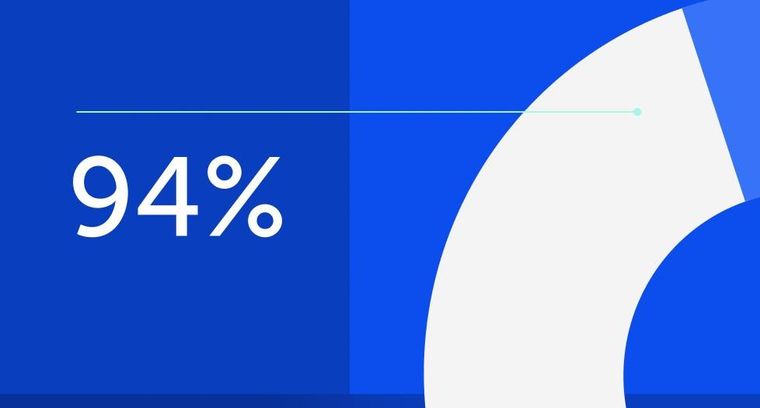
94% of researchers rate our articles as excellent or good
Learn more about the work of our research integrity team to safeguard the quality of each article we publish.
Find out more
ORIGINAL RESEARCH article
Front. Physiol., 22 July 2021
Sec. Vascular Physiology
Volume 12 - 2021 | https://doi.org/10.3389/fphys.2021.616317
This article is part of the Research TopicCardioprotection, Sex and Gender DifferencesView all 13 articles
Previous reports suggest that diabetes may differentially affect the vascular beds of females and males. The objectives of this study were to examine whether there were (1) sex differences in aortic function and (2) alterations in the relative contribution of endothelium-derived relaxing factors in modulating aortic reactivity in UC Davis Type 2 Diabetes Mellitus (UCD-T2DM) rats. Endothelium-dependent vasorelaxation (EDV) in response to acetylcholine (ACh) was measured in aortic rings before and after exposure to pharmacological inhibitors. Relaxation responses to sodium nitroprusside were assessed in endothelium-denuded rings. Moreover, contractile responses to phenylephrine (PE) were measured before and after incubation of aortic rings with a nitric oxide synthase (NOS) inhibitor in the presence of indomethacin. Metabolic parameters and expression of molecules associated with vascular and insulin signaling as well as reactive oxygen species generation were determined. Diabetes slightly but significantly impaired EDV in response to ACh in aortas from females but potentiated the relaxation response in males. The potentiation of EDV in diabetic male aortas was accompanied by a traces of nitric oxide (NO)- and prostanoid-independent relaxation and elevated aortic expression of small- and intermediate conductance Ca2+-activated K+ channels in this group. The smooth muscle sensitivity to NO was not altered, whereas the responsiveness to PE was significantly enhanced in aortas of diabetic groups in both sexes. Endothelium-derived NO during smooth muscle contraction, as assessed by the potentiation of the response to PE after NOS inhibition, was reduced in aortas of diabetic rats regardless of sex. Accordingly, decreases in pAkt and peNOS were observed in aortas from diabetic rats in both sexes compared with controls. Our data suggest that a decrease in insulin sensitivity via pAkt-peNOS-dependent signaling and an increase in oxidative stress may contribute to the elevated contractile responses observed in diabetic aortas in both sexes. This study demonstrates that aortic function in UCD-T2DM rats is altered in both sexes. Here, we provide the first evidence of sexual dimorphism in aortic relaxation in UCD-T2DM rats.
Over the past decade, obesity and type 2 diabetes (T2D) have reached epidemic levels worldwide, becoming one of the most challenging health problems in the 21st century (Tabish, 2007; Zheng et al., 2018). Cardiovascular diseases (CVDs) are one of the primary causes of morbidity and mortality in patients with diabetes (Brunner et al., 2005). Premenopausal women have a lower incidence of CVD when compared with age-matched men (Barrett-Connor, 1994). However, premenopausal women with diabetes not only lose the sex-based cardiovascular protection but also experience a higher relative risk of CVD compared to diabetic men (Steinberg et al., 2000). It has been established that hyperglycemia and diabetes affect female and male vascular beds differently. We previously reported sex differences in the development of vascular dysfunction in arteries of streptozotocin-induced type 1 diabetic rats (Zhang et al., 2012; Han et al., 2016). However, the pathophysiology of vascular dysfunction in T2D is likely to differ from that in type 1 diabetes.
With the increasing prevalence of T2D, creating effective preclinical models of the disease has become crucial for disease prevention and treatment. Dr. Havel and colleagues at the University of California (UC) Davis developed a validated rat model of T2D, the UC Davis Type 2 Diabetes Mellitus (UCD-T2DM) rats. UCD-T2DM rats exhibit all of the key features of the disease in humans such as polygenic adult-onset obesity, insulin resistance, intact leptin signaling, and spontaneous β-cell decompensation with preserved fertility in both sexes (Cummings et al., 2008; Kleinert et al., 2018).
Recently, we reported sex differences in the development of impaired vascular reactivity in mesenteric arteries from UCD-T2DM rats (Shaligram et al., 2020). Nevertheless, it remains to be established whether this reported sexual dimorphism is specific to the small arteries or is generalizable to larger conduit arteries in type 2 diabetic arteries. Thus, the initial aim of our study was to determine whether the aortic response to endothelium-dependent and independent vasodilators and vasoconstrictors varies with sex in UCD-T2DM rats.
Endothelium-dependent vasorelaxation (EDV) is considered a reproducible factor for assessing endothelial function (De Vriese et al., 2000). In diabetes, enhanced (Aloysius et al., 2012), impaired (Vanhoutte et al., 2009), and preserved EDV (Miller and Vanhoutte, 1991) have been reported. Altered EDV can result from alteration in synthesis or release of endothelium-derived relaxing factors (EDRF) [nitric oxide (NO), prostacyclin (PGI2), and endothelium-derived hyperpolarizing factor (EDHF)] or endothelium-derived contracting factors.
Nitric oxide has been considered a major contributor to EDV in large conduit arteries (Félétou, 2011), whereas EDHF plays a predominant role in small resistance arteries (Garland et al., 1995). In large conduit arteries, it is widely accepted that NO levels are reduced in diabetes (Han et al., 2014) and that changes in the level of endothelial NO synthase (eNOS) and/or increased generation of reactive oxygen species (ROS) such as superoxide may contribute to the reduction of NO production or bioavailability.
It has also been proposed that EDHF may play a role as a backup vasodilator in small resistance vessels when NO bioavailability is compromised (Brandes et al., 2000). The chemical identity of EDHF varies with vascular size, vascular bed and species (Leo et al., 2011). The classical EDHF pathway involves the opening of small- and intermediate-conductance calcium-activated potassium channels (SKCa and IKCa) on the endothelium and the subsequent hyperpolarizing of smooth muscle cells via activation of Na-K-ATPase and/or Kir channels or through myoendothelial gap junctions (Edwards et al., 1998; Parkington et al., 2002; Sandow et al., 2002). Previous studies have provided evidence of EDHF-type responses induced by acetylcholine (ACh) in rabbit conduit arteries that are potentiated by the elevation of cAMP but inhibited by disruption of gap junctions or a combination of SKCa and IKCa channel blockers (Griffith et al., 2002).
Overall, studies in various experimental models have evaluated the effects of diabetes on endothelial NO production. However, the sex-specific effects of T2D on the relative contribution of EDRF to the vascular reactivity of large conduit arteries remain unclear. Here, we examined changes in the relative importance of EDRF in modulating aortic relaxation in male and female UCD-T2DM rats.
Insulin resistance, a key element in the pathogenesis of T2D (Ormazabal et al., 2018), is associated with endothelial dysfunction by several mechanisms including oxidative stress. Here, we evaluated the responsiveness to insulin signaling by measuring the aortic expression of insulin receptor substrates (IRS-1 and IRS-2), total and phosphorylated levels of Akt, and eNOS. Since NADPH oxidases (NOX) are a potent cellular source of superoxide in the cardiovascular system (Drummond and Sobey, 2014), experiments were also carried out to determine the aortic expression of NOX subtypes. Moreover, ROS generation was determined in primary aortic endothelial cells isolated from male and female UCD-T2DM rats.
This study demonstrates that aortic function in UCD-T2DM rats is altered in both sexes. Here, we provide the first evidence of sexual dimorphism in aortic relaxation in UCD-T2DM rats.
Male and female UCD-T2DM rats were generated by breeding obese Sprague–Dawley (SD) rats with Zucker Diabetic Fatty (ZDF) lean rats that were homozygous wild type for the leptin receptor and had inherent β-cell defects. Rats were bred at the animal facility in the Department of Nutrition at the UC Davis (Cummings et al., 2008).
Rats were maintained with water and standard rodent chow food ad libitum at constant humidity and temperature, with a light/dark cycle of 12 h. After acclimation for 1 week at the animal facility at the University of the Pacific, animals were euthanized for experiments using carbon dioxide as the euthanasia agent, according to the recommendations from the 2013 AVMA Guidelines on Euthanasia and the NIH Guidelines for the Care and Use of Laboratory Animals: Eighth Edition (US National Institutes of Health, 2011). Age-matched male and female non-obese and non-diabetic SD rats (Simonsen Laboratories, Gilroy, CA, United States) (∼average 19–20 weeks old) were employed as controls for UCD-T2DM rats. Diabetic phase was determined by measuring blood glucose levels for three subsequent measurements using a standard glucose test meter (OneTouch, LifeScan, CA). Animals were considered diabetic when non-fasting blood glucose levels were higher than 300 mg/dl. The diabetic animals used in the study were diabetic for 35 ± 2.7 (males) and 31 ± 3.1 (females) days. UCD-T2DM rats exhibit insulin resistance prior to the onset of diabetes, similar to humans (Cummings et al., 2008).
All animal protocols were approved by the Animal Care Committee of the University of the Pacific and UC Davis Institutional Animal Care and Use Committee and complied with the Guide for the Care and Use of Laboratory Animals: Eighth Edition (US National Institutes of Health, 2011) and with ARRIVE guidelines.
