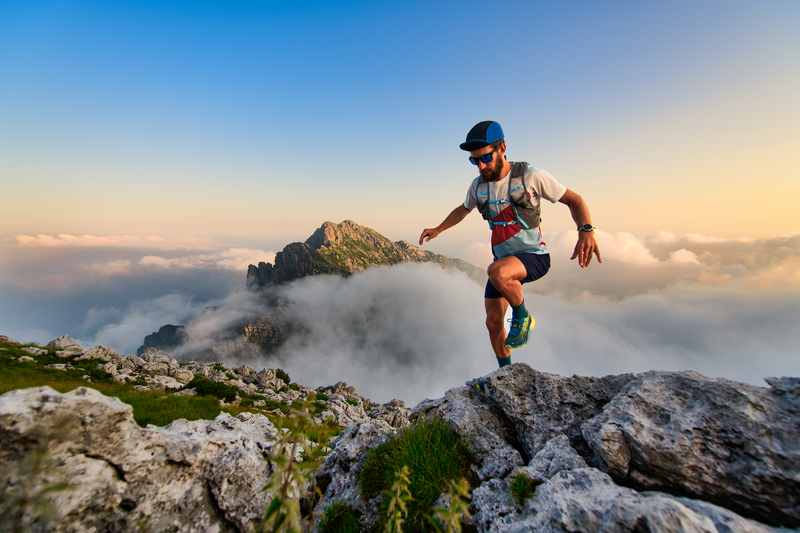
95% of researchers rate our articles as excellent or good
Learn more about the work of our research integrity team to safeguard the quality of each article we publish.
Find out more
REVIEW article
Front. Physiol. , 04 March 2021
Sec. Gastrointestinal Sciences
Volume 12 - 2021 | https://doi.org/10.3389/fphys.2021.574913
Lead (Pb) is a toxic heavy metal, having profound threats to the global population. Multiple organs such as kidney, and liver, as well as nervous, hematologic, and reproductive systems, are commonly considered the targets of Pb toxicity. Increasing researches reported that the effects of Pb on gastrointestinal tracts are equally intensive, especially on intestinal microbiota. This review summarized Pb toxicity on gut physiology and microbiota in different animal models and in humans, of which the alterations may further have effects on other organs in host. To be more specific, Pb can impair gut barrier and increase gut permeability, which make inflammatory cytokines, immunologic factors, as well as microbial metabolites such as bile acids (BA) and short-chain fatty acids (SCFAs) enter the enterohepatic circulation easily, and finally induce multiple systematic lesion. In addition, we emphasized that probiotic treatment may be one of the feasible and effective strategies for preventing Pb toxicity.
Lead (Pb), one of the non-essential heavy metals, which is widely considered an environmental pollutant, possesses quite serious health hazards (Watson et al., 2005). In particular, this universal metal exists as a major global public health issue due to its widespread environmental pollution, superior ability to induce a broad spectrum of toxic effects, and the quantity of susceptible demographic groups (Satcher, 2000). It is well known that most urban soils are contaminated with Pb (Mielke et al., 1984; Datko-Williams et al., 2014). Researchers documented that central tendency of Pb concentrations in urban soils ranges from about 100 to 1,000 mg/kg, which is higher than environmental background value (Mielke et al., 1983; Clark et al., 2008; Szolnoki et al., 2013; Mitchell et al., 2014). In recent years, the phasing out of Pb in gasoline and the limitation of Pb content in paint have greatly reduced the heavy metal’s exposure in America (Bellinger, 2016). However, human Pb exposure cannot be simply ignored. Several investigators reported that the average Pb concentration in blood of children in low- and middle-income countries including China (3.71 μg/dl) (Li T. et al., 2017), India (5.46 μg/dl) (Rashid et al., 2019), and Egypt (6 μg/dl) (Mostafa et al., 2009) were higher than that obtained for children in the United States (0.84 μg/dl) (Tsoi et al., 2016). Moreover, Pb exposure can cause health effects at a quite low level (2 μg/dl) (Gilbert and Weiss, 2006). Thus, further reduction is urgently required.
Heavy social burdens of Pb exposure are produced owing to its role in many disorders. Particularly, this heavy metal causes notable neurotoxicity and cognitive developmental problems, for which children are the most susceptible ones than adults (Flora et al., 2012; Evens et al., 2015; Al Osman et al., 2019). Aside from the nervous system, complications of the liver, kidney, hematopoietic, circulatory, cardiovascular, reproductive, gastrointestinal, immunological, and renal systems may also occur from Pb intoxication (Ahamed and Siddiqui, 2007; Navas-Acien et al., 2007; Flora et al., 2012; Riaz et al., 2016). Scholars estimated that Pb exposure significantly increases mortality by 18.0% in the United States (Lanphear et al., 2018).
The mechanisms of Pb’s effects on target organs are universally considered to be general metal toxicity like any other heavy metals. Numerous studies reported that Pb has the capability of relieving oxidative stress (Jomova and Valko, 2011; Huang et al., 2019), inflammation (Metryka et al., 2018), immune response (Gao et al., 2007), and essential metal dyshomeostasis (Liu et al., 2014). In a recent study, the metal was found to function as an endocrine disruptor (He et al., 2017). Unfortunately, these may not address the mechanisms of Pb toxicity; nonetheless, further investigations which will be in favor of appraisal and prevention of the metal’s effects are highly required.
Gut microbiome, well established as our “second genome” (Ley et al., 2006; Zmora et al., 2016), has received increasing attention due to its significant role in a series of physiological functions. For example, regulating host metabolism, immunity, and inflammation, are reactions tightly associated with health and disease (Tremaroli and Backhed, 2012; Dabke et al., 2019). Dysbiosis or disruption of gut microbiota may mediate the process and progress diverse diseases, including allergies (Ver Heul et al., 2019), cancer (Marchesi et al., 2016; Gao R. et al., 2017; Yu and Schwabe, 2017), cardiovascular diseases (Org et al., 2015), obesity (Cavalcante-Silva et al., 2015), intestinal diseases (Ni et al., 2017; Nishida et al., 2018), neurological diseases (Cryan and Dinan, 2012; Mangiola et al., 2016), and diabetes (Wu et al., 2017). Recent reports have suggested gut microbiome as a target for multiple environmental pollutants including persistent organic pollutants, pesticides, antibiotics, nanoparticles, air pollution, endocrine-disrupting chemicals, microplastics, mycotoxins, as well as heavy metals (Becattini et al., 2016; Jin et al., 2017; Velmurugan et al., 2017; Liew and Mohd-Redzwan, 2018; Fackelmann and Sommer, 2019; Yuan et al., 2019; Feng et al., 2020). However, there exists limited knowledge about the possible role of disordered intestinal microbes in Pb toxicity.
Therefore, in this review, we primarily reviewed the effects of Pb exposure on gut microbiota, intestinal structure and function, as well as relevant host health. In addition, we estimated the data for whether gut and its microbiome may be considered mediators for Pb toxicity on other organs, and probiotics treatment may be an operative strategy to prevent Pb toxicity.
Gut microbiota has been recognized as an “organ” on itself, which consists of more than 1014 microbes and surprisingly possesses 150 times more genes than the human genome (Ley et al., 2006; O’Toole and Jeffery, 2015). Gut microbiome of humans is mainly composed of two major phyla, Bacteroidetes, and Firmicutes. Some researchers considered the F/B ratio (Firmicutes vs. Bacteroidetes) crucial to human health, while some do not (Turnbaugh et al., 2009; Zhang et al., 2016; Stojanov et al., 2020). Accumulating literature shows that intestinal microbiota plays a critical part in the development of various diseases, such as cardiovascular diseases, diabetes, obesity, neurological diseases, cancer, and gastrointestinal diseases (Clemente et al., 2012; Lo Presti et al., 2019). Interestingly, gut microbiota also acts as a protective element for heavy metal toxicity, particularly Pb. Increasing reports demonstrated that metabolites derived from microorganisms, including SCFAs, BA, amino acid derivatives, liposaccharides (LPS), and vitamins were crucial signaling molecules linking gut microbiota-host responses (Adak and Khan, 2019; Wang R.X. et al., 2020). The intestinal microbiota is susceptible to the impacts of Pb exposure, generally including alteration of composition, diversity, as well as related metabolites of microbiota.
