Cohen, T. V., Zhang, Y., et al. (2016). Concordant but varied phenotypes among duchenne muscular dystrophy patient-specific myoblasts derived using a human iPSC-based model. Cell Rep. 15, 2301–2312. doi: 10.1016/j.celrep.2016.05.016
Choi, S. J., and Widrick, J. J. (2010). Calcium-activated force of human muscle fibers following a standardized eccentric contraction. Am. J. Physiol. Cell Physiol. 299, C1409–C1417.
Choi, Y. J., Jun, Y. J., Kim, D. Y., Yi, H. G., Chae, S. H., Kang, J., et al. (2019). A 3D cell printed muscle construct with tissue-derived bioink for the treatment of volumetric muscle loss. Biomaterials 206, 160–169. doi: 10.1016/j.biomaterials.2019.03.036
Close, R. (1969). Dynamic properties of fast and slow skeletal muscles of the rat after nerve cross-union. J. Physiol. 204, 331–346. doi: 10.1113/jphysiol.1969.sp008916
Close, R. I. (1972). Dynamic properties of mammalian skeletal muscles. Physiol. Rev. 52, 129–197. doi: 10.1152/physrev.1972.52.1.129
Coley, W. D., Bogdanik, L., Vila, M. C., Yu, Q., Van Der Meulen, J. H., Rayavarapu, S., et al. (2016). Effect of genetic background on the dystrophic phenotype in mdx mice. Hum. Mol. Genet. 25, 130–145.
Collet, J. P., Shuman, H., Ledger, R. E., Lee, S., and Weisel, J. W. (2005). The elasticity of an individual fibrin fiber in a clot. Proc. Natl. Acad. Sci. U.S.A. 102, 9133–9137. doi: 10.1073/pnas.0504120102
Collins, C. A., Olsen, I., Zammit, P. S., Heslop, L., Petrie, A., Partridge, T. A., et al. (2005). Stem cell function, self-renewal, and behavioral heterogeneity of cells from the adult muscle satellite cell niche. Cell 122, 289–301. doi: 10.1016/j.cell.2005.05.010
Colon, A., Guo, X., Akanda, N., Cai, Y., and Hickman, J. J. (2017). Functional analysis of human intrafusal fiber innervation by human gamma-motoneurons. Sci. Rep. 7:17202.
Cooper, S. T., Maxwell, A. L., Kizana, E., Ghoddusi, M., Hardeman, E. C., Alexander, I. E., et al. (2004). C2C12 co-culture on a fibroblast substratum enables sustained survival of contractile, highly differentiated myotubes with peripheral nuclei and adult fast myosin expression. Cell Motil. Cytoskeleton 58, 200–211. doi: 10.1002/cm.20010
Corona, B. T., Henderson, B. E., Ward, C. L., and Greising, S. M. (2017). Contribution of minced muscle graft progenitor cells to muscle fiber formation after volumetric muscle loss injury in wild-type and immune deficient mice. Physiol. Rep. 5:e13249. doi: 10.14814/phy2.13249
Corona, B. T., Rivera, J. C., and Greising, S. M. (2018). Inflammatory and physiological consequences of debridement of fibrous tissue after volumetric muscle loss injury. Clin. Transl. Sci. 11, 208–217. doi: 10.1111/cts.12519
Corona, B. T., Wenke, J. C., and Ward, C. L. (2016). Pathophysiology of Volumetric muscle loss injury. Cells Tissues Organs 202, 180–188. doi: 10.1159/000443925
Crabtree, H. G. (1929). Observations on the carbohydrate metabolism of tumours. Biochem. J. 23, 536–545. doi: 10.1042/bj0230536
Das, M., Rumsey, J. W., Bhargava, N., Stancescu, M., and Hickman, J. J. (2010). A defined long-term in vitro tissue engineered model of neuromuscular junctions. Biomaterials 31, 4880–4888. doi: 10.1016/j.biomaterials.2010.02.055
Das, M., Rumsey, J. W., Gregory, C. A., Bhargava, N., Kang, J. F., Molnar, P., et al. (2007). Embryonic motoneuron-skeletal muscle co-culture in a defined system. Neuroscience 146, 481–488. doi: 10.1016/j.neuroscience.2007.01.068
Das, S., Browne, K. D., Laimo, F. A., Maggiore, J. C., Hilman, M. C., Kaisaier, H., et al. (2020). Pre-innervated tissue-engineered muscle promotes a pro-regenerative microenvironment following volumetric muscle loss. Commun. Biol. 3:330.
Deconinck, A. E., Rafael, J. A., Skinner, J. A., Brown, S. C., Potter, A. C., Metzinger, L., et al. (1997). Utrophin-dystrophin-deficient mice as a model for Duchenne muscular dystrophy. Cell 90, 717–727. doi: 10.1016/s0092-8674(00)80532-2
Demestre, M., Orth, M., Fohr, K. J., Achberger, K., Ludolph, A. C., Liebau, S., et al. (2015). Formation and characterisation of neuromuscular junctions between hiPSC derived motoneurons and myotubes. Stem. Cell Res. 15, 328–336. doi: 10.1016/j.scr.2015.07.005
Dennis, R. G., and Dow, D. E. (2007). Excitability of skeletal muscle during development, denervation, and tissue culture. Tissue Eng. 13, 2395–2404. doi: 10.1089/ten.2006.0367
Dennis, R. G., and Kosnik, P. E. II (2000). Excitability and isometric contractile properties of mammalian skeletal muscle constructs engineered in vitro. In Vitro Cell Dev. Biol. Anim. 36, 327–335.
Dennis, R. G., Kosnik, P. E. II, Gilbert, M. E., and Faulkner, J. A. (2001). Excitability and contractility of skeletal muscle engineered from primary cultures and cell lines. Am. J. Physiol. Cell Physiol. 280, C288–C295. doi: 10.1290/1071-2690(2000)036<0327:eaicpo>2.0.co;2
Dennis, R. G., Smith, B., Philp, A., Donnelly, K., and Baar, K. (2009). Bioreactors for guiding muscle tissue growth and development. Adv. Biochem. Eng. Biotechnol. 112, 39–79. doi: 10.1007/978-3-540-69357-4_3
Deschenes, M. R., Gaertner, J. R., and O’reilly, S. (2013). The effects of sarcopenia on muscles with different recruitment patterns and myofiber profiles. Curr. Aging Sci. 6, 266–272. doi: 10.2174/18746098113066660035
Dhawan, V., Lytle, I. F., Dow, D. E., Huang, Y. C., and Brown, D. L. (2007). Neurotization improves contractile forces of tissue-engineered skeletal muscle. Tissue Eng. 13, 2813–2821. doi: 10.1089/ten.2007.0003
Di Maso, N. A., Caiozzo, V. J., and Baldwin, K. M. (2000). Single-fiber myosin heavy chain polymorphism during postnatal development: modulation by hypothyroidism. Am. J. Physiol. Regul. Integr. Comp. Physiol. 278, R1099–R1106.
DiMario, J. X., and Stockdale, F. E. (1997). Both myoblast lineage and innervation determine fiber type and are required for expression of the slow myosin heavy chain 2 gene. Dev. Biol. 188, 167–180. doi: 10.1006/dbio.1997.8619
Dimos, J. T., Rodolfa, K. T., Niakan, K. K., Weisenthal, L. M., Mitsumoto, H., Chung, W., et al. (2008). Induced pluripotent stem cells generated from patients with ALS can be differentiated into motor neurons. Science 321, 1218–1221. doi: 10.1126/science.1158799
Donnelly, K., Khodabukus, A., Philp, A., Deldicque, L., Dennis, R. G., and Baar, K. (2010). A novel bioreactor for stimulating skeletal muscle in vitro. Tissue Eng. Part C Methods 16, 711–718. doi: 10.1089/ten.tec.2009.0125
Drummond, M. J., Fry, C. S., Glynn, E. L., Dreyer, H. C., Dhanani, S., Timmerman, K. L., et al. (2009). Rapamycin administration in humans blocks the contraction-induced increase in skeletal muscle protein synthesis. J. Physiol. 587, 1535–1546. doi: 10.1113/jphysiol.2008.163816
Dumont, N. A., Wang, Y. X., Von Maltzahn, J., Pasut, A., Bentzinger, C. F., Brun, C. E., et al. (2015). Dystrophin expression in muscle stem cells regulates their polarity and asymmetric division. Nat. Med. 21, 1455–1463. doi: 10.1038/nm.3990
Dykens, J. A., and Will, Y. (2007). The significance of mitochondrial toxicity testing in drug development. Drug Discov. Today 12, 777–785. doi: 10.1016/j.drudis.2007.07.013
Eberstein, A., and Pachter, B. R. (1986). The effect of electrical stimulation on reinnervation of rat muscle: contractile properties and endplate morphometry. Brain Res. 384, 304–310. doi: 10.1016/0006-8993(86)91166-2
Ebert, A. D., Yu, J., Rose, F. F. Jr., Mattis, V. B., Lorson, C. L., Thomson, J. A., et al. (2009). Induced pluripotent stem cells from a spinal muscular atrophy patient. Nature 457, 277–280. doi: 10.1038/nature07677
Ehlers, M. L., Celona, B., and Black, B. L. (2014). NFATc1 controls skeletal muscle fiber type and is a negative regulator of MyoD activity. Cell Rep. 8, 1639–1648. doi: 10.1016/j.celrep.2014.08.035
Eken, T., Elder, G. C., and Lomo, T. (2008). Development of tonic firing behavior in rat soleus muscle. J. Neurophysiol. 99, 1899–1905. doi: 10.1152/jn.00834.2007
Eken, T., and Gundersen, K. (1988). Electrical stimulation resembling normal motor-unit activity: effects on denervated fast and slow rat muscles. J. Physiol. 402, 651–669. doi: 10.1113/jphysiol.1988.sp017227
Ekmark, M., Rana, Z. A., Stewart, G., Hardie, D. G., and Gundersen, K. (2007). De-phosphorylation of MyoD is linking nerve-evoked activity to fast myosin heavy chain expression in rodent adult skeletal muscle. J. Physiol. 584, 637–650. doi: 10.1113/jphysiol.2007.141457
Elkalaf, M., Andel, M., and Trnka, J. (2013). Low glucose but not galactose enhances oxidative mitochondrial metabolism in C2C12 myoblasts and myotubes. PLoS One 8:e70772. doi: 10.1371/journal.pone.0070772
Engler, A. J., Griffin, M. A., Sen, S., Bonnemann, C. G., Sweeney, H. L., and Discher, D. E. (2004). Myotubes differentiate optimally on substrates with tissue-like stiffness: pathological implications for soft or stiff microenvironments. J. Cell Biol. 166, 877–887. doi: 10.1083/jcb.200405004
Engler, A. J., Sen, S., Sweeney, H. L., and Discher, D. E. (2006). Matrix elasticity directs stem cell lineage specification. Cell 126, 677–689. doi: 10.1016/j.cell.2006.06.044
Eynon, N., Ruiz, J. R., Femia, P., Pushkarev, V. P., Cieszczyk, P., Maciejewska-Karlowska, A., et al. (2012). The ACTN3 R577X polymorphism across three groups of elite male European athletes. PLoS One 7:e43132. doi: 10.1371/journal.pone.0043132
Faravelli, I., Bucchia, M., Rinchetti, P., Nizzardo, M., Simone, C., Frattini, E., et al. (2014). Motor neuron derivation from human embryonic and induced pluripotent stem cells: experimental approaches and clinical perspectives. Stem Cell Res. Ther. 5:87. doi: 10.1186/scrt476
Flanigan, K. M., Ceco, E., Lamar, K. M., Kaminoh, Y., Dunn, D. M., Mendell, J. R., et al. (2013). LTBP4 genotype predicts age of ambulatory loss in Duchenne muscular dystrophy. Ann. Neurol. 73, 481–488.
