- 1Key Laboratory of Bio-Resources and Eco-Environment, Ministry of Education, College of Life Sciences, Sichuan University, Chengdu, China
- 2Key Laboratory of Birth Defects and Related Diseases of Women and Children, Ministry of Education, West China Second University Hospital, Sichuan University, Chengdu, China
It is well-established that anterior pituitary contains multiple endocrine cell populations, and each of them can secrete one/two hormone(s) to regulate vital physiological processes of vertebrates. However, the gene expression profiles of each pituitary cell population remains poorly characterized in most vertebrate groups. Here we analyzed the transcriptome of each cell population in adult chicken anterior pituitaries using single-cell RNA sequencing technology. The results showed that: (1) four out of five known endocrine cell clusters have been identified and designated as the lactotrophs, thyrotrophs, corticotrophs, and gonadotrophs, respectively. Somatotrophs were not analyzed in the current study. Each cell cluster can express at least one known endocrine hormone, and novel marker genes (e.g., CD24 and HSPB1 in lactotrophs, NPBWR2 and NDRG1 in corticotrophs; DIO2 and SOUL in thyrotrophs, C5H11ORF96 and HPGDS in gonadotrophs) are identified. Interestingly, gonadotrophs were shown to abundantly express five peptide hormones: FSH, LH, GRP, CART and RLN3; (2) four non-endocrine/secretory cell types, including endothelial cells (expressing IGFBP7 and CFD) and folliculo-stellate cells (FS-cells, expressing S100A6 and S100A10), were identified in chicken anterior pituitaries. Among them, FS-cells can express many growth factors, peptides (e.g., WNT5A, HBEGF, Activins, VEGFC, NPY, and BMP4), and progenitor/stem cell-associated genes (e.g., Notch signaling components, CDH1), implying that the FS-cell cluster may act as a paracrine/autocrine signaling center and enrich pituitary progenitor/stem cells; (3) sexually dimorphic expression of many genes were identified in most cell clusters, including gonadotrophs and lactotrophs. Taken together, our data provides a bird’s-eye view on the diverse aspects of anterior pituitaries, including cell composition, heterogeneity, cell-to-cell communication, and gene expression profiles, which facilitates our comprehensive understanding of vertebrate pituitary biology.
Introduction
It has been well-established that in vertebrates, the anterior pituitary (Pars distalis) contains multiple distinct hormone-secreting cell populations, including somatotrophs, lactotrophs, gonadotrophs, corticotrophs, and thyrotrophs. Each cell type is believed to secrete one or two endocrine hormones to regulate many vital physiological processes (Scanes, 2014; Kaiser and Ho, 2016). In mammals, pituitary somatotrophs secrete growth hormone (GH); lactotrophs secrete prolactin (PRL); gonadotrophs produce two gonadotropins, namely follicle-stimulating hormone (FSH) and luteinizing hormone (LH), both of which share a common glycoprotein α-subunit (CGA) and a hormone-specific β-subunit (FSHB and LHB); corticotrophs produce adrenocorticotropin (ACTH), and thyrotrophs secrete TSH, consisting of an α-subunit (CGA, common for FSH and LH) and a hormone-specific β-subunit (TSHB) (Zhu et al., 2007; Edwards and Raetzman, 2018). These pituitary hormones play vital roles in the regulation of growth, development, metabolism, reproduction, and stress (Edwards and Raetzman, 2018). In addition to the endocrine cell population, several non-endocrine/secretory cell populations have also been identified in anterior pituitaries, including progenitor/stem cells, endothelial cells, and folliculo-stellate cells (FS-cells) (Denef, 2008; Gleiberman et al., 2008). Progenitor/stem cells are reported to mainly localize in the periluminal area between anterior lobe and intermediate lobe and sparsely in the parenchyma of mouse anterior pituitaries. These cells can express progenitor/stem-cell markers, e.g., sex-determining region Y-box 2 (SOX2), and have the capacity to differentiate into endocrine cells in vitro, thus they are proposed to play critical roles in maintaining organ homeostasis (Fauquier et al., 2008). FS-cells can express S100 proteins specifically and are reported to play important roles in the anterior pituitary, such as supportive effects on endocrine cells, stem cell function, ion transport, phagocytic and catalytic activity (Traverso et al., 1999; Inoue et al., 2002). There is also evidence that FS-cells can secrete growth factors, such as vascular endothelial growth factor (VEGF), fibroblast growth factor 2 (FGF2), leptin (LEP) and Annexin 1 (ANXA1), suggesting a role in paracrine/autocrine communication within the anterior pituitary (Traverso et al., 1999; Denef, 2008).
In mammalian anterior pituitaries, both endocrine cells and FS-cells are likely originated from the oral ectoderm during embryogenesis, although a recent study reported some pituitary endocrine cells are derived from an endodermal origins, at least in fish (Fabian et al., 2020). Under the induction of paracrine signals dorsally from ventral diencephalon (e.g., bone morphogenetic protein 4, BMP4; fibroblast growth factors, FGFs; WNT5A) and ventrally from the oral ectoderm and mesenchyme (e.g., Sonic hedgehog, Shh; bone morphogenetic protein 2, BMP2), the oral ectoderm forms a rudimentary Rathke’s pouch and expresses a specific set of transcription factors spatio-temporally (e.g., POU1F1, GATA2, NR5A1, and TBX19), which further induces cell differentiation to form distinct endocrine cell lineages (Treier et al., 1998; Zhu et al., 2007; Kelberman et al., 2009; Rizzoti, 2015) in mammals. For instance, in mouse embryonic pituitaries, POU1F1 (Pit-1) expression causes the emergence of three Pit-1 cell lineages (somatotrophs, lactotrophs and thyrotrophs) and GATA2 and NR5A1 expression are necessary for specification of the gonadotrophs (Kelberman et al., 2009). After birth, these endocrine cell populations further expand and differentiate under the influence of hypothalamic and peripheral signals, thus forming a functional endocrine gland in vertebrates (Edwards and Raetzman, 2018).
Although pituitary cell populations and functions have been extensively studied in vertebrates, the gene expression profiles of each pituitary cell population remains poorly understood. In recent years, the transcriptome of each pituitary cell population in vertebrate pituitaries by single-cell RNA sequencing (scRNA-Seq) was available for rat (Fletcher et al., 2019), mouse (Cheung et al., 2018; Ho et al., 2020), human (Zhang et al., 2020), zebrafish (Fabian et al., 2020) and medaka (Siddique et al., 2020). However, such study is lacking in other non-mammalian vertebrates including birds. This limitation, undoubtedly, prevents our better understanding of vertebrate pituitary biology. Like mammalian anterior pituitaries, avian anterior pituitaries contain five hormone-secreting cells, including somatotrophs, lactotrophs, thyrotrophs, gonadotrophs, and corticotrophs. These endocrine cell populations secrete GH, PRL, TSH, LH (and FSH), and ACTH, respectively, to regulate avian growth, metabolism, reproduction, and stress (Scanes, 2014). As in mammals, the secretion and/or expression of each pituitary hormone has been reported to be controlled by signals from the hypothalamus and peripheral tissues in birds (Scanes, 2014). GH secretion is controlled by hypothalamic GH-releasing hormone (GHRH), thyrotropin-releasing hormone (TRH) and somatostatin (SST) (Harvey et al., 2014; Meng et al., 2014; Bu et al., 2016). LH secretion is controlled by hypothalamic gonadotropin-releasing hormone 1 (GnRH1). PRL secretion is controlled by hypothalamic vasoactive intestine polypeptide (VIP), TRH, arginine vasotocin (AVT) and negatively, by dopamine (DA) (Bu et al., 2016; Lv et al., 2018). ACTH secretion is controlled by corticotropin-releasing hormone (CRH), AVT and glucocorticoids (CORT), and TSH secretion is controlled by CRH, SST, glucagon-like peptide (GCGL), and thyroid hormones (T4/T3) (Huang et al., 2014; Bu et al., 2019; Wu et al., 2019).
Despite the similarity in pituitary cell populations, functions, and their regulatory mechanisms across vertebrates, we and others have reported functional differences in anterior pituitary between birds and mammals. For instance, chicken anterior pituitaries can produce two novel peptide hormones: gastrin-releasing peptide (GRP) and cocaine-and amphetamine-regulate transcript (CART, also named CARTPT) (Cai et al., 2015; Mo et al., 2017, 2019). The functional conservation and difference of anterior pituitary between mammals and birds promotes us to further investigate the gene expression profiles of each pituitary cell types in birds. Therefore, using chicken as an animal model, our present study aims to: (1) identify the major pituitary cell clusters; (2) investigate the gene expression profiles of each pituitary cell population. Our data, for the first time, revealed the gene expression profiles of each anterior pituitary cell population in an avian model.
Materials and Methods
Ethics Statement
Adult chickens (Lohmann layer) used in this study were purchased from local commercial companies. Single cell suspensions were prepared from anterior pituitaries of sexually mature chickens (male: N = 6, female: N = 6) at 1-year-old stage. All animal experiments were conducted in accordance with the Guidelines for Experimental Animals issued by the Ministry of Science and Technology of People’s Republic of China. All animal experimental protocols were approved by the Animal Ethics Committee of the College of Life Sciences, Sichuan University (Chengdu, China).
Chemicals, Antibodies, and Primers
All chemicals were purchased from Sigma (Sigma-Aldrich, St. Louis, MO, United States). Rabbit polyclonal anti-NPY antibody (ab10980) was purchased from Abcam (Cambridge, MA, United States) and Donkey anti-rabbit IgG (H + L) cross adsorbed secondary antibody (Dylight 488 conjugate) was purchased from ThermoFisher Scientific (Waltham, MA, United States). All primers were synthesized by Beijing Genome Institute (BGI, Shenzhen, China) and listed in Supplementary Table 1.
Single-Cell Dissociation and 10x Genomics Chromium Library Construction of Adult Chicken Anterior Pituitary
Pituitary cell dispersion was carried out as described in our previous studies (Meng et al., 2014). Briefly, anterior pituitaries were separated from adult male and female chickens (1-year-old). Then, six male/female pituitaries were pooled and washed in 1 × Hank’s balanced salt solution (HBSS) thrice. These pituitaries were treated by 0.25% trypsin solution for 30 min at 37°C. Dissociated cells were filtered through a 40-μm cell strainer and subject to centrifugation at 500 g for 3 min. Then the cell pellet was re-suspended in a solution containing 0.1% bovine serum albumin (BSA) in 1 × PBS solution (calcium- and magnesium-free). Cell viability and numbers were estimated by trypan blue staining. Then, pituitary cells were loaded onto the Chromium system (10x Genomics 3′ GEX V2) to produce cDNA libraries according to the Chromium Single Cell 3′ Reagents Kits V2 User guide (10x Genomics Inc., Pleasanton, CA, United States). The cDNA libraries were quantified by Agilent Tapestation System (Agilent Technologies, Santa Clara, CA, United States) and sequenced by BGI500 (BGI, Shenzhen, China).
scRNA-Seq Analysis and Data Visualization
For RNA-seq analysis of single pituitary cells, the raw base call (BCL) files generated from the BGI500 system were demultiplexed into paired-end, gzip-compressed FASTQ files using the Cell Ranger software v1.3.1 provided by 10x Genomics (Supplementary Figure 1). Then, both pairs of FASTQ files were provided as input and converted to gene-count matrix using the alevin software v1.4.0 (Srivastava et al., 2019), which is a fast end-to-end pipeline to process droplet-based single-cell RNA sequencing data, cell barcode detection, read mapping, unique molecular identifier (UMI) deduplication and gene count estimation. Quality control, read alignment (with reference to the GRCg6a transcriptome downloaded from the Ensembl database (Ensembl release 1031) and raw count quantification for each cell was achieved using the alevin pipeline with default command line options.
Using tximport packages v1.18.0 (Soneson et al., 2015), the counts matrix and associated metadata processed through Alevin were then imported to Seurat package v4.0 (Butler et al., 2018; Stuart et al., 2019; Hao et al., 2020) in R to clusters and further analyzed, using default parameters unless specified otherwise. We first filtered out genes detected in fewer than five cells and those cells containing more than 2.5% mitochondria reads or less than 200 unique genes detected. Doublets that may affect clustering analysis were detected and eliminated using the R package DoubletFinder v.2.0.3 with default command line options (Assuming 15% doublet formation rate) (McGinnis et al., 2019). After this initial quality control (QC) filtering process, 9, 919 cells from female pituitaries and 6, 733 cells from male pituitaries were used for further analysis. After filtering and normalization, independent pituitary samples from the female and male chickens were integrated by Canonical Correlation Analysis, identifying common sources of variation to align the datasets. The top 2000 highly variable genes were identified and used as input for dimensionality reduction via canonical correlation analysis. Data dimensionality reduction was performed using a principal component analysis (PCA), and the first 30 principal components were used in the downstream analyses.
According to Seurat ‘FindClusters’ function (using 30 PCs given the highly variable genes and a resolution of 0.5), all cells were partitioned into 16 distinct clusters, and visualized using Uniform Manifold Approximation and Projection (UMAP) (Becht et al., 2018). According to Seurat ‘FindAllMarkers’ function (min.pct = 0.25, log2fc.threshold = 0.25, p_val_adj < 0.001) using the MAST test (Finak et al., 2015), unique cluster-specific marker genes were identified. The selected marker genes for each cell cluster with high log2fc.threshold are listed in Table 1. Cell types were determined using a combination of marker genes identified from the literature (described in section “Cell Populations Identified in Adult Chicken Anterior Pituitary”). Clusters that appeared to correspond to the same cell types were merged. Significantly upregulated genes (signature genes) between female and male samples were found using a MAST test implemented in Seurat by the ‘FindMarkers’ function (min.pct = 0.25, only.pos = TRUE, log2fc.threshold = 0.5, p_val_adj < 0.001). The violin plots, feature plot, and dotplot were generated using Seurat software. Cell communication analysis was based on the network analysis and pattern recognition approaches provided by CellChat (version 1.0.0) R package (Jin et al., 2021). Standard workflow was used to predict major signaling inputs and outputs of cells and how these cells and signals coordinate for functions.