Blood glucose levels were measured in 12-h fasted rats using a standard glucose test meter (OneTouch, LifeScan, CA) and triglycerides were measured by using an Accutrend Plus System (hand-held point-of-care device) and specific test strips (Roche Farma, Barcelona, Spain) with a drop of blood collected from the tail vein. Blood samples were collected from intracardiac puncture and obtained in tubes containing heparin as an anticoagulant. Plasma was obtained by centrifugation at 10,000 × g for 5 min at 4°C and stored at −80°C until used. Insulin levels were determined in plasma samples by using ELISA kits according to the manufacturer’s protocol (Spi Bio, Montigny Le Bretonneux, France). Insulin sensitivity index (ISI) was determined from fasting plasma glucose and insulin using the following formula: ISI = [2/(blood insulin (nM) × blood glucose (μM) + 1)] (Sangüesa et al., 2017). HbA1c was measured using the Bayer A1cNow test kit, according to the manufacturer’s instructions, and animals with A1c levels greater than 6.5% on two separate tests were considered diabetic.
The thoracic aorta was cut into 2-mm rings after being excised and cleaned off adhering connective tissues. To measure isometric tension, the rings were suspended horizontally between two stainless steel hooks in individual organ baths containing 20 ml of Krebs buffer (in mM: 119 NaCl, 4.7 KCl, 1.18 KH2PO4, 1.17 MgSO4, 24.9 NaHCO3, 0.023 EDTA, 1.6 CaCl2, and 6.0 glucose) at 37°C bubbled with 95% O2 and 5% CO2. Isometric tension was continuously monitored with a computer-based data acquisition system (PowerLab; ADInstruments, Colorado Springs, CO, United States). To develop a stable basal tone, aortic rings were equilibrated under 1g resting tension for 40 min. Rings were stimulated two times with 80 mM KCl every 20 min until maximum contraction was achieved. The ability of ACh (10 μM) to induce relaxation of phenylephrine (PE, 2 μM) pre-contracted vessels was taken as evidence for the preservation of an intact endothelium. For the relaxation studies, an equal submaximal concentration of PE (2 μM) was used in both males and females.
Aortic rings were precontracted with PE (2 μM), which represented a concentration that produced 80% of the maximal effect (EC80). The concentration response curves (CRCs) were obtained by the addition of increasing concentrations of ACh (10–8 to 10–5 M). In addition, CRCs to ACh were obtained before and after 20 min incubation with indomethacin (Indo; 10 μM; dissolved in DMSO), a cyclooxygenase (COX) inhibitor, Indo plus 1H-[1,2,4] oxadiazolo [4,3-a] quinoxalin-1-one (ODQ; 10 μM), an inhibitor of soluble guanylate cyclase (sGC), and finally after incubation with Indo, ODQ, and N-nitro-L-arginine (L-NNA; 100 μM), a non-selective NO synthase (NOS) inhibitor. Tissues were washed with Krebs buffer between each CRC to allow the rings to return to basal tone.
CRCs to SNP (10–9 to 10–5 M), a NO-donor, were obtained in endothelium-denuded aortic rings pre-contracted with PE (2 μM) taken from all experimental groups.
The constrictor CRCs to PE (10–8 to 10–5 M) were generated before and after incubation with Nω -nitro-L-arginine methyl ester (L-NAME, 200 μM), a NOS inhibitor, in the presence of Indo (10 μM, dissolved in DMSO), a COX inhibitor. Tissues were washed with Krebs buffer between each CRC to allow the rings to return to basal tone. A vehicle-only (no drugs present) study was performed simultaneously in aortic rings from the same animal (data not shown).
Aortic tissue samples were micronized through freezing with liquid nitrogen and grinding with a mortar and pestle as previously described (Baena et al., 2015). To obtain total protein extract, samples were incubated with RIPA buffer (Sigma-Aldrich, St. Louis, MO, United States) containing Protease Inhibitor Cocktail (UltraCruz, Santa Cruz Biotechnology, Dallas, TX, United States) for 1.5 h at 4°C and centrifuged at 15,000 × g for 15 min at 4°C, and supernatants were collected. Protein concentrations were determined by the bicinchoninic acid (BCA) assay.
Protein (20–30 μg) was subjected to sodium dodecyl sulfate polyacrylamide gel electrophoresis (SDS-PAGE). Proteins were then transferred to 0.45-μm nitrocellulose membranes (Bio Rad Laboratories Inc., Hercules, CA, United States), blocked for 1 h at room temperature with 5% w/v BSA in 0.1% Tween-20 Tris-buffered saline (TBS), and incubated overnight at 4°C with primary antibodies. All primary antibodies were diluted 1:1000 unless otherwise noted. Primary antibodies for endothelial NO synthase (eNOS), phospho-eNOS (p-eNOS) (Ser-1177), V-akt murine thymoma viral oncogene homolog-2 (Akt), phospho-AKT(p-AKT) (Ser-473), and insulin receptor substrates 1 and 2 (IRS-1 and IRS-2) were supplied by Cell Signaling (Boston, MA, United States). Antibodies against NOX1, NOX4, KCNN3 (SKCa), and KCNN4 (IKCa) were obtained from Abcam (Cambridge, MA, United States). Incubation with secondary antibody (LI-COR donkey anti-Rabbit IgG IRDye 680 or anti-mouse IgG IRDye 800CW, 1:10,000) was performed in the blocking buffer for 1 h at room temperature. Before analyzing, the membrane was washed four times with TBS containing 0.1% Tween-20. Detection was done by using a LI-COR Odyssey imaging system (Lincoln, NE, United States). The bands were quantified by densitometry using Image Studio Lite software. To confirm the uniformity of protein loading, blots were incubated with GAPDH and β-actin antibodies (Cell Signaling, Boston, MA, United States) and were normalized for GAPDH and β-actin (data expressed as fold change from control group).
Aortas were cut open lengthwise to expose the endothelial surface. Vessels were incubated in a collagenase II (Worthington) solution (2 mg/ml in DMEM) at 37°C with the endothelial surface facing down for 30 min. Collagenase was blocked 1:1 with complete endothelial cell growth medium [DMEM supplemented with 10% fetal bovine serum, 1% Antibiotic-Antimycotic solution (Gibco, MA), 4 μg/ml endothelial cell growth supplement (Corning, 354006), 1% Non-essential amino acids (Gibco, MA), and 10 mM HEPES (Gibco, MA)]. The endothelial surface of each vessel was scraped into fibronectin-coated tissue culture dishes containing complete medium. Cultures were expanded and frozen at passage 1. The endothelial phenotype of the preparation was confirmed by evaluating cellular uptake of the endothelium-specific marker DiI-acetylated low-density lipoprotein. Experiments were conducted in cells obtained from three control and three diabetic male and four control and four diabetic female rats. The day before the experiments, cells were plated in commercial endothelial cell growth medium (ScienCell, CA) supplemented with 25 mM glucose (ScienCell, CA).
H2O2 generation was measured using Amplex Red and horseradish peroxidase (HRP) as previously described (Vázquez-Medina et al., 2016). Briefly, cells were incubated with 50 μM Amplex Red (Thermo Fisher, MA) and 2.5 U/ml HRP (Sigma) for 30 min at 37°C. The medium was collected, and absorbance was measured at 572 nm. At the end of the experiments, the cells were dissociated from the dishes. Protein content was measured by the BCA gold assay (Thermo Scientific, MA) and results were normalized to protein content. Intracellular oxidant generation was monitored by fluorescence microscopy using CellROX reagent (Thermo Scientific, MA). Cells loaded with 5 μM CellROX and NucBlue (Thermo Fisher, MA) were incubated for 30 min at 37°C. Cells were rinsed three times and imaged using an inverted fluorescence microscope (Zeiss Axio Observer) fitted with a 20× objective and Zen software. Fluorescence intensity in five fields per sample was quantified using ImageJ (NIH) and normalized to cell number.
All values are expressed as mean ± standard error of the mean (SEM). Relaxation responses to each concentration of ACh and SNP were calculated as the percentage of relaxation from maximum PE contraction. Similarly, the recorded increase in the force of contraction was calculated as the percentage of maximum contraction obtained with PE at the highest dose or as changes in tension with increasing concentration of PE in the aortic rings. The concentration of agonist that produced half of the maximum effect (Emax) was expressed as EC50 and calculated by a sigmoidal dose–response model (for variable slope) using GraphPad Prism v7 (GraphPad Software Inc., San Diego, CA, United States). Sensitivity to each agonist was expressed as pD2 values (−log [EC50]), which were normally distributed. The area under the curve (AUC) was determined using GraphPad Prism 8 with trapezoidal technique. To compare the effect of different EDRFs such as COX, the ACh results were expressed as differences in the area under the concentration response curve (ΔAUC) between control (absence of Indo) and experimental (presence of Indo) conditions. One-way ANOVA was used to compare means among experimental groups (e.g., EC50, Emax, and metabolic data). When the one-way ANOVA test returned p < 0.05, post hoc analyses were performed using Tukey’s test. Comparison of CRCs between two groups was done using two-way ANOVA, with one factor being concentration and the other being group (male vs. female and control vs. diabetic). When the ANOVA test returned p < 0.05, post hoc analyses were performed using Bonferroni’s or Tukey’s test. Comparison of CRCs in a pre/post-test format within a group was done using two-way ANOVA with repeated measures. Three-way ANOVA with factors being sex, diabetes, and drugs (male vs. female, diabetic vs. non-diabetic and no drug vs. drug treatment) was used to compare group means. When the ANOVA test returned p < 0.05, post hoc analyses were performed using Tukey’s test.
For ROS generation assays, means were compared between control and diabetic groups using unpaired Student’s t-tests for both males and females. Normality was confirmed using the Shapiro–Wilk test. Statistical analyses were conducted using GraphPad Prism v8.4.3. Values were considered different when p < 0.05. Student’s unpaired t-test was used for comparisons of two group means (e.g., protein expression studies).