Fu, X., Wang, H., and Hu, P. (2015). Stem cell activation in skeletal muscle regeneration. Cell Mol. Life Sci. 72, 1663–1677. doi: 10.1007/s00018-014-1819-5
Fujita, H., Endo, A., Shimizu, K., and Nagamori, E. (2010). Evaluation of serum-free differentiation conditions for C2C12 myoblast cells assessed as to active tension generation capability. Biotechnol. Bioeng. 107, 894–901. doi: 10.1002/bit.22865
Gawlitta, D., Boonen, K. J., Oomens, C. W., Baaijens, F. P., and Bouten, C. V. (2007). The influence of serum-free culture conditions on skeletal muscle differentiation in a tissue-engineered model. Tissue Eng. 14, 161–171. doi: 10.1089/ten.2007.0095
Gharaibeh, B., Lu, A., Tebbets, J., Zheng, B., Feduska, J., Crisan, M., et al. (2008). Isolation of a slowly adhering cell fraction containing stem cells from murine skeletal muscle by the preplate technique. Nat. Protoc. 3, 1501–1509. doi: 10.1038/nprot.2008.142
Gholobova, D., Decroix, L., Van Muylder, V., Desender, L., Gerard, M., Carpentier, G., et al. (2015). Endothelial network formation within human tissue-engineered skeletal muscle. Tissue Eng. Part A 21, 2548–2558. doi: 10.1089/ten.tea.2015.0093
Gholobova, D., Terrie, L., Gerard, M., Declercq, H., and Thorrez, L. (2020a). Vascularization of tissue-engineered skeletal muscle constructs. Biomaterials 235:119708. doi: 10.1016/j.biomaterials.2019.119708
Gholobova, D., Terrie, L., Mackova, K., Desender, L., Carpentier, G., Gerard, M., et al. (2020b). Functional evaluation of prevascularization in one-stage versus two-stage tissue engineering approach of human bio-artificial muscle. Biofabrication 12:035021. doi: 10.1088/1758-5090/ab8f36
Gibson, M. C., and Schultz, E. (1982). The distribution of satellite cells and their relationship to specific fiber types in soleus and extensor digitorum longus muscles. Anat. Rec. 202, 329–337. doi: 10.1002/ar.1092020305
Gilbert, P. M., Havenstrite, K. L., Magnusson, K. E., Sacco, A., Leonardi, N. A., Kraft, P., et al. (2010). Substrate elasticity regulates skeletal muscle stem cell self-renewal in culture. Science 329, 1078–1081. doi: 10.1126/science.1191035
Gorza, L., Gundersen, K., Lomo, T., Schiaffino, S., and Westgaard, R. H. (1988). Slow-to-fast transformation of denervated soleus muscles by chronic high-frequency stimulation in the rat. J. Physiol. 402, 627–649. doi: 10.1113/jphysiol.1988.sp017226
Grady, R. M., Teng, H., Nichol, M. C., Cunningham, J. C., Wilkinson, R. S., and Sanes, J. R. (1997). Skeletal and cardiac myopathies in mice lacking utrophin and dystrophin: a model for Duchenne muscular dystrophy. Cell 90, 729–738. doi: 10.1016/s0092-8674(00)80533-4
Greising, S. M., Rivera, J. C., Goldman, S. M., Watts, A., Aguilar, C. A., and Corona, B. T. (2017). Unwavering pathobiology of volumetric muscle loss injury. Sci. Rep. 7:13179.
Grifone, R., Laclef, C., Spitz, F., Lopez, S., Demignon, J., Guidotti, J. E., et al. (2004). Six1 and Eya1 expression can reprogram adult muscle from the slow-twitch phenotype into the fast-twitch phenotype. Mol. Cell Biol. 24, 6253–6267. doi: 10.1128/mcb.24.14.6253-6267.2004
Guo, X., Colon, A., Akanda, N., Spradling, S., Stancescu, M., Martin, C., et al. (2017). Tissue engineering the mechanosensory circuit of the stretch reflex arc with human stem cells: Sensory neuron innervation of intrafusal muscle fibers. Biomaterials 122, 179–187. doi: 10.1016/j.biomaterials.2017.01.005
Guo, X., Das, M., Rumsey, J., Gonzalez, M., Stancescu, M., and Hickman, J. (2010). Neuromuscular junction formation between human stem-cell-derived motoneurons and rat skeletal muscle in a defined system. Tissue Eng. Part C Methods 16, 1347–1355. doi: 10.1089/ten.tec.2010.0040
Haddad, F., Adams, G. R., Bodell, P. W., and Baldwin, K. M. (2006). Isometric resistance exercise fails to counteract skeletal muscle atrophy processes during the initial stages of unloading. J. Appl. Physiol. 100, 433–441. doi: 10.1152/japplphysiol.01203.2005
Hagiwara, N., Ma, B., and Ly, A. (2005). Slow and fast fiber isoform gene expression is systematically altered in skeletal muscle of the Sox6 mutant, p100H. Dev. Dyn. 234, 301–311. doi: 10.1002/dvdy.20535
Hagiwara, N., Yeh, M., and Liu, A. (2007). Sox6 is required for normal fiber type differentiation of fetal skeletal muscle in mice. Dev. Dyn. 236, 2062–2076. doi: 10.1002/dvdy.21223
Hamalainen, N., and Pette, D. (1997). Coordinated fast-to-slow transitions of myosin and SERCA isoforms in chronically stimulated muscles of euthyroid and hyperthyroid rabbits. J. Muscle Res. Cell Motil. 18, 545–554.
Handschin, C., Chin, S., Li, P., Liu, F., Maratos-Flier, E., Lebrasseur, N. K., et al. (2007). Skeletal muscle fiber-type switching, exercise intolerance, and myopathy in PGC-1alpha muscle-specific knock-out animals. J. Biol. Chem. 282, 30014–30021. doi: 10.1074/jbc.m704817200
Hansen, P. A., Gulve, E. A., and Holloszy, J. O. (1994). Suitability of 2-deoxyglucose for in vitro measurement of glucose transport activity in skeletal muscle. J. Appl. Physiol. (1985) 76, 979–985. doi: 10.1152/jappl.1994.76.2.979
Harridge, S. D., Bottinelli, R., Canepari, M., Pellegrino, M. A., Reggiani, C., Esbjornsson, M., et al. (1996). Whole-muscle and single-fibre contractile properties and myosin heavy chain isoforms in humans. Pflugers Arch. 432, 913–920. doi: 10.1007/s004240050215
Hatfaludy, S., Shansky, J., and Vandenburgh, H. H. (1989). Metabolic alterations induced in cultured skeletal muscle by stretch-relaxation activity. Am. J. Physiol. 256, C175–C181.