Immunofluorescence Staining
Anterior pituitaries collected from 3-week-old male chicks or adult male chickens were fixed in 4% paraformaldehyde (PFA) at 4°C overnight, embedded in paraffin wax, and then sectioned at a thickness of 8 μm. Deparaffinization and immunofluorescence staining were then performed as described in our recent studies (Meng et al., 2014; Mo et al., 2017). Briefly, pituitary sections were deparaffinized in xylene, and rehydrated. Antigen retrieval was performed by heating in citrate buffer (pH = 6) at 95°C for 10 min. After blocking by 5% BSA in PBS for 1 h at room temperature, the sections were incubated with the primary rabbit polyclonal anti-NPY antibody (1:500) at 4°C overnight. After PBS wash, the sections were incubated with fluorochrome-conjugated secondary antibodies (1:300) for 1 h at room temperature. Finally, the pituitary sections were counterstained with DAPI and observed under a fluorescence microscope (Nikon ECLIPSE Ti, Nikon Instruments Inc., United States). Pituitary sections incubated with pre-immune rabbit serum, instead of anti-NPY antibody, were used as a negative control.
Quantitative Real-Time RT-PCR (qPCR) and Transcriptomic Data Analysis
Total RNA was extracted from chicken pituitaries using RNAzol (Molecular Research Center, Cincinnati, OH, United States) according to the manufacturer’s instructions and reversely transcribed using M-MLV reverse transcriptase (Takara Bio, Shiga, Japan). In brief, oligodeoxythymide (0.5 μg) and total RNA (2 μg) were mixed in a total volume of 5 μL, incubated at 70°C for 10 min, and cooled at 4°C for 2 min. Then, the first strand buffer, 0.5 mM each deoxynucleotide triphosphate and 100 U Moloney murine leukemia virus (MMLV) reverse transcriptase were added into the reaction mix in a total volume of 10 μL. Reverse transcription (RT) was performed at 42°C for 90 min. RT samples were then used to examine the mRNA expression of target genes in chicken anterior pituitaries by quantitative real-time PCR (qPCR) assay on the CFX96 Real-time PCR Detection System (Bio-Rad Richmond, CA, United States), as described in our previous study (Zhang et al., 2017, 2018).
RNA-Seq data obtained in our laboratory was used to evaluate the mRNA expression level of RLN3 gene (accession no: MT263682) in granulosa cells of growing ovarian follicles (6 mm follicle, F5 preovulatory follicle, and F1 preovulatory follicles) from egg-laying hens (Zhu et al., 2019). In brief, using Tophat v2.0.12 as a mapping tool (Trapnell et al., 2012), clean reads were filtered from raw reads and mapped to the reference genome of Gallus gallus2. HTSeq v0.6.1 was used to count the reads mapped to each gene. The FPKM (Fragments per kilobase of transcript per million mapped reads) values were used as an indicator of the relative abundance of RLN3 at different stages.
Data Analysis
The relative mRNA levels of selected target genes were first calculated as the ratios to that of β-actin and then expressed as the fold difference compared to the male/female anterior pituitaries. The data was analyzed by Student t-test (between two groups) using GraphPad Prism 7 (GraphPad Software, San Diego CA, United States).
Results and Discussion
Cell Populations Identified in Adult Chicken Anterior Pituitary
To investigate the gene expression profiles of chicken pituitary cell populations, we dispersed the cells of anterior pituitaries from adult female (egg-laying stage) and male chickens, and performed single-cell RNA-seq (scRNA-Seq) analysis using the 10x Genomics system (Macosko et al., 2015). A total of 16, 652 pituitary cells passed the quality control check: 9, 919 cells for the female pituitary pool (six pituitaries), and 6, 733 cells for the male pituitary pool (six pituitaries).
We performed a canonical correlation analysis on gene expression matrix and integrated datasets from male and female pituitaries to classify major cell clusters. As a result, we identified eight major transcriptionally distinct cell clusters. According to the known marker genes of pituitary endocrine cell lineages, we designated four endocrine cell clusters, as lactotrophs (Lac, abundantly expressing PRL), gonadotrophs (Gona, abundantly expressing LHB, FSHB, GRP, and CART), thyrotrophs (Thy, abundantly expressing TSHB), and corticotrophs (Cort, abundantly expressing POMC) (Figure 1A). These endocrine cell clusters could also be identified in the anterior pituitaries of both sexes, and no sex specific cluster was found (Figure 1B).
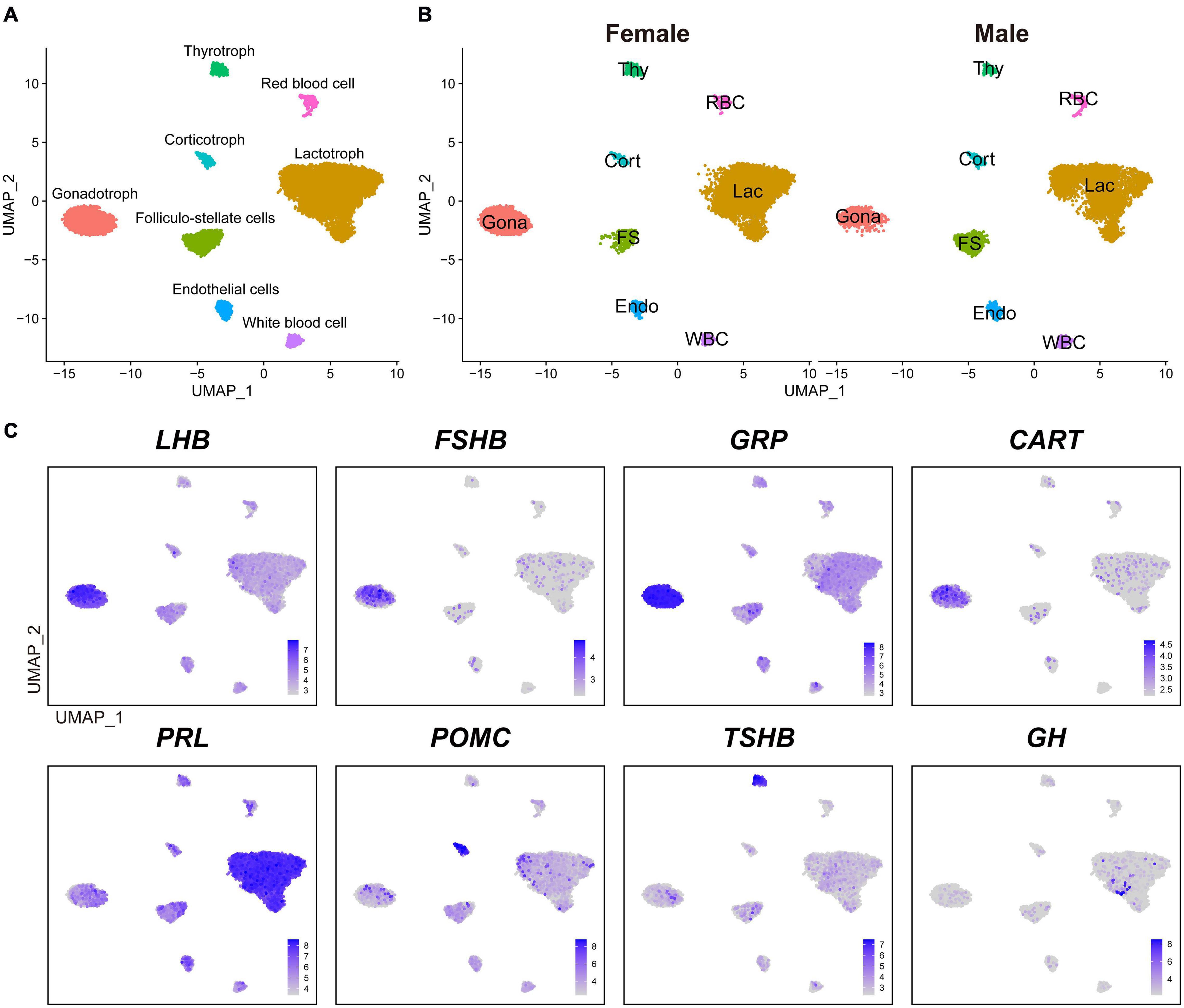
Figure 1. The major cell clusters identified in adult chicken anterior pituitaries. (A) Uniform Manifold Approximation and Projection (UMAP) map showing the identified eight pituitary cell types based on the transcriptomes of 16, 652 cells. Cells are colored by Seurat clustering and annotated by cell types (each point represents a single cell). Gona, gonadotrophs; Lac, lactotrophs; Cort, corticotrophs; Thy, thyrotrophs; FS, folliculo-stellate cells; Endo, endothelial cells; WBC, white blood cells; RBC, red blood cells; UMAP, Uniform Manifold Approximation and Projection. (B) UMAP maps of the eight cell types identified in female (9, 919 cells) and male (6, 733 cells) adult chicken anterior pituitaries. (C) UMAP maps showing the abundant expression of pituitary hormone(s) in distinct cell clusters (LHB, FSHB, GRP, and CART are abundantly expressed in Gona-cluster, PRL in Lac-cluster, POMC in Cort-cluster, TSHB in Thy-cluster, and GH). Color bar indicates natural log transformed normalized expression.
In vertebrates, the anterior pituitary contains five classical pituitary endocrine cell populations, including lactotrophs, somatotrophs, gonadotrophs, thyrotrophs, and corticotrophs/melanotropes (Cheung et al., 2018; Fletcher et al., 2019; Ho et al., 2020; Zhang et al., 2020). Except somatotrophs, our scRNA-seq data successfully reveal four major cell clusters in adult chicken anterior pituitaries (Figure 1). Since immunostaining of GH in the chicken pituitary reveal an abundance of somatotroph cells in the caudal lobe (Bu et al., 2016), the lack of somatotroph population is likely due to loss of GH-expressing cells during cell dissociation and collection with sub-optimal cytocentrifugation speed in this study. The low number of GH-positive cells located in lactotrophs did not form a separate cluster during analysis (Figure 1C), which is likely a technical shortcomings of our study and need further investigations.
Excluding somatotrophs, our scRNA-seq data suggest that lactotrophs are likely the largest cell population in adult chicken pituitaries of both sexes (Figure 1B), which is consistent with the observation in adult rodents as revealed by scRNA-seq analyses (Cheung et al., 2018; Fletcher et al., 2019). This result is also consistent with our recent finding that PRL-immunoreactive (PRL-ir) cells are the mainly population in adult female chicken pituitaries (Mo et al., 2017). Previous reports also support the idea that plasma PRL is at an extremely high level in chickens and other birds at various adult reproductive stages (e.g., egg-laying, incubation) (Harvey et al., 1979; Scanes et al., 1979; Sharp et al., 1979). The large proportion of lactotrophs and high plasma PRL levels tend to support the important roles of lactotrophs in the reproduction of both male and female chickens, and possibly in other birds as well (Sharp et al., 1998; Sreekumar and Sharp, 1998).
Besides the four major endocrine cell clusters mentioned above, four non-endocrine/non-secretory cell clusters, including folliculo-stellate cell (FS), endothelial cell (Endo), white blood cell (WBC), and red blood cell (RBC), were also identified in chicken anterior pituitaries (Figure 1), similar to recent findings in rodent anterior pituitaries (Cheung et al., 2018; Fletcher et al., 2019). FS-cluster is known for the expression of marker genes encoding S100 proteins in mammals (e.g., S100B, S100A6, S100A1, and S100A11) (Denef, 2008; Fletcher et al., 2019) and chickens (e.g., S100A6, S100A10, S100A1, and S100A11) (Supplementary Figure 2). Endo-cluster expresses marker genes, such as insulin like growth factor binding protein 7 (IGFBP7) and complement factor D (CFD). RBC-cluster expresses marker genes, such as hemoglobin beta, subunit A (HBBA) and hemoglobin alpha, subunit D (HBAD); WBC-cluster expresses marker genes such as joining chain of multimeric IgA and IgM (JCHAIN), cysteine rich protein 1 (CRIP1) and lysosomal thiol reductase (IFI30) (Table 1). These findings, taken together, suggest that the major cell populations of the anterior pituitary from birds and mammals share common markers.
Marker Genes Identified in Adult Chicken Anterior Pituitary
In this study, we identified 33-138 marker genes for each pituitary cell cluster (Table 1, Supplementary Data 1) (Note: Table 1 shows the selected marker genes for each cell cluster, while all marker genes and their full names for eight cell clusters are listed in Supplementary Data 1). The expression profiles of selected marker genes for each cluster are presented as a dot plot in Figure 2. In the Gona-cluster, we identified 93 marker genes, such as relaxin 3 (RLN3), C5H11ORF96, hematopoietic prostaglandin-D synthase (HPGDS, catalyzing the conversion of prostaglandin H2 to prostaglandin D2), complexin 1 (CPLX1), GnRH receptor (GnRHR, also named GnRHR2 in birds), amyloid beta precursor protein (APP), and novel transcripts (e.g., ENSGALG00000045362, ENSGALG00000001805, and ENSGALG00000053991) (Table 1 and Figures 2, 3A).