Body weights of both male and female diabetic rats were significantly higher compared with the respective non-diabetic controls (Table 1). Accordingly, the weight of intra-abdominal adipose tissue, as well as its ratio to body weight (adiposity), was higher in diabetic rats than in non-diabetic control groups for both sexes. Moreover, male and female UCD-T2DM rats had higher triglyceride levels in plasma than did the respective non-diabetic controls. When compared to male UCD-T2DM rats, female UCD-T2DM rats had significantly higher circulating triglyceride levels and adiposity. Furthermore, both fasting glucose and HbA1c levels were higher in male and female diabetic rats compared to their respective non-diabetic controls. Fasting plasma insulin concentration was significantly higher in female diabetic rats compared with those in both the non-diabetic female control and male diabetic groups (Table 1). Similar to the previous report (Shaligram et al., 2020), there was no difference in plasma insulin levels in male diabetic rats when compared with their respective non-diabetic controls. However, the ISI was significantly lower in diabetic groups, regardless of sex, indicating that insulin signaling may be impaired in diabetic groups of both sexes. When compared to male UCD-T2DM rats, female UCD-T2DM rats had a lower ISI (Table 1).
Table 1. Body weight and adipose weight, blood glucose levels, HbA1c, and other metabolic parameters of male and female control and diabetic rats.
The reduced ISI observed in the current study prompted us to analyze the expression of the main insulin signal transducers, insulin receptor substrate-1 (IRS-1) and insulin receptor substrate-2 (IRS-2), in aortic tissue. As shown in Figure 1A, IRS-1 expression was significantly reduced in both male and female diabetic groups (by 0.5-fold and 0.6-fold in male and female diabetic groups, respectively). In contrast, only the female diabetic group displayed reduced IRS-2 protein expression (0.5-fold) compared to the sex-specific non-diabetic control (Figure 1B).
Figure 1. Western blot analysis of IRS1 and IRS2 expression in control and UCD-T2DM rat aorta. Protein levels of (A) aortic insulin receptor substrate-I (IRS1) and (B) insulin receptor substrate-2 (IRS2) from the samples of male and female control and diabetic rats. IRS1 (A) and IRS2 (B) were quantified by densitometry and normalized to corresponding beta actin. Values are represented as mean ± SEM. Each bar represents the values obtained from n = 4–5 animal s per group. To show representative bands, images from different parts of the same gel have been juxtaposed, indicated by white dividing lines. Capped lines indicate significant differences between two groups (p < 0.05), as analyzed by unpaired Student’s t-test.
A sex difference was observed in aortic relaxation responses to ACh in non-diabetic control rats. Both sensitivity, as assessed by −log [EC50] (pD2) value, and Emax to ACh were significantly higher in female than in male aortas (Table 2). In controls, the pD2 to ACh was 6.57 ± 0.1 in male and 7.20 ± 0.1 in female aortas; the ACh Emax was 81.14% ± 1.6% in male and 94.72% ± 1.9% in female aortas (n = 6–12 per group, p < 0.05, one-way ANOVA).
Table 2. pD2 and Emax to acetylcholine (ACh) in aortic rings from male and female control and diabetic rats.
When compared to the male non-diabetic control group, a potentiated relaxation response to ACh was observed in the male diabetic group (Figure 2A). Both the pD2 and Emax of aortic rings to ACh were significantly enhanced in the male diabetic group (Table 2). The pD2 to ACh was 6.57 ± 0.1 in control males and 7.14 ± 0.0 in diabetic males; the ACh Emax was 81.14% ± 1.6% in control males and 94.04% ± 0.9% in diabetic males (n = 11–12 per group, p < 0.05, one-way ANOVA). However, the Emax but not the sensitivity of aortic rings to ACh was reduced slightly but significantly in the female diabetic group compared to its respective control and the diabetic male group (Figure 2B and Table 2). The ACh Emax was 94.7% ± 1.9% in control females and 85.9% ± 2.0% in diabetic females (n = 6–7 per group, p < 0.05, one-way ANOVA).
Figure 2. Concentration-response curves to acetylcholine (ACh) in control and UCD-T2DM rat aorta. Relaxation responses to cumulative concentrations of ACh (10–8 to 10–5 M) in intact aortic rings pre-contracted with phenylephrine (PE, 2 μM) from male (A) and female (B) control and diabetic rats. Data are expressed as mean ± SEM. n = 6–12 animals per group. *p < 0.05 between two groups analyzed using two-way ANOVA followed by Bonferroni’s post hoc test.
The relative contributions of PGI2, cGMP, and NO to vasorelaxation induced by ACh were estimated by sequentially inhibiting COX, sGC, and NOS. Specifically, EDV to ACh (10–8 to 10–5 M) in rat aortic rings pre-contracted with PE (2 μM) was obtained before and after pretreatment with Indo (10 μM), followed by the addition of ODQ (10 μM) and L-NNA (100 μM). When ODQ was added, the EDV reduction is thought to represent the impact of NO-dependent cGMP on EDV (Pieper and Siebeneich, 1997). Finally, addition of L-NNA, after inhibition of sGC by ODQ, represents the impact of NO independent of cGMP (Bolotina et al., 1994), and the slight remaining EDV to ACh is referred to as the L-NNA, Indo-insensitive component-type relaxation (Feletou and Vanhoutte, 1988).
The administration of Indo to block COX activity had no apparent effects on pD2 and Emax to ACh, regardless of sex or diabetes status. The ΔAUC, defined as the difference in the AUC between the CRC to ACh before and after Indo, was not different between UCD-T2DM groups and respective non-diabetic control groups in either sex (Figures 3A–D and Table 3). Addition of ODQ completely blocked the remaining relaxation in all experimental groups except for the male diabetic group (Figure 3B). After adding ODQ, a slight but significant relaxation response remained in aortic rings of the male diabetic group compared to the control group (Figure 3B vs. Figure 3A). The Emax to ACh in male control and male diabetic aortas was 2.22% ± 0.2% and 10.14% ± 0.5%, respectively (p < 0.05, one-way ANOVA) (Table 3, third column). To examine whether the slight residual ACh-induced relaxation in male diabetic aortas may be due to the direct action of NO (independent of cGMP), a NOS inhibitor was used. The addition of L-NNA, a non-selective NOS inhibitor, had no apparent effect on the remaining Indo- and ODQ-resistant relaxation in male diabetic aortas (Figure 3B). After the addition of L-NNA, there was still a significant difference in the Emax to ACh between male control and male diabetic groups. The Emax to ACh was 0.43% ± 0.4% in male control and 13.38% ± 1.2% in male diabetic rats (p < 0.05, one-way ANOVA) (Table 3, fourth column). The remaining AUC after addition of L-NNA in the male diabetic group was significantly different from the male control group, suggesting a slight role of NO-PGI2-independent relaxation responses in this group (Table 3, fourth column, Figure 3B, gray shaded area).
Figure 3. Endothelium-derived relaxing factors (EDRF) contribution to acetylcholine (ACh)− induced relaxation responses in control and UCD-T2DM rat aorta. Effects of inhibiting cyclooxygenase, soluble guanylyl cyclase and nitric oxide synthase on ACh-induced vasorelaxation in aortic rings taken from (A) male control and (B) male diabetic, (C) female control and (D) female diabetic rats. ACh relaxation was measured in the presence of indomethacin (Indo, 10 μM), followed by addition of ODQ (10 μM), and then with N-nitro-L-arginine (L-NNA; 100 μM). Data are expressed as mean ± SEM. *p < 0.05 vs. no drug; #P < 0.05 vs. Indo; analyzed using two-way ANOVA with repeated measures followed by Bonferroni post hoc test (n = 5–8 per group). Light gray shaded area: contribution of endothelium-derived hyperpolarizing factor (EDHF)-type to endothelium-dependent vasorelaxation (EDV).
Table 3. Area under the curve (ΔAUC), Sensitivity (pD2: -logEC50) and maximum response (Emax) to ACh in rat aortic rings from male and female control and diabetic rats.
It is well known now that smooth muscle hyperpolarization results indirectly from the opening of endothelial SKCa and IKCa channels (McNeish et al., 2006). Therefore, an elevated NO-PGI2-independent-type relaxation in aortas from the male diabetic group could be expected to result from significant overexpression of these hyperpolarizing KCa channels on the endothelium (Gillham et al., 2007). Next, Western blot analysis revealed that the expression of both SKCa and IKCa was significantly upregulated (by 9.0-fold and by 1.0-fold, respectively) in the aortic tissues from male diabetic rats compared with those in non-diabetic controls (Figures 4A,B).
Figure 4. Western blot analysis IKCa and SKCa expression in control and UCD-T2DM rat aorta. Protein levels of (A) aortic intermediate conductance calcium activated potassium channel (IKCa) and (B) small conductance calcium activated potassium channels (SKCa) were measured from the samples of male control and diabetic rats. IKCa (A) and SKCa (B) were quantified by densitometry and normalized to corresponding GAPDH. Values are represented as mean ± SEM. Each bar represents the values obtained from n = 4–5 per group. To show representative bands, images from different parts of the same gel have been juxtaposed, indicated by white dividing lines. Capped lines indicate significant differences between two groups (p < 0.05), as analyzed by unpaired Student’s t-test. MC, male control; MD, male diabetic.
The smooth muscle sensitivity to NO was investigated by generating CRC to SNP (10–9 to 10–5 M) in endothelium-denuded aortic rings. No significant changes in either pD2 values or Emax of SNP were observed in diabetic animals of either sex. The pD2 values to SNP was 8.06 ± 0.0 in male control, 8.19 ± 0.1 in male diabetic, 8.50 ± 0.0 in female control, and 8.32 ± 0.0 in female diabetic animals. The Emax to SNP in male control and male diabetic animals was 100.15% ± 0.3% and 101.46% ± 1.4%, respectively. The Emax to SNP in female control and female diabetic animals was 104.34% ± 3.2% and 100.18% ± 0.4%, respectively.