Hay, M., Thomas, D. W., Craighead, J. L., Economides, C., and Rosenthal, J. (2014). Clinical development success rates for investigational drugs. Nat. Biotechnol. 32, 40–51. doi: 10.1038/nbt.2786
Hennig, R., and Lomo, T. (1985). Firing patterns of motor units in normal rats. Nature 314, 164–166. doi: 10.1038/314164a0
Hicks, M. R., Hiserodt, J., Paras, K., Fujiwara, W., Eskin, A., Jan, M., et al. (2018). ERBB3 and NGFR mark a distinct skeletal muscle progenitor cell in human development and hPSCs. Nat. Cell Biol. 20, 46–57. doi: 10.1038/s41556-017-0010-2
Hinds, S., Bian, W., Dennis, R. G., and Bursac, N. (2011). The role of extracellular matrix composition in structure and function of bioengineered skeletal muscle. Biomaterials 32, 3575–3583. doi: 10.1016/j.biomaterials.2011.01.062
Hoh, J. F. (1975). Selective and non-selective reinnervation of fast-twitch and slow-twitch rat skeletal muscle. J. Physiol. 251, 791–801. doi: 10.1113/jphysiol.1975.sp011122
Honda, M., Hidaka, K., Fukada, S. I., Sugawa, R., Shirai, M., Ikawa, M., et al. (2017). Vestigial-like 2 contributes to normal muscle fiber type distribution in mice. Sci. Rep. 7:7168.
Hoppeler, H. (2016). Molecular networks in skeletal muscle plasticity. J. Exp. Biol. 219, 205–213. doi: 10.1242/jeb.128207
Huang, Y. C., Dennis, R. G., and Baar, K. (2006). Cultured slow vs. fast skeletal muscle cells differ in physiology and responsiveness to stimulation. Am. J. Physiol. Cell Physiol. 291, C11–C17.
Huang, Y. C., Dennis, R. G., Larkin, L., and Baar, K. (2005). Rapid formation of functional muscle in vitro using fibrin gels. J. Appl. Physiol. 98, 706–713. doi: 10.1152/japplphysiol.00273.2004
Hudecki, M. S., Pollina, C. M., Granchelli, J. A., Daly, M. K., Byrnes, T., Wang, J. C., et al. (1993). Strength and endurance in the therapeutic evaluation of prednisolone-treated MDX mice. Res. Commun. Chem. Pathol Pharmacol. 79, 45–60.
Hughes, D. C., Ellefsen, S., and Baar, K. (2018). Adaptations to endurance and strength training. Cold Spring Harb. Perspect. Med. 8:a029769.
Hughes, S. M., Koishi, K., Rudnicki, M., and Maggs, A. M. (1997). MyoD protein is differentially accumulated in fast and slow skeletal muscle fibres and required for normal fibre type balance in rodents. Mech. Dev. 61, 151–163. doi: 10.1016/s0925-4773(96)00631-4
Ito, A., Yamamoto, Y., Sato, M., Ikeda, K., Yamamoto, M., Fujita, H., et al. (2014). Induction of functional tissue-engineered skeletal muscle constructs by defined electrical stimulation. Sci. Rep. 4:4781.
Jorgenson, K. W., Phillips, S. M., and Hornberger, T. A. (2020). Identifying the structural adaptations that drive the mechanical load-induced growth of skeletal muscle: a scoping review. Cells 9:1658. doi: 10.3390/cells9071658
Jornayvaz, F. R., and Shulman, G. I. (2010). Regulation of mitochondrial biogenesis. Essays Biochem. 47, 69–84.
Juhas, M., Abutaleb, N., Wang, J. T., Ye, J., Shaikh, Z., Sriworarat, C., et al. (2018). Incorporation of macrophages into engineered skeletal muscle enables enhanced muscle regeneration. Nat. Biomed. Eng. 2, 942–954. doi: 10.1038/s41551-018-0290-2
Juhas, M., Engelmayr, G. C. Jr., Fontanella, A. N., Palmer, G. M., and Bursac, N. (2014). Biomimetic engineered muscle with capacity for vascular integration and functional maturation in vivo. Proc. Natl. Acad. Sci. U.S.A. 111, 5508–5513. doi: 10.1073/pnas.1402723111
Kalhovde, J. M., Jerkovic, R., Sefland, I., Cordonnier, C., Calabria, E., Schiaffino, S., et al. (2005). “Fast” and “slow” muscle fibres in hindlimb muscles of adult rats regenerate from intrinsically different satellite cells. J. Physiol. 562, 847–857. doi: 10.1113/jphysiol.2004.073684
Kern, H., Boncompagni, S., Rossini, K., Mayr, W., Fano, G., Zanin, M. E., et al. (2004). Long-term denervation in humans causes degeneration of both contractile and excitation-contraction coupling apparatus, which is reversible by functional electrical stimulation (FES): a role for myofiber regeneration? J. Neuropathol. Exp. Neurol. 63, 919–931. doi: 10.1093/jnen/63.9.919
Kern, H., and Carraro, U. (2014). Home-based functional electrical stimulation for long-term denervated human muscle: history, basics, results and perspectives of the vienna rehabilitation strategy. Eur. J. Transl. Myol. 24:3296.
Kern, H., Carraro, U., Adami, N., Biral, D., Hofer, C., Forstner, C., et al. (2010). Home-based functional electrical stimulation rescues permanently denervated muscles in paraplegic patients with complete lower motor neuron lesion. Neurorehabil. Neural. Repair. 24, 709–721. doi: 10.1177/1545968310366129
Kerr, J. P., Ziman, A. P., Mueller, A. L., Muriel, J. M., Kleinhans-Welte, E., Gumerson, J. D., et al. (2013). Dysferlin stabilizes stress-induced Ca2+ signaling in the transverse tubule membrane. Proc. Natl. Acad. Sci. U.S.A. 110, 20831–20836. doi: 10.1073/pnas.1307960110
Khan, N., Eliopoulos, H., Han, L., Kinane, T. B., Lowes, L. P., Mendell, J. R., et al. (2019). Eteplirsen treatment attenuates respiratory decline in ambulatory and non-ambulatory patients with duchenne muscular dystrophy. J. Neuromuscul. Dis. 6, 213–225. doi: 10.3233/jnd-180351
Khodabukus, A., and Baar, K. (2009). Regulating fibrinolysis to engineer skeletal muscle from the C2C12 Cell Line. Tissue Eng. Part C Methods 15, 501–511. doi: 10.1089/ten.tec.2008.0286
Khodabukus, A., and Baar, K. (2012). Defined electrical stimulation emphasizing excitability for the development and testing of engineered skeletal muscle. Tissue Eng. Part C Methods 18, 349–357. doi: 10.1089/ten.tec.2011.0364
Khodabukus, A., and Baar, K. (2014). The effect of serum origin on tissue engineered skeletal muscle function. J. Cell Biochem. 115, 2198–2207. doi: 10.1002/jcb.24938
Khodabukus, A., and Baar, K. (2015a). Contractile and metabolic properties of engineered skeletal muscle derived from slow and fast phenotype mouse muscle. J. Cell Physiol. 230, 1750–1757. doi: 10.1002/jcp.24848
Khodabukus, A., and Baar, K. (2015b). Glucose concentration and streptomycin alter in vitro muscle function and metabolism. J. Cell Physiol. 230, 1226–1234. doi: 10.1002/jcp.24857
Khodabukus, A., and Baar, K. (2015c). Streptomycin decreases the functional shift to a slow phenotype induced by electrical stimulation in engineered muscle. Tissue Eng. Part A 21, 1003–1012. doi: 10.1089/ten.tea.2014.0462
Khodabukus, A., Baehr, L. M., Bodine, S. C., and Baar, K. (2015). Role of contraction duration in inducing fast-to-slow contractile and metabolic protein and functional changes in engineered muscle. J. Cell Physiol. 230, 2489–2497. doi: 10.1002/jcp.24985
Khodabukus, A., Kaza, A., Wang, J., Prabhu, N., Goldstein, R., Vaidya, V. S., et al. (2020). Tissue-engineered human myobundle system as a platform for evaluation of skeletal muscle injury biomarkers. Toxicol. Sci. 176, 124–136. doi: 10.1093/toxsci/kfaa049
Khodabukus, A., Madden, L., Prabhu, N. K., Koves, T. R., Jackman, C. P., Muoio, D. M., et al. (2019). Electrical stimulation increases hypertrophy and metabolic flux in tissue-engineered human skeletal muscle. Biomaterials 198, 259–269. doi: 10.1016/j.biomaterials.2018.08.058
Khodabukus, A., Paxton, J. Z., Donnelly, K., and Baar, K. (2007). Engineered muscle: a tool for studying muscle physiology and function. Exerc. Sport Sci. Rev. 35, 186–191. doi: 10.1097/jes.0b013e318156df01
Khodabukus, A., Prabhu, N., Wang, J., and Bursac, N. (2018). In vitro tissue-engineered skeletal muscle models for studying muscle physiology and disease. Adv. Healthc Mater. 7:e1701498.
Kim, J. H., Kim, I., Seol, Y. J., Ko, I. K., Yoo, J. J., Atala, A., et al. (2020). Neural cell integration into 3D bioprinted skeletal muscle constructs accelerates restoration of muscle function. Nat. Commun. 11:1025.
Kim, S. H., Lee, H. R., Yu, S. J., Han, M. E., Lee, D. Y., Kim, S. Y., et al. (2015). Hydrogel-laden paper scaffold system for origami-based tissue engineering. Proc. Natl. Acad. Sci. U.S.A. 112, 15426–15431. doi: 10.1073/pnas.1504745112
Kirschbaum, B. J., Kucher, H. B., Termin, A., Kelly, A. M., and Pette, D. (1990). Antagonistic effects of chronic low frequency stimulation and thyroid hormone on myosin expression in rat fast-twitch muscle. J. Biol. Chem. 265, 13974–13980. doi: 10.1016/s0021-9258(18)77444-9
Kivela, R., Salmela, I., Nguyen, Y. H., Petrova, T. V., Koistinen, H. A., Wiener, Z., et al. (2016). The transcription factor Prox1 is essential for satellite cell differentiation and muscle fibre-type regulation. Nat. Commun. 7:13124.