Short-term Pb exposure was reported to induce immediate impacts on gut microbiome. In an early study (Sadykov et al., 2009), a significant increase in lactose-negative Escherichia coli in the gut microbiota was observed in adult rats after 2 weeks of Pb oral exposure. It is worth knowing that, Pb treatment has profound effects on gut microbiome at phylum, order, and genus levels. It has been demonstrated that Pb (30 μg/L) exposure for 7 days induced significant changes in microbial richness and diversity, a marked increase in Firmicutes and Bacteroidetes, as well as a significant reduction in Fusobacteria, and Proteobacteria in gut microbiota on phylum level of zebrafish. Further metabolomics analysis of the liver indicated that a total of 41 metabolites involved in glucose, lipid, amino acid, and nucleotide metabolism were altered. Consistently, the expression of glycolysis and lipid metabolism-related genes, including Gk, Aco, Acc1, Fas, Apo, and Dgat, were notably declined (Xia et al., 2018b). Patsiou et al. (2020) also reported a notable increase in the phylum of Alphaproteobacteria and a reduction in Gammaproteobacteria of zebrafish following Pb (500 mg/kg) exposure via drinking water for 14 days. At the order level, the relative abundance of Alteromonadales was decreased with significance. At the genus level, gut microbiota was featured by an upregulation in the relative abundance of Pseudomonas, Halomonadaceae, Arcobacter, and Polaribacter (Patsiou et al., 2020). Interestingly, specific Pseudomonas strains were reported to be Pb-tolerant bacteria in Pb-contaminated water and soil (Li D. et al., 2017), which revealed that some Pb-against bacteria may exist in zebrafish.
In addition to the short-term Pb exposure, chronic situations are more common. Chronic Pb exposure has been reported to alter both microbial biodiversity and richness to cause dysbiosis of gut microbiota. Cultivable anaerobes increased while cultivable aerobes decreased in the feces of adult mice, which suffered from a constant 2 ppm Pb exposure by drinking water during gestation and lactation periods. In particular, the relative abundance of Firmicutes significantly increased, while Bacteroidetes exhibited the opposite trend, which indicated that the Pb exposure enhanced the abundant ratio of Firmicutes vs. Bacteroidetes (F/B) to a certain extent (Wu et al., 2016). In some views, an abnormal abundant ratio of F/B is recognized as a vital biomarker of adiposity and lipid metabolic disorder (Turnbaugh et al., 2009; Stojanov et al., 2020). Moreover, the authors also detected a total of six taxonomic genera change by early life Pb exposure, with three genera dropped (Lactococcus, Enterorhabdus, and Caulobacterales) and three raised (Desulfovibrionaceae, Barnesiella, and Clostridium XIVb). Desulfovibrio was reported to convert choline to trimethylamine (TMA), which further oxidized to TMA N-oxide (TMAO) in liver. Accumulation of TMAO was highly correlated to colon cancer and cardiovascular diseases (Baker et al., 1962; Tang et al., 2013; Bae et al., 2014). Lactococcus was reported to be probiotics being widely applied in food (Juturu and Wu, 2018). Further correlation analysis revealed that these alterations of microbiota were highly correlated to increased body weight in only males. Oppositely, the F/B ratio showed an evident reduction in the gut microbiota of Japanese quails after 49 days of Pb (1,000 ppm) (Kou et al., 2019). In particular, at the genus level, intestinal bacterial communities were characterized by a remarkable increase in the relative abundance of Bacteroides, and a reduction of Faecalibacterium and Bifidobacteria, accompanied with disrupted intestinal structure and altered immune status. There also exist studies that reported controversial data for the F/B ratio (Breton et al., 2013b; Xia et al., 2018a; Kou et al., 2019). Thus, whether the F/B ratio is a fair index for Pb’s toxic effects needs further explorations. Breton et al. (2013b) investigated the fecal and cecal microorganisms of Balb/C mice affected by Pb at an environmental dose (100 and 500 ppm) via drinking water for 8 consecutive weeks. Minor but specific alterations in gut microbiota at both family and genera levels were observed based on 16S rRNA pyrosequencing. The abundance of Lachnospiraceae was reduced, whereas the abundance of Lactobacillaceae and Erysipelotrichaceae were enhanced in the feces and cecum contents of Pb-administrated mice as compared with the control group. Zhai et al.’s (2019c) study also revealed a significant increase of Ruminococcus and a decrease of Turicibacter in the intestinal microbiome on genera level following C57BL/6 mice exposure to Pb (1 g/L) in drinking water for 8 weeks. However, findings of the changes in Bacteroidetes and Firmicutes were of no significance. In the same year, Cheng et al. (2019) also observed that Pb resulted in notable changes in gut microbiome of Kunming mice following the same Pb exposure duration and dose, which were distinguished by a significantly higher population of Lachnospiraceae_NK4A136_group, and a lower population of Helicobacter, together with structure and function damage of kidney and liver, as well as cognitive impairment. There were no consistent changes of microbes in these two studies, which might be attributed to the distinct species of mice. Zhai’s study demonstrated that alteration in the intestinal microbiome induced by Pb exposure was much more obvious in the first 4 weeks as compared with the latter 4 weeks (Zhai et al., 2017a). The authors further suggested the existence of a sensitive period of gut microbiota to Pb. Another interesting phenomenon was that Pb exposure dominantly reduced the population of certain bacteria. For example, eight core OTUs were significantly reduced at the end of 8 weeks’ Pb exposure, whereas no significant upregulation was observed in any genera. Consistent with Monast’s report, chronic Pb exposure reduced the relative abundance of Ruminococcaceae family strains, and resulted in further gut dysfunction (Monast et al., 2016), and chronic Pb exposure reduced the relative abundance of Ruminococcaceae family strains, which may result in further gut dysfunction. Interestingly, the abundance of Akkermansia, considered a marker for colitis (Wang L. et al., 2020), was pronounceably downregulated. According to previous reports, the alterations of these core gut strains were correlated with intestinal inflammation, colitis, and other gut disorders (McLellan et al., 2013; Schwab et al., 2014; Breton et al., 2016). For example, Oscillibacter strains were reported to be valeric acid producing bacteria, possessing the potential to be negatively related to gut dysfunction (Park et al., 2014). Certain Lachnoclostridium strains were involved in the production of secondary bile acids (Ridlon et al., 2015). Furthermore, a significant reduction in the abundance of Ruminoclostridium in IBD patients has been reported (Monast et al., 2016). In addition, a significant decline in diversity of intestinal microbiome was detected by Giri et al. (2018), especially the population of lactic acid bacteria (LAB) in Cyprinus carpio fish, exposed to Pb (1 mg/L) for 6 weeks. Gao B. et al. (2017) adopted the 16S rRNA sequencing as well as metagenomics to examine the gut microbiota of mice stool after Pb (10 ppm) exposure by drinking water for 0, 4, and 13 weeks. As expected, the α-diversity and microbiome community structures (assessed by β-diversity metrics) at weeks 4 and 13 were significantly altered. Moreover, the changes of gut microorganisms were almost consistent with Zhai et al.’s (2017a) study. With regard to chronic exposure to low Pb concentration in mice (0.1 mg/L Pb for 15 weeks), the β-diversity was significantly changed (Xia et al., 2018a). Specifically, the relative abundance of Firmicutes was reduced, whereas Bacteroidetes was increased, leading to a downregulation of F/B ratio. At genus level, the percentage of Parabacteroides strains increased, while the percentage of Dehalobacterium population dropped. Parabacteroides, considered opportunistic pathogens, are generally involved in infectious diseases such as intra-abdominal processes and bacteremia (Aldridge, 1995). Moreover, some critic genes related to hepatic lipid metabolism were notably upregulated, consisted with increased hepatic total cholesterol (TCH) and triglyceride (TG) levels, indicating a high-relevant status between changed gut microbes and hepatic function disturbance (Aldridge, 1995).
Exposure to Pb can also disturb the homeostasis of human intestinal microorganisms. Accumulated reports have demonstrated that Pb exposure has profound negative impacts on human gut microbiota both in adults and children. Bisanz et al. (2014) discovered that increasing blood Pb levels were related to a higher relative abundance of Succinivibrionaceae and Gammaproteobacteria in feces among Tanzanian pregnant women and school children. Recently, Eggers et al. (2019) investigated urinary Pb concentration and composition of gut microbiota in 696 adult participants in the Survey of the Health of Wisconsin (SHOW) cohort. Results suggested positive associations between α-diversity, richness of gut microbiome, and urinary Pb levels, while different microbial β-diversity were linked to different urinary Pb levels. In particular, the upregulated percent of Proteobacteria population, including members of the Burkholderiales, were significantly related to increased urine Pb. Shao and Zhu (2020) also adopted 16S rRNA sequencing to investigate the gut microbiota of residents surrounding a mining and smelting area. The microbial diversity and composition profile were altered due to long-term exposure to multiple metals including Pb (Shao and Zhu, 2020). Additionally, a higher abundance of Lachnospiraceae, Eubacterium eligens, Ruminococcaceae UGG-014, Erysipelotrichaceae UCG-003, Tyzzerella 3, Bacteroides, Slackia, italics, and Roseburia and lower abundance of Prevotella 9 were observed in the stool of various metal exposure participants. Moreover, there existed some differences between females and males, in which microbial richness and evenness were greater for men who received long-term metal exposure in the mining and smelting areas. Reports indicate that, Lachnospiraceae, Erysipelotrichaceae, and Eubacterium eligens are usually considered to be linked to intestinal inflammation. While Roseburia is associated with immunity maintenance and anti-inflammatory properties, Ruminococcaceae, is majorly associated with mucosa, and is generally recognized as beneficial bacteria (Tamanai-Shacoori et al., 2017).