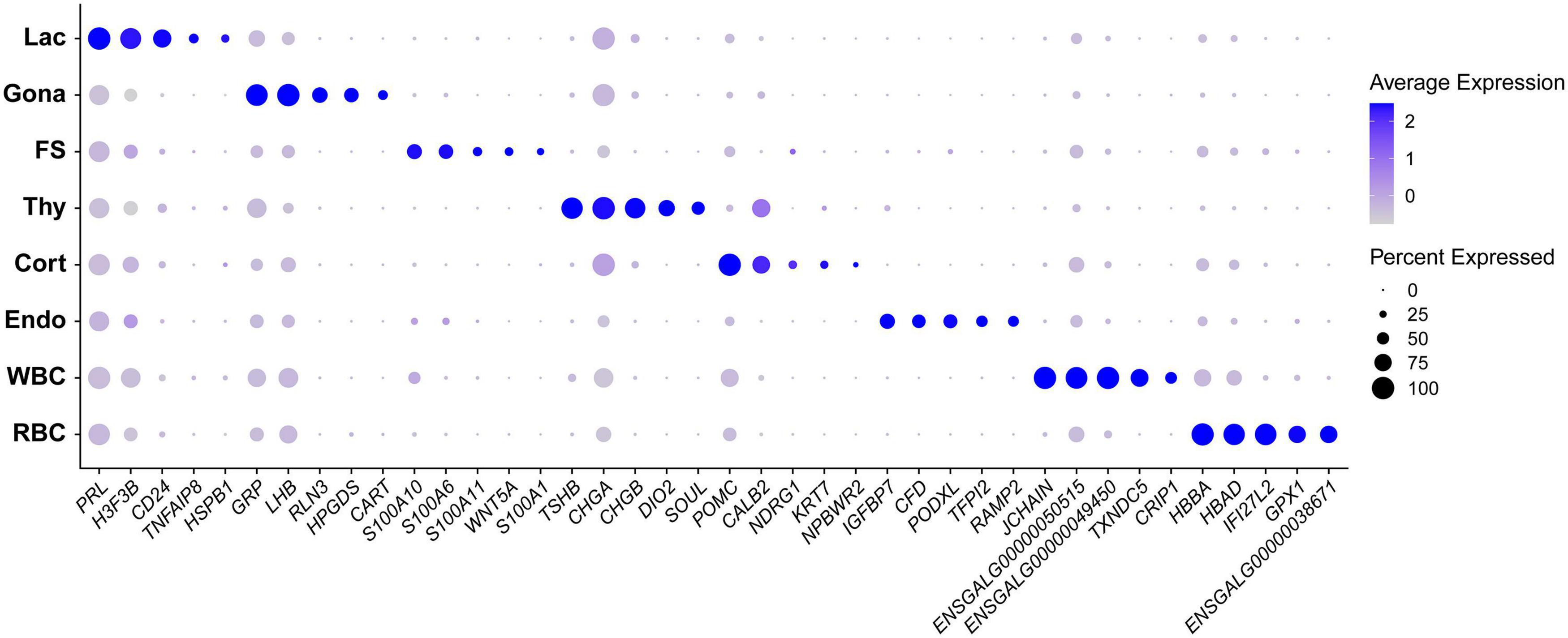
Figure 2. Dot plot showing the expression pattern and level of some established marker genes (shown in columns) for each major cell type (shown in rows) in chicken anterior pituitaries. Color intensities show the expression level of the indicated gene.
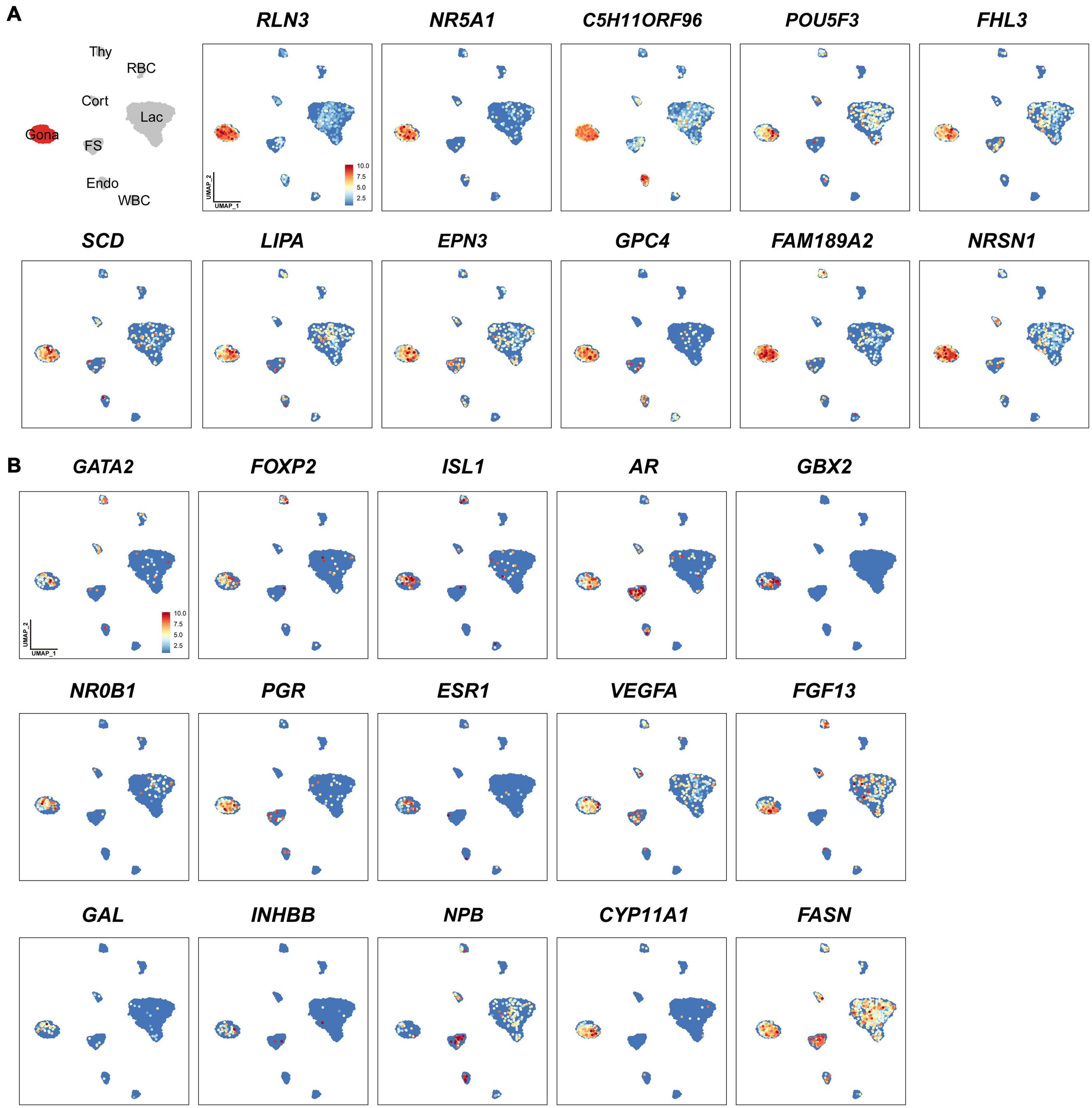
Figure 3. Uniform Manifold Approximation and Projection (UMAP) maps showing the expression of some genes expressed in gonadotrophs of chicken anterior pituitaries. (A) The selected marker genes in pituitary gonadotrophs. (B) UMAP maps showing the expression of some non-marker genes, including transcription factors (GATA2, FOXP2, ISL1, AR, GBX2, NR0B1, PGR, ESR1), growth factor/peptide (VEGFA, FGF13, GAL, activin B (encoded by INHBB), and NPB), and enzymes (CYP11A1, FASN) in pituitary gonadotrophs. Color bar indicates the relative expression level of each marker gene from the lowest (light blue dots) to the highest expression level (dark red dots).
In lactotroph cluster, we identified 138 marker genes, such as H3F3B, CD24, TNFAIP8, HSPB1, SIX3, POU1F1, NEUROD1, EGR1, and ANGPTL4 (Table 1 and Figures 2, 4A). In thyrotroph cluster, 69 marker genes, such as CGA, chromogranin A (CHGA), chromogranin B (CHGB), iodothyronine deiodinase 2 (DIO2, an enzyme for conversion of T4 to T3), SOUL, CALB2, RGS2, and ENSGALG000000052026 were identified (Table 1 and Figures 2, 4B). In corticotroph cluster, 33 marker genes, such as CALB2, NDRG1, NPBWR2, KRT7, C12ORF75, and dickkopf 3 (DKK3, an WNT signaling pathway inhibitor) were identified (Table 1 and Figures 2, 4C).
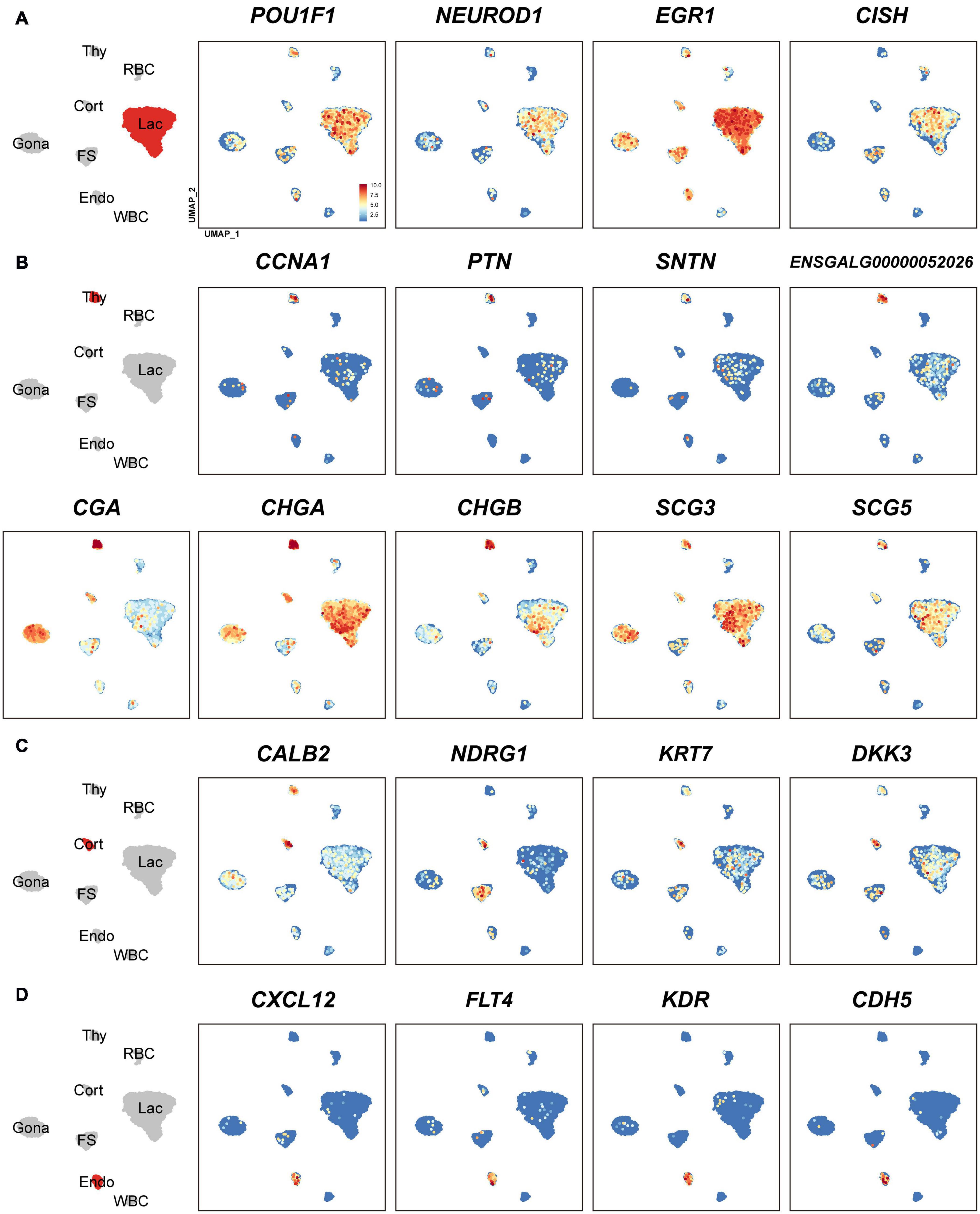
Figure 4. Uniform Manifold Approximation and Projection (UMAP) maps showing the expression of some marker genes in lactotrophs (A: Lac), thyrotrophs (B: Thy), corticotrophs (C: Cort), and endothelial cells (D: Endo). Color bar indicates the relative expression level of each marker gene from the lowest expression (light blue dots) to highest expression (dark red dots).
In FS-cell cluster, 74 marker genes were identified, and some of them including S100A1, S100A6, S100A10, S100A11, and ANXA5, have been reported in fish, birds, and mammals (Table 1 and Figure 2, Supplementary Figure 2) (Van Nassauw et al., 1987; Denef, 2008; Anbalagan et al., 2018; Fletcher et al., 2019). In endothelial cell population, we identified 83 marker genes, such as RAMP2, CDH5, CXCL12, VEGF receptors (FLT4, KDR), GPR1, RARRES2, ACVRL1, and NOTCH1 (Table 1 and Figures 2, 4D). In blood cells, 137 marker genes were also identified in WBC- and RBC-clusters and listed in the Table 1 and Supplementary Data 1.
Genes like LHB, FSHB, GnRHR and transcriptional factors (e.g., NR5A1) have been reported in rodent/avian pituitaries (Cheung et al., 2018; Fletcher et al., 2019), and could be identified in the Gona-cluster of both chickens and mammals, indicating their conserved roles in gonadotroph, such as lineage specification and gonadotropin expression/secretion (Edwards and Raetzman, 2018). Likewise, marker genes for lactotrophs (e.g., EGR1), thyrotrophs (e.g., TSHB, CGA, CHGA, CHGB, and DIO2), corticotrophs (POMC), FS-cells (S100 proteins and ANXAs) and endothelial cells have been identified in chickens or mammals (Fletcher et al., 2019). Further studies on these genes will help to reveal their specific roles in chicken anterior pituitaries.