Kjaer, M. (2004). Role of extracellular matrix in adaptation of tendon and skeletal muscle to mechanical loading. Physiol. Rev. 84, 649–698. doi: 10.1152/physrev.00031.2003
Ko, I. K., Lee, B. K., Lee, S. J., Andersson, K. E., Atala, A., and Yoo, J. J. (2013). The effect of in vitro formation of acetylcholine receptor (AChR) clusters in engineered muscle fibers on subsequent innervation of constructs in vivo. Biomaterials 34, 3246–3255. doi: 10.1016/j.biomaterials.2013.01.029
Koffler, J., Kaufman-Francis, K., Shandalov, Y., Egozi, D., Pavlov, D. A., Landesberg, A., et al. (2011). Improved vascular organization enhances functional integration of engineered skeletal muscle grafts. Proc. Natl. Acad. Sci. U.S.A. 108, 14789–14794. doi: 10.1073/pnas.1017825108
Kosnik, P. E., Faulkner, J. A., and Dennis, R. G. (2001). Functional development of engineered skeletal muscle from adult and neonatal rats. Tissue Eng. 7, 573–584. doi: 10.1089/107632701753213192
Kuang, S., Kuroda, K., Le Grand, F., and Rudnicki, M. A. (2007). Asymmetric self-renewal and commitment of satellite stem cells in muscle. Cell 129, 999–1010. doi: 10.1016/j.cell.2007.03.044
Kubis, H. P., Hanke, N., Scheibe, R. J., Meissner, J. D., and Gros, G. (2003). Ca2+ transients activate calcineurin/NFATc1 and initiate fast-to-slow transformation in a primary skeletal muscle culture. Am. J. Physiol. Cell Physiol. 285, C56–C63.
Kubis, H. P., Scheibe, R. J., Meissner, J. D., Hornung, G., and Gros, G. (2002). Fast-to-slow transformation and nuclear import/export kinetics of the transcription factor NFATc1 during electrostimulation of rabbit muscle cells in culture. J. Physiol. 541, 835–847. doi: 10.1113/jphysiol.2002.017574
Lam, M. T., Huang, Y. C., Birla, R. K., and Takayama, S. (2009). Microfeature guided skeletal muscle tissue engineering for highly organized 3-dimensional free-standing constructs. Biomaterials 30, 1150–1155. doi: 10.1016/j.biomaterials.2008.11.014
Lamar, K. M., and Mcnally, E. M. (2014). Genetic modifiers for neuromuscular diseases. J. Neuromuscul. Dis. 1, 3–13. doi: 10.3233/jnd-140023
Lamboley, C. R., Murphy, R. M., Mckenna, M. J., and Lamb, G. D. (2013). Endogenous and maximal sarcoplasmic reticulum calcium content and calsequestrin expression in type I and type II human skeletal muscle fibres. J. Physiol. 591, 6053–6068. doi: 10.1113/jphysiol.2013.265900
Lanner, J. T., Katz, A., Tavi, P., Sandstrom, M. E., Zhang, S. J., Wretman, C., et al. (2006). The role of Ca2+ influx for insulin-mediated glucose uptake in skeletal muscle. Diabetes 55, 2077–2083. doi: 10.2337/db05-1613
Larkin, L. M., Calve, S., Kostrominova, T. Y., and Arruda, E. M. (2006a). Structure and functional evaluation of tendon-skeletal muscle constructs engineered in vitro. Tissue Eng. 12, 3149–3158. doi: 10.1089/ten.2006.12.3149
Larkin, L. M., Van Der Meulen, J. H., Dennis, R. G., and Kennedy, J. B. (2006b). Functional evaluation of nerve-skeletal muscle constructs engineered in vitro. In Vitro Cell Dev. Biol. Anim. 42, 75–82. doi: 10.1290/0509064.1
Larouche, J., Greising, S. M., Corona, B. T., and Aguilar, C. A. (2018). Robust inflammatory and fibrotic signaling following volumetric muscle loss: a barrier to muscle regeneration. Cell Death Dis. 9:409.
Le Grand, F., Jones, A. E., Seale, V., Scime, A., and Rudnicki, M. A. (2009). Wnt7a activates the planar cell polarity pathway to drive the symmetric expansion of satellite stem cells. Cell Stem. Cell 4, 535–547. doi: 10.1016/j.stem.2009.03.013
Lee, H., Lim, J. Y., and Choi, S. J. (2017). Oleate prevents palmitate-induced atrophy via modulation of mitochondrial ROS production in skeletal myotubes. Oxid. Med. Cell Longev. 2017:2739721.
Lee, P. H., and Vandenburgh, H. H. (2013). Skeletal muscle atrophy in bioengineered skeletal muscle: a new model system. Tissue Eng. Part A 19, 2147–2155. doi: 10.1089/ten.tea.2012.0597
Levenberg, S., Rouwkema, J., Macdonald, M., Garfein, E. S., Kohane, D. S., Darland, D. C., et al. (2005). Engineering vascularized skeletal muscle tissue. Nat. Biotechnol. 23, 879–884.
Li, M., Dickinson, C. E., Finkelstein, E. B., Neville, C. M., and Sundback, C. A. (2011). The role of fibroblasts in self-assembled skeletal muscle. Tissue Eng. Part A 17, 2641–2650. doi: 10.1089/ten.tea.2010.0700
Liao, I. C., Liu, J. B., Bursac, N., and Leong, K. W. (2008). Effect of electromechanical stimulation on the maturation of myotubes on aligned electrospun fibers. Cell Mol. Bioeng. 1, 133–145. doi: 10.1007/s12195-008-0021-y
Ljubicic, V., Miura, P., Burt, M., Boudreault, L., Khogali, S., Lunde, J. A., et al. (2011). Chronic AMPK activation evokes the slow, oxidative myogenic program and triggers beneficial adaptations in mdx mouse skeletal muscle. Hum. Mol. Genet. 20, 3478–3493. doi: 10.1093/hmg/ddr265
Long, C., Li, H., Tiburcy, M., Rodriguez-Caycedo, C., Kyrychenko, V., Zhou, H., et al. (2018). Correction of diverse muscular dystrophy mutations in human engineered heart muscle by single-site genome editing. Sci. Adv. 4:eaa9004.
Loureiro, A. C., Do Rego-Monteiro, I. C., Louzada, R. A., Ortenzi, V. H., De Aguiar, A. P., De Abreu, E. S., et al. (2016). Differential expression of NADPH oxidases depends on skeletal muscle fiber type in rats. Oxid. Med. Cell Longev. 2016:6738701.
Lunde, I. G., Anton, S. L., Bruusgaard, J. C., Rana, Z. A., Ellefsen, S., and Gundersen, K. (2011). Hypoxia inducible factor 1 links fast-patterned muscle activity and fast muscle phenotype in rats. J. Physiol. 589, 1443–1454. doi: 10.1113/jphysiol.2010.202762
Mackey, A. L., Kjaer, M., Charifi, N., Henriksson, J., Bojsen-Moller, J., Holm, L., et al. (2009). Assessment of satellite cell number and activity status in human skeletal muscle biopsies. Muscle Nerve. 40, 455–465. doi: 10.1002/mus.21369
Madden, L., Juhas, M., Kraus, W. E., Truskey, G. A., and Bursac, N. (2015). Bioengineered human myobundles mimic clinical responses of skeletal muscle to drugs. Elife 4:e04885.
Maesner, C. C., Almada, A. E., and Wagers, A. J. (2016). Established cell surface markers efficiently isolate highly overlapping populations of skeletal muscle satellite cells by fluorescence-activated cell sorting. Skelet Muscle 6:35.
Maffioletti, S. M., Sarcar, S., Henderson, A. B. H., Mannhardt, I., Pinton, L., Moyle, L. A., et al. (2018). Three-dimensional human iPSC-derived artificial skeletal muscles model muscular dystrophies and enable multilineage tissue engineering. Cell Rep. 23, 899–908. doi: 10.1016/j.celrep.2018.03.091
Marabita, M., Baraldo, M., Solagna, F., Ceelen, J. J. M., Sartori, R., Nolte, H., et al. (2016). S6K1 is required for increasing skeletal muscle force during hypertrophy. Cell Rep. 17, 501–513. doi: 10.1016/j.celrep.2016.09.020
Marg, A., Escobar, H., Gloy, S., Kufeld, M., Zacher, J., Spuler, A., et al. (2014). Human satellite cells have regenerative capacity and are genetically manipulable. J. Clin. Invest. 124, 4257–4265. doi: 10.1172/jci63992
Marroquin, L. D., Hynes, J., Dykens, J. A., Jamieson, J. D., and Will, Y. (2007). Circumventing the Crabtree effect: replacing media glucose with galactose increases susceptibility of HepG2 cells to mitochondrial toxicants. Toxicol. Sci. 97, 539–547. doi: 10.1093/toxsci/kfm052
Martin, N. R., Passey, S. L., Player, D. J., Mudera, V., Baar, K., Greensmith, L., et al. (2015). Neuromuscular junction formation in tissue-engineered skeletal muscle augments contractile function and improves cytoskeletal organization. Tissue Eng. Part A 21, 2595–2604. doi: 10.1089/ten.tea.2015.0146
Maschmeyer, I., Lorenz, A. K., Schimek, K., Hasenberg, T., Ramme, A. P., Hubner, J., et al. (2015). A four-organ-chip for interconnected long-term co-culture of human intestine, liver, skin and kidney equivalents. Lab. Chip. 15, 2688–2699. doi: 10.1039/c5lc00392j
Mazaleyrat, K., Badja, C., Broucqsault, N., Chevalier, R., Laberthonniere, C., Dion, C., et al. (2020). Multilineage differentiation for formation of innervated skeletal muscle fibers from healthy and diseased human pluripotent stem cells. Cells 9:1531. doi: 10.3390/cells9061531
Mccullagh, K. J., Calabria, E., Pallafacchina, G., Ciciliot, S., Serrano, A. L., Argentini, C., et al. (2004). NFAT is a nerve activity sensor in skeletal muscle and controls activity-dependent myosin switching. Proc. Natl. Acad. Sci. U.S.A. 101, 10590–10595. doi: 10.1073/pnas.0308035101
Medler, S. (2019). Mixing it up: the biological significance of hybrid skeletal muscle fibers. J. Exp. Biol. 222(Pt 23):jeb200832. doi: 10.1242/jeb.200832
Meissner, J. D., Gros, G., Scheibe, R. J., Scholz, M., and Kubis, H. P. (2001). Calcineurin regulates slow myosin, but not fast myosin or metabolic enzymes, during fast-to-slow transformation in rabbit skeletal muscle cell culture. J. Physiol. 533, 215–226. doi: 10.1111/j.1469-7793.2001.0215b.x
Mendell, J. R., Rodino-Klapac, L. R., Sahenk, Z., Roush, K., Bird, L., Lowes, L. P., et al. (2013). Eteplirsen for the treatment of Duchenne muscular dystrophy. Ann. Neurol. 74, 637–647.