Therefore, Pb exposure does have varying degrees of impacts on intestinal flora no matter the animal model or human on the basis of substantial studies, and are summarized in Table 1. More generally, a marked increase in the F/B ratio was suggested in most of the previous studies, attributed to increased Firmicutes population and/or decreased Bacteroidetes population. However, there are some other studies with the opposite results and the rest with no significant changes. In addition, some key bacteria producing SCFAs, BA, and/or other materials related to host disease were differently altered after Pb exposure, such as Desulfovibrio, Lactococcus, Akkermansia, Ruminococcus, and Turicibacter. The existing contradictions of Pb effects on specific intestinal microbes may be attributed to the distinct exposure dose, time duration, exposure period of life, different animal models, or different subjects.
Microbial metabolites can interact with the host system locally and systemically, which may trigger intensive biological effects (Holmes et al., 2011). Changes of relative abundance in gut flora consequently influence the microbial metabolic profiles (Sharon et al., 2014; Adak and Khan, 2019). Typical intestinal microbial metabolites such as bile acids and SCFAs, generally act as signaling molecules and bind to cellular receptors (Koh et al., 2016; Wahlström et al., 2016; Wang R.X. et al., 2020). Specifically, bile acids can bind to GPCR TGR5 and nuclear receptor farnesin X receptor (FXR), and SCFAs can bind to G-protein-coupled receptors (GPCRs) (Tremaroli and Backhed, 2012). In addition, upregulation of SCFAs (especially butyric acid) can lower the pH of intestinal lumen and modulate the mucin synthesis and secretion, which may further enhance the intestinal barrier function (Wong et al., 2006; Burger-van Paassen et al., 2009). Activation of signaling pathways may trigger crucial biological effects. Pb exposure is found to have impacts on gut bacteria and some metabolic pathways. Gao B. et al. (2017) adopted multi-omics approaches and elucidated alteration of the microbiome and relevant metabolic profile of C57 BL/6 mice induced by Pb (10 ppm) for 4 and 13 weeks. Data showed that microbial metabolic profiles were seriously perturbed by Pb exposure. In particular, 1,314 molecular features with p < 0.05 and fold changes > 1.5 were identified, and specifically considerable metabolic pathways, including vitamin E, bile acids, nitrogen metabolism, energy metabolism, oxidative stress, and the defense/detoxification mechanism, were changed. The levels of vitamin E and bile acids were significantly disturbed. For example, α-tocopherol and γ-tocopherol, the primary bile acids cholic acid and ursodeoxycholic acid, the secondary bile acid deoxycholic acid, and cholesterol, as well as its derivative coprostanol, were notably reduced in Pb-treated group. Nitric oxide (NO) plays a key role in physiological and pathophysiological events in the gut (Cho, 2001; Lamattina et al., 2003). It was further suggested that Pb exposure may increase NO generation in the gut bacteria for gene encoding copper-containing nitrite reductase upregulated after 13-week Pb exposure. Applying nuclear magnetic resonance (1NMR) analysis, Xia et al. (2018a) observed that 15 metabolites in cecum contents were significantly changed in mice exposed to a low concentration of Pb (0.1 mg/L) in drinking water for 15 weeks. An increase in the levels of 4-guanidinobutyrate, choline, citrate, glutamate, isobutyrate (a short-chain fatty acid, SCFA), and lysine, as well as a decrease of alanine, glycine, isoleucine, leucine, phenylalanine, tyrosine, valine, and β-galactose were noted. Furthermore, these microbial metabolites were involved in amino acid metabolism, the tricarboxylic acid cycle (TCA cycle), and energy metabolism in host. Interestingly, the related metabolic genes in the host exhibited consistent changes with microbial metabolites. For example, the genes related to lipid metabolism in the liver, some involved in both de novo fatty acids synthesis and transport pathways as well as genes involved in TG synthesis were upregulated in a dose-dependent manner in liver of mice treated with Pb. Thus, the authors hypothesized that the gut microbiome and its metabolites might be closely associated with the perturbation of lipid metabolism in mice exposed to Pb. Cheng et al. (2019) also demonstrated that the levels of fecal SCFAs, such as propionate, butyrate, and acetate, were notably reduced in mice after 8-week Pb exposure compared with the control group. These findings are in agreement with Zhai et al.’s (2019b) earlier study.
Taken together, the evidences above support that Pb exposure has the ability to induce dysbiosis of gut flora as well as their metabolites, which may consequently interact with host metabolism, and therefore contribute to host health. However, the specific microbe and its metabolites were not fully elucidated in these researches. It is speculated that some important key microbes, not all the microbiota, affect the intestinal health as well as host health. Multi-omics such as metagenome, metabolome, and transcriptome may need further application to investigate the progress profoundly.
It has been reported that Pb exposure significantly affected the structure and barrier function of the small intestine in rats. Specifically, rough surface villi, extensive areas with degenerative lesions, and microvilli of enterocytes within these areas sometimes completely absent were observed by scanning electron microscopy (Tomczok et al., 1988). However, the results of Breton’s research (Breton et al., 2013a) held a relatively harmless view of Pb toxicity on gut barrier and permeability. Histological features of the ileum, duodenum, and colon of mice administrated with Pb (100 and 500 mg/L) for 4, 8, and 12 weeks via drinking water were consistent with the control group. In particular, the length of villi, depth of crypts, and number of goblet and Paneth cells in the small intestine were unchanged. Moreover, genes related to intestinal barrier functions such as ZO-1, Foxp3, and Foxo4 in different intestinal parts were almost unchanged by Pb. Regarding the inflammatory-related gene expression, a significant reduction for both proinflammatory mRNA (Il1b, Tnf, and Ifng) and anti-inflammatory mRNA (Tgfb and Il-10) were detected. Their later study (Breton et al., 2016) demonstrated that transepithelial electric resistance was reduced in human cell-based models. Zhai et al.’s (2019c) research showed that oral administration of high concentration of Pb (1 g/L) for 8 weeks in mice remarkably upregulated the levels of serum DX-4000-FITC and downregulated the mRNA expression of colonic Muc2 as well as intestinal tight junctions (TJ), including ZO-1, claudin-1, and occludin, accompanied with an increasing abundance of Ruminococcus and a decreasing abundance of Turicibacter, indicating severely destroyed gut barrier and permeability. This team further evaluated Pb toxicity on gut permeability in mice at colon and small intestine, respectively. The gene expression level of ZO-1, ZO-2, claudin-1, and occludin in both colon and small intestine decreased significantly in Pb-treated mice (Zhai et al., 2019b). In addition, an interesting phenomenon was observed by the authors. The mRNA expression of tight junction was further downregulated in Pb-treated mice with a predepletion of gut microbiome. This result gave an evidence that intestinal commensal bacteria might compete with gut for Pb absorption, thereby limiting the bioavailability of Pb. Kou et al.’s (2019) findings also indicated that chronic Pb exposure had intensive effects on cecum histology of Japanese quails, including mucosa abscission, Lieberkühn glands destruction, and lymphocyte hyperplasia. Ultrastructural damages characterized by nucleus pyknosis, mitochondrial vacuolation, and microvilli contraction were further observed via transmission electron microscope. Moreover, changes in cecum morphology was related to downregulated expression levels of IL-2 and IFN-γ, while upregulated levels of IL-6, TNF-α, and NF-κB, accompanied by increased Bacteroides whereas decreased Faecalibacterium and Bifidobacteria abundance (Supplementary Figure 1).