Through the transcriptomic analysis of single pituitary cell, we identified many non-marker genes of interest in each cell type. As an example, we found that additional transcriptional factors, such as GATA2, AR, FOXP2 (French et al., 2007), NR0B1 (also called DAX-1, critical for the development and function of adrenal and hypothalamus-pituitary-gonadal axis) (Ikeda et al., 1996; Achermann et al., 2001), GBX2 (a homeobox gene involved in the hindbrain development during gastrulation) (Waters and Lewandoski, 2006) and ISL1 (crucial for pancreatic cell lineage development) (Larsson, 1998), are co-expressed in the Gona-cluster (Figure 3B). The question whether all these genes are involved in the control of gonadotroph cell differentiation and functions would be an interesting topic worthy of further investigations. Similarly, several non-marker genes were identified in other cell types (Figure 3B), therefore, future extensive studies are required to address their functions in these pituitary cell populations.
Endocrine Hormone Genes Are Expressed by Multiple Pituitary Cell Types
Although PRL is abundantly expressed in lactotroph cluster, we found that it is also weakly expressed in nearly all other endocrine cell clusters identified. Similarly, LHB, GRP, and CART highly expressed in gonadotrophs are weakly expressed in the other pituitary cell clusters; TSHB and POMC mRNAs are abundantly expressed in thyrotrophs and corticotrophs, respectively, and weakly expressed in some other cell clusters (Figure 1 and Supplementary Figure 3). CGA encoding α-subunit common for TSH, FSH and LH is abundantly expressed in thyrotroph and gonadotroph clusters and weakly expressed in other cell types (Figure 4B). Like endocrine hormone genes, other genes encoding potential bioactive peptides, e.g., CHGA, CHGB, SCG3, and SCG5 are expressed abundantly in thyrotrophs and moderately in other endocrine cell clusters (Figure 4B).
It is generally accepted that each endocrine cell population can express one or two hormones in the anterior pituitary of vertebrates (Zhu et al., 2007; Kelberman et al., 2009; Scanes, 2014). Recent scRNA research in Japanese medaka confirm a ‘one cell type, one hormone’ division of labor, in which major hormones are produced by a single, dedicated cell type (Siddique et al., 2020). This is different from another scRNA report on the rat pituitary, in which cells are often responsible for producing and releasing multiple hormones (Ho et al., 2020). At least for gonadotrophs, the situation is quite different between fish and other tetrapods. In all fish, LH and FSH are produced by different cells, whereas in most tetrapods they seem to be produced in the same cell (Siddique et al., 2020). There are lines of evidence also showing that one pituitary cell type expressing multiple hormone mRNAs exists in normal rodent pituitaries (Childs et al., 2000; Seuntjens et al., 2002; Villalobos et al., 2004; Childs et al., 2020). In chicken pituitaries, we found that lactotrophs predominantly express PRL, however, they can also weakly express LHB, GRP, GH, POMC, and TSHB. Similarly, gonadotrophs could also weakly express PRL, TSHB, GH, and POMC (Figure 1 and Supplementary Figure 3). Presumably, the weak expression of multiple hormone genes in one endocrine cell type may be correlated with the weak expression of multiple transcription factors in that population, e.g., POU1F1, NR5A1 (SF1), GATA2, and EGR1 (Supplementary Figure 4), which have been proposed, either alone or in combination, to be expressed in one cell lineage specifically, and thus control its hormone expression (Edwards and Raetzman, 2018). For instance, POU1F1, which has been proposed to be exclusively expressed in three Pit-1 lineages (lactotrophs, thyrotrophs, and somatotrophs) and responsible for their differentiation and hormone expression (Edwards and Raetzman, 2018), is also weakly expressed in other cell lineages, including gonadotrophs and corticotrophs (Figure 4A). Future study on the protein expression and secretion of multiple hormones from these cells will help to define the nature of these so-called ‘multi-hormone’ expressing cells (Ho et al., 2020).
Gonadotrophs Produce Five Hormones: LH, FSH, GRP, CART, and RLN3 in Chickens
Since we and others reported that LHB, GRP, CART, and FSHB are abundantly expressed in chicken anterior pituitaries (Proudman et al., 1999; Mo et al., 2017; Mo et al., 2019), we visualized the co-expression of the four genes using Seurat software. As shown in Figures 5A,B, FSHB, GRP, and CART are co-expressed in gonadotrophs expressing LHB (> 99% overlapping signals). It has long been believed that in birds, two gonadotropins, FSH and LH, are mainly expressed in separate sub-populations of gonadotrophs detected by immuno-histochemistry (Proudman et al., 1999; Puebla-Osorio et al., 2002). However, we found that FSHB mRNA is localized in gonadotrophs expressing LHB nearly exclusively. Our study clearly indicates that, as in mammals (Gregory et al., 2005) and frog (Pinelli et al., 1996), FSH and LH can be co-expressed in the same gonadotroph cells in chickens (Figure 5A). This ambiguity in chicken may be caused by the characteristics of the immunological reagents used in previous studies, which need further elucidation.
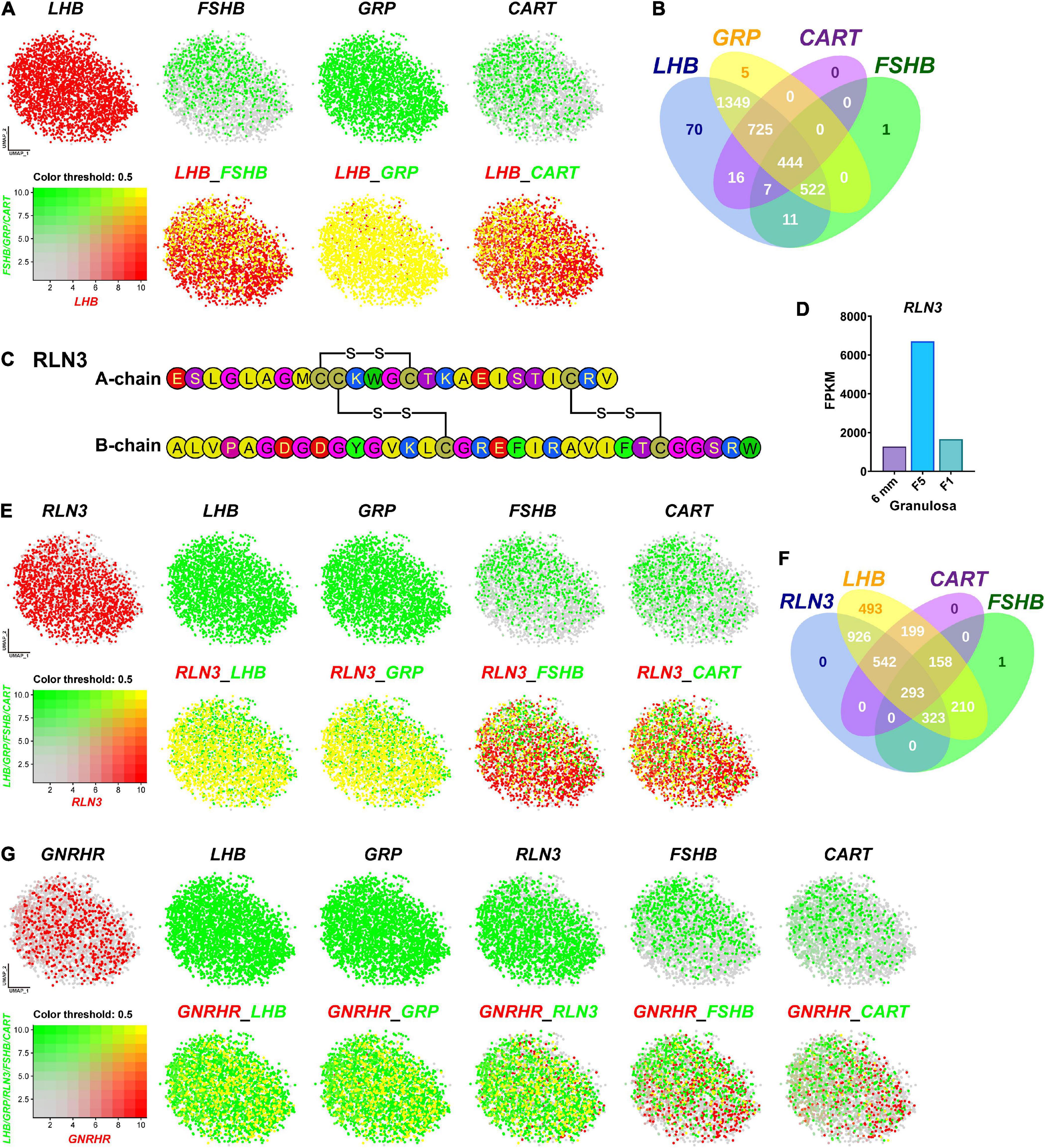
Figure 5. Visualization of the genes co-expressed in gonadotrophs. (A) Co-expression of LHB and other hormone gene (FSHB/GRP/CART) in gonadotrophs. Gene expression levels are color coded. Red/Green indicates the high expression level and gray indicates the low expression level, as shown in the bottom left corner. Cells abundantly co-expressing LHB and FSHB/GRP/CART are shown in yellow. (B) Venn diagram showing the numbers of LHB, GRP, CART and FSHB-positive cells in Gona-cluster. (C) Amino acid sequence of the A- and B-chains of chicken RLN3 (accession no.: MT263682). The three disulfide bonds formed by six cysteine residues (red) are highlighted by red lines. (D) RNA-Seq analysis showing the abundant expression of RLN3 in granulosa cells of growing follicles (6 mm, F5, F1) in adult chicken ovaries. The expression levels of the RLN3 transcripts were expressed in FPKM values. (E) Co-expression of RLN3 with LHB/GRP/FSHB/CART in gonadotrophs. (F) Venn diagram showing the numbers of RLN3-, LHB-, CART-, and FSHB-positive cells in the gonadotroph cluster. (G) Co-expression of GNRHR (also called GnRHR2 in birds) and LHB/GRP/RLN3/FSHB/CART in gonadotrophs.
Like FSHB, GRP and CART are abundantly expressed in gonadotrophs expressing LHB. This is also supported by our recent findings in chickens, in which GRP-ir mainly co-localizes with LH-ir cells, and CART peptide secretion is strongly induced by chicken GnRH (Mo et al., 2017, 2019). Our finding on the co-expression of these hormones in gonadotrophs, for the first time, provides convincing evidence on our hypothesis that gonadotrophs can produce the four peptide hormones, i.e., LH, FSH, GRP and CART, in chickens and all of them may be actively involved in the regulation of male/female chicken reproduction (and possibly other physiological processes) (Mo et al., 2019). Like our findings in chickens, CART peptide is reported to be abundantly expressed in rat gonadotrophs (Kuriyama et al., 2004). These findings suggest that in addition to LH and FSH, other peptide hormone (s) (e.g., CART) can be produced by gonadotrophs in avian/mammalian species (Kappeler et al., 2006; Mo et al., 2019).
Besides the four hormones mentioned above, Relaxin 3 (RLN3) is abundantly and specifically expressed in the gonadotroph of female (and not male) chickens. RLN3 is a hetero-dimeric peptide (consisting of A-chain and B-chain) and shares structural similarity to insulin (INS) in vertebrates (Halls et al., 2015). In this study, we found that chicken RLN3 (accession no: MT263682) is a novel marker abundantly expressed in Gona-cluster (Figure 3), which is consisting of A-chain (25 a.a.) and B-chain (33 a.a.) and has three pairs of cysteines involved in disulfide bridges (Figure 5C). Our transcriptomic data demonstrated the extremely abundant expression of RLN3 in granulosa cells of growing follicles (6 mm, F5, and F1) in adult chicken ovaries (Figure 5D; Zhu et al., 2019). We further visualized its co-expression with LHB, GRP, FSHB, and CART in Gona-cluster. As shown in Figures 5E,F, nearly all cells expressing RLN3 overlap with cells expressing LHB and GRP. In contrast, RLN3 partially co-localize with FSHB and CART (∼30%/40%) in gonadotrophs. This finding led us to hypothesize that RLN3 is most likely a ‘novel endocrine hormone’ secreted by pituitary gonadotrophs and ovaries, which may be actively involved in the regulation of female reproduction. In accordance with our hypothesis, pituitary RLN3 mRNA levels have also been reported to change significantly between brooding and egg-laying chickens and ducks (Shen et al., 2016; Ye et al., 2019). Future studies on RLN3 actions on the anterior pituitary, ovary, oviduct, and other tissues will help to define the actions of this ‘novel hormone’ in birds.
Since GnRHR mediates the effects of GnRH signaling on gonadotroph secretion, we further visualized the co-localization of GnRHR with LHB, GRP, RLN3, FSHB, or CART, respectively. As shown in Figure 5G, GnRHR-expressing cells overlap with the majority of LHB/GRP/RLN3-cells, however, GnRHR only co-localizes with FSHB/CART partially. Notably, the expression pattern we reported here is similar to that observed in mature female medaka (Hodne et al., 2019) and in mice during embryogenesis (Wen et al., 2010), where GnRHR is expressed exclusively in LH cells and not in FSH cells. These results suggest that FSH and CART may be more dependent on other regulation factors than GnRH in chicken, which need additional investigations.
Receptors Expressed in Pituitary Cell Clusters
In vertebrates, the receptors found in anterior pituitary can receive signals from the hypothalamus and peripheral tissues, such as the gonads, thyroid, and adrenal gland, thus forming multiple closed-loop feedback axes, such as the hypothalamus-pituitary-gonad (HPG), hypothalamus-pituitary-thyroid (HPT), and hypothalamus-pituitary-adrenal (HPA) axes (Vazquez-Borrego et al., 2018). Since receptors can mediate the actions of hypothalamic hormones, peripheral signals, and local pituitary-derived factors, we examined the expression of receptor genes in each pituitary cell type (Figure 6).