Mills, R. J., Parker, B. L., Monnot, P., Needham, E. J., Vivien, C. J., Ferguson, C., et al. (2019). Development of a human skeletal micro muscle platform with pacing capabilities. Biomaterials 198, 217–227. doi: 10.1016/j.biomaterials.2018.11.030
Montarras, D., Morgan, J., Collins, C., Relaix, F., Zaffran, S., Cumano, A., et al. (2005). Direct isolation of satellite cells for skeletal muscle regeneration. Science 309, 2064–2067. doi: 10.1126/science.1114758
Mourkioti, F., Kustan, J., Kraft, P., Day, J. W., Zhao, M. M., Kost-Alimova, M., et al. (2013). Role of telomere dysfunction in cardiac failure in Duchenne muscular dystrophy. Nat. Cell Biol. 15, 895–904. doi: 10.1038/ncb2790
Muntener, M., Berchtold, M. W., and Heizmann, C. W. (1985). Parvalbumin in cross-reinnervated and denervated muscles. Muscle Nerve 8, 132–137. doi: 10.1002/mus.880080209
Murgia, M., Nagaraj, N., Deshmukh, A. S., Zeiler, M., Cancellara, P., Moretti, I., et al. (2015). Single muscle fiber proteomics reveals unexpected mitochondrial specialization. EMBO Rep. 16, 387–395. doi: 10.15252/embr.201439757
Murphy, R. M., Larkins, N. T., Mollica, J. P., Beard, N. A., and Lamb, G. D. (2009). Calsequestrin content and SERCA determine normal and maximal Ca2 + storage levels in sarcoplasmic reticulum of fast- and slow-twitch fibres of rat. J. Physiol. 587, 443–460. doi: 10.1113/jphysiol.2008.163162
Nagamori, E., Ngo, T. X., Takezawa, Y., Saito, A., Sawa, Y., Shimizu, T., et al. (2013). Network formation through active migration of human vascular endothelial cells in a multilayered skeletal myoblast sheet. Biomaterials 34, 662–668. doi: 10.1016/j.biomaterials.2012.08.055
Nesmith, A. P., Wagner, M. A., Pasqualini, F. S., O’connor, B. B., Pincus, M. J., August, P. R., et al. (2016). A human in vitro model of Duchenne muscular dystrophy muscle formation and contractility. J. Cell Biol. 215, 47–56. doi: 10.1083/jcb.201603111
Ngo, T. X., Nagamori, E., Kikuchi, T., Shimizu, T., Okano, T., Taya, M., et al. (2013). Endothelial cell behavior inside myoblast sheets with different thickness. Biotechnol. Lett. 35, 1001–1008. doi: 10.1007/s10529-013-1174-x
Nguyen, E. H., Daly, W. T., Le, N. N. T., Farnoodian, M., Belair, D. G., Schwartz, M. P., et al. (2017). Versatile synthetic alternatives to Matrigel for vascular toxicity screening and stem cell expansion. Nat. Biomed. Eng. 1:0096.
Niro, C., Demignon, J., Vincent, S., Liu, Y., Giordani, J., Sgarioto, N., et al. (2010). Six1 and Six4 gene expression is necessary to activate the fast-type muscle gene program in the mouse primary myotome. Dev. Biol. 338, 168–182. doi: 10.1016/j.ydbio.2009.11.031
Okano, T., and Matsuda, T. (1997). Hybrid muscular tissues: preparation of skeletal muscle cell-incorporated collagen gels. Cell Transplant. 6, 109–118. doi: 10.1016/s0963-6897(96)00255-2
Okano, T., and Matsuda, T. (1998). Tissue engineered skeletal muscle: preparation of highly dense, highly oriented hybrid muscular tissues. Cell Transplant. 7, 71–82. doi: 10.1016/s0963-6897(97)00067-5
Oleaga, C., Bernabini, C., Smith, A. S., Srinivasan, B., Jackson, M., Mclamb, W., et al. (2016). Multi-organ toxicity demonstration in a functional human in vitro system composed of four organs. Sci. Rep. 6:20030.
Osaki, T., Sivathanu, V., and Kamm, R. D. (2018a). Crosstalk between developing vasculature and optogenetically engineered skeletal muscle improves muscle contraction and angiogenesis. Biomaterials 156, 65–76. doi: 10.1016/j.biomaterials.2017.11.041
Osaki, T., Uzel, S. G. M., and Kamm, R. D. (2018b). Microphysiological 3D model of amyotrophic lateral sclerosis (ALS) from human iPS-derived muscle cells and optogenetic motor neurons. Sci. Adv. 4:eaat5847 doi: 10.1126/sciadv.aat5847
Park, K. H., Brotto, L., Lehoang, O., Brotto, M., Ma, J., and Zhao, X. (2012). Ex vivo assessment of contractility, fatigability and alternans in isolated skeletal muscles. J. Vis. Exp. 2:e4198.
Pasut, A., Jones, A. E., and Rudnicki, M. A. (2013). Isolation and culture of individual myofibers and their satellite cells from adult skeletal muscle. J. Vis. Exp. 3:e50074.
Patani, R., Hollins, A. J., Wishart, T. M., Puddifoot, C. A., Álvarez, S., De Lera, A. R., et al. (2011). Retinoid-independent motor neurogenesis from human embryonic stem cells reveals a medial columnar ground state. Nat. Commun. 2:214.
Patkova, J., Andel, M., and Trnka, J. (2014). Palmitate-induced cell death and mitochondrial respiratory dysfunction in myoblasts are not prevented by mitochondria-targeted antioxidants. Cell Physiol. Biochem. 33, 1439–1451. doi: 10.1159/000358709
Patterson, M. F., Stephenson, G. M., and Stephenson, D. G. (2006). Denervation produces different single fiber phenotypes in fast- and slow-twitch hindlimb muscles of the rat. Am. J. Physiol. Cell Physiol. 291, C518–C528.
Pavesi, A., Adriani, G., Rasponi, M., Zervantonakis, I. K., Fiore, G. B., and Kamm, R. D. (2015). Controlled electromechanical cell stimulation on-a-chip. Sci. Rep. 5:11800.
Pegoraro, E., Hoffman, E. P., Piva, L., Gavassini, B. F., Cagnin, S., Ermani, M., et al. (2011). SPP1 genotype is a determinant of disease severity in Duchenne muscular dystrophy. Neurology 76, 219–226. doi: 10.1212/wnl.0b013e318207afeb
Perry, L., Flugelman, M. Y., and Levenberg, S. (2017). Elderly patient-derived endothelial cells for vascularization of engineered muscle. Mol. Ther. 25, 935–948. doi: 10.1016/j.ymthe.2017.02.011
Petchey, L. K., Risebro, C. A., Vieira, J. M., Roberts, T., Bryson, J. B., Greensmith, L., et al. (2014). Loss of Prox1 in striated muscle causes slow to fast skeletal muscle fiber conversion and dilated cardiomyopathy. Proc. Natl. Acad. Sci. U.S.A. 111, 9515–9520. doi: 10.1073/pnas.1406191111
Phua, W. W. T., Wong, M. X. Y., Liao, Z., and Tan, N. S. (2018). An aPPARent functional consequence in skeletal muscle physiology via peroxisome proliferator-activated receptors. Int. J. Mol. Sci. 19:1425. doi: 10.3390/ijms19051425
Picard, M., Hepple, R. T., and Burelle, Y. (2012). Mitochondrial functional specialization in glycolytic and oxidative muscle fibers: tailoring the organelle for optimal function. Am. J. Physiol. Cell Physiol. 302, C629–C641.
Pieber, K., Herceg, M., Paternostro-Sluga, T., and Schuhfried, O. (2015). Optimizing stimulation parameters in functional electrical stimulation of denervated muscles: a cross-sectional study. J. Neuroeng. Rehabil. 12:51.
Potthoff, M. J., Wu, H., Arnold, M. A., Shelton, J. M., Backs, J., Mcanally, J., et al. (2007). Histone deacetylase degradation and MEF2 activation promote the formation of slow-twitch myofibers. J. Clin. Invest. 117, 2459–2467. doi: 10.1172/jci31960
Powell, C. A., Smiley, B. L., Mills, J., and Vandenburgh, H. H. (2002). Mechanical stimulation improves tissue-engineered human skeletal muscle. Am. J. Physiol. Cell Physiol. 283, C1557–C1565.
