- Department of Cardiology, The Second Xiangya Hospital of Central South University, Changsha, China
Vascular aging plays a pivotal role in the morbidity and mortality of elderly people. Decrease in autophagy leads to acceleration of vascular aging, while increase in autophagy leads to deceleration of vascular aging. And emerging evidence indicates that acetylation plays an important role in autophagy regulation; therefore, recent research has focused on an in-depth analysis of the mechanisms underlying this regulation. In this review, current knowledge on the role of acetylation of autophagy-related proteins and the mechanisms by which acetylation including non-autophagy-related acetylation and autophagy related acetylation regulate vascular aging have been discussed. We conclude that the occurrence of acetylation modification during autophagy is a fundamental mechanism underlying autophagy regulation and provides promising targets to retard vascular aging.
Introduction
Aging is a process of functional decline of life and is associated with an increase in age-related diseases (Lopez-Otin et al., 2013). With increase in the life expectancy of humans, the incidence of age-related pathologies is also increasing and exceeds the sustainability of economy and society. As a matter of result, it is urgent to find effective interventions to prevent the deterioration of age-associated conditions. Therefore, research is being performed on identifying potential mechanisms underlying aging and effective ways to retard aging.
Post-translational modifications (PTMs) play a pivotal role in determining the structure, destination, activity, and function of proteins. PTMs includes phosphorylation, glycosylation, ubiquitination, lipidation, methylation, and acetylation (Wani et al., 2015). Various factors, for example, nutrient availability, and proper organellar function determine the kind of PTMs that a protein undergoes, and different PTMs are tightly regulated (Wani et al., 2015; Xie et al., 2015). An increasing number of recent studies have indicated that acetylation, a kind of PTMs, is an important regulator of autophagy. Autophagy is a predominantly cytoprotective process, and the autophagic rate decreases with increase in age (Rubinsztein et al., 2011). Emerging evidence shows that autophagy plays a prominent role in life span determination and age-related conditions, including those involving the respiratory system (Kuwano et al., 2016) or the immune system (Zhang et al., 2016) and conditions such as cardiovascular diseases (Stern et al., 2003; Shirakabe et al., 2016; Humphrey and Milewicz, 2017; Ren and Zhang, 2018), neurodegeneration (Fivenson et al., 2017; Plaza-Zabala et al., 2017), and bone aging (Ma et al., 2018). Autophagy is a multistep process involving autophagosome formation and content degradation by lysosomes. Researchers are focusing on whether regulating the acetylation of autophagy-related proteins can enhance autophagy, thereby partly reverting aging and age-related conditions including vascular aging.
Bánréti et al. (2013) has summarize how previously identified histone acetylases (HATs) and deacetylases (HDACs) modify key autophagic effector proteins, and discussed how they play a role in neurodegenerative diseases and cancer. In the current article, we have added the research of acetylation modification during the multistep process of autophagy in the recent years, discussed the existed researches about acetylation in vascular aging, and suggested regulating the acetylation of autophagy may be a potential way to retard vascular aging.
Autophagy and Vascular Aging: An Overview
Autophagy is a cytoprotective process and includes three different types, which are macro-autophagy, micro-autophagy, and chaperone-mediated autophagy (CMA). Macro-autophagy is the principal and most commonly studied type of autophagy, and is commonly referred as autophagy (Ktistakis and Tooze, 2016). Classical autophagy involves five steps: initiation, nucleation, vesicle elongation, autophagosome maturation and lysosome infusion, degradation, the detailed processes have been shown in these literatures (Khan et al., 2016; Leidal et al., 2018; Dossou and Basu, 2019). In the recent years, the effects of autophagy in vascular aging have been extensively studied. A growing number of studies have provided evidence that autophagy is a fundamental process to ensure vascular health during aging, and compromised autophagic functions may be important in the development of aging (Jiang, 2016; Abdellatif et al., 2018). Thus, regulating autophagy in vessels is a promising way to prevent vascular aging and age-related vascular diseases.
Acetylation Modification During the Different Processes of Autophagy
Acetyl-CoA (Ac-CoA), acetyltransferases, deacetyl transferases, and targeted protein sites are the three main elements of acetylation modification. Ac-CoA is the only source of acetyl (Pietrocola et al., 2015), and acetyltransferases and deacetyl transferases transfer or remove the acetyl to or from the targeted proteins. HATs and HDACs comprise acetyl–deacetyl transferase pairs (Peserico and Simone, 2011). Acetylation was first detected nearly 50 years ago, histones containing lysine were the first and extensively studied target proteins of acetylation modification, while some non-histones including transcription factors and cytoplasmic proteins were also targeted proteins (N-termini of target proteins),which regulate energy metabolism, endocytosis, and cytoskeleton (Deribe et al., 2010). Bánréti et al. (2013) reviewed different classes of HATs and HDACs. HATs fall into three major classes: lysine acetyltransferase (KAT)2/general control non-derepressible 5 (GCN5)-related N-acetyltransferases (GNAT family), E1A-binding protein p300 (EP300; CREBBP family), and the MYST family. Deacetylases are also divided into several classes: class I, IIa, IIb, and zinc-dependent class IV enzymes; class III family, which uses NAD+ to complete deacetylation reactions. Class I consists of HDAC1-3 and HDAC8; class IIa includes HDAC4, HDAC5, HDAC7, and HDAC9; class IIb includes HDAC6 and HDAC10; class IV includes HDAC11; and the class III family comprises sirtuins (SIRT), including SIRT1–7 (Sadoul et al., 2011; Narita et al., 2019).
Acetylation and Autophagy Initiation
In mammalian cells, autophagy is induced by unc-51-like kinase 1 (ULK1) [Autophagy-related gene 1 (ATG1), yeast homolog)], which interacts with ATG13, FAK family kinase-interacting protein of 200 kDa [FIP200 (ATG17, yeast homolog)], and ATG101 (no known yeast homolog) (Hosokawa et al., 2009; Zachari and Ganley, 2017; Mercer et al., 2018). ULK1 is mainly regulated by mammalian target of rapamycin (mTOR) (Dossou and Basu, 2019). Under growth factor deprivation, human immunodeficiency virus (HIV) Tat-interactive protein 60 kDa (TIP60), an acetyltransferase can regulate autophagy by activating ULK1 (Lin et al., 2012a,b). Under endoplasmic reticulum (ER) stress, autophagy can also be modulated through the same way (Nie et al., 2016). Under both growth factor deprivation and ER stress, the glycogen synthase kinase-3β (GSK3β)/TIP60/ULK1 pathway is engaged to increase autophagy. In these studies, it was found that GSK3β first phosphorylated TIP60-Ser86 and activated TIP60. Then, the activated TIP60 directly acetylated ULK1 and activated the protein kinase. The acetylation sites on ULK1 are located at K162 and K606. Researchers found that in ULK1–/– mouse embryonic fibroblasts, the acetylation-defective mutant ULK1 showed decreased kinase activity and failed to rescue autophagy, which further proved that acetylation is vital to ULK1 activation (Lin et al., 2012b). These studies revealed that induction of autophagy on ULK1 activation integrates phosphorylation and acetylation modification.
Acetylation During Nucleation in Autophagy
On specific membranes, phosphoinositide-3-kinase class 3 (PIK3C3) or vacuolar protein sorting 34 (VPS34) combines with its regulatory proteins to form the PIK3C3/VPS34-Beclin1 (BECN1)-PIK3R4/VPS15/p150 core-based complex. This process is essential for PIK3C3/VPS34 to exert its lipid kinase activity in cells, thus promoting the nucleation of autophagy. Through interacting with PIK3C3/VPS34 or recruiting it to the specific membranes, these regulatory proteins affect nucleation of autophagy. Both in vitro and in vivo trials, evidence shows that EP300-dependent acetylation turns PIK3C3/VPS34 off, while deacetylation turns PIK3C3/VPS34 on, wherein deacetylation site K771 is required for its full activation. The PIK3C3/VPS34 activation mechanism referred above happens not only in starvation-induced autophagy but also in the process of autophagy that does not involve Adenosine 5′-monophosphate-activated protein kinase (AMPK), mTORC1, or ULK1 (Su et al., 2017; Kary, 2018; Su and Liu, 2018).