Contractile responses to an α-adrenoceptor agonist (PE) were analyzed by measuring the CRC to PE (10–8 to 10–5 M). There were no sex differences in PE contractile responses in aortic rings from non-diabetic control groups (Figure 5). However, both maximal tension developed in response to PE (Tensionmax) and the sensitivity to PE in aortic rings were significantly enhanced in aortic rings of diabetic groups compared with the non-diabetic control rats, regardless of sex (Figures 5A,B and Table 4).
Figure 5. Concentration-response curves to phenylephrine (PE) in control and UCD-T2DM rat aorta. Contractile responses to cumulative concentrations of PE (10–8 to 10–5 M) in intact aortic rings of (A) male and (B) female control and diabetic rats. Data are expressed as mean ± SEM. n = 5–6 per group. *p < 0.05 between two groups analyzed using two-way ANOVA followed by Bonferroni’s post hoc test.
Table 4. pD2 and Tensionmax to phenylephrine (PE) in aortic rings from male and female control and diabetic rats.
Next, the CRC to PE (10–8 to 10–5 M) was determined in aortic rings before and after pretreatment with the NO synthase inhibitor, L-NAME (200 μM), in the presence of Indo (10 μM). The changes in the contractile response to PE after the addition of L-NAME reveal the role of endothelium-derived NO during smooth muscle contraction in response to PE, as previously reported by us (Zhang et al., 2012; Sangüesa et al., 2017) and others (Hayashi et al., 1992; Dora et al., 2000).
The administration of Indo to block COX metabolites slightly but significantly reduced Emax to PE in aortas from male control and female diabetic groups, suggesting a slight elevation of the contractile metabolite of COX in this group, with no apparent effect on the maximal contractile response in female control and male diabetic groups (Figure 6 and Table 5). The addition of L-NAME resulted in a significant increase of the contractile responses to PE in all experimental groups (Figure 6). However, ΔAUC (the difference in AUC between PE CRC before and after L-NAME) was lower in aortas of the diabetic rats compared with the control group, regardless of sex (Table 5).
Figure 6. Concentration-response curves to phenylephrine (PE) in intact aortic rings from (A) male control, (B) male diabetic, (C) female control, and (D) female diabetic rats. Contraction to PE was measured in absence of any drug (ND) or in presence of indomethacin (Indo, 10 μM) followed by addition of N-Nitro-L-arginine methyl ester (Indo + L-NAME, 200 μM). Results are expressed as a percent of the maximal response to PE (10 μM) obtained in the absence of any drug. Data are expressed as mean ± SEM, analyzed using two-way ANOVA with repeated measures followed by Tukey’s post hoc test: ∗p < 0.05 vs. ND; #p < 0.05 vs. Indo, n = 6–8 per group.
Table 5. Emax, Tensionmax, pD2, and ΔAUC to phenylephrine (PE) in aortic rings from male and female control and diabetic rats.
To investigate the mechanism by which endothelium-derived NO generation in response to PE might be reduced in diabetic animals, eNOS activation by phosphorylation was investigated by Western blot analysis. As shown in Figure 7B, the phosphorylation of eNOS at Ser-1177 was significantly reduced in aortic tissue from diabetic rats relative to controls, regardless of sex. Although the expression of total eNOS showed no significant difference between diabetic males and controls, its levels were significantly reduced in aortas from diabetic females (Figure 7A).
Figure 7. Western blot analysis of eNOS and peNOS expression in control and UCD-T2DM rat aorta. Protein levels of (A) total endothelial nitric oxide synthase (eNOS) and (B) phosphorylated eNOS (peNOS) in aortic samples from male and female control and diabetic rats. eNOS (A) and peNOS (B) were quantified by densitometry and normalized to corresponding beta actin. Each bar represents the mean ± SEM of values obtained from n = 4–5 animals per group. To show representative bands, images from different parts of the same gel have been juxtaposed, indicated by white dividing lines. Capped lines indicate significant differences between two groups (p < 0.05), as analyzed by unpaired Student’s t-test.
Vascular dysfunction could also be related to insulin resistance, and our results suggest that insulin signaling could be impaired in diabetic rats in both sexes. Next, we determined the expression of pAkt, which is downstream of IRS and an upstream mediator of eNOS phosphorylation at Ser-1177 in aortic tissues. Figure 8B shows that pAkt (Ser-473) content was significantly reduced in the aorta of diabetic rats compared with the control groups for both sexes, whereas total Akt protein content remained unaffected by diabetes status, regardless of sex (Figure 8A).
Figure 8. Western blot analysis of Akt and pAkt expression in control and UCD-T2DM rat aorta. Protein levels of aortic (A) total V-akt murine thymoma viral oncogene homolog-2 (Akt) and (B) phosphorylated V-akt murine thymoma viral oncogene homolog-2 (pAkt) from the samples of male and female control and diabetic rats. Akt (A) and pAkt (B) were quantified by densitometry and normalized to corresponding GAPDH. Each bar represents the mean ± SEM of values obtained from n = 4–5 animals per group. To show representative bands, images from different parts of the same gel have been juxtaposed, indicated by white dividing lines. Capped lines indicate significant differences between two groups (p < 0.05), as analyzed by unpaired Student’s t-test.
To further investigate the possible mechanisms underlying the elevated responses to contractile agents in this model, the protein expression of NADPH oxidase (NOX) subtypes NOX1 and NOX4 was measured. NOX1 expression was significantly elevated in aortic tissues from diabetic groups, regardless of sex (1.5-fold in male diabetic and 1-fold in female diabetic rats, Figure 9A). However, NOX4 expression showed no significant differences among all experimental groups (Figure 9B).
Figure 9. Western blot analysis of NOX1 and NOX4 expression in intact aorta, and oxidant generation in primary aortic endothelial cells isolated from control and UCD-T2DM rats. Protein levels of aortic NADPH oxidases (NOX1) (A) and NOX4 (B) from the samples of male and female control and diabetic rats. NOX1 and NOX4 were quantified by densitometry and normalized to corresponding GAPDH. To show representative bands, images from different parts of the same gel have been juxtaposed, indicated by white dividing lines. Hydrogen peroxide (H202) (C) and intracellular oxidant (CellROX oxidation) (D) generation in primary aortic endothelial cells isolated from male and female control and diabetic rats. Values are presented as mean ± SEM. Each bar represents the values obtained from n = 4–5 animals per group for NOX expression and n = 3–4 animals per group for oxidant generation studies. Capped lines indicate significant differences between two groups (p < 0.05), analyzed by unpaired Student’s t-test.
Lastly, to examine whether the elevated expression of NOX1 in aortic tissues of diabetic rats was associated with elevated basal ROS levels in this group, intracellular and extracellular ROS generation was measured in primary endothelial cells isolated from aortic tissues using Amplex Red and CellROX assays. Both assays demonstrated that ROS generation was higher in the endothelial cells isolated from arteries of diabetic groups compared with those in the non-diabetic controls, regardless of sex (Figures 9C,D).
The present study demonstrates that aortic function in UCD-T2DM rats is altered in both sexes. It also provides the first evidence of sexual dimorphism in aortic relaxation in UCD-T2DM rats.
In the current study, while both male and female diabetic rats had higher body weight and hyperglycemia compared with non-diabetic control rats, the female diabetic group exhibited higher adiposity, triglyceride, and insulin levels than control or male diabetic rats. This is consistent with the results from our previous study (Shaligram et al., 2020). Similarly, Ohta et al. (2014) which reported elevated blood insulin levels in spontaneously diabetic torii (SDT) female rats compared with SDT male rats. In the current study, the ISI was lowered in diabetic groups, irrespective of sex. However, when compared to male diabetic rats, female diabetic rats exhibited a lower ISI. Accordingly, here, we showed that insulin signaling was impaired in the aortic tissues in diabetic groups in both sexes. Notably, aortic IRS-1 was reduced to a similar extent in both diabetic groups, but IRS-2 was reduced only in the female diabetic group. It has been reported that the downregulation of IRS-2 levels in endothelial cells is induced by hyperinsulinemia in obese subjects (Kubota et al., 2011). Similarly, our results on elevated insulin levels in female diabetic rats may suggest that the decreased IRS-2 expression observed in the aorta could be in part due to hyperinsulinemia in this group.
In T2D, impaired (Sakamoto et al., 1998), enhanced (Zhong et al., 2012), or preserved (Bohlen and Lash, 1995) EDV has been reported. Here, a slight but significant decrease in maximum relaxation to ACh was observed in aortic rings from female UCD-T2DM rats compared to their respective controls. However, an intriguing observation of this study was that aortic rings from male diabetic animals exhibited a potentiation in EDV compared with that in male controls. Similar observations were also made by our group using Zucker diabetic fatty (ZDF) male rats. Specifically, obesity-induced diabetes (ZDF model) significantly impaired relaxation responses to ACh in aortic rings taken from females, but potentiated the relaxation in males (data not shown). In accordance with our current study, Zhong et al. (2012) reported elevated relaxation responses to ACh in aortic rings of GK male rats. On the other hand, Kazuyama et al. (2009) and Nemoto et al. (2011) reported an impaired EDV in aortic rings from GK male rats.
It has been well established that in conduit arteries, NO plays a major role in EDV (Shimokawa et al., 1996; Gao et al., 2011). The impaired EDV may result from either a decreased NO production or an increased inactivation of NO by ROS. It has been reported that T2D reduces the synthesis of NO in rat aorta by phosphorylation of eNOS at Ser-1177 (Nemoto et al., 2011). Here, we did not directly measure NO production, but our data show that the expression of the active, phosphorylated form of eNOS is decreased while the expression of NOX1 and ROS generation are increased in aortas from diabetic groups in both sexes, suggesting that decreased NO bioavailability may in part contribute to reduced responses to ACh in diabetic female arteries. However, elevated ACh responses in diabetic male aorta cannot be attributed to decreased NO due to decreased eNOS activation or elevation of ROS, suggesting that other factors may be involved.