The enterohepatic circulation has been intensively reported in previous studies, by which numerous materials in gut such as bile acids (linkage of gut-liver axis) can play vital roles in lipid metabolism, heavy metal excretion, glucose homeostasis, hepatic bile formation, and intestinal function in the host (Jones et al., 2008; Hofmann and Hagey, 2014; Ridlon and Bajaj, 2015). An investigation implemented by Ma et al. (2018), using liver cancer model showed that primary BA accumulation increased natural killer T cell population and inhibited liver tumor growth. Increasing studies indicate that the intestinal microbiome performs a critical role in regulating bile acid homeostasis (Tremaroli and Backhed, 2012; Adak and Khan, 2019). Pb treatment in mice significantly changed intestinal microbial composition as well as mRNA expression of BA metabolic genes such as Cyp8b1 in the liver and Fgf15 in the ileum (Zhai et al., 2019c). Xia et al. (2018a) reported that chronic Pb exposure led to liver metabolism perturbance, including lipid metabolism and TCA circle, accompanied by alteration of gut microbes and key genes related to lipid metabolism. Moreover, the authors further detected marked changes in hepatic metabolism and core genes associated with hepatic lipid and glycolysis metabolism (Gk, Aco, Acc1, Fas, Apo, and Dgat) in Pb-treated zebrafish (Xia et al., 2018b). Moreover, relative abundance of certain gut microbes were changed and were reported to be tightly associated with lipid and glycolysis metabolism, disease and inflammation, such as Bacteroides, Flavobacterium, Roseburia, Alloprevotella, and Ruminococcus. Zhai et al. (2019b) reported a significant reduction of SCFAs, especially butyric acid, when mice were orally exposed to Pb (1,304 mg/kg) for 3 days. Use of antibiotics in mice model of liver disease suggested that depletion of intestinal flora can alleviate liver inflammation by reducing the transport of lipopolysaccharide in liver (Tripathi et al., 2018). As described in Sections “Effect of Pb on intestinal microbiota” and “Effects of Pb on intestinal barrier,” these evidences indicate that the manipulation of gut microbiota composition, diversity, or richness affects BA, SCFA metabolism, and/or other metabolisms and may mediate the interactions between the gut and liver.
Quite a few studies about probiotics treatment functioning as a therapeutic method in alleviating Pb toxicity have been published (Supplementary Figure 2), as Pb is considered one of the most toxic heavy metals. Topcu and Bulat (2010) firstly investigated the Pb-removal properties of Enterococcus faecium strains (E. faecium EF031 and E. faecium M74) in water. The preliminary results suggested that both two E. faecium stains could bind the Pb efficiently. Bhakta et al. (2012) isolated and identified some Pb-removal lactic acid bacteria (LAB) from the environment, of which E. faecium Pb12 strains were the most excellent stains exhibiting the potential for uptaking Pb from fish intestine and further reducing Pb bioaccumulation in tissues and organs. In a recent study, Giri et al. (2019) reported that Lactobacillus reuteri P16, isolated from intestinal contents of Cyprinus carpio fish, possessed efficient Pb-binding ability (>15% Pb removal), extraordinary antioxidant activity (hydroxyl radical-scavenging, 42.18%), as well as satisfactory probiotics properties (high tolerance to both bile and acid in vitro). Daisley et al. (2019) also adopted a Caco-2 model of the intestinal epithelium and investigated the anti-Pb property of Lactobacillus rhamnosus GR-1 (LGR-1). The results showed that Pb formed anomalous cell-surface clusters on LGR-1. LGR-1 possessed the ability to effectively reduce apical-to-basolateral translocation of Pb, which thus availably reduced Pb translocation across the intestinal epithelium. Regarding probiotics against Pb toxicity in vivo, multiple investigations have also been conducted in the past few years. Having identified Lactobacillus reuteri P16 as an effective Pb-removal strain, Giri et al. (2018) further explored its therapeutic effects for waterborne Pb toxicity in fish (Cyprinus carpio). The fish were exposed to waterborne Pb (1 mg/L) with L. reuteri P16 (108 CFU/g) supplementary diet provided for 6 weeks. Results demonstrated that this probiotic could decrease mortality and Pb accumulation in tissues, improve the growth performance, as well as partly reverse Pb-induced alterations of host, including biochemical parameters, oxidative stress, intestinal enzymatic activities, intestinal microbiota, and expressions of proinflammatory cytokines (TNF-α and IL-1β) and heat shock proteins (HSP70 and HSP90). Yi et al. (2017) also isolated a lead-resistant LAB strain 96 from a portion of Korean fermented food, identified as Leuconostoc mesenteroides. Use of Leuconostoc mesenteroides significantly decreased the levels of glutamate oxaloacetate transaminase and glutamate pyruvate transaminase, and particularly restored partial male reproductive function such as the ATP content and mobility of epididymal spermatozoa in Pb-administrated mice. Correspondingly, oral supplementation of Pb-intolerant gut microbiota (Oscillibacter ruminantium, Faecalibacterium prausnitzii, and Akkermansia muciniphila, especially O. ruminantium and F. prausnitzii) in Pb-exposed mice markedly enhanced the excretion of Pb and increased the expression of tight junction proteins to alleviate the disruption of gut permeability. Notably, SCFAs were upregulated by colonic microbiota, which allowed the authors to propose that probiotics possess the Pb-against potential by regulating intestinal microbiome (Zhai et al., 2019b). Moreover, Raghuvanshi et al. (2017) developed a transgenic probiotic that markedly prevented LPS-induced impairment in rats exposed to Pb, as well as recovered the normal absorption of essential metal ions. In a previous study, Tian et al. (2012) firstly gave evidence that Lactobacillus plantarum CCFM8661 could bind Pb in vitro, and which also offered remarkable protective effects on hematology and oxidative stress in mice. In a subsequent study, L. plantarum CCFM8661 supplementation effectively reversed Pb-induced innate immune status and reduced the frequency of erythrocyte nuclear abnormalities in peripheral blood of fish (Zhai et al., 2017b). The studies above uniformly indicate that the protective effect of probiotics against Pb toxicity might be related to direct biding of the metal via a rapid and metabolism-independent surface process or co-precipitation with Pb in the gut (Beyenal and Lewandowski, 2004; Halttunen et al., 2007; Tian et al., 2012). In Zhai et al.’s (2019a) follow-up study, it was found that enterohepatic circulation was modulated by probiotics (L. plantarum CCFM8661), which increased bile flow and biliary glutathione output, finally leading to enhanced biliary Pb output and fecal Pb excretion. Furthermore, the expression of target genes in the enterohepatic farnesoid X receptor–fibroblast growth factor (FXR-FGF15) axis was related to this effect, and the use of FXR agonist reversed the effect significantly. In addition, mixed dietary supplements containing probiotics as well as other substances may function as Pb-against material better, which even make a recovery of learning and memory capacities of mice exposed to Pb (Zhai et al., 2018).
Existing findings demonstrate that Pb in gastrointestinal tracts have both direct and indirect effects on the gut. On the one hand, the intestinal epithelium and tight junctions are the targets for Pb toxicity, of which the damage and disruption together with Pb-induced inflammation and immune dysregulation in the local intestine may lead to increased gut permeability to macromolecules. On the other hand, Pb can also cause dysbiosis of intestinal microbiome, which are usually the first “victims” in gut, thereby compromising the function of gut barrier or change the expression of multiple microbial metabolites. Furthermore, Pb-induced alteration in intestinal microbiome can lead to gut permeability impairment, while disrupted gut barrier may in turn have impacts on microbial diversity, construction, and metabolites. Based on the subsequent alterations of both gut permeability and intestinal microbiome induced by Pb, we hypothesis that Pb exposure may cause an increased production of LPS which may be derived from dysbiosis of gut microbiota and damaged gut wall, thereby resulting in endotoxemia, through the impaired gut barrier. In addition, impairment of gut barrier may lead to bacterial translocation into other tissues, which may further induce endotoxemia and infections in the host. Finally, all the turbulent microbial metabolites, inflammatory cytokines, and immunologic factors enter the blood system, then go through the enterohepatic circulation, which may exert their functions on multiple target organs (Figure 1). Numerous studies have documented that LPS plays a vital role in the development of various diseases such as metabolic diseases (Saito et al., 2007), cardiovascular diseases (Neves et al., 2013; Troseid, 2017), liver, and kidney damage (McIntyre et al., 2011; Perea et al., 2017). Disturbed microbial metabolites are also involved in diseases by interacting with host metabolism. Considerable literature have documented quite a few probiotics as effective detoxicant, and a feasible treatment strategy for Pb toxicity. However, most of the studies focused on the general alteration of microbial communities by Pb, not the specific strain. In view of this, the metagenome and metabonome may need additional examinations to explore some key microbes and their functions on gut and other organs. The related mechanisms of Pb toxicity on gut and the further damage to other organs are not fully elucidated (especially Pb-induced alteration of LPS and related microbial metabolites). Moreover, further investigations on efficient mechanisms of probiotics against Pb are needed.
Figure 1. The proposed effects of Pb toxicity on gut, and the critical role of gut barrier and microbiome in Pb toxicity (reproduced from Yuan et al., 2019). Pb entered the gut then changed the microbial homeostasis, including the balance of gut microbe and microbial metabolites such as SCFAs, BA, and/or LPS. Local inflammation and oxidative stress of gut could be induced by Pb generally. Pb could disrupt the gut barrier and increased gut permeability directly or indirectly. Then inflammatory cytokines, immunologic factors, as well as microbial metabolites such as bile acids (BA) and short-chain fatty acids (SCFAs), might enter the enterohepatic circulation via a weak gut barrier to finally induce systematic lesion.