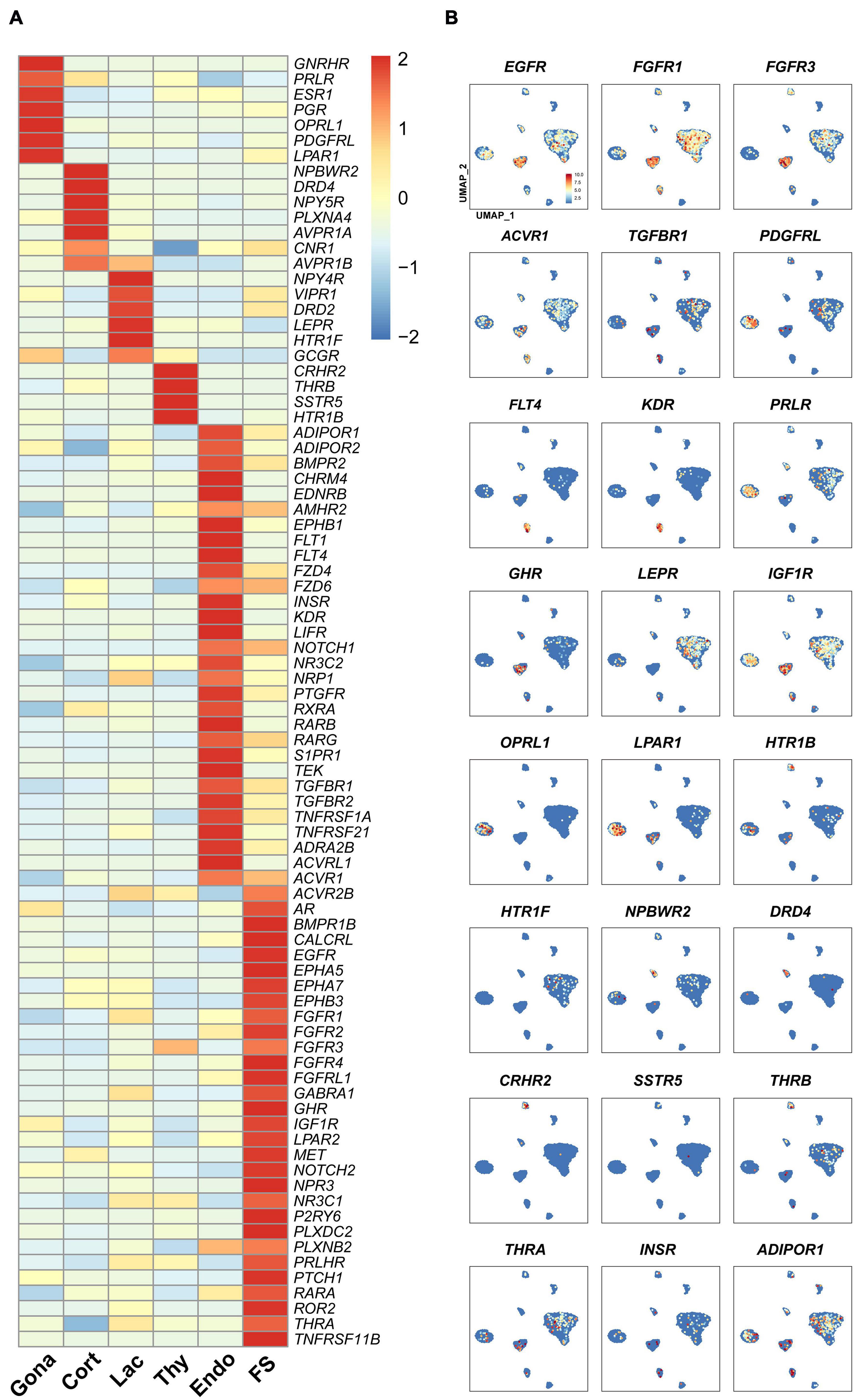
Figure 6. The expression of genes encoding the receptors for endocrine/paracrine/autocrine signals in chicken pituitary cell clusters. (A) Heat-map showing the expression of receptor genes in different pituitary cell types. The expression level of each gene was normalized to the z-score and color-coded. (B) mRNA signals for some receptor genes shown in (A) were projected on the UMAP plots.
In gonadotrophs, the receptors for GnRH (GnRHR), nociceptin (OPRL1), gonadal steroids (androgen receptor, AR; progesterone receptor, PGR; estradiol receptor α, ESR1), PRL (PRLR), SHH (PTCH1), PDGFs (PDGFRL), and prostaglandin E2 (PTGER4) (Kwok et al., 2012) etc., were found to be highly expressed (Figure 6), suggesting that gonadotroph function is likely controlled by hypothalamic input (e.g., GnRH) and peripherals signals from the gonads and extra-gonadal tissues, such as the adrenal gland, pancreas, gastrointestinal tract, liver and adipose tissue (Scanes, 2014).
In corticotrophs, the receptors for NPW (NPBWR2), dopamine (DRD4), AVT (AVPR1A, AVPR1B), NPY (NPY5R), and cannabinoid (CN1R) etc., were also found to be highly expressed (Figure 6). In lactotrophs, the receptors for hypothalamic factors [e.g., VIP (VIPR1), AVT (AVPR1B), dopamine (DRD2)], peripheral/local signals [e.g., leptin (LEPR), serotonin (HTR1F), and pancreatic polypeptide PP (NPY4R) (Gao et al., 2017)], were found to be expressed (Figure 6). In thyrotroph, the receptors for CRH (CRHR2), TH (THRB), SST (SSTR5), and serotonin (HTR1B) etc., were also found to be highly expressed (Figure 6).
Like endocrine cells, endothelial cells also express many receptor genes (Figure 6), including the receptors for VEGFC (FLT4), VEGFA (KDR and FLT1), BMP, FGFs, TGFBs, RA, NOTCH1 ligands, prostaglandin F (PTGFR) (Kwok et al., 2012), chemerin (GPR1), as well as endothelin (EDNRB) critical for endothelial cell differentiation and functions (Liu et al., 2019), suggesting that these hormones may regulate Endothelial cells functions.
In this study, we found that many receptors are expressed in endocrine/non-endocrine cell clusters (Figure 6). All these findings not only support previous pioneering findings regarding the hormonal regulation of avian pituitary gonadotrophs (e.g., GnRH and gonadal steroids) (Hall et al., 1986; Sharp et al., 1987; Millar, 2005), lactotrophs (VIP, dopamine, CORT, and NPW) (Macnamee et al., 1986; el Halawani et al., 1990; Al Kahtane et al., 2003; Fu and Porter, 2004; Bu et al., 2016; Scanes, 2016; Lv et al., 2018), thyrotroph (CRH, T3, GCGL and SST) (De Groef et al., 2003; De Groef et al., 2007; Huang et al., 2014), and corticotroph functions (AVT, CRH, and CORT) (Mikhailova et al., 2007; Selvam et al., 2013; Wu et al., 2019), but also hints that many novel signals from the hypothalamus and peripheral tissues (e.g., NPW, serotonin, SST, insulin, PP, and PRLH) could regulate pituitary cell functions, in which nearly all functional axes (e.g., HPG, HPT, and HPA) are inter-connected. Meanwhile, our findings also strongly suggest that peripheral signals from the adipose tissue (adiponectin), gastrointestinal tract (serotonin), and pancreas (GCG, INS, SST, and PP) are likely involved in the regulation of avian pituitary functions. Taken together, avian pituitary functions are coordinately regulated by the hypothalamic inputs, peripheral signals, and local signals derived from endocrine cells (e.g., GH and PRL) or non-endocrine cells (e.g., WNT5A, HBEGF and activin derived from FS-cells).
FS-Cell Is a Paracrine/Autocrine Signaling Center
To investigate the cell-cell communications among these cell subsets, we analyzed the expression levels of ligand-receptor interacting pairs within six pituitary cell types using CellChat (Jin et al., 2021). CellChat analysis detected 166 significant ligand-receptor pairs among the six cell groups, which were further categorized into 35 signaling pathways, including TGFβ, BMP, ACTIVIN, FGF, VEGF, CDH, IGF, NOTCH, SEMA4, and MIF pathways (Figure 7A). FS-cells were identified as one of the dominant communication “hubs”, which secretes and receives signals via 47 and 42 ligand-receptor pairs, respectively (Figures 7A,B). Using pattern recognition analysis, we further investigated the detailed pattern in the outgoing signaling (levels of ligands) and incoming signaling (levels of receptors) across these pathways (Figure 7C). Lactotrophs dominantly drives PRL, ANGPTL, and MK signaling, while endothelial cells drive outgoing CXCL, TGFβ, CDH5, COLLAGEN, FN1, and APP signaling. At the incoming end of signaling, gonadotrophs cells respond to ACTIVIN, NRG, CDH and NOTCH signaling; Lactotrophs and thyrotrophs cells respond to FGF, IGF, PTN, and NCAM signaling, and corticotrophs cells respond to NCAM, CADM, PTN, and MK signaling. It also revealed that FS-cells coordinate outgoing signals and incoming signals for BMP, ACTIVIN, NT (Neurotrophin), CDH, OCLN, and SEMA4 pathways (Figure 7C). This finding supports the existence of an ultra-short communication between pituitary cell populations (Schwartz, 2000; Le Tissier et al., 2012). This idea is supported by in vitro studies in turkeys, in which PRL can decrease LH and PRL expression and secretion in cultured pituitary cells (You et al., 1995). The reciprocal regulation or auto-regulation of endocrine cell functions adds a further level of complexity to the regulatory network of anterior pituitaries.
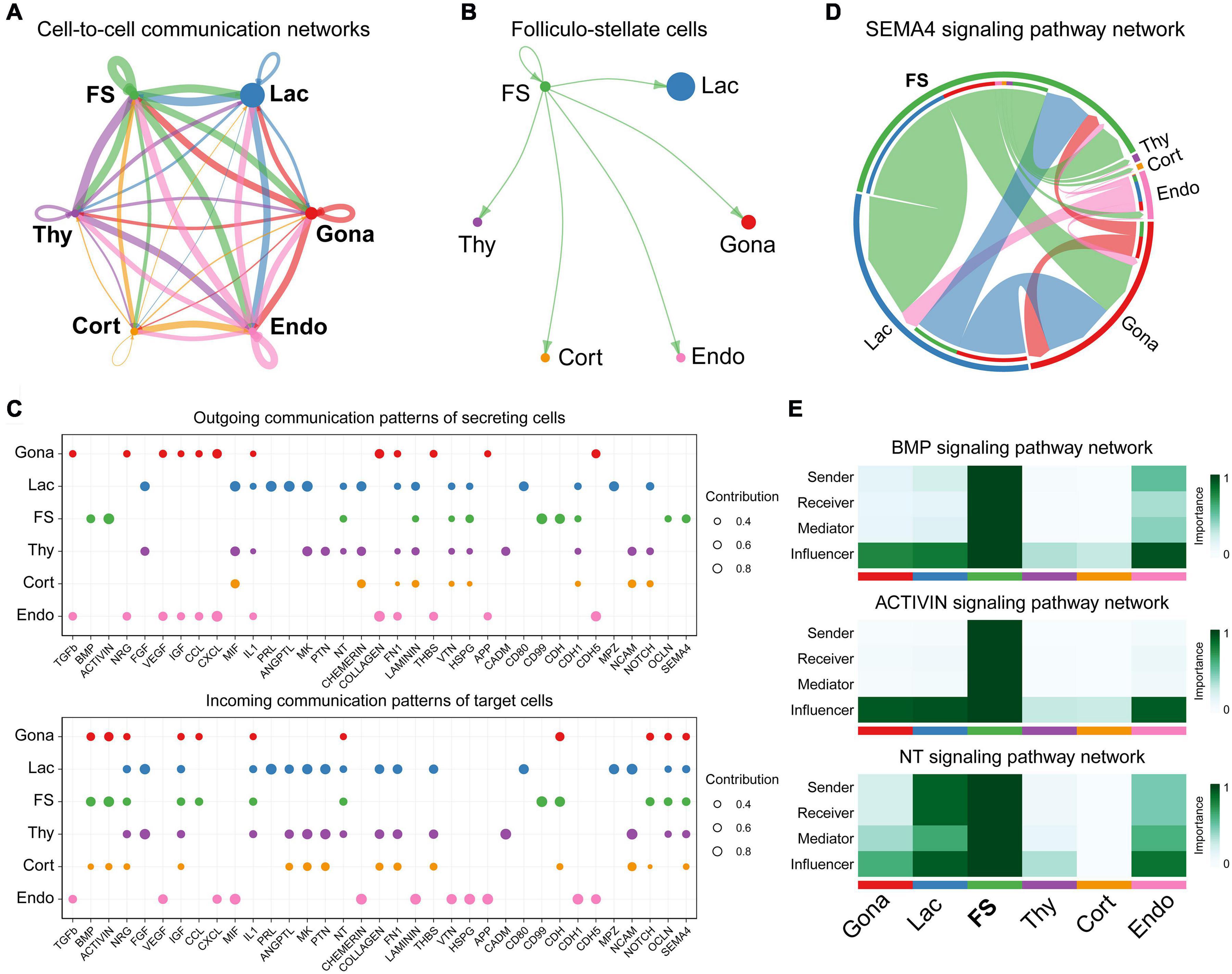
Figure 7. Cell-to-cell communication exploration of six chicken pituitary cell types. (A) Network view of cell-to-cell communications within and across six pituitary cell types. The circle sizes are proportional to the number of cells in each cell group, and edges are in proportion to the counts of ligand-receptor interaction pairs. (B) The inferred FS-cells signaling networks. (C) Dot plots showing the alteration in outgoing (ligand) or incoming (receptor) signaling pathways in pituitary cell types. The dot size is proportional to the contribution score, which is calculated from pattern recognition analysis. Higher contribution score suggests that the signaling pathway is more enriched in the corresponding cell subset. (D) The inferred SEMA4 signaling network. The circle segments represent the six main intercellular communication cell types with different colors. The edge width is proportional to the communication score between interacting cell clusters. (E) Heatmap shows the relative importance of each cell group based on the computed four network centrality measures of BMP, ACTIVIN, and NT signaling network, respectively.