Prado, L. G., Makarenko, I., Andresen, C., Kruger, M., Opitz, C. A., and Linke, W. A. (2005). Isoform diversity of giant proteins in relation to passive and active contractile properties of rabbit skeletal muscles. J. Gen. Physiol. 126, 461–480. doi: 10.1085/jgp.200509364
Prosser, B. L., Hernandez-Ochoa, E. O., Lovering, R. M., Andronache, Z., Zimmer, D. B., Melzer, W., et al. (2010). S100A1 promotes action potential-initiated calcium release flux and force production in skeletal muscle. Am. J. Physiol. Cell Physiol. 299, C891–C902.
Purves-Smith, F. M., Sgarioto, N., and Hepple, R. T. (2014). Fiber typing in aging muscle. Exerc. Sport Sci. Rev. 42, 45–52. doi: 10.1249/jes.0000000000000012
Putman, C. T., Sultan, K. R., Wassmer, T., Bamford, J. A., Skorjanc, D., and Pette, D. (2001). Fiber-type transitions and satellite cell activation in low-frequency-stimulated muscles of young and aging rats. J. Gerontol. A Biol. Sci. Med. Sci. 56, B510–B519.
Puttonen, K. A., Ruponen, M., Naumenko, N., Hovatta, O. H., Tavi, P., and Koistinaho, J. (2015). Generation of functional neuromuscular junctions from human pluripotent stem cell lines. Front. Cell Neurosci. 9:473.
Quarta, M., Brett, J. O., Dimarco, R., De Morree, A., Boutet, S. C., Chacon, R., et al. (2016). An artificial niche preserves the quiescence of muscle stem cells and enhances their therapeutic efficacy. Nat. Biotechnol. 34, 752–759. doi: 10.1038/nbt.3576
Quarta, M., Cromie, M., Chacon, R., Blonigan, J., Garcia, V., Akimenko, I., et al. (2017). Bioengineered constructs combined with exercise enhance stem cell-mediated treatment of volumetric muscle loss. Nat. Commun. 8:15613.
Quiat, D., Voelker, K. A., Pei, J., Grishin, N. V., Grange, R. W., Bassel-Duby, R., et al. (2011). Concerted regulation of myofiber-specific gene expression and muscle performance by the transcriptional repressor Sox6. Proc. Natl. Acad. Sci. U.S.A. 108, 10196–10201. doi: 10.1073/pnas.1107413108
Rangarajan, S., Madden, L., and Bursac, N. (2014). Use of flow, electrical, and mechanical stimulation to promote engineering of striated muscles. Ann. Biomed. Eng. 42, 1391–1405. doi: 10.1007/s10439-013-0966-4
Rao, L., Qian, Y., Khodabukus, A., Ribar, T., and Bursac, N. (2018). Engineering human pluripotent stem cells into a functional skeletal muscle tissue. Nat. Commun. 9:126.
Rasbach, K. A., Gupta, R. K., Ruas, J. L., Wu, J., Naseri, E., Estall, J. L., et al. (2010). PGC-1alpha regulates a HIF2alpha-dependent switch in skeletal muscle fiber types. Proc. Natl. Acad. Sci. U.S.A. 107, 21866–21871. doi: 10.1073/pnas.1016089107
Renzini, A., Benedetti, A., Bouche, M., Silvestroni, L., Adamo, S., and Moresi, V. (2018). Culture conditions influence satellite cell activation and survival of single myofibers. Eur. J. Transl. Myol. 28:7567.
Ronaldson-Bouchard, K., Ma, S. P., Yeager, K., Chen, T., Song, L., Sirabella, D., et al. (2018). Advanced maturation of human cardiac tissue grown from pluripotent stem cells. Nature 556, 239–243. doi: 10.1038/s41586-018-0016-3
Rosenblatt, J. D., Parry, D. J., and Partridge, T. A. (1996). Phenotype of adult mouse muscle myoblasts reflects their fiber type of origin. Differentiation 60, 39–45. doi: 10.1046/j.1432-0436.1996.6010039.x
Rudolf, R., Deschenes, M. R., and Sandri, M. (2016). Neuromuscular junction degeneration in muscle wasting. Curr. Opin. Clin. Nutr. Metab. Care 19, 177–181.
Ryall, J. G., Dell’orso, S., Derfoul, A., Juan, A., Zare, H., Feng, X., et al. (2015). The NAD(+)-dependent SIRT1 deacetylase translates a metabolic switch into regulatory epigenetics in skeletal muscle stem cells. Cell Stem. Cell 16, 171–183. doi: 10.1016/j.stem.2014.12.004
Sacco, A., Mourkioti, F., Tran, R., Choi, J., Llewellyn, M., Kraft, P., et al. (2010). Short telomeres and stem cell exhaustion model Duchenne muscular dystrophy in mdx/mTR mice. Cell 143, 1059–1071. doi: 10.1016/j.cell.2010.11.039
Sakakibara, I., Santolini, M., Ferry, A., Hakim, V., and Maire, P. (2014). Six homeoproteins and a Iinc-RNA at the fast MYH locus lock fast myofiber terminal phenotype. PLoS Genet. 10:e1004386. doi: 10.1371/journal.pgen.1004386
Salmons, S., and Sreter, F. A. (1976). Significance of impulse activity in the transformation of skeletal muscle type. Nature 263, 30–34. doi: 10.1038/263030a0
Sances, S., Bruijn, L. I., Chandran, S., Eggan, K., Ho, R., Klim, J. R., et al. (2016). Modeling ALS with motor neurons derived from human induced pluripotent stem cells. Nat. Neurosci. 19, 542–553.
Sato, M., Ito, A., Kawabe, Y., Nagamori, E., and Kamihira, M. (2011). Enhanced contractile force generation by artificial skeletal muscle tissues using IGF-I gene-engineered myoblast cells. J. Biosci. Bioeng. 112, 273–278. doi: 10.1016/j.jbiosc.2011.05.007
Schiaffino, S. (2010). Fibre types in skeletal muscle: a personal account. Acta. Physiol. (Oxf). 199, 451–463. doi: 10.1111/j.1748-1716.2010.02130.x
Schiaffino, S., and Reggiani, C. (2011). Fiber types in mammalian skeletal muscles. Physiol. Rev. 91, 1447–1531. doi: 10.1152/physrev.00031.2010
Schuh, R. A., Jackson, K. C., Khairallah, R. J., Ward, C. W., and Spangenburg, E. E. (2012). Measuring mitochondrial respiration in intact single muscle fibers. Am. J. Physiol. Regul. Integr. Comp. Physiol. 302, R712–R719.
Schuler, M., Ali, F., Chambon, C., Duteil, D., Bornert, J. M., Tardivel, A., et al. (2006). PGC1alpha expression is controlled in skeletal muscles by PPARbeta, whose ablation results in fiber-type switching, obesity, and type 2 diabetes. Cell Metab. 4, 407–414. doi: 10.1016/j.cmet.2006.10.003
Schwaller, B., Dick, J., Dhoot, G., Carroll, S., Vrbova, G., Nicotera, P., et al. (1999). Prolonged contraction-relaxation cycle of fast-twitch muscles in parvalbumin knockout mice. Am. J. Physiol. 276, C395–C403.
Sellers, J. R. (2004). Fifty years of contractility research post sliding filament hypothesis. J. Muscle Res. Cell Motil. 25, 475–482. doi: 10.1007/s10974-004-4239-6
Selvaraj, S., Mondragon-Gonzalez, R., Xu, B., Magli, A., Kim, H., Laine, J., et al. (2019). Screening identifies small molecules that enhance the maturation of human pluripotent stem cell-derived myotubes. Elife 8:e147970.
Seto, J. T., Quinlan, K. G., Lek, M., Zheng, X. F., Garton, F., Macarthur, D. G., et al. (2013). ACTN3 genotype influences muscle performance through the regulation of calcineurin signaling. J. Clin. Invest. 123, 4255–4263. doi: 10.1172/jci67691
Shansky, J., Del Tatto, M., Chromiak, J., and Vandenburgh, H. (1997). A simplified method for tissue engineering skeletal muscle organoids in vitro. In Vitro Cell Dev. Biol. Anim. 33, 659–661. doi: 10.1007/s11626-997-0118-y
Shen, H. W., Jiang, X. L., Gonzalez, F. J., and Yu, A. M. (2011). Humanized transgenic mouse models for drug metabolism and pharmacokinetic research. Curr. Drug Metab. 12, 997–1006. doi: 10.2174/138920011798062265
Shoji, E., Sakurai, H., Nishino, T., Nakahata, T., Heike, T., Awaya, T., et al. (2015). Early pathogenesis of Duchenne muscular dystrophy modelled in patient-derived human induced pluripotent stem cells. Sci. Rep. 5:12831.
Sicari, B. M., Rubin, J. P., Dearth, C. L., Wolf, M. T., Ambrosio, F., Boninger, M., et al. (2014). An acellular biologic scaffold promotes skeletal muscle formation in mice and humans with volumetric muscle loss. Sci. Transl. Med. 6:234ra258.
Sim, W. Y., Park, S. W., Park, S. H., Min, B. H., Park, S. R., and Yang, S. S. (2007). A pneumatic micro cell chip for the differentiation of human mesenchymal stem cells under mechanical stimulation. Lab. Chip. 7, 1775–1782. doi: 10.1039/b712361m
Skardal, A., Murphy, S. V., Devarasetty, M., Mead, I., Kang, H. W., Seol, Y. J., et al. (2017). Multi-tissue interactions in an integrated three-tissue organ-on-a-chip platform. Sci. Rep. 7:8837.