There is an established negative regulatory effect of NO on EDHF synthesis (Bauersachs et al., 1996; Brandes et al., 2000), and an augmented EDHF response was also shown to compensate for the loss of NO in arteries in diabetic rats (Garland et al., 1995; Malakul et al., 2008). In agreement with those studies that demonstrate compensatory interactions between pathways, the potentiation of the ACh response (regardless of decreased eNOS activity) in aortic rings from the male diabetic group suggests that other vasodilatory molecules besides NO may be involved in ACh relaxation in this group.
In the present study, we showed that the inhibition of COX metabolites by Indo did not alter relaxation responses to ACh significantly in aortic rings of any of the four experimental groups. Consistent with these results, Malakul et al. (2008) reported that hypercholesterolemia and type 1 diabetes did not have any effect on COX-mediated EDV in rat aortas. Here, addition of ODQ completely abolished the EDV in aortic rings of control groups of both sexes as well as female, but not male, diabetic groups, suggesting that EDV is solely mediated by NO acting on the sGC (NO-dependent cGMP) pathway in the above-mentioned groups. Although the vasodilatory effect of NO on vascular smooth muscle is mainly mediated by cGMP (via a cGMP-dependent K+-channel activation) (Taylor et al., 2001), a direct effect of NO on Ca2+-dependent K+-channels (Bolotina et al., 1994) and L-type calcium current (Summers et al., 1999) without requiring cGMP has also been demonstrated. Here, the slight remaining Indo-ODQ-resistant relaxation in the aorta of male diabetic rats was unaffected by L-NNA, suggesting that NO-independent cGMP does not play a role in EDV in this group. An alternative explanation for the relaxation resistance to sGC and NOS inhibition in male diabetic aortas may be the contribution of other factors (NO- and PGI2-independent) on relaxation in this group. Similarly, Malakul et al. (2008) reported a potential role of EDHF in EDV in aortas of streptozotocin-induced type 1 diabetic male rats. However, they did not include females in their studies to determine whether there was a sex effect in the type 1 diabetic rat aorta. There are also other reports of a decreased NO-dependent relaxation response and increased EDHF activity in saphenous arteries (Chadha et al., 2010) and carotid arteries (Leo et al., 2010) of high-fat diet-induced obese and type 1 diabetic male rats, respectively.
Epidemiological studies suggest that males are at higher risk for CVD compared to age-matched females during their reproductive years (Liu et al., 2003). This sex difference has been attributed to estrogen’s protective effect in females and/or a detrimental androgen effect in males (Thompson and Khalil, 2003). However, a growing body of evidence suggests that androgens exhibit protective actions on the cardiovascular system (Nettleship et al., 2009). Administration of testosterone has been shown to induce both endothelium-dependent and independent vasorelaxation in rabbit aorta (Yue et al., 1995), rat aorta (Costarella et al., 1996), and porcine coronary artery (Crews and Khalil, 1999).
Here, our data show that control female aortas exhibit greater ACh-mediated vasorelaxation compared to male aortas. This is in accordance with our previous report on the sex difference in rat aortic relaxation (Rahimian et al., 1997); however, we have now extended these findings in reporting that under the T2D condition, beneficial effects of female hormones could be lost, yet, intriguingly, male aortas exhibit greater ACh-mediated relaxation.
The KCa currents are mainly mediated by IKCa and SKCa channels (Brähler et al., 2009) in conduit and resistance-sized arteries in many species, including humans (Taylor et al., 2001; Félétou, 2009; Grgic et al., 2009). Taylor et al. (2001) reported that NO-independent relaxations evoked by ACh in rabbit conduit arteries were sensitive to a combination of SKCa and IKCa channel blockers. Although the effects of SKCa and IKCa channel blockers were not examined in the current study design, our data on the significant increase in expression of IKCa and SKCa channels in male diabetic arteries suggest that the slight NO- and PGI2-independent relaxation observed in this group may be associated with these channels. In a preliminary functional study, further examination of IKCa and SKCa, using selective inhibitors of these channels, suggested a role for IKCa in ACh-induced relaxation in aorta of male diabetic rats (data not shown). These results are also in accordance with Schach et al. (2014) who reported an elevation of expression and contribution of IKCa in mesenteric arteries of male ZDF rats. In the current study, we observed no significant differences in the expression of IKCa channels in aorta from female diabetic compared with female control animals (n = 5–6, data not shown). It is also important to note that in aorta from males, the contribution of a NO-independent factor to the ACh response was only observed in the diabetic state and not in the control (or healthy) state. Sandow et al. (2009) reported that EDHF is present in aortas of juvenile rats (Martínez-Orgado et al., 1998) and disease models (such as hypercholesterolemic, diabetic, hypertensive, and with altered estrogen levels), but absent in healthy adult aorta (Kagota et al., 2000; Matsumoto et al., 2004; Woodman and Boujaoude, 2004; Malakul et al., 2008).
Besides the possibility of a modified contribution of NO, alteration of EDV to ACh in aortas of the UCD-T2DM model could be explained by changes in smooth muscle responsiveness to NO or contractile agents. However, our data showed that SNP (a NO donor)-induced relaxation of endothelium-denuded aortic rings was not altered in male or female diabetic groups. Similarly, Nemoto et al. (2011) observed no significant difference in SNP-induced relaxation in aortic rings of male GK rats. This suggests that smooth muscle responsiveness to NO in the aorta was not affected in UCD-T2DM rats. On the other hand, in the current study, the sensitivity and maximum tension to PE were enhanced significantly in aortic rings of UCD-T2DM groups compared with their respective controls, regardless of sex (Figure 5). The elevated PE response may in part explain the slight but significant decrease in the maximum relaxation in ACh responses in aorta of female diabetic rats. However, it is important to note that regardless of increased PE-induced contraction, the ACh response was enhanced in male diabetic arteries. This, therefore, excludes the diminished PE contractile responsiveness as the cause of the increased ACh responses observed in male diabetic arteries. Nevertheless, our data on PE responses are in line with previous findings that type 1 diabetes results in increased vascular contraction in rat aortas (Abebe et al., 1990) and mesenteric arteries (White and Carrier, 1990).
Phenylephrine may indirectly stimulate endothelial cells to release NO via a signal transmitted either through myoendothelial gap junctions (Dora et al., 2000; Jackson et al., 2008) or by mechanical stress (Fleming et al., 1999). Therefore, the elevated contractile responses to PE observed in UCD-T2DM male and female rats may in part result from a decreased release of NO from the endothelium during smooth muscle contraction or an enhanced release of contracting factors (Zhang et al., 2012).
In the current study, we assessed the role of endothelium-derived NO by measuring the difference in the degree of PE-induced contraction in the absence and presence of L-NAME (Hayashi et al., 1992; Han et al., 2016). Pretreatment with L-NAME caused a significantly lower potentiation of the PE response in aortic rings from UCD-T2DM rats, regardless of sex (Figures 6B,D) compared with their controls. This suggests that decreased basal NO activity may in part be responsible for the elevated PE contractile responsiveness in UCD-T2DM rats in both sexes. Here, our study was limited in that we did not directly measure basal NO level. Nevertheless, consistent with an important role for eNOS phosphorylation on serine 1177 by Akt in regulating basal NO release (Scotland et al., 2002; Kobayashi et al., 2004), a reduction in eNOS expression by phosphorylation at Ser-1177 was observed in aortas from diabetic rats in both sexes compared with their controls. Additional studies will be needed to document the direction and magnitude of these interactions along with the relative importance of NO to elevated contractile responses in UCD-T2DM male and female rats.
Insulin resistance is a key element in the pathogenesis of T2D (Ormazabal et al., 2018). Insulin resistance is associated with endothelial dysfunction by several mechanisms including increased production of pro-inflammatory vasoconstrictor factors and oxidative stress (Schneider et al., 2000; Del Turco et al., 2011). Previous studies on experimental models of insulin resistance revealed impaired insulin-mediated PI3K/Akt-dependent signaling in the vasculature (Jiang et al., 1999). Our data on the significant decrease in expression of IRS and pAkt (a downstream mediator of IRS and upstream of eNOS phosphorylation at Ser-1177) in aortic tissues of diabetic animals in both sexes, suggest that the decreased peNOS levels in the diabetic aorta could arise from altered activation by pAkt.
Finally, it has also been reported that in diabetes, oxidative stress and superoxide radical production derived from insulin resistance may play a crucial role in enhancing the contracting responses (Shi et al., 2007). Superoxide scavenges NO, decreasing its bioavailability (Rubanyi et al., 1986), and elevating endothelium-dependent contractions. In the present study, we determined expression of NOX proteins, a source of superoxide. Vascular walls express high levels of NOX1, NOX2, and NOX4 (Griendling et al., 2000). NOX1 is mainly expressed in large conduit vessels (Lassègue et al., 2001), whereas NOX2 is more highly expressed in resistance vessels (Touyz et al., 2002). Here, we observed an elevated expression of NOX1 in aorta from diabetic groups, irrespective of sex, whereas NOX4 expression was not changed. Youn et al. (2012) reported that the activation of NOX1 was associated with eNOS uncoupling and endothelial dysfunction in streptozotocin-induced type 1 diabetic mice aorta. Furthermore, Gray et al. (2013) reported that genetic deletion of NOX1 in diabetic mice led to reduced diabetes mellitus symptoms, suggesting a key role of NOX1-derived ROS in diabetes. Consistent with these results is the observation that ROS generation in aortic primary endothelial cells isolated from diabetic rats was higher than in cells isolated from control animals, regardless of sex. Taken together, our results on NOX1 upregulation and increased ROS generation in diabetic arteries suggest that the elevation of responses to PE observed in diabetic animals in both sexes may be partially due to the reduced NO bioavailability or increased in generation of potential vasoconstrictor substances (such as superoxide anions).