Network centrality analysis of the SEMA4 signaling pathway network identified that FS-cell population is the most prominent source for SEMA4 ligands acting onto gonadotrophs, lactotrophs, and thyrotrophs cells (Figure 7D). Interestingly, CellChat also predicted that FS-cell population significantly contributes to dominated BMP and ACTIVIN signal production in the pituitary, and FS-cells are the primary NTF3 ligand source, which mainly acts onto lactotrophs and endothelial cell populations, in which NTRK3 was highly expressed (Figure 7E).
Interestingly, we also found a list of genes encoding growth factors and peptides abundantly expressed in this FS-cell cluster by heatmap analysis (Figure 8). These genes include semaphorin 3B (SEMA3B), SEMA4B, BMP4, INHBA (producing activin A), WNT5A, heparin-binding EGF-like growth factor (HBEGF), aphiregulin (AREG) (Wang et al., 2007), vascular endothelial growth factor C (VEGFC), transforming growth factor β2 (TGFB2), TGFB3, and neuropeptide Y (NPY) (Gao et al., 2017), suggesting that unlike other pituitary cell types (Figure 8), FS-cells can produce a number of growth factors and peptides.
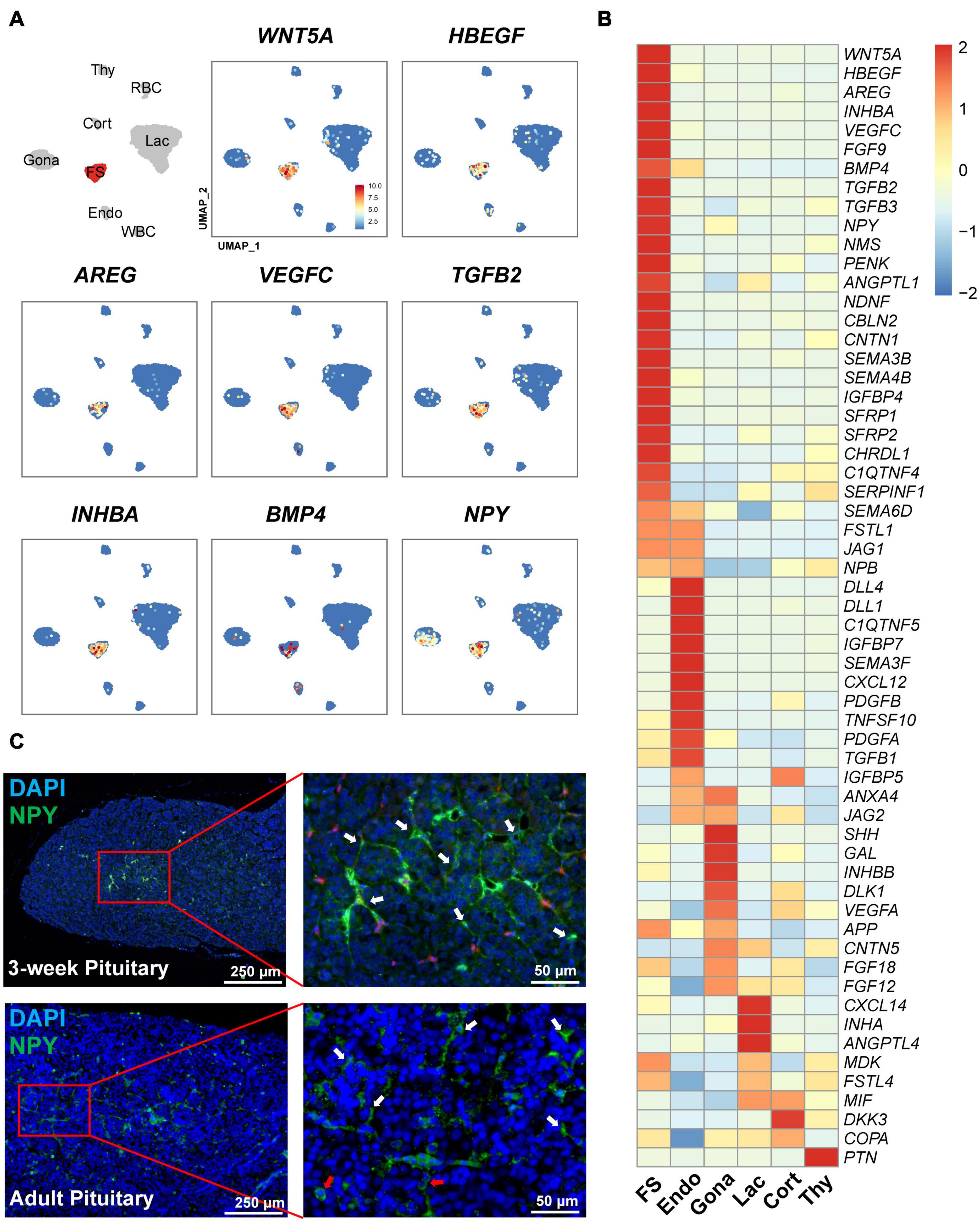
Figure 8. Expression of the genes encoding growth factors and peptides in FS-cells and other cell clusters. (A) UMAP maps showing the abundant/specific expression of some genes encoding growth factors and peptides (WNT5A, HBEGF, AREG, VEGFC, TGFβ2, activin A (encoded by INHBA), BMP4, and NPY). Dark red and light blue indicate high and low expression levels, respectively. (B) Heat-map showing the mRNA levels of the genes encoding growth factors and peptides in FS-cells and other cell types. The expression level of each gene was normalized to the z-score and color-coded. (C) Validation of neuropeptide Y (NPY) expression in FS-cells (marked by white arrows) and endocrine cells (red arrows) of 3-week chick and adult chicken anterior pituitaries by immunofluorescent staining. The NPY immuno-reactive signal was labeled with Alexa Fluor 488 (green). Nuclei are stained with DAPI (blue). Scale bar: 250 μm/50 μm.
In line with scRNA-seq analysis showing NPY expression in FS-cell (and Gona-) cluster, NPY-immunoreactive (NPY-ir) signals were likely localized in ‘star/stellate-shape’ FS-cells and some endocrine cells distributed within the pituitary cephalic lobe and caudal lobe, which resembles the distribution of LH-cells (Proudman et al., 1999). This finding also supports the reliability of scRNA-sequencing results (Figure 8C).
Apart from genes encoding growth factors/peptides, many genes encoding transcription factors, WNT receptors, Shh signaling components, the pathway inhibitors of WNT, BMP and IGFs signaling (Supplementary Figure 4), and proteins associated with the extracellular organization (Supplementary Figure 5), cell-cell junction (Supplementary Figure 6), biological oxidation (Supplementary Figure 7), fatty acid metabolism (Supplementary Figure 8), steroid metabolism (Supplementary Figure 9), cholesterol biosynthesis (Supplementary Figure 10), peptide/protein processing and epithelial-to-mesenchymal transition (EMT) (Supplementary Figure 11), have also been found to be expressed in FS-cells. Moreover, we noted that the mRNA levels of the afore-mentioned genes differ from those in all other pituitary cell types (Supplementary Figures 4–11).
It has been hypothesized that in mammals, FS-cell may regulate endocrine cell functions by secretion of paracrine/autocrine factors, such as VEGF, annexin 1 (AXNA1), follistatin, PACAP, TGFβ1, WNT5A, and leptin (LEP) (Denef, 2008). However, this hypothesis still lacks experimental support from other model animals. In this study, we found that FS-cells can abundantly or specifically express many genes encoding growth factors and peptides (Figure 8). Moreover, the cognate receptors for most of these factors/peptides were found to be expressed in other cell populations. In agree with the finding in cell-cell communications by CellChat, our findings strongly suggest that FS-cells are capable of producing a list of growth factors/peptides (Figure 8), and thus likely act as a paracrine/autocrine signaling center to control the proliferation, differentiation, apoptosis and functions of pituitary cells. For instance, activin A (a homodimer of activin βA subunit) from FS-cells may stimulate FSHB expression in gonadotrophs expressing activin receptors (ACVRs), as demonstrated in mammals and teleosts (Gregory et al., 2005; Lau and Ge, 2005), while HBEGF/AREG/FGFs from FS-cells may stimulate cell proliferation, or prevent cell apoptosis/differentiation via EGFR/FGFR family members, as documented in various normal/cancerous tissues (Normanno et al., 2006; Wang et al., 2007; Tiong et al., 2013).
Besides the growth factors/peptides, we also found the abundant expression of ALDH1A3 and ALDH1A1, the two enzymes for retinoic acid (RA) synthesis, in FS-cells. This finding points to a possibility that RA is likely produced in the FS-cells and acts as an additional paracrine/autocrine factor to regulate the differentiation and function of cells expressing RA receptors (RARs)/rational X receptors (RXRs) (Figure 6), similar to that proposed in rat anterior pituitary (Maliza et al., 2017). Interestingly, the antagonists or inhibitors for WNT (SRFP1, SRFP2), BMP (FSTL1, CHRDL), and IGF1 (IGFBP4) signaling were also expressed in FS-cells. These findings suggest their involvement in fine-tuning the paracrine/autocrine signaling of WNTs/BMPs/IGFs in responsive cells (Figures 7C, 8).
In this study, we also noted that many receptors are expressed in FS-cells, such as SHH receptor (PTCH1), PRLR, and activin/TGFβ/BMP receptors (Figure 6). Since SHH is highly expressed in gonadotrophs (Supplementary Figure 4), and PRL is predominantly expressed in lactotrophs, respectively, our finding strongly suggests that FS-cells can also receive signals from neighboring cells, including endocrine cells and FS-cells. This ‘bi-directional cell-to-cell communication’ may be crucial for dynamic control of pituitary cell composition and function to meet the physiological demands in chickens, as reported in mammals (Denef, 2008; Ren et al., 2019).
FS-Cell May Enrich Progenitor/Stem Cells
Interestingly, in the FS-cell cluster, we also observed a relatively high expression of NOTCH signaling components (NOTCH1, NOTCH2, HEY1, HEYL, HES1, HES5, and HES5-like (HES5L, ENSGALG00000001136). This finding, together with the expression of Notch ligands (JAG1, JAG2, DLL1, DLL4, and DLK1) in neighboring cells, support the existence of NOTCH signaling in FS sub-population (Figures 7C, 9A,B).
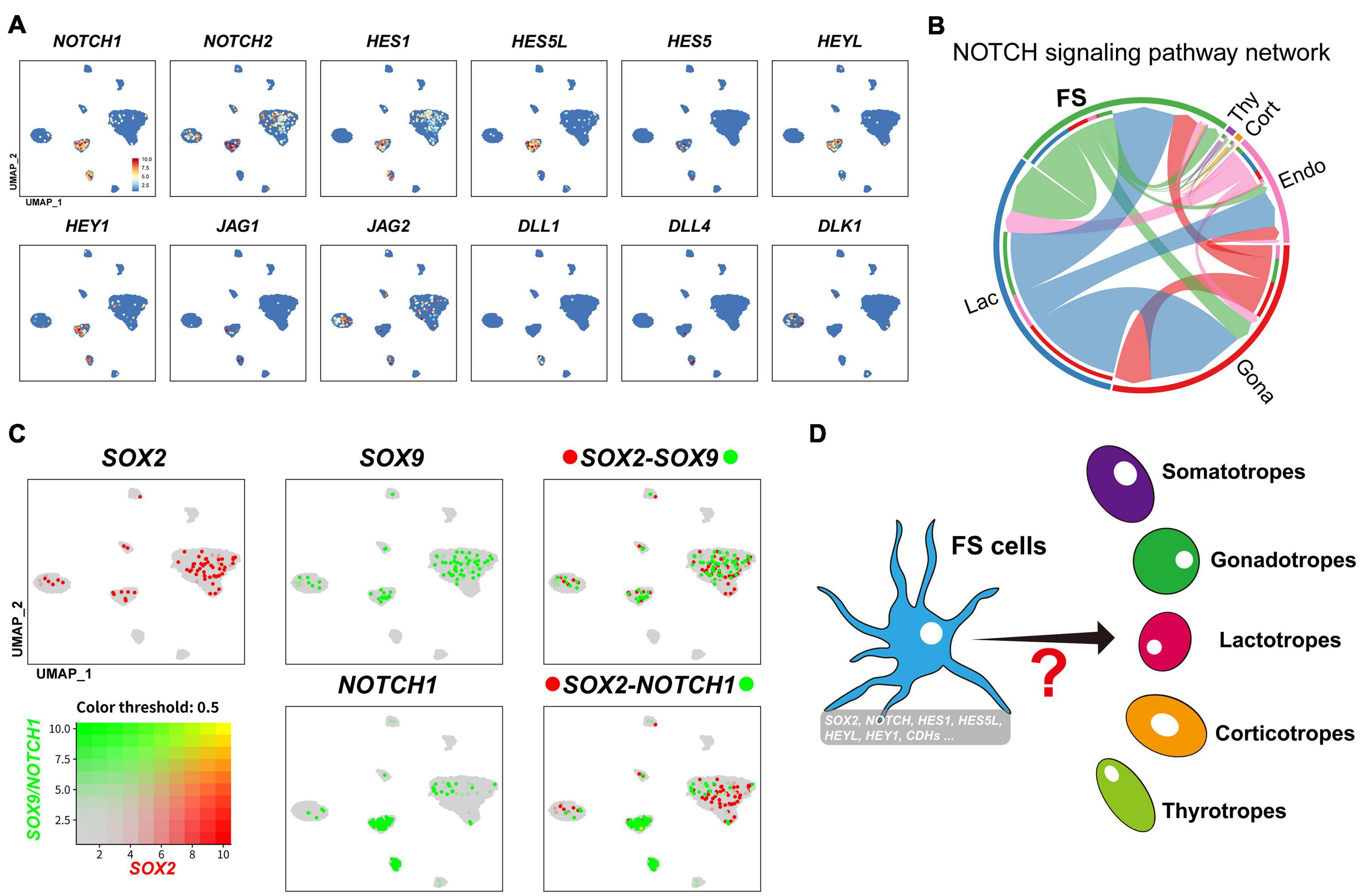
Figure 9. (A) UMAP maps showing the abundant/specific expression of genes encoding Notch signaling components (NOTCH1, NOTCH2, HES1, HES5L, HES5, HEYL, and HEY1) in FS-cell cluster and Notch ligands (JAG1, JAG2, DLL1, DLL4, and DLK1) in FS-cells, endothelial cells, and endocrine cell clusters (Gona and Lac). Dark red and light blue indicate high and low expression levels, respectively. (B) The intercellular communication network of NOTCH signaling pathway. The circle segments represent the six main intercellular communication cell types with different colors. The edge width is proportional to the communication score between interacting cell clusters. (C) Co-expression analysis of SOX2 and SOX9 in chicken pituitary FS-cells and other cell clusters. Cells abundantly co-expressing SOX2 and SOX9 are shown in yellow. (D) A simplified model showing that a sub-population of FS-cell cluster co-expressing many progenitor/stem cell-associated factors previously reported (Notch signaling components, CDH1 and SOX2 etc. (Vankelecom, 2010; Edwards and Raetzman, 2018) may represent the progenitor/stem cell sub-population, which could differentiate into endocrine cells (e.g., lactotrophs and gonadotrophs) under some unknown induction signals in adult pituitary (marked by question mark).