A study found that the phosphorylation of BECN1 at S409 is required for the subsequent BECN1 acetylation, which is mediated by p300 at lysine 430, and SIRT1 can reverse acetylation of BECN1 at lysine 437 (Sun et al., 2015). BECN1 is also regulated via several different types of ubiquitination (Boutouja et al., 2017). Acetylated inducible heat shock protein (HSP)70 increases under autophagy-inducing stress, and HSP70 binds to the BECN1-VPS34 complex. The mechanism is that acetylated HSP70 recruits KRAB-ZFP-associated protein 1 (KAP1), an E3 ligase, for SUMOylation, thereby inducing Lys840 SUMOylation and increasing the activity of VPS34 bound to BECN1 (Yang et al., 2013). TRIM (Tripartite motif), an E3 ligase protein family, enhances the binding of BECN1 with ULK1 and promotes autophagy activities via BECN1 ubiquitination; The Lys-372 residue of TRIM50, critical for its acetylation, is also necessary for its E3 ligase activity controlling BECN1 ubiquitination; this reveals acetylation–ubiquitination-dependent control of autophagy modulation (Fusco et al., 2018). Similar to the phosphorylation–acetylation cascade in ULK1 acetylation, the acetylation–ubiquitination cascade is vital for the regulation of autophagy by BECN1. In summary, BECN1 acetylation inhibits autophagosome maturation, leading to impairment of autophagic flux (Esteves et al., 2019). Studies targeting BECN1 found that supplementation of omega-3 polyunsaturated fatty acid induces the autophagy pathway through upregulation of SIRT1-mediated deacetylation of BECN1, thus attenuates neuronal apoptosis in traumatic brain injury (Chen et al., 2018). In colorectal cancer cells, aspirin induces autophagosome formation, while aspirin-mediated BECN1 acetylation blocks autophagic degradation (Sun et al., 2017). The result indicates that if deacetylation in autophagy is enhanced, aspirin-induced autophagy will also be enhanced.
Acetylation Modification During Autophagy Vesicle Elongation
In yeast, researchers found that ATG3 is deacetylated by HDAC Rpd3, which controls its interaction with ATG8 and regulate autophagy by affecting the dynamics, flux, and duration of autophagy shortly after induction of starvation; KAT5/TIP60 (mammalian homolog of Esa1) can also regulate autophagy by ATG3 acetylation (Yi and Yu, 2012). Under the condition of nutrient deprivation, nuclear microtubule-associated proteins light chain 3 (LC3); LC3 deacetylation by SIRT1 deepens its communication with the nuclear factor TP53INP2/DOR; As a result, It causes the distribution of nuclear LC3 to the cytoplasm, LC3 then interacts with ATG7, leading to LC3 lipidation and autophagosome biogenesis (Liu and Klionsky, 2015). This process referred above is highly regulated by the nuclear deacetylase SIRT1 (deacetylation at K49 and K51), which helps LC3 translocated from nuclear to cytoplasm (Huang et al., 2015). In HepG2 cells, enhanced binding of SIRT1-LC3 reduces the endogenous LC3 acetylation and SIRT1 inactivation inhibits autophagy (Li X. et al., 2016). Increased SIRT1 expression decreases ATG5 acetylation on lysine residues and increases autophagy (Jiang et al., 2016). While preventing p300 autoinhibition and promoting LC3 acetylation impedes LC3 lipidation (Wan et al., 2017). HLA-B–associated transcript 3 (BAT3) increases p53 acetylation and the expression of its target gene; while it limits p300-dependent acetylation of ATG7; Thus, we conclude that BAT3 highly regulate autophagy by modulating the localization of intracellular p300, thereby affecting the p300 targeted on its substrates, p53 and ATG7 (Sebti et al., 2014). In summary, SIRT1 and p300 comprise a key pair of regulators in acetylation modification during autophagy vesicle elongation. During this stage, protein deacetylation activates autophagy, whereas acetylation inhibits autophagy.
Acetylation Modification During Autophagosome Maturation
Acetylation occurring upstream of autophagy also affects the later stages of autophagy. BECN1 acetylation negatively regulates autophagosome maturation by inhibiting Rubicon recruitment (Matsunaga et al., 2009; Zhong et al., 2009). Although lysosomal biogenesis seems to be triggered as a compensatory response when BECN1 acetylation impairs autophagic flux, autophagosome fusion with lysosomes is compromised, contributing to Alzheimer’s disease (AD) neurodegeneration (Esteves et al., 2019). The GSK3-TIP60 pathway (Lin et al., 2012a,b; Nie et al., 2016), involved in an early stage of autophagy, also regulates autophagosome maturation mediated by autophagy regulator–RUBCNL/pacer acetylation (Cheng and Sun, 2019; Cheng et al., 2019), which is the opposite of the regulating results for RUBCN/Rubicon. RUBCNL and RUBCN compose a dual molecular switch model controlling autophagosome maturation (Cheng and Sun, 2019). Furthermore, LC3B-II deacetylation, which is partly mediated by HDAC6, increases the degradation of p62/SQSTM1 and is involved in autophagic degradation during serum starvation (Liu et al., 2013).
Researches have proven that HDAC6 deacetylase domain is also required for aggresomal formation, autophagosome–lysosome fusion, and autophagic turnover (Kawaguchi et al., 2003; Iwata et al., 2005; Pandey et al., 2007). And researchers also found that SIRT1 may compensate for the function of HDAC6. Cortactin deacetylation mediated by cytosolic HDAC6 is required for autophagosome–lysosome fusion (Lee et al., 2010). SIRT1 is predominantly located in the cytosol (Li et al., 2008), where SIRT1 interacts with cortactin and deacetylates it. Both HDAC6 and SIRT1 have been found that they can bind and deacetylate cortactin independently. However, depending on specific cell types or tissues, HDAC6 and SIRT1 can also work cooperatively or competitively (Zhang et al., 2009). For example, in HeLa cells, either HDAC6 or SIRT1 can bind and deacetylate cortactin independently, and they can also work cooperatively toward cortactin (Zhang et al., 2007). Either SIRT1 or HDAC6 binds to the same domain of cortactin, thus, researchers speculate that SIRT1 and HDAC6 may compete for the domain to bind cortactin in OV2008 cells (Zhang et al., 2009). Moreover, ATP13A2 can recruit HDAC6 to lysosomes to deacetylate cortactin, thereby promoting autophagosome–lysosome fusion and autophagy (Wang et al., 2019).
There is evidence that reversible acetylation of α-tubulin can regulate microtubule stability and function, which is also essential for the fusion of autophagosomes to lysosomes (Kochl et al., 2006; Xie et al., 2010). In acidic pH–dependent autophagy in cardiomyocytes, decreasing α-tubulin acetylation impairs autophagy maturation and causes cardiomyocyte injury while increasing α-tubulin acetylation can revert the process (Yang et al., 2019). Reactive oxygen species (ROS)–mediated hyperacetylation of microtubule was reported to stimulate autophagy under stress and nutrient starvation (Geeraert et al., 2010; Mackeh et al., 2014). Hyperacetylation of microtubule is mediated by acetyltransferase MEC17 at the residue of lys40 (Mackeh et al., 2014). Spermine increases microtubule acetylation and facilitates selective autophagic degradation of prion aggregates by binding to the microtubule protein Tubb6A (Phadwal et al., 2018). A recent study reported that zinc oxide nanoparticles (a nanomaterial) inhibited autophagy by blocking autophagosome–lysosome fusion, while microtubule acetylation helped promote the autophagic degradation process (Liu J. et al., 2019). Chen et al. (2015) found that potassium bisperoxo (1,10-phenanthroline)oxovanadate [bpV(phen)] suppresses acetylated microtubule–dependent degradation of autophagosomes by disrupting the HDAC6 interacted with p62.
Other researchers have obtained contrasting results. They found that HDAC6-mediated α-tubulin deacetylation was also important for autophagosome maturation. Heterogeneous nuclear ribonucleoprotein K (hnRNPK) deficiency was found to decrease α-tubulin K40 acetylation by HDAC6, consequently enhancing autophagosome–lysosome fusion in the 293-cell line (human renal epithelial cell line) (Li et al., 2018). The ROS pathway has also been reported to reduce the fusion of autophagosome and lysosome by increasing tubulin acetylation (Bonet-Ponce et al., 2016). These findings show that tubulin acetylation does not always enhance autophagy and hyperacetylation of tubulin might impair autophagy flux. The factors leading to the differences in findings between these studies are still not clear. Autophagosomes are usually delivered along the microtubule tracks by dynein to the centrosomes where lysosomes are usually gathered (Geeraert et al., 2010). Since the acetylation during this stage is complex, we speculate that no matter tubulin is acetylated or deacetylated, decreased autophagosome–lysosome formation is due to the disturbed stability of the microtubule system. Thus, balancing the acetylation/deacetylation of tubulin is a key aspect to be considered for autophagy regulation during this phase. Based on these findings, we can conclude that acetylation participates in the entire process of autophagy, from the initial step to the completion of autophagosome degradation.
During the early stages, p300/SIRT1 seems to be the major regulator pair, while HDAC6 seems to be the major regulator in the later stages. Therefore, if an p300/SIRT1/HDAC6 inhibitor or activator is used to regulate autophagy, the acetylation condition of the entire process of autophagy will be affected. Because autophagy is a constant process, blocking or activation of any key protein in the process will influence the effective autophagy flux, further indicating that acetylation modification is an important mechanism for regulating autophagy (Figure 1).