WL wrote the manuscript. HF, IM, and XW revised the manuscript. SZ, SX, and CZ discussed the manuscript. FY designed, revised, and finalized the manuscript. All the authors contributed to the article and approved the submitted version.
This work was supported by the Hunan Province Excellent Youth Fund (2020JJ3053), the Key Research and Development Projects in Hunan Province (2019SK2041), National Natural Science Foundation of China (81773393), and the National Key Research and Development Program of China (2016YFC0900802).
The authors declare that the research was conducted in the absence of any commercial or financial relationships that could be construed as a potential conflict of interest.
The Supplementary Material for this article can be found online at: https://www.frontiersin.org/articles/10.3389/fphys.2021.574913/full#supplementary-material
Supplementary Figure 1 | Pb toxicity on gut barrier. Pb can damage the gut barrier by inducing oxidative stress and decreasing tight junctions such as ZO-1, claudin-1, and occludin.
Supplementary Figure 2 | Probiotics prevent Pb toxicity and improve gut health. Probiotics prevent Pb’s toxicity by uptaking and binding with it directly, or by producing SCFAs, increasing bile flow, upregulating the expression of tight junction, and reversing adverse reactions.
Supplementary Figure 3 | Pb toxicity on ion transportation. At the time of lead exposure, lead enters inside the cell through Ca2+ channel and binds with calmodulin in place of Ca2+, thereby altering the cellular function.
Supplementary Figure 4 | α diversity of gut microbiome affected by Pb.
Adak, A., and Khan, M. R. (2019). An insight into gut microbiota and its functionalities. Cell. Mol. Sci. 76, 473–493. doi: 10.1007/s00018-018-2943-4
Ahamed, M., and Siddiqui, M. K. (2007). Low level lead exposure and oxidative stress: current opinions. Clin. Chim. Acta 383, 57–64. doi: 10.1016/j.cca.2007.04.024
Al Osman, M., Yang, F., and Massey, I. Y. (2019). Exposure routes and health effects of heavy metals on children. Biometals 32, 563–573. doi: 10.1007/s10534-019-00193-5
Aldridge, K. E. (1995). The occurrence, virulence, and antimicrobial resistance of anaerobes in polymicrobial infections. Am. J. Surg. 169(5A Suppl), 2S–7S.
Bae, S., Ulrich, C. M., Neuhouser, M. L., Malysheva, O., Bailey, L. B., Xiao, L., et al. (2014). Plasma choline metabolites and colorectal cancer risk in the Women’s health initiative observational study. Cancer Res. 74, 7442–7452. doi: 10.1158/0008-5472.can-14-1835
Baker, F., Papiska, H., and Campbell, L. L. (1962). Choline fermentation by Desulfovibrio desulfuricans. J. Bacteriol. 84, 973–978. doi: 10.1128/jb.84.5.973-978.1962
Becattini, S., Taur, Y., and Pamer, E. G. (2016). Antibiotic-induced changes in the intestinal microbiota and disease. Trends Mol. Med. 22, 458–478. doi: 10.1016/j.molmed.2016.04.003
Bellinger, D. C. (2016). Lead contamination in flint–an abject failure to protect public health. N. Engl. J. Med. 374, 1101–1103. doi: 10.1056/nejmp1601013
Beyenal, H., and Lewandowski, Z. (2004). Dynamics of lead immobilization in sulfate reducing biofilms. Water Res. 38, 2726–2736. doi: 10.1016/j.watres.2004.03.023
Bhakta, J. N., Munekage, Y., Ohnishi, K., and Jana, B. B. (2012). Isolation and identification of cadmium- and lead-resistant lactic acid bacteria for application as metal removing probiotic. Int. J. Environ. Sci. Technol. 9, 433–440. doi: 10.1007/s13762-012-0049-3
Bisanz, J. E., Enos, M. K., Mwanga, J. R., Changalucha, J., Burton, J. P., Gloor, G. B., et al. (2014). Randomized open-label pilot study of the influence of probiotics and the gut microbiome on toxic metal levels in Tanzanian pregnant women and school children. mBio 5:e01580-14.
Breton, J., Daniel, C., Vignal, C., Body-Malapel, M., Garat, A., Ple, C., et al. (2016). Does oral exposure to cadmium and lead mediate susceptibility to colitis? The dark-and-bright sides of heavy metals in gut ecology. Sci. Rep. 6:19200.
Breton, J., Le Clere, K., Daniel, C., Sauty, M., Nakab, L., Chassat, T., et al. (2013a). Chronic ingestion of cadmium and lead alters the bioavailability of essential and heavy metals, gene expression pathways and genotoxicity in mouse intestine. Arch. Toxicol. 87, 1787–1795. doi: 10.1007/s00204-013-1032-6
Breton, J., Massart, S., Vandamme, P., De Brandt, E., Pot, B., and Foligne, B. (2013b). Ecotoxicology inside the gut: impact of heavy metals on the mouse microbiome. BMC Pharmacol. Toxicol. 14:62.
Burger-van Paassen, N., Vincent, A., Puiman, P. J., van der Sluis, M., Bouma, J., Boehm, G., et al. (2009). The regulation of intestinal mucin MUC2 expression by short-chain fatty acids: implications for epithelial protection. Biochem. J. 420, 211–219. doi: 10.1042/bj20082222
Cavalcante-Silva, L. H., Galvao, J. G., da Silva, J. S., de Sales-Neto, J. M., and Rodrigues-Mascarenhas, S. (2015). Obesity-driven gut microbiota inflammatory pathways to metabolic syndrome. Front. Physiol. 6:341.
Cheng, D., Li, H., Zhou, J., and Wang, S. (2019). Chlorogenic acid relieves lead-induced cognitive impairments and hepato-renal damage via regulating the dysbiosis of the gut microbiota in mice. Food Funct. 10, 681–690. doi: 10.1039/c8fo01755g
Cho, C. H. (2001). Current roles of nitric oxide in gastrointestinal disorders. J. Physiol. Paris 95, 253–256. doi: 10.1016/s0928-4257(01)00034-1
Clark, H. F., Hausladen, D. M., and Brabander, D. J. (2008). Urban gardens: lead exposure, recontamination mechanisms, and implications for remediation design. Environ. Res. 107, 312–319. doi: 10.1016/j.envres.2008.03.003
Clemente, J. C., Ursell, L. K., Parfrey, L. W., and Knight, R. (2012). The impact of the gut microbiota on human health: an integrative view. Cell 148, 1258–1270. doi: 10.1016/j.cell.2012.01.035
Cryan, J. F., and Dinan, T. G. (2012). Mind-altering microorganisms: the impact of the gut microbiota on brain and behaviour. Nat. Rev. Neurosci. 13, 701–712. doi: 10.1038/nrn3346
Dabke, K., Hendrick, G., and Devkota, S. (2019). The gut microbiome and metabolic syndrome. J. Clin. Invest. 129, 4050–4057. doi: 10.1172/jci129194
Daisley, B. A., Monachese, M., Trinder, M., Bisanz, J. E., Chmiel, J. A., and Burton, J. P. (2019). Immobilization of cadmium and lead by Lactobacillus rhamnosus GR-1 mitigates apical-to-basolateral heavy metal translocation in a Caco-2 model of the intestinal epithelium. Gut Microbes 10, 321–333. doi: 10.1080/19490976.2018.1526581
Datko-Williams, L., Wilkie, A., and Richmond-Bryant, J. (2014). Analysis of U.S. soil lead (Pb) studies from 1970 to 2012. Sci. Total Environ. 468-469, 854–863. doi: 10.1016/j.scitotenv.2013.08.089
Eggers, S., Safdar, N., Sethi, A. K., Suen, G., Peppard, P. E., Kates, A. E., et al. (2019). Urinary lead concentration and composition of the adult gut microbiota in a cross-sectional population-based sample. Environ. Int. 133:105122. doi: 10.1016/j.envint.2019.105122
Evens, A., Hryhorczuk, D., Lanphear, B. P., Rankin, K. M., Lewis, D. A., Forst, L., et al. (2015). The impact of low-level lead toxicity on school performance among children in the Chicago Public Schools: a population-based retrospective cohort study. Environ. Health 14:21.