It was proposed that FS-cells may be one source of progenitor/stem cells in mammals, however, this hypothesis has received little attention to date (Inoue et al., 2002; Vankelecom, 2007). In this study, we found that chicken FS-cells can express many transcription factors (e.g., Pitx1, Pitx2, Lhx3, PRRX1, and PRRX2), which resembles the characteristics of embryonic pituitary stem/progenitors (Supplementary Figure 4; Kelberman et al., 2009). Moreover, FS-cells can also express a set of NOTCH signaling components, which have been shown to be crucial for the maintenance, expansion, and cell-fate choice of progenitor/stem cells of the anterior pituitary and other tissues in mammals (Suh et al., 2002; Edwards and Raetzman, 2018). Since NOTCH ligands could be identified in neighboring cells, such as gonadotrophs (JAG2, DLK1), lactotrophs (JAG2), endothelial cells (DLL1 and DLL4), and FS-cells (JAG1) (Figures 8, 9), this supports the existence of juxtracrine NOTCH signaling in chicken pituitary FS-cells. In addition, many genes associated with mammalian stem cell niche formation (E-cadherin) (Batchuluun et al., 2017), or the stem cell renewal, survival, and fate determination of the pituitary and other tissues (e.g., PAX6, ID1-4, Shh-PTCH signaling, WNT signaling, EGFR and FGFR signaling, ALDH1A1, and ALDH1A3) (Ying et al., 2003; Chen et al., 2005; Fauquier et al., 2008; Jiang et al., 2008; Sansom et al., 2009; Vankelecom and Chen, 2014; Zhu et al., 2015; Tomita et al., 2016), are abundantly expressed in chicken FS-cells. The co-expression of so many progenitor/stem cell-associated genes (shown in Supplementary Figure 11) in the FS-cell cluster (Vankelecom, 2010; Yavropoulou et al., 2015) led us to propose that adult progenitor/stem cells, resembling the features of embryonic progenitor/stem cells, may be enriched in the FS sub-population, and thereby confer some plasticity to meet the physiological needs in adult pituitary. Clearly, more studies are required to substantiate this hypothesis.
As the pituitary progenitor/stem-cell markers reported in mammals (Fauquier et al., 2008), SOX2 was also found to be expressed in the FS-cell and endocrine cells (e.g., gonadotroph) of chicken pituitaries (Figure 9B and Supplementary Figure 12). In mammals, SOX2/SOX9-positive cells have been suggested to be pituitary progenitor/stem cells (Yoshida et al., 2011; Andoniadou et al., 2013). In this study, we found that SOX2/SOX9 is expressed in FS-cells and other endocrine cells, such as in lactotrophs and gonadotrophs abundantly expressing PRL and LHB, respectively (Figure 9B and Supplementary Figure 12). The expression of SOX2/SOX9 in fully differentiated endocrine cells indicates that unlike that in mammals (Cheung et al., 2018), SOX2 may not be the sole marker for adult pituitary progenitor/stem cells in chickens. Therefore, future study focused on FS sub-population co-expressing the set of progenitor/stem cell-associated genes (NOTCH signaling molecules, CDH1, SOX2, and Pitx2 etc.) (Supplementary Figure 12) may help to precisely define the avian pituitary progenitor/stem cells, and thereby, allow us to trace their differentiation routes, when signaled by inner physiological demands and environmental cues (e.g., light, temperature, food availability) (Figure 9C).
Sexual Dimorphism of Gene Expression Pattern in Pituitary Cell Clusters
In this study, sexually dimorphic expression of many genes in endocrine cell, FS-cell and Endo-cell clusters have been found, particularly, in gonadotroph (173 genes), lactotroph (123 genes) and FS-cell (46 genes) clusters (Figure 10A and Supplementary Data 2). More than 98 genes including RLN3, GRP, C5H11ORF96, CPLX1 and HPDGS are shown to be specifically/preferentially expressed in gonadotrophs of female chicken anterior pituitaries, while 75 genes including HBA1, HBBA, HSPA2, EGR1, and JUN, are found to be expressed in the male gonadotroph cluster specifically/preferentially (Figure 10B and Supplementary Data 2). Using quantitative real-time PCR (qPCR), we also confirmed the sexually dimorphic expression patterns of GRP, RLN3, and HPGDS at the whole anterior pituitary level (Figure 10C).
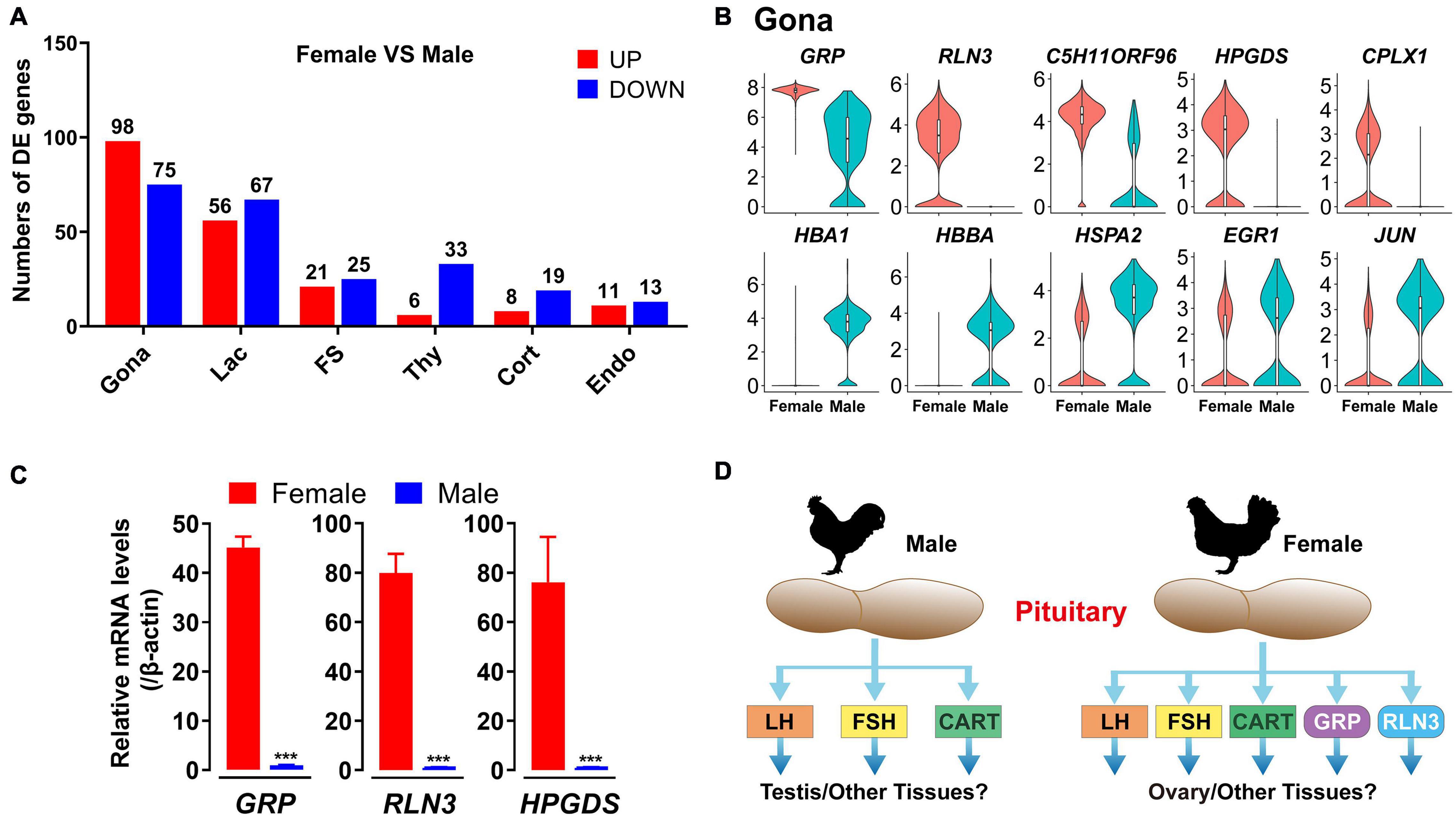
Figure 10. Sexually dimorphic expression of genes in adult chicken male and female anterior pituitary cell clusters. (A) Bar charts presenting the numbers of differentially expressed (DE) genes in each cell cluster in male and female chicken anterior pituitaries. Differences in gene expression level were significant if the adjusted P-value was below the multiple-testing threshold of 0.001 and the absolute log2 expression fold change was above 0.5; (B) Violin plots showing the normalized expression levels of selected genes differentially expressed in gonadotrophs (Gona) of male and female chicken pituitaries. Box plot: median (center black line), interquartile range (white box) and minimum-maximum range (whiskers). (C) qPCR detection of chicken GRP, RLN3 and HPGDS mRNA levels between male and female chicken pituitaries. Each data point represents the mean ± SEM of four laying hens/male chickens (N = 4). ***, P < 0.001 between two groups. (D) Major endocrine hormones secreted by pituitary gonadotrophs of adult male and female chickens. In male chickens, gonadotroph can abundantly express LHB, FSHB and CART, and thus may produce three major endocrine hormones, which may play important roles in the testis (and other tissues), whereas in hens, gonadotrophs may produce 5 major hormones (LH, FSH, CART, GRP, and RLN3) and likely play roles in the ovary (and other tissues). However, this hypothesis needs further verification.
Marked sexual dimorphism was also evident in endocrine cell clusters, particularly in lactotrophs and gonadotrophs, in rodents (Fletcher et al., 2019; Ho et al., 2020). The predominant expression of FSHB mRNA in male chicken gonadotrophs was consistent with previously reports in rodents. These findings, taken together, support a functional difference of the anterior pituitary between the two sexes in birds and mammals. We noted that some genes with dimorphic expression are associated with their localization on sex chromosomes Z (18 genes, including RLN3, GRP, CPLX1, HEXB, FAM189A2 etc.) or W (2 genes, HINTW and ENSGALG00000040263) (Supplementary Data 2). However, most genes with sexually dimorphic expression are localized on autosomes, which expression is likely controlled by sex-dependent factors, such as sex steroids.
The sexually dimorphic expression patterns of genes in pituitary cells may have important physiological relevance. As an example, novel peptide hormones GRP and RLN3, highly expressed in female (and not male) chicken pituitary gonadotrophs, may play important roles in female reproduction, such as ovary/oviduct development and functions (Figure 10D). Future studies on these genes in chickens and other birds not only can help to uncover their influence on growth, metabolism, stress, and reproduction of male and female birds, but also help to reveal their association with the phenotypic traits of birds (e.g., egg-laying performance, reproduction mode, meat production, body composition, and behaviors) (Fallahshahroudi et al., 2019).
Conclusion
In summary, we identified four endocrine and four non-endocrine cell population within the chicken pituitary and characterized their gene expression profiles, including their sexually dimorphic gene expression. We also found several inter-cellular crosstalk between the cell clusters in adult chickens (Figure 7A). Among these cell types, FS-cells can express many genes encoding growth factors and peptides and they likely act as a paracrine/autocrine signaling center to influence neighboring cells. Undoubtedly, our data sets a critical reference point to reveal the novel aspects of pituitary biology across vertebrates, and helps to figure out how the anterior pituitary, as a pivotal signal converging and output center for the brain and peripheral tissues, can orchestrate so many physiological processes (e.g., growth, reproduction, metabolism, and stress) to meet the changing physiological demands or pathological status observed in vertebrates, including birds and humans.
Data Availability Statement
The scRNA-seq clean data reported in this paper have been deposited in the Genome Sequence Archive in National Genomics Data Center, China National Center for Bioinformation/Beijing Institute of Genomics, Chinese Academy of Sciences, under accession number CRA003604 that are publicly accessible at https://ngdc.cncb.ac.cn/gsa/.
Ethics Statement
All animal experiments were conducted in accordance with the Guidelines for Experimental Animals issued by the Ministry of Science and Technology of People’s Republic of China. All animal experimental protocols were approved by the Animal Ethics Committee of the College of Life Sciences, Sichuan University (Chengdu, China).
Author Contributions
JZ did the investigation, data analysis, writing-original draft, and writing-review and editing. CL did the conceptualization, investigation, and writing-original draft. CM did the conceptualization and investigation. ML and YPW did the investigation. JL did the resources and writing-review and editing. YJW did the conceptualization, resources, writing-original draft, and writing-review and editing. All authors contributed to the article and approved the submitted version.
Funding
This work was supported by grants from National Natural Science Foundation of China (31771375, 31772590, 31802056, and 31572391), the Fundamental Research Funds for the Central Universities, and the Project Funded by China Postdoctoral Science Foundation (2019M653412 and 2020T130439).
Conflict of Interest
The authors declare that the research was conducted in the absence of any commercial or financial relationships that could be construed as a potential conflict of interest.