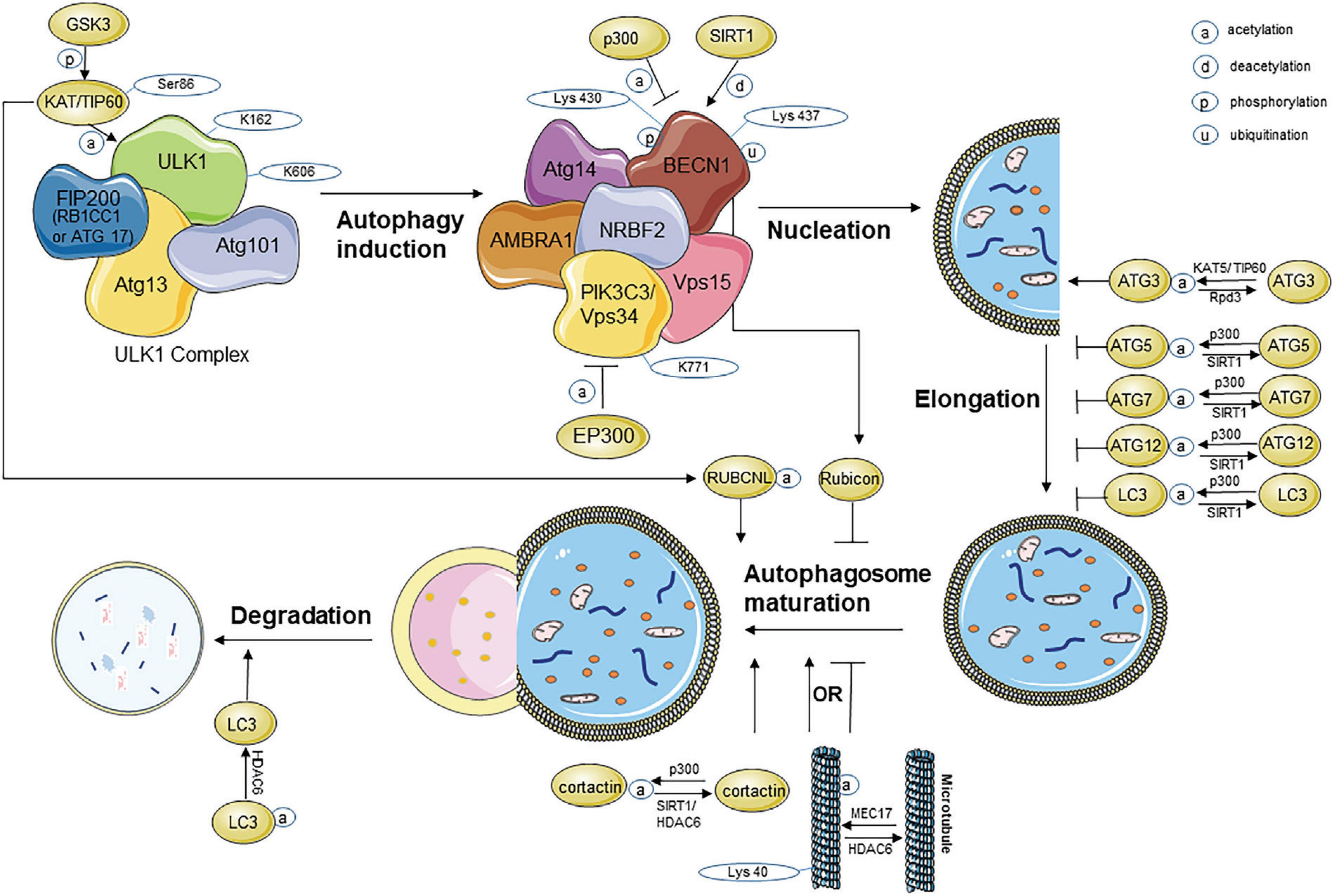
Figure 1. Acetylation modification during the process of autophagy. ATG, autophagy-related protein; BECN1, beclin1; EP300, E1A binding protein p300; FIP200, FAK family kinase-interacting protein of 200 kDa; GSK3β, glycogen synthase kinase-3β; HDAC, histone deacetylase; KAT, lysine acetyltransferase; LC3, microtubule-associated protein light chain 3; PIK3C3, phosphoinositide-3-kinase class 3; RB1CC1, RB1-inducible coiled-coil protein 1; TIP60, Tat-interactive protein 60 kDa; ULK1, unc-51-like kinase 1; VPS, vacuolar protein sorting.
Acetylated FOXO1 and Autophagy Flux
The autophagy process is affected not only by direct acetylation but also by the acetylation of some transcription factors. Fullgrabe et al. (2014) reported several transcription factors related to the transcriptional control of autophagy. Of these, acetylation of transcription factors is best illustrated in the case of forkhead box O (FOXO) family members. FOXO1 is frequently reported to be closely associated with autophagy. Activated FOXO1 enhances autophagy by increasing the expression of numerous autophagy-related genes in various cells (Zhao et al., 2007, 2008; Sengupta et al., 2009; Xu et al., 2011; Warr et al., 2013; Chi et al., 2016; Wang et al., 2018; Liu Y. et al., 2019). Activation of the FOXO family is regulated by complex mechanisms in various cells and tissues, while acetylation is one of them.
SIRT1 directly influences autophagy by deacetylating key components of autophagy-related proteins, including the products of ATG5, ATG7, and ATG8 (Lan et al., 2008). SIRT1 localizes in the nucleus and activates transcription factor from FOXO family, which is also a classic way to induce the expression of autophagy-related proteins (Xiong et al., 2013). Under glucose deprivation conditions, SIRT1 increases FOXO1 deacetylation, thereby increasing the expression of Rab7, which is a small GTP-binding protein that mediates autophagosome–lysosome fusion in the later stages (Hariharan et al., 2010). This is also the mechanism by which resveratrol reverses myocardial oxidative stress injury in diabetic mice (Wang et al., 2014), and by which curcumin protects HUVEC survival from oxidative stress damage (Han J. et al., 2012). The SIRT1/FOXO1/Rab7 axis is also important for maintaining left ventricular function during starvation (Hariharan et al., 2010) and preventing podocyte injury (Majumder and Advani, 2015; Wang et al., 2016) in the same manner. Researchers have found that autophagy induced by cytosolic FOXO1 is capable of suppressing tumor (Zhao et al., 2010) and that, in pancreatic cancer, miR-138-5p inhibits autophagy by blocking the SIRT1/FoxO1/Rab7 axis (Tian et al., 2017). The SIRT1/FOXO1/Rab7 axis comprises a classical pathway that is targeted by researchers to modulate autophagy and to attenuate diseases. Acetylated FOXO1 was found to mediate high glucose induced autophagy in H9C2 cardiomyoblasts as well (Liu et al., 2014).
Another study reported that in QBC939 cells, FOXO1 acetylation and its subsequent interaction with ATG7 regulate basal and serum starvation–induced autophagy, as evidenced by LC3 accumulation and p62 degradation. These findings identify FOXO1 as a potential therapeutic target for treating human cholangiocarcinoma via regulating autophagy (He et al., 2018). In the case of myocardial infarction, total cardiac FOXO1 expression is downregulated at first and partly recovers after 7 days, which is accompanied by fundamental PTMs in FOXO1, particularly acetylation, suggesting that FOXO1 acetylation contributes to cardiac remodeling in post-ischemic heart failure (Kappel et al., 2016). Moreover, Akt2 ablation can protect against cardiac aging through restoring FOXO1-related autophagy (Ren et al., 2017). Liver-specific knockout of glucose-6-phosphatase-α (G6Pase-α; L-G6pc-/-) causes downregulation of SIRT1 signaling and deacetylation of the FOXO family, leading to inactivation of autophagy, which in turn transactivates autophagy genes (Cho et al., 2017). Li J. et al. (2016) found that SIRT3 activation is also essential for reducing the acetylation modification on FOXO1, which in turn alleviates myocardial hypertrophy with chronic angiotensin II (Ang II) infusion through improving autophagy flux.
Targets for Regulating the Acetylation Modification in Vascular Aging
Vascular aging is one of the main aspects of aging. Endothelial cells (ECs) and vascular smooth muscle cells (VSMCs) are the main structure of blood vessels. The properties of ECs and VSMCs change greatly during vascular aging, gradually leading to compromised vascular function and progressive vascular diseases. Mistriotis and Andreadis (2017) performed an in-depth analysis of the molecular mechanism underlying vascular aging concerning two categories, that is, extrinsic and intrinsic changes. The former category includes chronic inflammation, atherosclerosis, hypertension, vascular wall stiffness, and vascular cell communication. The latter includes telomere attrition, mitochondrial dysfunction, DNA damage, epigenetic changes, nuclear organization loss, and cellular senescence. The role of acetylation in vascular aging, which is the focus of the current review, was not specifically discussed in that study.