Fackelmann, G., and Sommer, S. (2019). Microplastics and the gut microbiome: how chronically exposed species may suffer from gut dysbiosis. Mar. Pollut. Bull. 143, 193–203. doi: 10.1016/j.marpolbul.2019.04.030
Feng, J., Cavallero, S., Hsiai, T., and Li, R. (2020). Impact of air pollution on intestinal redox lipidome and microbiome. Free Radic. Biol. Med. 151, 99–110. doi: 10.1016/j.freeradbiomed.2019.12.044
Flora, G., Gupta, D., and Tiwari, A. (2012). Toxicity of lead: a review with recent updates. Interdiscip. Toxicol. 5, 47–58. doi: 10.2478/v10102-012-0009-2
Gao, B., Chi, L., Mahbub, R., Bian, X., Tu, P., Ru, H., et al. (2017). Multi-omics reveals that lead exposure disturbs gut microbiome development, key metabolites, and metabolic pathways. Chem. Res. Toxicol. 30, 996–1005.
Gao, D., Mondal, T. K., and Lawrence, D. A. (2007). Lead effects on development and function of bone marrow-derived dendritic cells promote Th2 immune responses. Toxicol. Appl. Pharmacol. 222, 69–79. doi: 10.1016/j.taap.2007.04.001
Gao, R., Gao, Z., Huang, L., and Qin, H. (2017). Gut microbiota and colorectal cancer. Eur. J. Clin. Microbiol. Infect. Dis. 36, 757–769.
Gilbert, S. G., and Weiss, B. (2006). A rationale for lowering the blood lead action level from 10 to 2 microg/dL. Neurotoxicology 27, 693–701. doi: 10.1016/j.neuro.2006.06.008
Giri, S. S., Jun, J. W., Yun, S., Kim, H. J., Kim, S. G., Kang, J. W., et al. (2019). Characterisation of lactic acid bacteria isolated from the gut of Cyprinus carpio that may be effective against lead toxicity. Probiotics Antimicrob. Proteins 11, 65–73. doi: 10.1007/s12602-017-9367-6
Giri, S. S., Yun, S., Jun, J. W., Kim, H. J., Kim, S. G., Kang, J. W., et al. (2018). Therapeutic effect of intestinal autochthonous Lactobacillus reuteri P16 against waterborne lead toxicity in Cyprinus carpio. Front. Immunol. 9:1824.
Halttunen, T., Salminen, S., and Tahvonen, R. (2007). Rapid removal of lead and cadmium from water by specific lactic acid bacteria. Int. J. Food Microbiol. 114, 30–35. doi: 10.1016/j.ijfoodmicro.2006.10.040
He, X., Wu, J., Yuan, L., Lin, F., Yi, J., Li, J., et al. (2017). Lead induces apoptosis in mouse TM3 Leydig cells through the Fas/FasL death receptor pathway. Environ. Toxicol. Pharmacol. 56, 99–105. doi: 10.1016/j.etap.2017.08.034
Hofmann, A. F., and Hagey, L. R. (2014). Key discoveries in bile acid chemistry and biology and their clinical applications: history of the last eight decades. J. Lipid Res. 55, 1553–1595.
Holmes, E., Li, J. V., Athanasiou, T., Ashrafian, H., and Nicholson, J. K. (2011). Understanding the role of gut microbiome-host metabolic signal disruption in health and disease. Trends Microbiol. 19, 349–359. doi: 10.1016/j.tim.2011.05.006
Huang, H., Wang, Y., An, Y., Jiao, W., Xu, Y., Han, Q., et al. (2019). Selenium alleviates oxidative stress and autophagy in lead-treated chicken testes. Theriogenology 131, 146–152. doi: 10.1016/j.theriogenology.2019.03.015
Jin, Y., Wu, S., Zeng, Z., and Fu, Z. (2017). Effects of environmental pollutants on gut microbiota. Environ. Pollut. 222, 1–9. doi: 10.1007/978-981-15-4759-1_1
Jomova, K., and Valko, M. (2011). Advances in metal-induced oxidative stress and human disease. Toxicology 283, 65–87. doi: 10.1016/j.tox.2011.03.001
Jones, B. V., Begley, M., Hill, C., Gahan, C. G., and Marchesi, J. R. (2008). Functional and comparative metagenomic analysis of bile salt hydrolase activity in the human gut microbiome. Proc. Natl. Acad. Sci. U.S.A. 105, 13580–13585. doi: 10.1073/pnas.0804437105
Juturu, V., and Wu, J. C. (2018). Microbial production of bacteriocins: latest research development and applications. Biotechnol. Adv. 36, 2187–2200. doi: 10.1016/j.biotechadv.2018.10.007
Koh, A., De Vadder, F., Kovatcheva-Datchary, P., and Bäckhed, F. J. C. (2016). From dietary fiber to host physiology: short-chain fatty acids as key bacterial metabolites. Cell 165, 1332–1345. doi: 10.1016/j.cell.2016.05.041
Kou, H., Fu, Y., He, Y., Jiang, J., Gao, X., and Zhao, H. (2019). Chronic lead exposure induces histopathological damage, microbiota dysbiosis and immune disorder in the cecum of female Japanese quails (Coturnix japonica). Ecotoxicol. Environ. Saf. 183:109588. doi: 10.1016/j.ecoenv.2019.109588
Lamattina, L., Garcia-Mata, C., Graziano, M., and Pagnussat, G. (2003). Nitric oxide: the versatility of an extensive signal molecule. Annu. Rev. Plant Biol. 54, 109–136. doi: 10.1146/annurev.arplant.54.031902.134752
Lanphear, B. P., Rauch, S., Auinger, P., Allen, R. W., and Hornung, R. W. (2018). Low-level lead exposure and mortality in US adults: a population-based cohort study. Lancet Public Health 3, e177–e184.
Ley, R. E., Peterson, D. A., and Gordon, J. I. (2006). Ecological and evolutionary forces shaping microbial diversity in the human intestine. Cell 124, 837–848. doi: 10.1016/j.cell.2006.02.017
Li, D., Xu, X., Yu, H., and Han, X. (2017). Characterization of Pb2+ biosorption by psychrotrophic strain Pseudomonas sp. I3 isolated from permafrost soil of Mohe wetland in Northeast China. J. Environ. Manage. 196, 8–15. doi: 10.1016/j.jenvman.2017.02.076
Li, T., Zhang, S., Tan, Z., and Dai, Y. (2017). Trend of childhood blood lead levels in cities of China in recent 10 years. Environ. Sci. Pollut. Res. Int. 24, 5824–5830. doi: 10.1007/s11356-016-8335-0
Liew, W. P., and Mohd-Redzwan, S. (2018). Mycotoxin: its impact on gut health and microbiota. Front. Cell Infect. Microbiol. 8:60.
Liu, D. J., Wu, J., Liu, Y. Q., Ouyang, L., and Wang, J. Y. (2014). [Effects of lead exposure on 18 elements in blood and excretions in rats]. Beijing Da Xue Xue Bao Yi Xue Ban 46, 232–236.
Lo Presti, A., Zorzi, F., Del Chierico, F., Altomare, A., Cocca, S., Avola, A., et al. (2019). Fecal and mucosal microbiota profiling in irritable bowel syndrome and inflammatory bowel disease. Front. Microbiol. 10:1655.
Ma, C., Han, M., Heinrich, B., Fu, Q., Zhang, Q., Sandhu, M., et al. (2018). Gut microbiome-mediated bile acid metabolism regulates liver cancer via NKT cells. Science 360:eaan5931. doi: 10.1126/science.aan5931
Mangiola, F., Ianiro, G., Franceschi, F., Fagiuoli, S., Gasbarrini, G., and Gasbarrini, A. (2016). Gut microbiota in autism and mood disorders. World J. Gastroenterol. 22, 361–368. doi: 10.3748/wjg.v22.i1.361
Marchesi, J. R., Adams, D. H., Fava, F., Hermes, G. D., Hirschfield, G. M., Hold, G., et al. (2016). The gut microbiota and host health: a new clinical frontier. Gut 65, 330–339. doi: 10.1136/gutjnl-2015-309990
McIntyre, C. W., Harrison, L. E., Eldehni, M. T., Jefferies, H. J., Szeto, C. C., John, S. G., et al. (2011). Circulating endotoxemia: a novel factor in systemic inflammation and cardiovascular disease in chronic kidney disease. Clin. J. Am. Soc. Nephrol. 6, 133–141. doi: 10.2215/cjn.04610510
McLellan, S. L., Newton, R. J., Vandewalle, J. L., Shanks, O. C., Huse, S. M., Eren, A. M., et al. (2013). Sewage reflects the distribution of human faecal L achnospiraceae. Environ. Microbiol. 15, 2213–2227. doi: 10.1111/1462-2920.12092
Metryka, E., Chibowska, K., Gutowska, I., Falkowska, A., Kupnicka, P., Barczak, K., et al. (2018). Lead (Pb) exposure enhances expression of factors associated with inflammation. Int. J. Mol. Sci. 19:1813. doi: 10.3390/ijms19061813
Mielke, H. W., Anderson, J. C., Berry, K. J., Mielke, P. W., Chaney, R. L., and Leech, M. (1983). Lead concentrations in inner-city soils as a factor in the child lead problem. Am. J. Public Health 73, 1366–1369. doi: 10.2105/ajph.73.12.1366
Mielke, H. W., Blake, B., Burroughs, S., and Hassinger, N. (1984). Urban lead levels in Minneapolis: the case of the Hmong children. Environ. Res. 34, 64–76. doi: 10.1016/0013-9351(84)90076-8
Mitchell, R. G., Spliethoff, H. M., Ribaudo, L. N., Lopp, D. M., Shayler, H. A., Marquez-Bravo, L. G., et al. (2014). Lead (Pb) and other metals in New York City community garden soils: factors influencing contaminant distributions. Environ. Pollut. 187, 162–169. doi: 10.1016/j.envpol.2014.01.007
Monast, C. S., Telesco, S., Li, K., Hayden, K., and Brodmerkel, C. J. G. (2016). Su1217 the role of the microbiome in clinical response to golimumab in ulcerative colitis. Gastroenterology 150:S498.