Sreetama, S. C., Chandra, G., Van Der Meulen, J. H., Ahmad, M. M., Suzuki, P., Bhuvanendran, S., et al. (2018). Membrane stabilization by modified steroid offers a potential therapy for muscular dystrophy due to dysferlin deficit. Mol. Ther. 26, 2231–2242. doi: 10.1016/j.ymthe.2018.07.021
Stearns-Reider, K. M., D’amore, A., Beezhold, K., Rothrauff, B., Cavalli, L., Wagner, W. R., et al. (2017). Aging of the skeletal muscle extracellular matrix drives a stem cell fibrogenic conversion. Aging Cell 16, 518–528. doi: 10.1111/acel.12578
Stein, C. A. (2016). Eteplirsen approved for duchenne muscular dystrophy: the FDA faces a difficult choice. Mol. Ther. 24, 1884–1885. doi: 10.1038/mt.2016.188
Steinbeck, J. A., Jaiswal, M. K., Calder, E. L., Kishinevsky, S., Weishaupt, A., Toyka, K. V., et al. (2016). Functional connectivity under optogenetic control allows modeling of human neuromuscular disease. Cell Stem. Cell 18, 134–143. doi: 10.1016/j.stem.2015.10.002
Stienen, G. J., Kiers, J. L., Bottinelli, R., and Reggiani, C. (1996). Myofibrillar ATPase activity in skinned human skeletal muscle fibres: fibre type and temperature dependence. J. Physiol. 493(Pt 2), 299–307. doi: 10.1113/jphysiol.1996.sp021384
Strohman, R. C., Bayne, E., Spector, D., Obinata, T., Micou-Eastwood, J., and Maniotis, A. (1990). Myogenesis and histogenesis of skeletal muscle on flexible membranes in vitro. In Vitro Cell Dev. Biol. 26, 201–208. doi: 10.1007/bf02624113
Summermatter, S., Thurnheer, R., Santos, G., Mosca, B., Baum, O., Treves, S., et al. (2012). Remodeling of calcium handling in skeletal muscle through PGC-1alpha: impact on force, fatigability, and fiber type. Am. J. Physiol. Cell Physiol. 302, C88–C99.
Sun, C., Choi, I. Y., Gonzalez, Y. I. R., Andersen, P., Talbot, C. C. Jr., Iyer, S. R., et al. (2020). Duchenne muscular dystrophy hiPSC-derived myoblast drug screen identifies compounds that ameliorate disease in mdx mice. JCI Insight 5:e134287.
Swoap, S. J., Hunter, R. B., Stevenson, E. J., Felton, H. M., Kansagra, N. V., Lang, J. M., et al. (2000). The calcineurin-NFAT pathway and muscle fiber-type gene expression. Am. J. Physiol. Cell Physiol. 279, C915–C924.
Takahashi, H., and Okano, T. (2015). Cell sheet-based tissue engineering for organizing anisotropic tissue constructs produced using microfabricated thermoresponsive substrates. Adv. Healthc. Mater. 4, 2388–2407. doi: 10.1002/adhm.201500194
Takahashi, H., Shimizu, T., Nakayama, M., Yamato, M., and Okano, T. (2013). The use of anisotropic cell sheets to control orientation during the self-organization of 3D muscle tissue. Biomaterials 34, 7372–7380. doi: 10.1016/j.biomaterials.2013.06.033
Takahashi, H., Shimizu, T., and Okano, T. (2018). Engineered human contractile myofiber sheets as a platform for studies of skeletal muscle physiology. Sci. Rep. 8:13932.
Terzis, G., Georgiadis, G., Stratakos, G., Vogiatzis, I., Kavouras, S., Manta, P., et al. (2008). Resistance exercise-induced increase in muscle mass correlates with p70S6 kinase phosphorylation in human subjects. Eur. J. Appl. Physiol. 102, 145–152. doi: 10.1007/s00421-007-0564-y
Thelen, M. H., Simonides, W. S., and Van Hardeveld, C. (1997). Electrical stimulation of C2C12 myotubes induces contractions and represses thyroid-hormone-dependent transcription of the fast-type sarcoplasmic-reticulum Ca2+-ATPase gene. Biochem. J. 321(Pt 3), 845–848. doi: 10.1042/bj3210845
Thorsteinsdottir, S., Deries, M., Cachaco, A. S., and Bajanca, F. (2011). The extracellular matrix dimension of skeletal muscle development. Dev. Biol. 354, 191–207. doi: 10.1016/j.ydbio.2011.03.015
Toli, D., Buttigieg, D., Blanchard, S., Lemonnier, T., Lamotte D’incamps, B., Bellouze, S., et al. (2015). Modeling amyotrophic lateral sclerosis in pure human iPSc-derived motor neurons isolated by a novel FACS double selection technique. Neurobiol. Dis. 82, 269–280. doi: 10.1016/j.nbd.2015.06.011
Tsika, R. W., Schramm, C., Simmer, G., Fitzsimons, D. P., Moss, R. L., and Ji, J. (2008). Overexpression of TEAD-1 in transgenic mouse striated muscles produces a slower skeletal muscle contractile phenotype. J. Biol. Chem. 283, 36154–36167. doi: 10.1074/jbc.m807461200
Tsukamoto, S., Shibasaki, A., Naka, A., Saito, H., and Iida, K. (2018). Lactate Promotes myoblast differentiation and myotube hypertrophy via a pathway involving MyoD in vitro and enhances muscle regeneration in vivo. Int. J. Mol. Sci. 19:3649. doi: 10.3390/ijms19113649
Uezumi, A., Nakatani, M., Ikemoto-Uezumi, M., Yamamoto, N., Morita, M., Yamaguchi, A., et al. (2016). Cell-surface protein profiling identifies distinctive markers of progenitor cells in human skeletal muscle. Stem Cell Rep. 7, 263–278. doi: 10.1016/j.stemcr.2016.07.004
Urbanchek, M. G., Kung, T. A., Frost, C. M., Martin, D. C., Larkin, L. M., Wollstein, A., et al. (2016). Development of a regenerative peripheral nerve interface for control of a neuroprosthetic limb. Biomed. Res. Int. 2016:5726730.
Uzel, S. G., Platt, R. J., Subramanian, V., Pearl, T. M., Rowlands, C. J., Chan, V., et al. (2016). Microfluidic device for the formation of optically excitable, three-dimensional, compartmentalized motor units. Sci. Adv. 2:e1501429. doi: 10.1126/sciadv.1501429
Van Putten, M., Putker, K., Overzier, M., Adamzek, W. A., Pasteuning-Vuhman, S., Plomp, J. J., et al. (2019). Natural disease history of the D2-mdx mouse model for Duchenne muscular dystrophy. FASEB J. 33, 8110–8124. doi: 10.1096/fj.201802488r
Vandenburgh, H., and Kaufman, S. (1979). In vitro model for stretch-induced hypertrophy of skeletal muscle. Science 203, 265–268. doi: 10.1126/science.569901
Vandenburgh, H., Shansky, J., Benesch-Lee, F., Barbata, V., Reid, J., Thorrez, L., et al. (2008). Drug-screening platform based on the contractility of tissue-engineered muscle. Muscle Nerve 37, 438–447. doi: 10.1002/mus.20931
Vandenburgh, H. H. (1982). Dynamic mechanical orientation of skeletal myofibers in vitro. Dev. Biol. 93, 438–443. doi: 10.1016/0012-1606(82)90131-2
Vandenburgh, H. H. (1988). A computerized mechanical cell stimulator for tissue culture: effects on skeletal muscle organogenesis. In Vitro Cell Dev. Biol. 24, 609–619. doi: 10.1007/bf02623597
Vandenburgh, H. H., Hatfaludy, S., Karlisch, P., and Shansky, J. (1989). Skeletal muscle growth is stimulated by intermittent stretch-relaxation in tissue culture. Am. J. Physiol. 256, C674–C682.
Vandenburgh, H. H., Hatfaludy, S., Karlisch, P., and Shansky, J. (1991). Mechanically induced alterations in cultured skeletal muscle growth. J. Biomech. 24(Suppl. 1), 91–99. doi: 10.1016/0021-9290(91)90380-6
Vandenburgh, H. H., and Karlisch, P. (1989). Longitudinal growth of skeletal myotubes in vitro in a new horizontal mechanical cell stimulator. In Vitro Cell Dev. Biol. 25, 607–616. doi: 10.1007/bf02623630
Vandenburgh, H. H., Karlisch, P., and Farr, L. (1988). Maintenance of highly contractile tissue-cultured avian skeletal myotubes in collagen gel. In Vitro Cell Dev. Biol. 24, 166–174. doi: 10.1007/bf02623542
Vandenburgh, H. H., and Kaufman, S. (1981). Stretch-induced growth of skeletal myotubes correlates with activation of the sodium pump. J. Cell Physiol. 109, 205–214. doi: 10.1002/jcp.1041090203
VanDusen, K. W., Syverud, B. C., Williams, M. L., Lee, J. D., and Larkin, L. M. (2014). Engineered skeletal muscle units for repair of volumetric muscle loss in the tibialis anterior muscle of a rat. Tissue Eng. Part A 20, 2920–2930. doi: 10.1089/ten.tea.2014.0060
Verdijk, L. B., Koopman, R., Schaart, G., Meijer, K., Savelberg, H. H., and Van Loon, L. J. (2007). Satellite cell content is specifically reduced in type II skeletal muscle fibers in the elderly. Am. J. Physiol. Endocrinol. Metab. 292, E151–E157.