From the literature that we reviewed, the existing research on acetylation modification in vascular aging focused on SIRT1. Decreased SIRT1 activity leads to increased lysine acetylation of important targets including p53, endothelial nitric oxide synthase (eNOS), peroxisome proliferator–activated receptor gamma coactivator (PGC)-1α, and matrix metalloproteinase (MMP)-14 at gene or protein level (Cencioni et al., 2015).
Ameliorating EC Senescence Through Non-autophagy Acetylation Regulation
In H2O2 induced senescence models of human umbilical vein endothelial cells (HUVECs), 2,3,5,4′-tetrahydroxystilbene-2-O-β-D-glucoside increases blood flow and partly reverse vascular senescence by increasing SIRT1 activity and eNOS expression, thereby decreasing p53 acetylation at the K373 site (Han X. et al., 2012); Ginsenoside Rb1 protects against endothelial senescence and dysfunction through stimulating the expression of SIRT1, which decreases eNOS acetylation and promotes NO production; Furthermore, when SIRT1 is knocked down, the effect of Rb1 on endothelial senescence decreases (Song et al., 2014). On the contrary, elevated trimethylamine-N-oxide (TMAO) levels may induce the EC senescence through decreased SIRT1, which promotes acetylation of eNOS and decreases the level of NO (Ke et al., 2018).
A study using aorta segments isolated from young Wistar rats assessed three different kinds of inhibitors of SIRT1: nicotinamide, sirtinol, and EX527 (Zarzuelo et al., 2013). Inhibitors causes endothelial dysfunction and increases NADPH oxidase–derived ROS in the vascular wall by impairing activities of SIRT1, leading to vascular aging. Moreover, SIRT1 activation decreases PGC-1α acetylation and subsequent PPARα activation, both NADPH oxidase–driven ROS production and NO inactivation decreases, and endothelial function is improved as a result (Zarzuelo et al., 2013). Supplementation with nicotinamide mononucleotide (NMN) in old mice restores endothelium-dependent diastole (EDD) and NO-mediated EDD, reduces brachial-ankle pulse wave velocity (aPWV), normalizes O2 production, decreases nitro tyrosine levels, reverses collagen-I deposition, increases elastin levels, and restores vascular SIRT1 activity. And (-)-epicatechin partially restores NO to the levels as in young cells by stimulating SIRT1 binding to the eNOS and decreasing synthase acetylation levels (Ramirez-Sanchez et al., 2018). These findings further prove that SIRT1-mediated deacetylation plays a protective role in vascular aging (de Picciotto et al., 2016).
SIRT1 and liver kinase B1 (LKB1)/AMPK are the two key molecules for regulating the function of EC. In high glucose-induced senescence in mouse microvascular ECs, SIRT1 downregulation plays a crucial role. Reduction of SIRT1 increased FOXO-1 and P53 acetylation, P21 expression. Metformin can protect HG-induced endothelial dysfunction through regulating SIRT1 expression/activity directly or partly via LKB1/AMPK pathway (Arunachalam et al., 2014). What’s more, the protective activities of SIRT1 may also be achieved partly by regulating the acetylation/deacetylation status and stability of LKB1 protein (Zu et al., 2010). In another study, selective overexpression of SIRT1 in the endothelium prevents hypertension and age-related adverse arterial remodeling in mice without eNOS; knockdown of HERC2 abolishes the protective effects by increasing the interaction between LKB1 and transforming growth factor (TGF)-β1 (Bai et al., 2016). From all above, SIRT1 cannot only regulate EC senescence through eNOS/NO pathway but also via LKB1/HERC2 pathway.
Ameliorating VSMC Senescence Through Non-autophagy Acetylation Regulation
In VSMC cells, Ang II was usually used to induce senescence. Ang II stimulates PGC-1α phosphorylation, leading to the binding of GCN5 to PGC-1α and for its lysine acetylation; Acetylated PGC-1α by Ang II interrupts the PGC-1α-FOXO1-SIRT1 feed-forward signaling circuit, leading to SIRT1 and catalase downregulation and vascular senescence; Thus, endogenous PGC-1α improves vascular hypertrophy by upregulating catalase expression and reducing ROS levels (Xiong et al., 2013). And a new study found that PGC-1α can ameliorate senescence by inducing autophagy in an SQSTM1-dependent manner (Salazar et al., 2020). α7 nicotinic acetylcholine receptor (α7nAChR) alleviates VSMC senescence induced by Ang II by promoting the NAD (+)-SIRT1 pathway, thereby decreasing p53 acetylation (Li D.J. et al., 2016). Neuregulin-1 markedly inhibits H2O2-induced premature senescence of VSMCs by decreasing p53 acetylation both in vitro and in the aorta of mice with diabetes in vivo (Shakeri et al., 2018). Exendin-4 increases the acetylation of Nrf2 in a dose-dependent way and the recruitment of the transcriptional coactivator CREBBP to Nrf2; CREBBP silencing attenuates the suppressive effects of exendin-4 on Ang II-induced VSMC senescence and decreases super-oxidant production (Chen et al., 2016). The summarizations are detailed in the following table (Table 1).
Targets for Regulating the Acetylation of Autophagy in Vascular Aging
Regarding the acetylation of autophagy in vascular aging, Liu et al. (2018) reported that SIRT3 in macrophages form a molecular complex with ATG5 and acetylation of ATG5 inhibits autophagosome maturation, thus promoting NLRP3 inflammasome activation. The incubation of human aortic ECs with macrophage-conditioned medium induced endothelial dysfunction. These findings revealed that SIRT3-deficient macrophages displayed impaired autophagy and accelerated NLRP3 inflammasome activation and endothelial dysfunction. While another report demonstrated that HDAC4 inhibited vascular inflammation by regulating autophagy in vascular ECs (Yang et al., 2018). This result provides the first evidence that HDAC4 increased rapidly in response to Ang II, and HDAC4 deficiency suppresses activation of autophagy by inhibiting FOXO3a deacetylation, leading to reduced inflammation in Ang II-induced vascular ECs. These evidences indicate SIRT3 or HDAC4 may alleviate vascular aging via regulating acetylation of autophagy. Moreover, Shi et al. (2020) reported Ginsenoside Rb1 alleviated ox-LDL-induced vascular endothelium senescence by regulating SIRT1/BECN1/autophagy, and inhibiting autophagic degradation of KAT2A/GCN5 preventd directional migration of VSMCs (Ouyang et al., 2020). Taken together, regulating the acetylation of autophagy may be a potential way to prevent vascular aging.
Conclusion and Future Perspectives
Autophagy is a classical pathway involved in many physiological processes, including the elimination of cellular organelles, stimulation of different stress factors, and remodeling of tissues during development. Decreased autophagy creates diverse cellular dysfunctions that exacerbate the aging process, whereas enhanced autophagy generally promotes cellular homeostasis and function to prolong life span and improve health span. Acetylation acts as a novel regulator of autophagy and participates in the whole process of autophagy. Therefore, targeting the acetylation modification during autophagy may be a promising approach for preventing aging and aging-related conditions, such as vascular aging.
The existing research on acetylation modification in vascular aging mainly focuses on SIRT1-mediated non-autophagy–related acetylation of proteins, including acetylation of p53, eNOS, PGC-1α, and Nrf2. Several studies indicate that regulating acetylation of autophagy can also ameliorate vascular aging. Thus, our review aims to provide an integrated view of acetylation modification during the multistep process of autophagy and provide evidence for that regulating autophagy acetylation may be potential therapeutic targets for vascular aging. Finding a way to attenuate vascular aging by modulating autophagy acetylation can provide a bright future (e.g., by helping in increasing their life span, health span, or quality of life) for aging individuals, including prematurely aging people.
Author Contributions
SZ defined the study theme and methods. JS collected the references and wrote the article. ST, LT, HY, MC, YX, XL, and ZZ were responsible for revising the article and providing valuable suggestions. All authors contributed to the article and approved the submitted version.
Funding
This research was supported by the National Natural Science Foundation of China (81670269 to SZ) and (81801394 to ST).
Conflict of Interest
The authors declare that the research was conducted in the absence of any commercial or financial relationships that could be construed as a potential conflict of interest.