Mostafa, G. A., El-Shahawi, H. H., and Mokhtar, A. (2009). Blood lead levels in Egyptian children from high and low lead-polluted areas: impact on cognitive function. Acta Neurol. Scand. 120, 30–37. doi: 10.1111/j.1600-0404.2009.01155.x
Navas-Acien, A., Guallar, E., Silbergeld, E. K., and Rothenberg, S. J. (2007). Lead exposure and cardiovascular disease–a systematic review. Environ. Health Perspect. 115, 472–482. doi: 10.1289/ehp.9785
Neves, A. L., Coelho, J., Couto, L., Leite-Moreira, A., and Roncon-Albuquerque, R. Jr. (2013). Metabolic endotoxemia: a molecular link between obesity and cardiovascular risk. J. Mol. Endocrinol. 51, R51–R64.
Ni, J., Wu, G. D., Albenberg, L., and Tomov, V. T. (2017). Gut microbiota and IBD: causation or correlation? Nat. Rev. Gastroenterol. Hepatol. 14, 573–584. doi: 10.1038/nrgastro.2017.88
Nishida, A., Inoue, R., Inatomi, O., Bamba, S., Naito, Y., and Andoh, A. (2018). Gut microbiota in the pathogenesis of inflammatory bowel disease. Clin. J. Gastroenterol. 11, 1–10.
Org, E., Mehrabian, M., and Lusis, A. J. (2015). Unraveling the environmental and genetic interactions in atherosclerosis: central role of the gut microbiota. Atherosclerosis 241, 387–399. doi: 10.1016/j.atherosclerosis.2015.05.035
Park, S. J., Kim, J., Lee, J. S., Rhee, S. K., and Kim, H. (2014). Characterization of the fecal microbiome in different swine groups by high-throughput sequencing. Anaerobe 28, 157–162. doi: 10.1016/j.anaerobe.2014.06.002
Patsiou, D., Del Rio-Cubilledo, C., Catarino, A. I., Summers, S., Mohd Fahmi, A., Boyle, D., et al. (2020). Exposure to Pb-halide perovskite nanoparticles can deliver bioavailable Pb but does not alter endogenous gut microbiota in zebrafish. Sci. Total Environ. 715:136941. doi: 10.1016/j.scitotenv.2020.136941
Perea, L., Coll, M., Sanjurjo, L., Blaya, D., Taghdouini, A. E., Rodrigo-Torres, D., et al. (2017). Pentraxin-3 modulates lipopolysaccharide-induced inflammatory response and attenuates liver injury. Hepatology 66, 953–968. doi: 10.1002/hep.29215
Raghuvanshi, R., Chaudhari, A., and Kumar, G. N. (2017). 2-Ketogluconic acid and pyrroloquinoline quinone secreting probiotic Escherichia coli Nissle 1917 as a dietary strategy against heavy metal induced damage in rats. J. Funct. Foods 37, 541–552. doi: 10.1016/j.jff.2017.08.013
Rashid, A., Bhat, R. A., Qadri, H., Mehmood, M. A., and Shafiq Ur, R. (2019). Environmental and socioeconomic factors induced blood lead in children: an investigation from Kashmir, India. Environ. Monit. Assess. 191:76.
Riaz, M., Mahmood, Z., Shahid, M., Saeed, M. U. I, Tahir, M., Shah, S. A., et al. (2016). Impact of reactive oxygen species on antioxidant capacity of male reproductive system. Int. J. Immunopathol. Pharmacol. 29, 421–425. doi: 10.1177/0394632015608994
Ridlon, J. M., and Bajaj, J. S. (2015). The human gut sterolbiome: bile acid-microbiome endocrine aspects and therapeutics. Acta Pharm. Sin B 5, 99–105. doi: 10.1016/j.apsb.2015.01.006
Ridlon, J. M., Kang, D. J., Hylemon, P. B., and Bajaj, J. S. (2015). Gut microbiota, cirrhosis, and alcohol regulate bile acid metabolism in the gut. Dig. Dis. 33, 338–345. doi: 10.1159/000371678
Sadykov, R., Digel, I., Artmann, A. T., Porst, D., Linder, P., Kayser, P., et al. (2009). Oral lead exposure induces dysbacteriosis in rats. J. Occup. Health 51, 64–73. doi: 10.1539/joh.m8009
Saito, T., Hayashida, H., and Furugen, R. (2007). Comment on: Cani et al. (2007) Metabolic endotoxemia initiates obesity and insulin resistance: diabetes 56:1761-1772. Diabetes 56:e20; author reply e21.
Satcher, D. S. (2000). The surgeon general on the continuing tragedy of childhood lead poisoning. Public Health Rep. 115, 579–580. doi: 10.1093/phr/115.6.579
Schwab, C., Berry, D., Rauch, I., Rennisch, I., Ramesmayer, J., Hainzl, E., et al. (2014). Longitudinal study of murine microbiota activity and interactions with the host during acute inflammation and recovery. ISME J. 8, 1101–1114. doi: 10.1038/ismej.2013.223
Shao, M., and Zhu, Y. (2020). Long-term metal exposure changes gut microbiota of residents surrounding a mining and smelting area. Sci. Rep. 10:4453.
Sharon, G., Garg, N., Debelius, J., Knight, R., Dorrestein, P. C., and Mazmanian, S. K. (2014). Specialized metabolites from the microbiome in health and disease. Cell Metab. 20, 719–730. doi: 10.1016/j.cmet.2014.10.016
Stojanov, S., Berlec, A., and Štrukelj, B. J. M. (2020). The influence of probiotics on the Firmicutes/Bacteroidetes ratio in the treatment of obesity and inflammatory bowel disease. Microorganisms 8:1715. doi: 10.3390/microorganisms8111715
Szolnoki, Z., Farsang, A., and Puskas, I. (2013). Cumulative impacts of human activities on urban garden soils: origin and accumulation of metals. Environ. Pollut. 177, 106–115. doi: 10.1016/j.envpol.2013.02.007
Tamanai-Shacoori, Z., Smida, I., Bousarghin, L., Loreal, O., Meuric, V., Fong, S. B., et al. (2017). Roseburia spp.: a marker of health? Future Microbiol. 12, 157–170.
Tang, W. W., Wang, Z., Levison, B. S., Koeth, R. A., Britt, E. B., Fu, X., et al. (2013). Intestinal microbial metabolism of phosphatidylcholine and cardiovascular risk. N. Engl. J. Med. 368, 1575–1584. doi: 10.1056/nejmoa1109400
Tian, F., Zhai, Q., Zhao, J., Liu, X., Wang, G., Zhang, H., et al. (2012). Lactobacillus plantarum CCFM8661 alleviates lead toxicity in mice. Biol. Trace Elem. Res. 150, 264–271. doi: 10.1007/s12011-012-9462-1
Tomczok, J., Grzybek, H., Sliwa, W., and Panz, B. (1988). Ultrastructural aspects of the small intestinal lead toxicology. Part I: surface ultrastructure of the small intestine mucosa in rats with lead acetate poisoning. Exp. Pathol. 35, 49–55. doi: 10.1016/s0232-1513(88)80122-1
Topcu, A., and Bulat, T. (2010). Removal of cadmium and lead from aqueous solution by Enterococcus faecium strains. J. Food Sci. 75, T13–T17.