Verdijk, L. B., Snijders, T., Drost, M., Delhaas, T., Kadi, F., and Van Loon, L. J. (2014). Satellite cells in human skeletal muscle; from birth to old age. Age (Dordr.) 36, 545–547.
Verma, M., Asakura, Y., Murakonda, B. S. R., Pengo, T., Latroche, C., Chazaud, B., et al. (2018). Muscle satellite cell cross-talk with a vascular niche maintains quiescence via VEGF and notch signaling. Cell Stem. Cell 23, e539.
Vernetti, L., Gough, A., Baetz, N., Blutt, S., Broughman, J. R., Brown, J. A., et al. (2017). Functional coupling of human microphysiology systems: intestine liver, kidney proximal tubule, blood-brain barrier and skeletal muscle. Sci. Rep. 7:42296.
Vila, O. F., Uzel, S. G. M., Ma, S. P., Williams, D., Pak, J., Kamm, R. D., et al. (2019). Quantification of human neuromuscular function through optogenetics. Theranostics 9, 1232–1246. doi: 10.7150/thno.25735
Wang, J., Khodabukus, A., Rao, L., Vandusen, K., Abutaleb, N., and Bursac, N. (2019). Engineered skeletal muscles for disease modeling and drug discovery. Biomaterials 221:119416. doi: 10.1016/j.biomaterials.2019.119416
Wang, X., Ono, Y., Tan, S. C., Chai, R. J., Parkin, C., and Ingham, P. W. (2011). Prdm1a and miR-499 act sequentially to restrict Sox6 activity to the fast-twitch muscle lineage in the zebrafish embryo. Development 138, 4399–4404. doi: 10.1242/dev.070516
Wang, Y. X., Feige, P., Brun, C. E., Hekmatnejad, B., Dumont, N. A., Renaud, J. M., et al. (2019). EGFR-aurka signaling rescues polarity and regeneration defects in dystrophin-deficient muscle stem cells by increasing asymmetric divisions. Cell Stem. Cell 24:e416.
Wang, Y. X., Zhang, C. L., Yu, R. T., Cho, H. K., Nelson, M. C., Bayuga-Ocampo, C. R., et al. (2004). Regulation of muscle fiber type and running endurance by PPARdelta. PLoS Biol. 2:e294. doi: 10.1371/journal.pbio.0020294
Watanabe, N., Komiya, Y., Sato, Y., Watanabe, Y., Suzuki, T., and Arihara, K. (2020). Oleic acid up-regulates myosin heavy chain (MyHC) 1 expression and increases mitochondrial mass and maximum respiration in C2C12 myoblasts. Biochem. Biophys. Res. Commun. 525, 406–411. doi: 10.1016/j.bbrc.2020.02.099
Webster, C., Silberstein, L., Hays, A. P., and Blau, H. M. (1988). Fast muscle fibers are preferentially affected in Duchenne muscular dystrophy. Cell 52, 503–513. doi: 10.1016/0092-8674(88)90463-1
Wehrle, U., Dusterhoft, S., and Pette, D. (1994). Effects of chronic electrical stimulation on myosin heavy chain expression in satellite cell cultures derived from rat muscles of different fiber-type composition. Differentiation 58, 37–46. doi: 10.1046/j.1432-0436.1994.5810037.x
Weinshilboum, R. M., and Wang, L. (2017). Pharmacogenomics: precision medicine and drug response. Mayo Clin. Proc. 92, 1711–1722. doi: 10.1016/j.mayocp.2017.09.001
Westerblad, H., and Allen, D. G. (1991). Changes of myoplasmic calcium concentration during fatigue in single mouse muscle fibers. J. Gen. Physiol. 98, 615–635. doi: 10.1085/jgp.98.3.615
Westerblad, H., Duty, S., and Allen, D. G. (1993). Intracellular calcium concentration during low-frequency fatigue in isolated single fibers of mouse skeletal muscle. J. Appl. Physiol. (1985) 75, 382–388. doi: 10.1152/jappl.1993.75.1.382
Westgaard, R. H., and Lomo, T. (1988). Control of contractile properties within adaptive ranges by patterns of impulse activity in the rat. J. Neurosci. 8, 4415–4426. doi: 10.1523/jneurosci.08-12-04415.1988
Williams, M. L., Kostrominova, T. Y., Arruda, E. M., and Larkin, L. M. (2013). Effect of implantation on engineered skeletal muscle constructs. J. Tissue Eng. Regen. Med. 7, 434–442. doi: 10.1002/term.537
Windisch, A., Gundersen, K., Szabolcs, M. J., Gruber, H., and Lomo, T. (1998). Fast to slow transformation of denervated and electrically stimulated rat muscle. J. Physiol. 510(Pt 2), 623–632. doi: 10.1111/j.1469-7793.1998.623bk.x
Woodworth-Hobbs, M. E., Perry, B. D., Rahnert, J. A., Hudson, M. B., Zheng, B., and Russ Price, S. (2017). Docosahexaenoic acid counteracts palmitate-induced endoplasmic reticulum stress in C2C12 myotubes: Impact on muscle atrophy. Physiol. Rep. 5:e13530.
Wu, H., Naya, F. J., Mckinsey, T. A., Mercer, B., Shelton, J. M., Chin, E. R., et al. (2000). MEF2 responds to multiple calcium-regulated signals in the control of skeletal muscle fiber type. EMBO J. 19, 1963–1973. doi: 10.1093/emboj/19.9.1963
Yamaguchi, M., Izumimoto, M., Robson, R. M., and Stromer, M. H. (1985). Fine structure of wide and narrow vertebrate muscle Z-lines. A proposed model and computer simulation of Z-line architecture. J. Mol. Biol. 184, 621–643. doi: 10.1016/0022-2836(85)90308-0
Yang, L., Van Der Werf, K. O., Koopman, B. F., Subramaniam, V., Bennink, M. L., Dijkstra, P. J., et al. (2007). Micromechanical bending of single collagen fibrils using atomic force microscopy. J. Biomed. Mater. Res. A 82, 160–168. doi: 10.1002/jbm.a.31127
Yang, N., Macarthur, D. G., Gulbin, J. P., Hahn, A. G., Beggs, A. H., Easteal, S., et al. (2003). ACTN3 genotype is associated with human elite athletic performance. Am. J. Hum. Genet. 73, 627–631. doi: 10.1086/377590
Young, C. S., Hicks, M. R., Ermolova, N. V., Nakano, H., Jan, M., Younesi, S., et al. (2016). A Single CRISPR-Cas9 deletion strategy that targets the majority of DMD patients restores dystrophin function in hiPSC-derived muscle cells. Cell Stem. Cell 18, 533–540. doi: 10.1016/j.stem.2016.01.021
Young, C. S., Mokhonova, E., Quinonez, M., Pyle, A. D., and Spencer, M. J. (2017). Creation of a novel humanized dystrophic mouse model of duchenne muscular dystrophy and application of a CRISPR/Cas9 gene editing therapy. J. Neuromuscul. Dis. 4, 139–145. doi: 10.3233/jnd-170218
Yucel, N., Chang, A. C., Day, J. W., Rosenthal, N., and Blau, H. M. (2018). Humanizing the mdx mouse model of DMD: the long and the short of it. NPJ. Regen. Med. 3:4.
Yuzefovych, L., Wilson, G., and Rachek, L. (2010). Different effects of oleate vs. palmitate on mitochondrial function, apoptosis, and insulin signaling in L6 skeletal muscle cells: role of oxidative stress. Am. J. Physiol. Endocrinol. Metab. 299, E1096–E1105.
Yuzefovych, L. V., Solodushko, V. A., Wilson, G. L., and Rachek, L. I. (2012). Protection from palmitate-induced mitochondrial DNA damage prevents from mitochondrial oxidative stress, mitochondrial dysfunction, apoptosis, and impaired insulin signaling in rat L6 skeletal muscle cells. Endocrinology 153, 92–100. doi: 10.1210/en.2011-1442
Zhang, D., Wang, X., Li, Y., Zhao, L., Lu, M., Yao, X., et al. (2014). Thyroid hormone regulates muscle fiber type conversion via miR-133a1. J. Cell Biol. 207, 753–766. doi: 10.1083/jcb.201406068
Keywords: skeletal muscle, tissue engineering, fiber-type, satellite cell, disease modeling, Duchenne Muscle dystrophy, innervation, myosin heavy chain
Citation: Khodabukus A (2021) Tissue-Engineered Skeletal Muscle Models to Study Muscle Function, Plasticity, and Disease. Front. Physiol. 12:619710. doi: 10.3389/fphys.2021.619710
Received: 20 October 2020; Accepted: 25 January 2021;
Published: 26 February 2021.
Edited by:
Enrique Jaimovich, University of Chile, ChileReviewed by:
Mariana Casas, University of Chile, ChileCopyright © 2021 Khodabukus. This is an open-access article distributed under the terms of the Creative Commons Attribution License (CC BY). The use, distribution or reproduction in other forums is permitted, provided the original author(s) and the copyright owner(s) are credited and that the original publication in this journal is cited, in accordance with accepted academic practice. No use, distribution or reproduction is permitted which does not comply with these terms.
*Correspondence: Alastair Khodabukus, YWlrMTJAZHVrZS5lZHU=
Disclaimer: All claims expressed in this article are solely those of the authors and do not necessarily represent those of their affiliated organizations, or those of the publisher, the editors and the reviewers. Any product that may be evaluated in this article or claim that may be made by its manufacturer is not guaranteed or endorsed by the publisher.
Research integrity at Frontiers
Learn more about the work of our research integrity team to safeguard the quality of each article we publish.