Abbreviations
Ac-CoA, acetyl-CoA; AD, Alzheimer’s disease; AMPK, Adenosine 5′-monophosphate-activated protein kinase; ATG, autophagy-related gene; Ang II, Angiotensin II; α 7nAChR, α 7 nicotinic acetylcholine receptor; aPWV, brachial-ankle pulse wave velocity; BAT3, HLA-B–associated transcript 3; BECN1, beclin1; CMA, chaperone-mediated autophagy; ECs, endothelium cells; eNOS, endothelial nitric oxide synthase; EDD, endothelium-dependent diastole; EP300, E1A binding protein p300; ER, endoplasmic reticulum; FIP200, FAK family kinase-interacting protein of 200 kDa; FOXO, fork head box O; GCN5, general control non-derepressible 5; GSK3β, glycogen synthase kinase-3 β; HATs, histone acetylases; HDACs, histone deacetylases; hnRNPK, heterogeneous nuclear ribonucleoprotein K; HSP, heat shock protein; KAT, lysine acetyltransferase; KAP1, KRAB-ZFP-associated protein 1; LC3, microtubule-associated protein light chain 3; LKB1, liver kinase B1; mTOR, mammalian target of rapamycin; MMP, matrix metalloproteinase; NMN, nicotinamide mononucleotide; NO, nitric oxide; PIK3C3, phosphoinositide-3-kinase class 3; PPARα, peroxisome proliferator-activated receptor α; PGC-1α, peroxisome proliferator-activated receptor gamma coactivator-1 alpha; PTMs, post translational modifications; SIRT, sirtuins; TGF, transforming growth factor; TIP60, Tat-interactive protein 60 kDa; TRIM, tripartite motif; TMAO, trimethylamine-N-oxide; ULK1, unc-51-like kinase 1; VPS, vacuolar protein sorting; VSMCs, vascular smooth muscle cells
References
Abdellatif, M., Sedej, S., Carmona-Gutierrez, D., Madeo, F., and Kroemer, G. (2018). Autophagy in Cardiovascular Aging. Circulat. Res. 123, 803–824. doi: 10.1161/CIRCRESAHA.118.312208
Arunachalam, G., Samuel, S. M., Marei, I., Ding, H., and Triggle, C. R. (2014). Metformin modulates hyperglycaemia-induced endothelial senescence and apoptosis through SIRT1. Br. J. Pharmacol. 171, 523–535. doi: 10.1111/bph.12496
Bai, B., Man, A. W., Yang, K., Guo, Y., Xu, C., Tse, H. F., et al. (2016). Endothelial SIRT1 prevents adverse arterial remodeling by facilitating HERC2-mediated degradation of acetylated LKB1. Oncotarget 7, 39065–39081. doi: 10.18632/oncotarget.9687
Bánréti, A., Sass, M., and Graba, Y. (2013). The emerging role of acetylation in the regulation of autophagy. Autophagy 9, 819–829. doi: 10.4161/auto.23908
Bonet-Ponce, L., Saez-Atienzar, S., da Casa, C., Sancho-Pelluz, J., Barcia, J. M., Martinez-Gil, N., et al. (2016). Rotenone Induces the Formation of 4-Hydroxynonenal Aggresomes. Role of ROS-Mediated Tubulin Hyperacetylation and Autophagic Flux Disruption. Mole. Neurobiol. 53, 6194–6208. doi: 10.1007/s12035-015-9509-3
Boutouja, F., Brinkmeier, R., Mastalski, T., El Magraoui, F., and Platta, H. W. (2017). Regulation of the Tumor-Suppressor BECLIN 1 by Distinct Ubiquitination Cascades. Int. J. Mole. Sci. 18:ijms18122541. doi: 10.3390/ijms18122541
Cencioni, C., Spallotta, F., Mai, A., Martelli, F., Farsetti, A., Zeiher, A. M., et al. (2015). Sirtuin function in aging heart and vessels. J. Mole. Cell. Cardiol. 83, 55–61. doi: 10.1016/j.yjmcc.2014.12.023
Chen, M., Zhou, X., Yu, L., Liu, Q., Sheng, X., Wang, Z., et al. (2016). Low-Level Vagus Nerve Stimulation Attenuates Myocardial Ischemic Reperfusion Injury by Antioxidative Stress and Antiapoptosis Reactions in Canines. J. Cardiovasc. Electrophysiol. 27, 224–231. doi: 10.1111/jce.12850
Chen, Q., Yue, F., Li, W., Zou, J., Xu, T., Huang, C., et al. (2015). Potassium Bisperoxo(1,10-phenanthroline)oxovanadate (bpV(phen)) Induces Apoptosis and Pyroptosis and Disrupts the P62-HDAC6 Protein Interaction to Suppress the Acetylated Microtubule-dependent Degradation of Autophagosomes. J.Biol. Chem. 290, 26051–26058. doi: 10.1074/jbc.M115.653568
Chen, X., Pan, Z., Fang, Z., Lin, W., Wu, S., Yang, F., et al. (2018). Omega-3 polyunsaturated fatty acid attenuates traumatic brain injury-induced neuronal apoptosis by inducing autophagy through the upregulation of SIRT1-mediated deacetylation of Beclin-1. J.Neuroinflam. 15:310. doi: 10.1186/s12974-018-1345-8
Cheng, X., and Sun, Q. (2019). RUBCNL/Pacer and RUBCN/Rubicon in regulation of autolysosome formation and lipid metabolism. Autophagy 15, 1120–1121. doi: 10.1080/15548627.2019.1596500
Cheng, X., Ma, X., Zhu, Q., Song, D., Ding, X., Li, L., et al. (2019). Pacer Is a Mediator of mTORC1 and GSK3-TIP60 Signaling in Regulation of Autophagosome Maturation and Lipid Metabolism. Mole. Cell 73, 788.e–802.e. doi: 10.1016/j.molcel.2018.12.017
Chi, Y., Shi, C., Zhao, Y., and Guo, C. (2016). Forkhead box O (FOXO) 3 modulates hypoxia-induced autophagy through AMPK signalling pathway in cardiomyocytes. Biosci. Rep. 36:bsr20160091. doi: 10.1042/bsr20160091
Cho, J. H., Kim, G. Y., Pan, C. J., Anduaga, J., Choi, E. J., Mansfield, B. C., et al. (2017). Downregulation of SIRT1 signaling underlies hepatic autophagy impairment in glycogen storage disease type Ia. PLoS Genet. 13:e1006819. doi: 10.1371/journal.pgen.1006819
de Picciotto, N. E., Gano, L. B., Johnson, L. C., Martens, C. R., Sindler, A. L., Mills, K. F., et al. (2016). Nicotinamide mononucleotide supplementation reverses vascular dysfunction and oxidative stress with aging in mice. Aging Cell 15, 522–530. doi: 10.1111/acel.12461
Deribe, Y. L., Pawson, T., and Dikic, I. (2010). Post-translational modifications in signal integration. Nat. Struct. Mol. Biol. 17, 666–672. doi: 10.1038/nsmb.1842
Dossou, A. S., and Basu, A. (2019). The Emerging Roles of mTORC1 in Macromanaging Autophagy. Cancers 11:cancers11101422. doi: 10.3390/cancers11101422
Esteves, A. R., Filipe, F., Magalhaes, J. D., Silva, D. F., and Cardoso, S. M. (2019). The Role of Beclin-1 Acetylation on Autophagic Flux in Alzheimer’s Disease. Mole. Neurobiol. 56, 5654–5670. doi: 10.1007/s12035-019-1483-8
Fivenson, E. M., Lautrup, S., Sun, N., Scheibye-Knudsen, M., Stevnsner, T., Nilsen, H., et al. (2017). Mitophagy in neurodegeneration and aging. Neurochem. Int. 109, 202–209. doi: 10.1016/j.neuint.2017.02.007
Fullgrabe, J., Klionsky, D. J., and Joseph, B. (2014). The return of the nucleus: transcriptional and epigenetic control of autophagy. Nat. Rev. Mole. Cell Biol. 15, 65–74. doi: 10.1038/nrm3716
Fusco, C., Mandriani, B., Di Rienzo, M., Micale, L., Malerba, N., Cocciadiferro, D., et al. (2018). TRIM50 regulates Beclin 1 proautophagic activity. Biochim. Biophys. Acta Mole.Cell Res. 1865, 908–919. doi: 10.1016/j.bbamcr.2018.03.011
Geeraert, C., Ratier, A., Pfisterer, S. G., Perdiz, D., Cantaloube, I., Rouault, A., et al. (2010). Starvation-induced hyperacetylation of tubulin is required for the stimulation of autophagy by nutrient deprivation. J. Biol. Chem. 285, 24184–24194. doi: 10.1074/jbc.M109.091553
Han, J., Pan, X. Y., Xu, Y., Xiao, Y., An, Y., Tie, L., et al. (2012). Curcumin induces autophagy to protect vascular endothelial cell survival from oxidative stress damage. Autophagy 8, 812–825. doi: 10.4161/auto.19471
Han, X., Ling, S., Gan, W., Sun, L., Duan, J., and Xu, J. W. (2012). 2,3,5,4′-tetrahydroxystilbene-2-O-beta-d-glucoside ameliorates vascular senescence and improves blood flow involving a mechanism of p53 deacetylation. Atherosclerosis 225, 76–82. doi: 10.1016/j.atherosclerosis.2012.08.011