Tremaroli, V., and Backhed, F. (2012). Functional interactions between the gut microbiota and host metabolism. Nature 489, 242–249. doi: 10.1038/nature11552
Tripathi, A., Debelius, J., Brenner, D. A., Karin, M., Loomba, R., Schnabl, B., et al. (2018). Publisher Correction: the gut-liver axis and the intersection with the microbiome. Nat. Rev. Gastroenterol. Hepatol. 15:785. doi: 10.1038/s41575-018-0031-8
Troseid, M. (2017). Gut microbiota and acute coronary syndromes: ready for use in the emergency room? Eur. Heart J. 38, 825–827. doi: 10.1093/eurheartj/ehx005
Tsoi, M. F., Cheung, C. L., Cheung, T. T., and Cheung, B. M. (2016). Continual decrease in blood lead level in Americans: United States national health nutrition and examination survey 1999-2014. Am. J. Med. 129, 1213–1218. doi: 10.1016/j.amjmed.2016.05.042
Turnbaugh, P. J., Hamady, M., Yatsunenko, T., Cantarel, B. L., Duncan, A., Ley, R. E., et al. (2009). A core gut microbiome in obese and lean twins. Nature 457, 480–484. doi: 10.1038/nature07540
Velmurugan, G., Ramprasath, T., Gilles, M., Swaminathan, K., and Ramasamy, S. (2017). Gut microbiota, endocrine-disrupting chemicals, and the diabetes epidemic. Trends Endocrinol. Metab. 28, 612–625. doi: 10.1016/j.tem.2017.05.001
Ver Heul, A., Planer, J., and Kau, A. L. (2019). The human microbiota and asthma. Clin. Rev. Allergy Immunol. 57, 350–363. doi: 10.1007/s12016-018-8719-7
Wahlström, A., Sayin, S. I., Marschall, H.-U., and Bäckhed, F. (2016). Intestinal crosstalk between bile acids and microbiota and its impact on host metabolism. Cell Metab. 24, 41–50. doi: 10.1016/j.cmet.2016.05.005
Wang, L., Tang, L., Feng, Y., Zhao, S., Han, M., Zhang, C., et al. (2020). A purified membrane protein from Akkermansia muciniphila or the pasteurised bacterium blunts colitis associated tumourigenesis by modulation of CD8+ T cells in mice. Gut. 69, 1988–1997.
Wang, R. X., Lee, J. S., Campbell, E. L., and Colgan, S. P. (2020). Microbiota-derived butyrate dynamically regulates intestinal homeostasis through regulation of actin-associated protein synaptopodin. Proc. Natl. Acad. Sci. U.S.A. 117, 11648–11657. doi: 10.1073/pnas.1917597117
Watson, W. A., Litovitz, T. L., Rodgers, G. C. Jr., Klein-Schwartz, W., Reid, N., Youniss, J., et al. (2005). 2004 annual report of the American association of poison control centers toxic exposure surveillance system. Am. J. Emerg. Med. 23, 589–666. doi: 10.1016/j.ajem.2005.05.001
Wong, J. M., de Souza, R., Kendall, C. W., Emam, A., and Jenkins, D. J. (2006). Colonic health: fermentation and short chain fatty acids. J. Clin. Gastroenterol. 40, 235–243.
Wu, H., Esteve, E., Tremaroli, V., Khan, M. T., Caesar, R., Manneras-Holm, L., et al. (2017). Metformin alters the gut microbiome of individuals with treatment-naive type 2 diabetes, contributing to the therapeutic effects of the drug. Nat. Med. 23, 850–858. doi: 10.1038/nm.4345
Wu, J., Wen, X. W., Faulk, C., Boehnke, K., Zhang, H., Dolinoy, D. C., et al. (2016). Perinatal lead exposure alters gut microbiota composition and results in sex-specific bodyweight increases in adult mice. Toxicol. Sci. 151, 324–333. doi: 10.1093/toxsci/kfw046
Xia, J., Jin, C., Pan, Z., Sun, L., Fu, Z., and Jin, Y. (2018a). Chronic exposure to low concentrations of lead induces metabolic disorder and dysbiosis of the gut microbiota in mice. Sci. Total Environ. 63, 439–448. doi: 10.1016/j.scitotenv.2018.03.053
Xia, J., Lu, L., Jin, C., Wang, S., Zhou, J., Ni, Y., et al. (2018b). Effects of short term lead exposure on gut microbiota and hepatic metabolism in adult zebrafish. Comp. Biochem. Physiol. C Toxicol. Pharmacol. 209, 1–8. doi: 10.1016/j.cbpc.2018.03.007
Yi, Y. J., Lim, J. M., Gu, S., Lee, W. K., Oh, E., Lee, S. M., et al. (2017). Potential use of lactic acid bacteria Leuconostoc mesenteroides as a probiotic for the removal of Pb(II) toxicity. J. Microbiol. 55, 296–303. doi: 10.1007/s12275-017-6642-x
Yu, L. X., and Schwabe, R. F. (2017). The gut microbiome and liver cancer: mechanisms and clinical translation. Nat. Rev. Gastroenterol. Hepatol. 14, 527–539. doi: 10.1038/nrgastro.2017.72
Yuan, X., Pan, Z., Jin, C., Ni, Y., Fu, Z., and Jin, Y. (2019). Gut microbiota: an underestimated and unintended recipient for pesticide-induced toxicity. Chemosphere 227, 425–434. doi: 10.1016/j.chemosphere.2019.04.088
Zhai, Q., Li, T., Yu, L., Xiao, Y., Feng, S., Wu, J., et al. (2017a). Effects of subchronic oral toxic metal exposure on the intestinal microbiota of mice. Sci. Bull. 62, 831–840. doi: 10.1016/j.scib.2017.01.031
Zhai, Q., Liu, Y., Wang, C., Qu, D., Zhao, J., Zhang, H., et al. (2019a). Lactobacillus plantarum CCFM8661 modulates bile acid enterohepatic circulation and increases lead excretion in mice. Food Funct. 10, 1455–1464. doi: 10.1039/c8fo02554a
Zhai, Q., Qu, D., Feng, S., Yu, Y., Yu, L., Tian, F., et al. (2019b). Oral supplementation of lead-intolerant intestinal microbes protects against lead (Pb) toxicity in mice. Front. Microbiol. 10:3161.
Zhai, Q., Wang, H., Tian, F., Zhao, J., Zhang, H., and Chen, W. (2017b). Dietary Lactobacillus plantarum supplementation decreases tissue lead accumulation and alleviates lead toxicity in Nile tilapia (Oreochromis niloticus). Aquacult. Res. 48, 5094–5103. doi: 10.1111/are.13326
Zhai, Q., Wang, J., Cen, S., Zhao, J., Zhang, H., Tian, F., et al. (2019c). Modulation of the gut microbiota by a galactooligosaccharide protects against heavy metal lead accumulation in mice. Food Funct. 10, 3768–3781. doi: 10.1039/c9fo00587k
Zhai, Q., Yang, L., Zhao, J., Zhang, H., Tian, F., and Chen, W. (2018). Protective effects of dietary supplements containing probiotics, micronutrients, and plant extracts against lead toxicity in mice. Front. Microbiol. 9:2134.
Zhang, Z., Zhang, X.-X., Wu, B., Yin, J., Yu, Y., and Yang, L. (2016). Comprehensive insights into microcystin-LR effects on hepatic lipid metabolism using cross-omics technologies. J. Hazardous Mater. 315, 126–134. doi: 10.1016/j.jhazmat.2016.05.011
Keywords: intestinal microbiota, lead, microbial metabolites, gut, toxicity
Citation: Liu W, Feng H, Zheng S, Xu S, Massey IY, Zhang C, Wang X and Yang F (2021) Pb Toxicity on Gut Physiology and Microbiota. Front. Physiol. 12:574913. doi: 10.3389/fphys.2021.574913
Received: 24 June 2020; Accepted: 27 January 2021;
Published: 04 March 2021.
Edited by:
Stephen J. Pandol, Cedars-Sinai Medical Center, United StatesReviewed by:
Kazi Mirajul Hoque, University of Maryland School of Medicine, Baltimore, United StatesCopyright © 2021 Liu, Feng, Zheng, Xu, Massey, Zhang, Wang and Yang. This is an open-access article distributed under the terms of the Creative Commons Attribution License (CC BY). The use, distribution or reproduction in other forums is permitted, provided the original author(s) and the copyright owner(s) are credited and that the original publication in this journal is cited, in accordance with accepted academic practice. No use, distribution or reproduction is permitted which does not comply with these terms.
*Correspondence: Fei Yang, eWFuZ2ZlaWxvbmdAMTI2LmNvbQ==
Disclaimer: All claims expressed in this article are solely those of the authors and do not necessarily represent those of their affiliated organizations, or those of the publisher, the editors and the reviewers. Any product that may be evaluated in this article or claim that may be made by its manufacturer is not guaranteed or endorsed by the publisher.
Research integrity at Frontiers
Learn more about the work of our research integrity team to safeguard the quality of each article we publish.