This study represents the first report showing that the aortic function in UCD-T2DM rats is altered in both sexes. Our data suggest that decreased insulin sensitivity, possibly via pAkt-dependent signaling and enhanced oxidative stress, may contribute to the elevated contractile responses in aorta of this model of T2D, regardless of sex. We also showed sex differences in aortic relaxation in this model. Specifically, our data show that under the T2D condition, beneficial effects of female hormones could be lost, yet, intriguingly, male aortas exhibit greater ACh-mediated relaxation. Additional studies will be needed to identify an underlying mechanism for the sex-specific differences observed in the aortic relaxation in this model.
Figure 10 depicts our proposed scheme based on the data presented in this report. Briefly, the elevation of contractile responses in aortic rings of both male and female UCD-T2DM could result from decreased basal NO activity, possibly due to the impaired insulin-mediated pAkt-peNOS dependent signaling and/or increased oxidative stress in this model (A). In the meantime, aortic vasorelaxation was elevated in aortic rings from male UCD-T2DM rats (B), but slightly impaired in female UCD-T2DM rats (C). The elevated vasorelaxation response in aortic rings from male diabetic rats (despite elevated contractile responses in this group) was accompanied by the elevated IKCa and SKCa channel expression and trace a of NO-independent responses (B). However, the decreased relaxation in female diabetic aortas could be in part attributed to the decreased NO activity and elevated contractile responses in this group (C).
Figure 10. Proposed drivers of an elevated contractile response in UCD-T2DM rat aortas. (A green one) Impaired insulin signaling, elevated ROS generation, and decreased basal NO activity may drive elevated contractile responses in aortic rings of both male and female UCD-T2DM rats. (B blue one) Male UCD-T2DM rat aortas display enhanced EDV (despite elevated contractile responses) along with elevated IKCa and SKCa channel expression and traces of NO-independent responses. (C red one) Female UCD-T2DM rat aortas display impaired EDV, possibly due to decreased NO activity and enhanced contractile responses. IRS, insulin receptor substrate; pAkt, phosphorylated V-Akt murine thymoma viral oncogene homolog-2; peNOS, phosphorylated endothelial nitric oxide synthase; NOX, NADPH oxidase; ROS, reactive oxygen species; NO, nitric oxide; IKCa, intermediate-conductance calcium- activated potassium channel; SKca, small-conductance calcium-activated potassium channel; EDV, endothelium-dependent vasorelaxation.
The original contributions presented in the study are included in the article/supplementary material, further inquiries can be directed to the corresponding author/s.
The animal study was reviewed and approved by the University of the Pacific and the University of California, Davis Institutional Animal Care and Use Committee.
FA designed and performed the majority of the experiments and drafted the manuscript. MR and SS assisted with the animal experiments. PH, KS, and JG generated the UCD-T2DM rat models and provided the intellectual input and critical reading of the manuscript. JV-M and KA performed the ROS experiments and provided the intellectual input. KA was also involved in editing the manuscript. RR was involved with the conception and design of research, revising the manuscript, and approving the final draft of manuscript. All the authors contributed to the article and approved the submitted version.
This work was supported by the National Heart, Lung and Blood Institute grant R15HL128988 to RR and the University of the Pacific. PH laboratory received support during the project period from National Institutes of Health Grants HL121324, DK095960, and U24 DK092993.
The authors declare that the research was conducted in the absence of any commercial or financial relationships that could be construed as a potential conflict of interest.
We thank Mujibullah Karimi and Grayson Dillon (undergraduate students) for their help with preparation for experiments.
Abebe, W., Harris, K. H., and MacLeod, K. M. (1990). Enhanced contractile responses of arteries from diabetic rats to α1-adrenoceptor stimulation in the absence and presence of extracellular calcium. J. Cardiovasc. Pharmacol. 16, 239–248.
Aloysius, U. I., Achike, F. I., and Mustafa, M. R. (2012). Mechanisms underlining gender differences in Phenylephrine contraction of normoglycaemic and short-term Streptozotocin-induced diabetic WKY rat aorta. Vascul. Pharmacol. 57, 81–90. doi: 10.1016/j.vph.2011.11.009
Baena, M., Sangüesa, G., Hutter, N., Sánchez, R. M., Roglans, N., Laguna, J. C., et al. (2015). Fructose supplementation impairs rat liver autophagy through mTORC activation without inducing endoplasmic reticulum stress. Biochim. Biophys. Acta 1851, 107–116. doi: 10.1016/j.bbalip.2014.11.003
Bauersachs, J., Popp, R., Hecker, M., Sauer, E., Fleming, I., and Busse, R. (1996). Nitric oxide attenuates the release of endothelium-derived hyperpolarizing factor. Circulation 94, 3341–3347. doi: 10.1161/01.cir.94.12.3341
Bohlen, H. G., and Lash, J. M. (1995). Endothelial-dependent vasodilation is preserved in non-insulin-dependent Zucker fatty diabetic rats. Am. J. Physiol. 268, H2366–H2374. doi: 10.1152/ajpheart.1995.268.6.H2366
Bolotina, V. M., Najibi, S., Palacino, J. J., Pagano, P. J., and Cohen, R. A. (1994). Nitric oxide directly activates calcium-dependent potassium channels in vascular smooth muscle. Nature 368, 850–853. doi: 10.1038/368850a0
Brähler, S., Kaistha, A., Schmidt, V. J., Wölfle, S. E., Busch, C., Kaistha, B. P., et al. (2009). Genetic deficit of SK3 and IK1 channels disrupts the endothelium-derived hyperpolarizing factor vasodilator pathway and causes hypertension. Circulation 119, 2323–2332. doi: 10.1161/CIRCULATIONAHA.108.846634
Brandes, R. P., Schmitz-Winnenthal, F. H., Félétou, M., Gödecke, A., Huang, P. L., Vanhoutte, P. M., et al. (2000). An endothelium-derived hyperpolarizing factor distinct from NO and prostacyclin is a major endothelium-dependent vasodilator in resistance vessels of wild-type and endothelial NO synthase knockout mice. Proc. Natl. Acad. Sci. U.S.A. 97, 9747–9752. doi: 10.1073/pnas.97.17.9747
Brunner, H., Cockcroft, J. R., Deanfield, J., Donald, A., Ferrannini, E., Halcox, J., et al. (2005). Endothelial function and dysfunction. Part II: association with cardiovascular risk factors and diseases. a statement by the working group on endothelins and endothelial factors of the european society of hypertension. J. Hypertens. 23, 233–246. doi: 10.1097/00004872-200502000-00001
Chadha, P. S., Haddock, R. E., Howitt, L., Morris, M. J., Murphy, T. V., Grayson, T. H., et al. (2010). Obesity up-regulates intermediate conductance calcium-activated potassium channels and myoendothelial gap junctions to maintain endothelial vasodilator function. J. Pharmacol. Exp. Ther. 335, 284–293. doi: 10.1124/jpet.110.167593
Costarella, C. E., Stallone, J. N., Rutecki, G. W., and Whittier, F. C. (1996). Testosterone causes direct relaxation of rat thoracic aorta. J. Pharmacol. Exp. Ther. 277, 34–39.
Crews, J. K., and Khalil, R. A. (1999). Antagonistic effects of 17 beta-estradiol, progesterone, and testosterone on Ca2+ entry mechanisms of coronary vasoconstriction. Arterioscler. Thromb. Vasc. Biol. 19, 1034–1040. doi: 10.1161/01.atv.19.4.1034
Cummings, B. P., Digitale, E. K., Stanhope, K. L., Graham, J. L., Baskin, D. G., Reed, B. J., et al. (2008). Development and characterization of a novel rat model of type 2 diabetes mellitus: the UC Davis type 2 diabetes mellitus UCD-T2DM rat. Am. J. Physiol. Regul. Integr. Comp. Physiol. 295, R1782–R1793. doi: 10.1152/ajpregu.90635.2008
De Vriese, A. S., Verbeuren, T. J., Van de Voorde, J., Lameire, N. H., and Vanhoutte, P. M. (2000). Endothelial dysfunction in diabetes. Br. J. Pharmacol. 130, 963–974. doi: 10.1038/sj.bjp.0703393
Del Turco, S., Navarra, T., Gastaldelli, A., and Basta, G. (2011). Protective role of adiponectin on endothelial dysfunction induced by AGEs: a clinical and experimental approach. Microvasc. Res. 82, 73–76. doi: 10.1016/j.mvr.2011.03.003
Dora, K. A., Hinton, J. M., Walker, S. D., and Garland, C. J. (2000). An indirect influence of phenylephrine on the release of endothelium-derived vasodilators in rat small mesenteric artery. Br. J. Pharmacol. 129, 381–387. doi: 10.1038/sj.bjp.0703052
Drummond, G. R., and Sobey, C. G. (2014). Endothelial NADPH oxidases: which NOX to target in vascular disease? Trends Endocrinol. Metab. 25, 452–463. doi: 10.1016/j.tem.2014.06.012
Edwards, G., Dora, K. A., Gardener, M. J., Garland, C. J., and Weston, A. H. (1998). K + is an endothelium-derived hyperpolarizing factor in rat arteries. Nature 396, 269–272. doi: 10.1038/24388
Félétou, M. (2009). Calcium-activated potassium channels and endothelial dysfunction: therapeutic options? Br. J. Pharmacol. 156, 545–562. doi: 10.1111/j.1476-5381.2009.00052.x
Félétou, M. (2011). The Endothelium: Part 1: Multiple Functions of the Endothelial Cells—Focus on Endothelium-Derived Vasoactive Mediators. San Rafael, CA: Morgan & Claypool Life Sciences.