Hariharan, N., Maejima, Y., Nakae, J., Paik, J., Depinho, R. A., and Sadoshima, J. (2010). Deacetylation of FoxO by Sirt1 Plays an Essential Role in Mediating Starvation-Induced Autophagy in Cardiac Myocytes. Circulat. Res. 107, 1470–1482. doi: 10.1161/CIRCRESAHA.110.227371
He, W., Zhang, A., Qi, L., Na, C., Jiang, R., Fan, Z., et al. (2018). FOXO1, a Potential Therapeutic Target, Regulates Autophagic Flux, Oxidative Stress, Mitochondrial Dysfunction, and Apoptosis in Human Cholangiocarcinoma QBC939 Cells. Cell. Physiol. Biochem. 45, 1506–1514. doi: 10.1159/000487576
Hosokawa, N., Sasaki, T., Iemura, S., Natsume, T., Hara, T., and Mizushima, N. (2009). Atg101, a novel mammalian autophagy protein interacting with Atg13. Autophagy 5, 973–979. doi: 10.4161/auto.5.7.9296
Huang, R., Xu, Y., Wan, W., Shou, X., Qian, J., You, Z., et al. (2015). Deacetylation of nuclear LC3 drives autophagy initiation under starvation. Mole. Cell 57, 456–466. doi: 10.1016/j.molcel.2014.12.013
Humphrey, J. D., and Milewicz, D. M. (2017). Aging, Smooth Muscle Vitality, and Aortic Integrity. Circulat. Res. 120, 1849–1851. doi: 10.1161/CIRCRESAHA.117.311075
Iwata, A., Riley, B. E., Johnston, J. A., and Kopito, R. R. (2005). HDAC6 and microtubules are required for autophagic degradation of aggregated huntingtin. J. Biol. Chem. 280, 40282–40292. doi: 10.1074/jbc.M508786200
Jiang, F. (2016). Autophagy in vascular endothelial cells. Clin. Exp. Pharmacol. Physiol. 43, 1021–1028. doi: 10.1111/1440-1681.12649
Jiang, Q., Hao, R., Wang, W., Gao, H., and Wang, C. (2016). SIRT1/Atg5/autophagy are involved in the antiatherosclerosis effects of ursolic acid. Mole. Cell Biochem. 420, 171–184. doi: 10.1007/s11010-016-2787-x
Kappel, B. A., Stohr, R., De Angelis, L., Mavilio, M., Menghini, R., and Federici, M. (2016). Posttranslational modulation of FoxO1 contributes to cardiac remodeling in post-ischemic heart failure. Atherosclerosis 249, 148–156. doi: 10.1016/j.atherosclerosis.2016.04.001
Kawaguchi, Y., Kovacs, J. J., McLaurin, A., Vance, J. M., Ito, A., and Yao, T. P. (2003). The deacetylase HDAC6 regulates aggresome formation and cell viability in response to misfolded protein stress. Cell 115, 727–738. doi: 10.1016/S0092-8674(03)00939-5
Ke, Y., Li, D., Zhao, M., Liu, C., Liu, J., Zeng, A., et al. (2018). Gut flora-dependent metabolite Trimethylamine-N-oxide accelerates endothelial cell senescence and vascular aging through oxidative stress. Free Radical Biol. Med. 116, 88–100. doi: 10.1016/j.freeradbiomed.2018.01.007
Khan, S., Bhat, Z. R., and Jena, G. (2016). Role of autophagy and histone deacetylases in diabetic nephropathy: Current status and future perspectives. Genes Dis. 3, 211–219. doi: 10.1016/j.gendis.2016.04.003
Kochl, R., Hu, X. W., Chan, E. Y., and Tooze, S. A. (2006). Microtubules facilitate autophagosome formation and fusion of autophagosomes with endosomes. Traffic 7, 129–145. doi: 10.1111/j.1600-0854.2005.00368.x
Ktistakis, N. T., and Tooze, S. A. (2016). Digesting the Expanding Mechanisms of Autophagy. Trends Cell Biol. 26, 624–635. doi: 10.1016/j.tcb.2016.03.006
Kuwano, K., Araya, J., Hara, H., Minagawa, S., Takasaka, N., Ito, S., et al. (2016). Cellular senescence and autophagy in the pathogenesis of chronic obstructive pulmonary disease (COPD) and idiopathic pulmonary fibrosis (IPF). Respir. Investig. 54, 397–406. doi: 10.1016/j.resinv.2016.03.010
Lan, F., Cacicedo, J. M., Ruderman, N., and Ido, Y. (2008). SIRT1 modulation of the acetylation status, cytosolic localization, and activity of LKB1. Possible role in AMP-activated protein kinase activation. J. Biol. Chem. 283, 27628–27635. doi: 10.1074/jbc.M805711200
Lee, J. Y., Koga, H., Kawaguchi, Y., Tang, W., Wong, E., Gao, Y. S., et al. (2010). HDAC6 controls autophagosome maturation essential for ubiquitin-selective quality-control autophagy. EMBO J. 29, 969–980. doi: 10.1038/emboj.2009.405
Leidal, A. M., Levine, B., and Debnath, J. (2018). Autophagy and the cell biology of age-related disease. Nat. Cell Biol. 20, 1338–1348. doi: 10.1038/s41556-018-0235-8
Li, D. J., Huang, F., Ni, M., Fu, H., Zhang, L. S., and Shen, F. M. (2016). alpha7 Nicotinic Acetylcholine Receptor Relieves Angiotensin II-Induced Senescence in Vascular Smooth Muscle Cells by Raising Nicotinamide Adenine Dinucleotide-Dependent SIRT1 Activity. Arterios. Thromb. Vasc. Biol. 36, 1566–1576. doi: 10.1161/ATVBAHA.116.307157
Li, J., Chen, T., Xiao, M., Li, N., Wang, S., Su, H., et al. (2016). Mouse Sirt3 promotes autophagy in AngII-induced myocardial hypertrophy through the deacetylation of FoxO1. Oncotarget 7, 86648–86659. doi: 10.18632/oncotarget.13429
Li, X., Wang, Y., Xiong, Y., Wu, J., Ding, H., Chen, X., et al. (2016). Galangin Induces Autophagy via Deacetylation of LC3 by SIRT1 in HepG2 Cells. Sci. Rep. 6:30496. doi: 10.1038/srep30496
Li, Y., Xu, W., McBurney, M. W., and Longo, V. D. (2008). SirT1 inhibition reduces IGF-I/IRS-2/Ras/ERK1/2 signaling and protects neurons. Cell Metab. 8, 38–48. doi: 10.1016/j.cmet.2008.05.004
Li, Z., Liu, X., Ma, J., Zhang, T., Gao, X., and Liu, L. (2018). hnRNPK modulates selective quality-control autophagy by downregulating the expression of HDAC6 in 293 cells. Int. J. Oncol. 53, 2200–2212. doi: 10.3892/ijo.2018.4517
Lin, S. Y., Li, T. Y., Liu, Q., Zhang, C., Li, X., Chen, Y., et al. (2012a). GSK3-TIP60-ULK1 signaling pathway links growth factor deprivation to autophagy. Science 336, 477–481. doi: 10.1126/science.1217032
Lin, S. Y., Li, T. Y., Liu, Q., Zhang, C., Li, X., Chen, Y., et al. (2012b). Protein phosphorylation-acetylation cascade connects growth factor deprivation to autophagy. Autophagy 8, 1385–1386. doi: 10.4161/auto.20959
Liu, J., Kang, Y., Yin, S., Chen, A., Wu, J., Liang, H., et al. (2019). Key Role of Microtubule and Its Acetylation in a Zinc Oxide Nanoparticle-Mediated Lysosome-Autophagy System. Small 15:e1901073. doi: 10.1002/smll.201901073
Liu, J., Tang, Y., Feng, Z., Hou, C., Wang, H., Yan, J., et al. (2014). Acetylated FoxO1 mediates high-glucose induced autophagy in H9c2 cardiomyoblasts: regulation by a polyphenol-(-)-epigallocatechin-3-gallate. Metabolism 63, 1314–1323. doi: 10.1016/j.metabol.2014.06.012
Liu, K. P., Zhou, D., Ouyang, D. Y., Xu, L. H., Wang, Y., Wang, L. X., et al. (2013). LC3B-II deacetylation by histone deacetylase 6 is involved in serum-starvation-induced autophagic degradation. Biochem. Biophys. Res. Commun. 441, 970–975. doi: 10.1016/j.bbrc.2013.11.007
Liu, P., Huang, G., Wei, T., Gao, J., Huang, C., Sun, M., et al. (2018). Sirtuin 3-induced macrophage autophagy in regulating NLRP3 inflammasome activation. Biochim. Biophys. Acta Mole. Dis. 1864, 764–777. doi: 10.1016/j.bbadis.2017.12.027
Liu, X., and Klionsky, D. J. (2015). TP53INP2/DOR protein chaperones deacetylated nuclear LC3 to the cytoplasm to promote macroautophagy. Autophagy 11, 1441–1442. doi: 10.1080/15548627.2015.1074373
Liu, Y., Li, J., Shang, Y., Guo, Y., and Li, Z. (2019). CARM1 contributes to skeletal muscle wasting by mediating FoxO3 activity and promoting myofiber autophagy. Exp. Cell Res. 374, 198–209. doi: 10.1016/j.yexcr.2018.11.024
Lopez-Otin, C., Blasco, M. A., Partridge, L., Serrano, M., and Kroemer, G. (2013). The hallmarks of aging. Cell 153, 1194–1217. doi: 10.1016/j.cell.2013.05.039