Feletou, M., and Vanhoutte, P. M. (1988). Endothelium-dependent hyperpolarization of canine coronary smooth muscle. Br. J. Pharmacol. 93, 515–524. doi: 10.1111/j.1476-5381.1988.tb10306.x
Fleming, I., Bauersachs, J., Schafer, A., Scholz, D., Aldershvile, J., and Busse, R. (1999). Isometric contraction induces the Ca2+-independent activation of the endothelial nitric oxide synthase. Proc. Natl. Acad. Sci. U.S.A. 96, 1123–1128. doi: 10.1073/pnas.96.3.1123
Gao, X., Martinez-Lemus, L. A., and Zhang, C. (2011). Endothelium-derived hyperpolarizing factor and diabetes. World J. Cardiol. 3, 25–31. doi: 10.4330/wjc.v3.i1.25
Garland, C. J., Plane, F., Kemp, B. K., and Cocks, T. M. (1995). Endothelium-dependent hyperpolarization: a role in the control of vascular tone. Trends Pharmacol. Sci. 16, 23–30. doi: 10.1016/s0165-6147(00)88969-5
Gillham, J. C., Myers, J. E., Baker, P. N., and Taggart, M. J. (2007). Regulation of endothelial-dependent relaxation in human systemic arteries by SKCa and IKCa channels. Reprod. Sci. 14, 43–50. doi: 10.1177/1933719106298197
Gray, S. P., Di Marco, E., Okabe, J., Szyndralewiez, C., Heitz, F., Montezano, A. C., et al. (2013). NADPH oxidase 1 plays a key role in diabetes mellitus-accelerated atherosclerosis. Circulation 127, 1888–1902. doi: 10.1161/CIRCULATIONAHA.112.132159
Grgic, I., Kaistha, B. P., Hoyer, J., and Köhler, R. (2009). Endothelial Ca+-activated K+ channels in normal and impaired EDHF-dilator responses–relevance to cardiovascular pathologies and drug discovery. Br. J. Pharmacol. 157, 509–526. doi: 10.1111/j.1476-5381.2009.00132.x
Griendling, K. K., Sorescu, D., and Ushio-Fukai, M. (2000). NAD(P)H oxidase: role in cardiovascular biology and disease. Circ. Res. 86, 494–501. doi: 10.1161/01.res.86.5.494
Griffith, T. M., Chaytor, A. T., Taylor, H. J., Giddings, B. D., and Edwards, D. H. (2002). cAMP facilitates EDHF-type relaxations in conduit arteries by enhancing electrotonic conduction via gap junctions. Proc. Natl. Acad. Sci. U.S.A. 99, 6392–6397. doi: 10.1073/pnas.092089799
Han, X., Shaligram, S., Zhang, R., Anderson, L., and Rahimian, R. (2016). Sex-specific vascular responses of the rat aorta: effects of moderate term (intermediate stage) streptozotocin-induced diabetes. Can. J. Physiol. Pharmacol. 94, 408–415. doi: 10.1139/cjpp-2015-0272
Han, X., Zhang, R., Anderson, L., and Rahimian, R. (2014). Sexual dimorphism in rat aortic endothelial function of streptozotocin-induced diabetes: possible involvement of superoxide and nitric oxide production. Eur. J. Pharmacol. 723, 442–450. doi: 10.1016/j.ejphar.2013.10.052
Hayashi, T., Fukuto, J. M., Ignarro, L. J., and Chaudhuri, G. (1992). Basal release of nitric oxide from aortic rings is greater in female rabbits than in male rabbits: implications for atherosclerosis. Proc. Natl. Acad. Sci. U.S.A. 89, 11259–11263. doi: 10.1073/pnas.89.23.11259
Jackson, W. F., Boerman, E. M., Lange, E. J., Lundback, S. S., and Cohen, K. D. (2008). Smooth muscle α1D-adrenoceptors mediate phenylephrine-induced vasoconstriction and increases in endothelial cell Ca2+ in hamster cremaster arterioles. Br. J. Pharmacol. 155, 514–524. doi: 10.1038/bjp.2008.276
Jiang, Z. Y., Lin, Y. W., Clemont, A., Feener, E. P., Hein, K. D., Igarashi, M., et al. (1999). Characterization of selective resistance to insulin signaling in the vasculature of obese Zucker (fa/fa) rats. J. Clin. Invest. 104, 447–457. doi: 10.1172/JCI5971
Kagota, S., Yamaguchi, Y., Nakamura, K., and Kunitomo, M. (2000). Altered endothelium-dependent responsiveness in the aortas and renal arteries of Otsuka Long–Evans Tokushima Fatty (OLETF) rats, a model of non-insulin-dependent diabetes mellitus. Gen. Pharmacol. Vasc. Syst. 34, 201–209. doi: 10.1016/S0306-3623(00)00061-6
Kazuyama, E., Saito, M., Kinoshita, Y., Satoh, I., Dimitriadis, F., and Satoh, K. (2009). Endothelial dysfunction in the early- and late-stage type-2 diabetic Goto-Kakizaki rat aorta. Mol. Cell. Biochem. 332, 95–102. doi: 10.1007/s11010-009-0178-2
Kleinert, M., Clemmensen, C., Hofmann, S. M., Moore, M. C., Renner, S., Woods, S. C., et al. (2018). Animal models of obesity and diabetes mellitus. Nat. Rev. Endocrinol. 14, 140–162. doi: 10.1038/nrendo.2017.161
Kobayashi, T., Taguchi, K., Yasuhiro, T., Matsumoto, T., and Kamata, K. (2004). Impairment of PI3-K/Akt pathway underlies attenuated endothelial function in aorta of type 2 diabetic mouse model. Hypertension 44, 956–962. doi: 10.1161/01.HYP.0000147559.10261.a7
Kubota, T., Kubota, N., Kumagai, H., Yamaguchi, S., Kozono, H., Takahashi, T., et al. (2011). Impaired insulin signaling in endothelial cells reduces insulin-induced glucose uptake by skeletal muscle. Cell Metab. 13, 294–307. doi: 10.1016/j.cmet.2011.01.018
Lassègue, B., Sorescu, D., Szöcs, K., Yin, Q., Akers, M., Zhang, Y., et al. (2001). Novel gp91(phox) homologues in vascular smooth muscle cells: nox1 mediates angiotensin II-induced superoxide formation and redox-sensitive signaling pathways. Circ. Res. 88, 888–894. doi: 10.1161/hh0901.090299
Leo, C. H., Hart, J. L., and Woodman, O. L. (2011). Impairment of both nitric oxide-mediated and EDHF-type relaxation in small mesenteric arteries from rats with streptozotocin-induced diabetes. Br. J. Pharmacol. 162, 365–377. doi: 10.1111/j.1476-5381.2010.01023.x
Leo, C. H., Joshi, A., and Woodman, O. L. (2010). Short-term type 1 diabetes alters the mechanism of endothelium-dependent relaxation in the rat carotid artery. Am. J. Physiol. Heart Circ. Physiol. 299, H502–H511. doi: 10.1152/ajpheart.01197.2009
Liu, P. Y., Death, A. K., and Handelsman, D. J. (2003). Androgens and cardiovascular disease. Endocr. Rev. 24, 313–340. doi: 10.1210/er.2003-0005
Malakul, W., Thirawarapan, S., Suvitayavat, W., and Woodman, O. L. (2008). Type 1 diabetes and hypercholesterolaemia reveal the contribution of endothelium-derived hyperpolarizing factor to endothelium-dependent relaxation of the rat aorta. Clin. Exp. Pharmacol. Physiol. 35, 192–200. doi: 10.1111/j.1440-1681.2007.04811.x
Martínez-Orgado, J., González, R., Alonso, M. J., and Marín, J. (1998). Nitric oxide-dependent and -independent mechanisms in the relaxation elicited by acetylcholine in fetal rat aorta. Life Sci. 64, 269–277. doi: 10.1016/S0024-3205(98)00562-1
Matsumoto, T., Wakabayashi, K., Kobayashi, T., and Kamata, K. (2004). Alterations in vascular endothelial function in the aorta and mesenteric artery in type II diabetic rats. Can. J. Physiol. Pharmacol. 82, 175–182. doi: 10.1139/y04-002
McNeish, A. J., Sandow, S. L., Neylon, C. B., Chen, M. X., Dora, K. A., and Garland, C. J. (2006). Evidence for involvement of both IKCa and SKCa channels in hyperpolarizing responses of the rat middle cerebral artery. Stroke 37, 1277–1282. doi: 10.1161/01.STR.0000217307.71231.43
Miller, V. M., and Vanhoutte, P. M. (1991). Progesterone and modulation of endothelium-dependent responses in canine coronary arteries. Am. J. Physiol. 261, R1022–R1027. doi: 10.1152/ajpregu.1991.261.4.R1022
Nemoto, S., Kobayashi, T., Taguchi, K., Matsumoto, T., and Kamata, K. (2011). Losartan improves aortic endothelium-dependent relaxation via proline-rich tyrosine kinase 2/Src/Akt pathway in type 2 diabetic Goto-Kakizaki rats. Am. J. Physiol. Heart Circ. Physiol. 301, H2383–H2394. doi: 10.1152/ajpheart.00178.2011
Nettleship, J., Jones, R., Channer, K., and Jones, T. (2009). Testosterone and coronary artery disease. Front. Horm. Res. 37, 91–107. doi: 10.1159/000176047
Ohta, T., Katsuda, Y., Miyajima, K., Sasase, T., Kimura, S., Tong, B., et al. (2014). Gender differences in metabolic disorders and related diseases in spontaneously diabetic torii-leprfa rats. J. Diabetes Res. 2014:e841957. doi: 10.1155/2014/841957
Ormazabal, V., Nair, S., Elfeky, O., Aguayo, C., Salomon, C., and Zuñiga, F. A. (2018). Association between insulin resistance and the development of cardiovascular disease. Cardiovasc. Diabetol. 17:122. doi: 10.1186/s12933-018-0762-4
Parkington, H. C., Chow, J. A. M., Evans, R. G., Coleman, H. A., and Tare, M. (2002). Role for endothelium-derived hyperpolarizing factor in vascular tone in rat mesenteric and hindlimb circulations in vivo. J. Physiol. 542, 929–937. doi: 10.1113/jphysiol.2002.021030
Pieper, G. M., and Siebeneich, W. (1997). Use of a nitronyl nitroxide to discriminate the contribution of nitric oxide radical in endothelium-dependent relaxation of control and diabetic blood vessels. J. Pharmacol. Exp. Ther. 283, 138–147.