Ma, Y., Qi, M., An, Y., Zhang, L., Yang, R., Doro, D. H., et al. (2018). Autophagy controls mesenchymal stem cell properties and senescence during bone aging. Aging cell 17:e12709. doi: 10.1111/acel.12709
Mackeh, R., Lorin, S., Ratier, A., Mejdoubi-Charef, N., Baillet, A., Bruneel, A., et al. (2014). Reactive oxygen species, AMP-activated protein kinase, and the transcription cofactor p300 regulate alpha-tubulin acetyltransferase-1 (alphaTAT-1/MEC-17)-dependent microtubule hyperacetylation during cell stress. J. Biol. Chem. 289, 11816–11828. doi: 10.1074/jbc.M113.507400
Majumder, S., and Advani, A. (2015). The epigenetic regulation of podocyte function in diabetes. J. Diabet. Complicat. 29, 1337–1344. doi: 10.1016/j.jdiacomp.2015.07.015
Matsunaga, K., Saitoh, T., Tabata, K., Omori, H., Satoh, T., Kurotori, N., et al. (2009). Two Beclin 1-binding proteins, Atg14L and Rubicon, reciprocally regulate autophagy at different stages. Nat. Cell Biol. 11, 385–396. doi: 10.1038/ncb1846
Mercer, T. J., Gubas, A., and Tooze, S. A. A. (2018). molecular perspective of mammalian autophagosome biogenesis. J. Biol. Chem. 293, 5386–5395. doi: 10.1074/jbc.R117.810366
Mistriotis, P., and Andreadis, S. T. (2017). Vascular aging: Molecular mechanisms and potential treatments for vascular rejuvenation. Ageing Res. Rev. 37, 94–116. doi: 10.1016/j.arr.2017.05.006
Narita, T., Weinert, B. T., and Choudhary, C. (2019). Functions and mechanisms of non-histone protein acetylation. Nat. Rev. Mole. Cell Biol. 20, 156–174. doi: 10.1038/s41580-018-0081-3
Nie, T., Yang, S., Ma, H., Zhang, L., Lu, F., Tao, K., et al. (2016). Regulation of ER stress-induced autophagy by GSK3beta-TIP60-ULK1 pathway. Cell Death Dis. 7:e2563. doi: 10.1038/cddis.2016.423
Ouyang, C., Mu, J., Lu, Q., Li, J., Zhu, H., Wang, Q., et al. (2020). Autophagic degradation of KAT2A/GCN5 promotes directional migration of vascular smooth muscle cells by reducing TUBA/α-tubulin acetylation. Autophagy 16, 1753–1770. doi: 10.1080/15548627.2019.1707488
Pandey, U. B., Nie, Z., Batlevi, Y., McCray, B. A., Ritson, G. P., Nedelsky, N. B., et al. (2007). HDAC6 rescues neurodegeneration and provides an essential link between autophagy and the UPS. Nature 447, 859–863. doi: 10.1038/nature05853
Peserico, A., and Simone, C. (2011). Physical and functional HAT/HDAC interplay regulates protein acetylation balance. J. Biomed. Biotechnol. 2011:371832. doi: 10.1155/2011/371832
Phadwal, K., Kurian, D., Salamat, M. K. F., MacRae, V. E., Diack, A. B., and Manson, J. C. (2018). Spermine increases acetylation of tubulins and facilitates autophagic degradation of prion aggregates. Sci. Rep. 8:10004. doi: 10.1038/s41598-018-28296-y
Pietrocola, F., Galluzzi, L., Bravo-San Pedro, J. M., Madeo, F., and Kroemer, G. (2015). Acetyl coenzyme A: a central metabolite and second messenger. Cell Metab. 21, 805–821. doi: 10.1016/j.cmet.2015.05.014
Plaza-Zabala, A., Sierra-Torre, V., and Sierra, A. (2017). Autophagy and Microglia: Novel Partners in Neurodegeneration and Aging. Int. J. Mole. Sci. 18:598. doi: 10.3390/ijms18030598
Ramirez-Sanchez, I., Mansour, C., Navarrete-Yanez, V., Ayala-Hernandez, M., Guevara, G., Castillo, C., et al. (2018). (-)-Epicatechin induced reversal of endothelial cell aging and improved vascular function: underlying mechanisms. Food Funct. 9, 4802–4813.
Ren, J., and Zhang, Y. (2018). Targeting Autophagy in Aging and Aging-Related Cardiovascular Diseases. Trends Pharmacol. Sci. 39, 1064–1076. doi: 10.1039/C8FO00483H
Ren, J., Yang, L., Zhu, L., Xu, X., Ceylan, A. F., Guo, W., et al. (2017). Akt2 ablation prolongs life span and improves myocardial contractile function with adaptive cardiac remodeling: role of Sirt1-mediated autophagy regulation. Aging Cell 16, 976–987. doi: 10.1016/j.tips.2018.10.005
Rubinsztein, D. C., Marino, G., and Kroemer, G. (2011). Autophagy and aging. Cell 146, 682–695. doi: 10.1111/acel.12616
Sadoul, K., Wang, J., Diagouraga, B., and Khochbin, S. (2011). The tale of protein lysine acetylation in the cytoplasm. J. Biomed. Biotechnol. 2011:970382. doi: 10.1016/j.cell.2011.07.030
Salazar, G., Cullen, A., Huang, J., Zhao, Y., Serino, A., Hilenski, L., et al. (2020). SQSTM1/p62 and PPARGC1A/PGC-1alpha at the interface of autophagy and vascular senescence. Autophagy 16, 1092–1110. doi: 10.1155/2011/970382
Sebti, S., Prebois, C., Perez-Gracia, E., Bauvy, C., Desmots, F., Pirot, N., et al. (2014). BAT3 modulates p300-dependent acetylation of p53 and autophagy-related protein 7 (ATG7) during autophagy. Proc. Natl. Acad. Sci. U S A 111, 4115–4120. doi: 10.1080/15548627.2019.1659612
Sengupta, A., Molkentin, J. D., and Yutzey, K. E. (2009). FoxO transcription factors promote autophagy in cardiomyocytes. J. Biol. Chem. 284, 28319–28331. doi: 10.1073/pnas.1313618111
Shakeri, H., Gevaert, A. B., Schrijvers, D. M., De Meyer, G. R. Y., De Keulenaer, G. W., Guns, P. D. F., et al. (2018). Neuregulin-1 attenuates stress-induced vascular senescence. Cardiovasc. Res. 114, 1041–1051. doi: 10.1074/jbc.M109.024406
Shi, G., Liu, D., Zhou, B., Liu, Y., Hao, B., Yu, S., et al. (2020). Ginsenoside Rb1 Alleviates Oxidative Low-Density Lipoprotein-Induced Vascular Endothelium Senescence via the SIRT1/Beclin-1/Autophagy Axis. J. Cardiovasc. Pharmacol. 75, 155–167. doi: 10.1093/cvr/cvy059
Shirakabe, A., Ikeda, Y., Sciarretta, S., Zablocki, D. K., and Sadoshima, J. (2016). Aging and Autophagy in the Heart. Circulat. Res. 118, 1563–1576. doi: 10.1097/FJC.0000000000000775
Song, Z., Liu, Y., Hao, B., Yu, S., Zhang, H., Liu, D., et al. (2014). Ginsenoside Rb1 prevents H2O2-induced HUVEC senescence by stimulating sirtuin-1 pathway. PLoS One 9:e112699. doi: 10.1161/CIRCRESAHA.116.307474
Stern, S., Behar, S., and Gottlieb, S. (2003). Cardiology patient pages. Aging and diseases of the heart. Circulation 108, e99–e101. doi: 10.1371/journal.pone.0112699
Su, H., and Liu, W. (2018). PIK3C3/VPS34 control by acetylation. Autophagy 14, 1086–1087. doi: 10.1161/01.CIR.0000086898.96021.B9
Su, H., Yang, F., Wang, Q., Shen, Q., Huang, J., Peng, C., et al. (2017). VPS34 Acetylation Controls Its Lipid Kinase Activity and the Initiation of Canonical and Non-canonical Autophagy. Mole. Cell 67, 907.e–921.e. doi: 10.1080/15548627.2017.1385676
Sun, T., Li, X., Zhang, P., Chen, W. D., Zhang, H. L., Li, D. D., et al. (2015). Acetylation of Beclin 1 inhibits autophagosome maturation and promotes tumour growth. Nat. Commun. 6:7215. doi: 10.1016/j.molcel.2017.07.024
Sun, T., Ming, L., Yan, Y., Zhang, Y., and Xue, H. (2017). Beclin 1 acetylation impairs the anticancer effect of aspirin in colorectal cancer cells. Oncotarget 8, 74781–74790. doi: 10.1038/ncomms8215
Tian, S., Guo, X., Yu, C., Sun, C., and Jiang, J. (2017). miR-138-5p suppresses autophagy in pancreatic cancer by targeting SIRT1. Oncotarget 8, 11071–11082. doi: 10.18632/oncotarget.20367
Wan, W., You, Z., Xu, Y., Zhou, L., Guan, Z., Peng, C., et al. (2017). mTORC1 Phosphorylates Acetyltransferase p300 to Regulate Autophagy and Lipogenesis. Mole. Cell 68, 323.e–335.e. doi: 10.1016/j.molcel.2017.09.020
Wang, B., Ding, W., Zhang, M., Li, H., Guo, H., Lin, L., et al. (2016). Role of FOXO1 in aldosterone-induced autophagy: a compensatory protective mechanism related to podocyte injury. Oncotarget 7, 45331–45351.