Rahimian, R., Laher, I., Dube, G., and van Breemen, C. (1997). Estrogen and selective estrogen receptor modulator LY117018 enhance release of nitric oxide in rat aorta. J. Pharmacol. Exp. Ther. 283, 116–122.
Rubanyi, G. M., Romero, J. C., and Vanhoutte, P. M. (1986). Flow-induced release of endothelium-derived relaxing factor. Am. J. Physiol. 250, H1145–H1149. doi: 10.1152/ajpheart.1986.250.6.H1145
Sakamoto, S., Minami, K., Niwa, Y., Ohnaka, M., Nakaya, Y., Mizuno, A., et al. (1998). Effect of exercise training and food restriction on endothelium-dependent relaxation in the Otsuka Long-Evans Tokushima Fatty rat, a model of spontaneous NIDDM. Diabetes Metab. Res. Rev. 47, 82–86. doi: 10.2337/diab.47.1.82
Sandow, S. L., Gzik, D. J., and Lee, R. M. K. W. (2009). Arterial internal elastic lamina holes: relationship to function? J. Anat. 214, 258–266. doi: 10.1111/j.1469-7580.2008.01020.x
Sandow, S. L., Tare, M., Coleman, H. A., Hill, C. E., and Parkington, H. C. (2002). Involvement of myoendothelial gap junctions in the actions of endothelium-derived hyperpolarizing factor. Circ. Res. 90, 1108–1113. doi: 10.1161/01.RES.0000019756.88731.83
Sangüesa, G., Shaligram, S., Akther, F., Roglans, N., Laguna, J. C., Rahimian, R., et al. (2017). Type of supplemented simple sugar, not merely calorie intake, determines adverse effects on metabolism and aortic function in female rats. Am. J. Physiol. Heart Circ. Physiol. 312, H289–H304. doi: 10.1152/ajpheart.00339.2016
Schach, C., Resch, M., Schmid, P. M., Riegger, G. A., and Endemann, D. H. (2014). Type 2 diabetes: increased expression and contribution of IKCa channels to vasodilation in small mesenteric arteries of ZDF rats. Am. J. Physiol. Heart Circ. Physiol. 307, H1093–H1102. doi: 10.1152/ajpheart.00240.2013
Schneider, M. P., Hilgers, K. F., Klingbeil, A. U., John, S., Veelken, R., and Schmieder, R. E. (2000). Plasma endothelin is increased in early essential hypertension. Am. J. Hypertens. 13, 579–585. doi: 10.1016/S0895-7061(99)00260-5
Scotland, R. S., Morales-Ruiz, M., Chen, Y., Yu, J., Rudic, R. D., Fulton, D., et al. (2002). Functional reconstitution of endothelial nitric oxide synthase reveals the importance of serine 1179 in endothelium-dependent vasomotion. Circ. Res. 90, 904–910. doi: 10.1161/01.RES.0000016506.04193.96
Shaligram, S., Akther, F., Razan, M. R., Graham, J. L., Roglans, N., Alegret, M., et al. (2020). Mesenteric arterial dysfunction in the UC Davis Type 2 Diabetes Mellitus rat model is dependent on pre-diabetic versus diabetic status and is sexually dimorphic. Eur. J. Pharmacol. 879:173089. doi: 10.1016/j.ejphar.2020.173089
Shi, Y., So, K.-F., Man, R. Y. K., and Vanhoutte, P. M. (2007). Oxygen-derived free radicals mediate endothelium-dependent contractions in femoral arteries of rats with streptozotocin-induced diabetes. Br. J. Pharmacol. 152, 1033–1041. doi: 10.1038/sj.bjp.0707439
Shimokawa, H., Yasutake, H., Fujii, K., Owada, M. K., Nakaike, R., Fukumoto, Y., et al. (1996). The importance of the hyperpolarizing mechanism increases as the vessel size decreases in endothelium-dependent relaxations in rat mesenteric circulation. J. Cardiovasc. Pharmacol. 28, 703–711. doi: 10.1097/00005344-199611000-00014
Steinberg, H. O., Paradisi, G., Cronin, J., Crowde, K., Hempfling, A., Hook, G., et al. (2000). Type II diabetes abrogates sex differences in endothelial function in premenopausal women. Circulation 101, 2040–2046. doi: 10.1161/01.cir.101.17.2040
Summers, B. A., Overholt, J. L., and Prabhakar, N. R. (1999). Nitric oxide inhibits L-type Ca2+ current in glomus cells of the rabbit carotid body via a cGMP-independent mechanism. J. Neurophysiol. 81, 1449–1457. doi: 10.1152/jn.1999.81.4.1449
Tabish, S. A. (2007). Is diabetes becoming the biggest epidemic of the twenty-first century? Int. J. Health Sci. 1, 5–8.
Taylor, H. J., Chaytor, A. T., Edwards, D. H., and Griffith, T. M. (2001). Gap junction-dependent increases in smooth muscle cAMP underpin the EDHF phenomenon in rabbit arteries. Biochem. Biophys. Res. Commun. 283, 583–589. doi: 10.1006/bbrc.2001.4791
Thompson, J., and Khalil, R. A. (2003). Gender differences in the regulation of vascular tone. Clin. Exp. Pharmacol. Physiol. 30, 1–15. doi: 10.1046/j.1440-1681.2003.03790.x
Touyz, R. M., Chen, X., Tabet, F., Yao, G., He, G., Quinn, M. T., et al. (2002). Expression of a functionally active gp91phox-containing neutrophil-type NAD(P)H oxidase in smooth muscle cells from human resistance arteries: regulation by angiotensin II. Circ. Res. 90, 1205–1213. doi: 10.1161/01.res.0000020404.01971.2f
US National Institutes of Health (2011). Guide for the Care and Use of Laboratory Animals, 8th Edn. Washington, DC: National Academies Press. Available online at: https://www.ncbi.nlm.nih.gov/books/NBK54050/
Vanhoutte, P. M., Shimokawa, H., Tang, E. H. C., and Feletou, M. (2009). Endothelial dysfunction and vascular disease. Acta Physiol. 196, 193–222. doi: 10.1111/j.1748-1716.2009.01964.x
Vázquez-Medina, J. P., Dodia, C., Weng, L., Mesaros, C., Blair, I. A., Feinstein, S. I., et al. (2016). The phospholipase A2 activity of peroxiredoxin 6 modulates NADPH oxidase 2 activation via lysophosphatidic acid receptor signaling in the pulmonary endothelium and alveolar macrophages. FASEB J. 30, 2885–2898. doi: 10.1096/fj.201500146R
White, R. E., and Carrier, G. O. (1990). Vascular contraction induced by activation of membrane calcium ion channels is enhanced in streptozotocin-diabetes. J. Pharmacol. Exp. Ther. 253, 1057–1062.
Woodman, O. L., and Boujaoude, M. (2004). Chronic treatment of male rats with daidzein and 17 beta-oestradiol induces the contribution of EDHF to endothelium-dependent relaxation. Br. J. Pharmacol. 141, 322–328. doi: 10.1038/sj.bjp.0705603
Youn, J. Y., Gao, L., and Cai, H. (2012). The p47phox- and NADPH oxidase organiser 1 (NOXO1)-dependent activation of NADPH oxidase 1 (NOX1) mediates endothelial nitric oxide synthase (eNOS) uncoupling and endothelial dysfunction in a streptozotocin-induced murine model of diabetes. Diabetologia 55, 2069–2079. doi: 10.1007/s00125-012-2557-6
Yue, P., Chatterjee, K., Beale, C., Poole-Wilson, P. A., and Collins, P. (1995). Testosterone relaxes rabbit coronary arteries and aorta. Circulation 91, 1154–1160. doi: 10.1161/01.cir.91.4.1154
Zhang, R., Thor, D., Han, X., Anderson, L., and Rahimian, R. (2012). Sex differences in mesenteric endothelial function of streptozotocin-induced diabetic rats: a shift in the relative importance of EDRFs. ’303, H1183–H1198. doi: 10.1152/ajpheart.00327.2012
Zheng, Y., Ley, S. H., and Hu, F. B. (2018). Global aetiology and epidemiology of type 2 diabetes mellitus and its complications. Nat. Rev. Endocrinol. 14, 88–98. doi: 10.1038/nrendo.2017.151
Keywords: sex differences, aorta, type-2 diabetes, nitric oxide, insulin resistance
Citation: Akther F, Razan MR, Shaligram S, Graham JL, Stanhope KL, Allen KN, Vázquez-Medina JP, Havel PJ and Rahimian R (2021) Potentiation of Acetylcholine-Induced Relaxation of Aorta in Male UC Davis Type 2 Diabetes Mellitus (UCD-T2DM) Rats: Sex-Specific Responses. Front. Physiol. 12:616317. doi: 10.3389/fphys.2021.616317
Received: 12 October 2020; Accepted: 20 May 2021;
Published: 22 July 2021.
Edited by:
Nazareno Paolocci, Johns Hopkins University, United StatesReviewed by:
Gabriel Tavares Vale, Minas Gerais State University, BrazilCopyright © 2021 Akther, Razan, Shaligram, Graham, Stanhope, Allen, Vázquez-Medina, Havel and Rahimian. This is an open-access article distributed under the terms of the Creative Commons Attribution License (CC BY). The use, distribution or reproduction in other forums is permitted, provided the original author(s) and the copyright owner(s) are credited and that the original publication in this journal is cited, in accordance with accepted academic practice. No use, distribution or reproduction is permitted which does not comply with these terms.
*Correspondence: Roshanak Rahimian, cnJhaGltaWFuQHBhY2lmaWMuZWR1
Disclaimer: All claims expressed in this article are solely those of the authors and do not necessarily represent those of their affiliated organizations, or those of the publisher, the editors and the reviewers. Any product that may be evaluated in this article or claim that may be made by its manufacturer is not guaranteed or endorsed by the publisher.
Research integrity at Frontiers
Learn more about the work of our research integrity team to safeguard the quality of each article we publish.