Wang, B., Yang, Q., Sun, Y. Y., Xing, Y. F., Wang, Y. B., Lu, X. T., et al. (2014). Resveratrol-enhanced autophagic flux ameliorates myocardial oxidative stress injury in diabetic mice. J. Cell. Mole. Med. 18, 1599–1611. doi: 10.18632/oncotarget.9644
Wang, C., Xu, W., Zhang, Y., Zhang, F., and Huang, K. (2018). PARP1 promote autophagy in cardiomyocytes via modulating FoxO3a transcription. Cell Death Dis. 9:1047. doi: 10.1111/jcmm.12312
Wang, R., Tan, J., Chen, T., Han, H., Tian, R., Tan, Y., et al. (2019). ATP13A2 facilitates HDAC6 recruitment to lysosome to promote autophagosome-lysosome fusion. J. Cell Biol. 218, 267–284. doi: 10.1038/s41419-018-1108-6
Wani, W. Y., Boyer-Guittaut, M., Dodson, M., Chatham, J., Darley-Usmar, V., and Zhang, J. (2015). Regulation of autophagy by protein post-translational modification. Lab. Investig. 95, 14–25. doi: 10.1083/jcb.201804165
Warr, M. R., Binnewies, M., Flach, J., Reynaud, D., Garg, T., Malhotra, R., et al. (2013). FOXO3A directs a protective autophagy program in haematopoietic stem cells. Nature 494, 323–327. doi: 10.1038/labinvest.2014.131
Xie, R., Nguyen, S., McKeehan, W. L., and Liu, L. (2010). Acetylated microtubules are required for fusion of autophagosomes with lysosomes. BMC Cell Biol. 11:89. doi: 10.1038/nature11895
Xie, Y., Kang, R., Sun, X., Zhong, M., Huang, J., Klionsky, D. J., et al. (2015). Posttranslational modification of autophagy-related proteins in macroautophagy. Autophagy 11, 28–45. doi: 10.1186/1471-2121-11-89
Xiong, S., Salazar, G., Patrushev, N., Ma, M., Forouzandeh, F., Hilenski, L., et al. (2013). Peroxisome proliferator-activated receptor gamma coactivator-1alpha is a central negative regulator of vascular senescence. Arterioscler. Thromb. Vasc. Biol. 33, 988–998. doi: 10.4161/15548627.2014.984267
Xu, P., Das, M., Reilly, J., and Davis, R. J. J. N. K. (2011). regulates FoxO-dependent autophagy in neurons. Genes Dev. 25, 310–322. doi: 10.1161/ATVBAHA.112.301019
Yang, D., Xiao, C., Long, F., Su, Z., Jia, W., Qin, M., et al. (2018). HDAC4 regulates vascular inflammation via activation of autophagy. Cardiovasc. Res. 114, 1016–1028. doi: 10.1101/gad.1984311
Yang, L., Zhao, L., Cui, L., Huang, Y., Ye, J., Zhang, Q., et al. (2019). Decreased alpha-tubulin acetylation induced by an acidic environment impairs autophagosome formation and leads to rat cardiomyocyte injury. J. Mole. Cell. Cardiol. 127, 143–153. doi: 10.1093/cvr/cvy051
Yang, Y., Fiskus, W., Yong, B., Atadja, P., Takahashi, Y., Pandita, T. K., et al. (2013). Acetylated hsp70 and KAP1-mediated Vps34 SUMOylation is required for autophagosome creation in autophagy. Proc. Natl. Acad. Sci.U S A 110, 6841–6846. doi: 10.1016/j.yjmcc.2018.12.011
Yi, C., and Yu, L. (2012). How does acetylation regulate autophagy? Autophagy 8, 1529–1530. doi: 10.1073/pnas.1217692110
Zachari, M., and Ganley, I. G. (2017). The mammalian ULK1 complex and autophagy initiation. Essays Biochem. 61, 585–596. doi: 10.4161/auto.21156
Zarzuelo, M. J., Lopez-Sepulveda, R., Sanchez, M., Romero, M., Gomez-Guzman, M., Ungvary, Z., et al. (2013). SIRT1 inhibits NADPH oxidase activation and protects endothelial function in the rat aorta: implications for vascular aging. Biochem. Pharmacol. 85, 1288–1296. doi: 10.1042/EBC20170021
Zhang, H., Puleston, D. J., and Simon, A. K. (2016). Autophagy and Immune Senescence. Trends Mole. Med. 22, 671–686. doi: 10.1016/j.bcp.2013.02.015
Zhang, X., Yuan, Z., Zhang, Y., Yong, S., Salas-Burgos, A., Koomen, J., et al. (2007). HDAC6 modulates cell motility by altering the acetylation level of cortactin. Mole. Cell 27, 197–213. doi: 10.1016/j.molmed.2016.06.001
Zhang, Y., Zhang, M., Dong, H., Yong, S., Li, X., Olashaw, N., et al. (2009). Deacetylation of cortactin by SIRT1 promotes cell migration. Oncogene 28, 445–460. doi: 10.1016/j.molcel.2007.05.033
Zhao, J., Brault, J. J., Schild, A., and Goldberg, A. L. (2008). Coordinate activation of autophagy and the proteasome pathway by FoxO transcription factor. Autophagy 4, 378–380. doi: 10.1038/onc.2008.388
Zhao, J., Brault, J. J., Schild, A., Cao, P., Sandri, M., Schiaffino, S., et al. (2007). FoxO3 coordinately activates protein degradation by the autophagic/lysosomal and proteasomal pathways in atrophying muscle cells. Cell Metabol. 6, 472–483. doi: 10.4161/auto.5633
Zhao, Y., Wang, L., Yang, J., Zhang, P., Ma, K., Zhou, J., et al. (2010). Anti-neoplastic activity of the cytosolic FoxO1 results from autophagic cell death. Autophagy 6, 988–990. doi: 10.1016/j.cmet.2007.11.004
Zhong, Y., Wang, Q. J., Li, X., Yan, Y., Backer, J. M., Chait, B. T., et al. (2009). Distinct regulation of autophagic activity by Atg14L and Rubicon associated with Beclin 1-phosphatidylinositol-3-kinase complex. Nat. Cell Biol. 11, 468–476. doi: 10.4161/auto.6.7.13289
Keywords: autophagy, acetylation, FOXO1, SIRT1, vascular aging
Citation: Sun J, Tai S, Tang L, Yang H, Chen M, Xiao Y, Li X, Zhu Z and Zhou S (2021) Acetylation Modification During Autophagy and Vascular Aging. Front. Physiol. 12:598267. doi: 10.3389/fphys.2021.598267
Received: 24 August 2020; Accepted: 26 February 2021;
Published: 22 March 2021.
Edited by:
Stefano Tarantini, University of Oklahoma Health Sciences Center, United StatesReviewed by:
Bing Gong, Nanjing Drum Tower Hospital, ChinaChao Peng, Shanghai Advanced Research Institute, China
Copyright © 2021 Sun, Tai, Tang, Yang, Chen, Xiao, Li, Zhu and Zhou. This is an open-access article distributed under the terms of the Creative Commons Attribution License (CC BY). The use, distribution or reproduction in other forums is permitted, provided the original author(s) and the copyright owner(s) are credited and that the original publication in this journal is cited, in accordance with accepted academic practice. No use, distribution or reproduction is permitted which does not comply with these terms.
*Correspondence: Shenghua Zhou, emhvdXNoZW5naHVhQGNzdS5lZHUuY24=
†These authors share first authorship