Acknowledgments
We would like to give special thanks to the reviewers for their constructive suggestions which help to improve the quality of this manuscript. We are grateful to Amy H.Y. Kwok and Tianming Wu for their comments and helpful suggestions during the revision.
Supplementary Material
The Supplementary Material for this article can be found online at: https://www.frontiersin.org/articles/10.3389/fphys.2021.562817/full#supplementary-material
Footnotes
- ^ http://www.ensembl.org/Gallus_gallus
- ^ ftp://ftp.ensembl.org/pub/release-72/fasta/gallus_gallus/dna
References
Achermann, J. C., Meeks, J. J., and Jameson, J. L. (2001). Phenotypic spectrum of mutations in DAX-1 and SF-1. Mol. Cell. Endocrinol. 185, 17–25. doi: 10.1016/s0303-7207(01)00619-0
Al Kahtane, A., Chaiseha, Y., and El Halawani, M. (2003). Dopaminergic regulation of avian prolactin gene transcription. J. Mol. Endocrinol. 31, 185–196. doi: 10.1677/jme.0.0310185
Anbalagan, S., Gordon, L., Blechman, J., Matsuoka, R. L., Rajamannar, P., Wircer, E., et al. (2018). Pituicyte cues regulate the development of permeable neuro-vascular interfaces. Dev. Cell 47, 711–726.e715. doi: 10.1016/j.devcel.2018.10.017
Andoniadou, C. L., Matsushima, D., Mousavy Gharavy, S. N., Signore, M., Mackintosh, A. I., Schaeffer, M., et al. (2013). Sox2(+) stem/progenitor cells in the adult mouse pituitary support organ homeostasis and have tumor-inducing potential. Cell Stem Cell 13, 433–445. doi: 10.1016/j.stem.2013.07.004
Batchuluun, K., Azuma, M., Yashiro, T., and Kikuchi, M. (2017). Notch signaling-mediated cell-to-cell interaction is dependent on E-cadherin adhesion in adult rat anterior pituitary. Cell Tissue Res. 368, 125–133. doi: 10.1007/s00441-016-2540-5
Becht, E., McInnes, L., Healy, J., Dutertre, C. A., Kwok, I. W. H., Ng, L. G., et al. (2018). Dimensionality reduction for visualizing single-cell data using UMAP. Nat. Biotechnol. 37, 38–44. doi: 10.1038/nbt.4314
Bu, G., Fan, J., Yang, M., Lv, C., Lin, Y., Li, J., et al. (2019). Identification of a novel functional corticotropin-releasing hormone (CRH2) in chickens and its roles in stimulating pituitary TSHbeta expression and ACTH secretion. Front. Endocrinol. (Lausanne) 10:595. doi: 10.3389/fendo.2019.00595
Bu, G., Lin, D., Cui, L., Huang, L., Lv, C., Huang, S., et al. (2016). Characterization of neuropeptide B (NPB), neuropeptide W (NPW), and their receptors in chickens: evidence for NPW being a novel inhibitor of pituitary GH and prolactin secretion. Endocrinology 157, 3562–3576. doi: 10.1210/en.2016-1141
Butler, A., Hoffman, P., Smibert, P., Papalexi, E., and Satija, R. (2018). Integrating single-cell transcriptomic data across different conditions, technologies, and species. Nat. Biotechnol. 36, 411–420. doi: 10.1038/nbt.4096
Cai, G., Mo, C., Huang, L., Li, J., and Wang, Y. (2015). Characterization of the two CART genes (CART1 and CART2) in chickens (Gallus gallus). PLoS One 10:e0127107. doi: 10.1371/journal.pone.0127107
Chen, J., Hersmus, N., Van Duppen, V., Caesens, P., Denef, C., and Vankelecom, H. (2005). The adult pituitary contains a cell population displaying stem/progenitor cell and early embryonic characteristics. Endocrinology 146, 3985–3998. doi: 10.1210/en.2005-0185
Cheung, L. Y. M., George, A. S., McGee, S. R., Daly, A. Z., Brinkmeier, M. L., Ellsworth, B. S., et al. (2018). Single-cell RNA sequencing reveals novel markers of male pituitary stem cells and hormone-producing cell types. Endocrinology 159, 3910–3924. doi: 10.1210/en.2018-00750
Childs, G. V., MacNicol, A. M., and MacNicol, M. C. (2020). Molecular mechanisms of pituitary cell plasticity. Front. Endocrinol. (Lausanne) 11:656. doi: 10.3389/fendo.2020.00656
Childs, G. V., Unabia, G., and Wu, P. (2000). Differential expression of growth hormone messenger ribonucleic acid by somatotropes and gonadotropes in male and cycling female rats. Endocrinology 141, 1560–1570. doi: 10.1210/endo.141.4.7429
De Groef, B., Goris, N., Arckens, L., Kuhn, E. R., and Darras, V. M. (2003). Corticotropin-releasing hormone (CRH)-induced thyrotropin release is directly mediated through CRH receptor type 2 on thyrotropes. Endocrinology 144, 5537–5544. doi: 10.1210/en.2003-0526
De Groef, B., Grommen, S. V., and Darras, V. M. (2007). Feedback control of thyrotropin secretion in the chicken: thyroid hormones increase the expression of hypophyseal somatostatin receptor types 2 and 5. Gen. Comp. Endocrinol. 152, 178–182. doi: 10.1016/j.ygcen.2007.01.036
Denef, C. (2008). Paracrinicity: the story of 30 years of cellular pituitary crosstalk. J. Neuroendocrinol. 20, 1–70. doi: 10.1111/j.1365-2826.2007.01616.x
Edwards, W., and Raetzman, L. T. (2018). Complex integration of intrinsic and peripheral signaling is required for pituitary gland development. Biol. Reprod. 99, 504–513. doi: 10.1093/biolre/ioy081
el Halawani, M. E., Silsby, J. L., and Mauro, L. J. (1990). Vasoactive intestinal peptide is a hypothalamic prolactin-releasing neuropeptide in the turkey (Meleagris gallopavo). Gen. Comp. Endocrinol. 78, 66–73. doi: 10.1016/0016-6480(90)90048-q
Fabian, P., Tseng, K. C., Smeeton, J., Lancman, J. J., Dong, P. D. S., Cerny, R., et al. (2020). Lineage analysis reveals an endodermal contribution to the vertebrate pituitary. Science 370, 463–467. doi: 10.1126/science.aba4767
Fallahshahroudi, A., Lotvedt, P., Belteky, J., Altimiras, J., and Jensen, P. (2019). Changes in pituitary gene expression may underlie multiple domesticated traits in chickens. Heredity (Edinb) 122, 195–204. doi: 10.1038/s41437-018-0092-z
Fauquier, T., Rizzoti, K., Dattani, M., Lovell-Badge, R., and Robinson, I. C. (2008). SOX2-expressing progenitor cells generate all of the major cell types in the adult mouse pituitary gland. Proc. Natl. Acad. Sci. U.S.A. 105, 2907–2912. doi: 10.1073/pnas.0707886105
Finak, G., McDavid, A., Yajima, M., Deng, J., Gersuk, V., Shalek, A. K., et al. (2015). MAST: a flexible statistical framework for assessing transcriptional changes and characterizing heterogeneity in single-cell RNA sequencing data. Genome Biol. 16:278. doi: 10.1186/s13059-015-0844-5
Fletcher, P. A., Smiljanic, K., Maso Previde, R., Iben, J. R., Li, T., Rokic, M. B., et al. (2019). Cell type- and sex-dependent transcriptome profiles of rat anterior pituitary cells. Front. Endocrinol. (Lausanne) 10:623. doi: 10.3389/fendo.2019.00623
French, C. A., Groszer, M., Preece, C., Coupe, A. M., Rajewsky, K., and Fisher, S. E. (2007). Generation of mice with a conditional Foxp2 null allele. Genesis 45, 440–446. doi: 10.1002/dvg.20305
Fu, X., and Porter, T. E. (2004). Glucocorticoid induction of lactotrophs and prolactin gene expression in chicken embryonic pituitary cells: a delayed response relative to stimulated growth hormone production. Endocrinology 145, 1322–1330. doi: 10.1210/en.2003-1064
Gao, S., Zhang, J., He, C., Meng, F., Bu, G., Zhu, G., et al. (2017). Molecular characterization of neuropeptide Y (NPY) receptors (Y1, Y4 and Y6) and investigation of the tissue expression of their ligands (NPY, PYY and PP) in chickens. Gen. Comp. Endocrinol. 240, 46–60. doi: 10.1016/j.ygcen.2016.09.005
Gleiberman, A. S., Michurina, T., Encinas, J. M., Roig, J. L., Krasnov, P., Balordi, F., et al. (2008). Genetic approaches identify adult pituitary stem cells. Proc. Natl. Acad. Sci. U.S.A. 105, 6332–6337. doi: 10.1073/pnas.0801644105
Gregory, S. J., Lacza, C. T., Detz, A. A., Xu, S., Petrillo, L. A., and Kaiser, U. B. (2005). Synergy between activin A and gonadotropin-releasing hormone in transcriptional activation of the rat follicle-stimulating hormone-beta gene. Mol. Endocrinol. 19, 237–254. doi: 10.1210/me.2003-0473
Hall, T. R., Cheung, A., and Harvey, S. (1986). Serotoninergic inhibition of LH secretion in the domestic fowl. J. Endocrinol. 110, 239–244. doi: 10.1677/joe.0.1100239
Halls, M. L., Bathgate, R. A., Sutton, S. W., Dschietzig, T. B., and Summers, R. J. (2015). International Union of Basic and Clinical Pharmacology. XCV. Recent advances in the understanding of the pharmacology and biological roles of relaxin family peptide receptors 1-4, the receptors for relaxin family peptides. Pharmacol. Rev. 67, 389–440. doi: 10.1124/pr.114.009472
Hao, Y., Hao, S., Andersen-Nissen, E., Mauck, W. M., Zheng, S., Butler, A., et al. (2020). Integrated analysis of multimodal single-cell data. bioRxiv [Preprint]. doi: 10.1101/2020.10.12.335331
Harvey, S., Gineste, C., and Gaylinn, B. D. (2014). Growth hormone (GH)-releasing activity of chicken GH-releasing hormone (GHRH) in chickens. Gen. Comp. Endocrinol. 204, 261–266. doi: 10.1016/j.ygcen.2014.06.007
Harvey, S., Scanes, C. G., Chadwick, A., and Bolton, N. J. (1979). Growth hormone and prolactin secretion in growing domestic fowl: influence of sex and breed. Br. Poult. Sci. 20, 9–17. doi: 10.1080/00071667908416544
Ho, Y., Hu, P., Peel, M. T., Chen, S., Camara, P. G., Epstein, D. J., et al. (2020). Single-cell transcriptomic analysis of adult mouse pituitary reveals sexual dimorphism and physiologic demand-induced cellular plasticity. Protein Cell 11, 565–583. doi: 10.1007/s13238-020-00705-x
Hodne, K., Fontaine, R., Ager-Wick, E., and Weltzien, F.-A. (2019). Gnrh1-induced responses are indirect in female medaka Fsh cells, generated through cellular networks. Endocrinology 160, 3018–3032. doi: 10.1210/en.2019-00595
Huang, G., He, C., Meng, F., Li, J., Zhang, J., and Wang, Y. (2014). Glucagon-like peptide (GCGL) is a novel potential TSH-releasing factor (TRF) in Chickens: I) Evidence for its potent and specific action on stimulating TSH mRNA expression and secretion in the pituitary. Endocrinology 155, 4568–4580. doi: 10.1210/en.2014-1331
Ikeda, Y., Swain, A., Weber, T. J., Hentges, K. E., Zanaria, E., Lalli, E., et al. (1996). Steroidogenic factor 1 and Dax-1 colocalize in multiple cell lineages: potential links in endocrine development. Mol. Endocrinol. 10, 1261–1272. doi: 10.1210/mend.10.10.9121493
Inoue, K., Mogi, C., Ogawa, S., Tomida, M., and Miyai, S. (2002). Are folliculo-stellate cells in the anterior pituitary gland supportive cells or organ-specific stem cells? Arch. Physiol. Biochem. 110, 50–53. doi: 10.1076/apab.110.1.50.911
Jiang, J., Chan, Y. S., Loh, Y. H., Cai, J., Tong, G. Q., Lim, C. A., et al. (2008). A core Klf circuitry regulates self-renewal of embryonic stem cells. Nat. Cell Biol. 10, 353–360. doi: 10.1038/ncb1698
Jin, S., Guerrero-Juarez, C. F., Zhang, L., Chang, I., Ramos, R., Kuan, C. H., et al. (2021). Inference and analysis of cell-cell communication using CellChat. Nat. Commun. 12:1088. doi: 10.1038/s41467-021-21246-9
Kaiser, U., and Ho, K. K. (2016). “Pituitary physiology and diagnostic evaluation,” in Williams Textbook of Endocrinology, eds S. Melmed, K. S. Polonsky, P. R. Larsen, and H. M. Kronenberg (Philadelphia, PA: Elsevier), 176–231. doi: 10.1016/b978-0-323-29738-7.00008-3
Kappeler, L., Gautron, L., Laye, S., Dantzer, R., Zizzari, P., Epelbaum, J., et al. (2006). Pituitary cocaine- and amphetamine-regulated transcript expression depends on the strain, sex and oestrous cycle in the rat. J. Neuroendocrinol. 18, 426–433. doi: 10.1111/j.1365-2826.2006.01435.x
Kelberman, D., Rizzoti, K., Lovell-Badge, R., Robinson, I. C., and Dattani, M. T. (2009). Genetic regulation of pituitary gland development in human and mouse. Endocr. Rev. 30, 790–829. doi: 10.1210/er.2009-0008
Kuriyama, G., Takekoshi, S., Tojo, K., Nakai, Y., Kuhar, M. J., and Osamura, R. Y. (2004). Cocaine- and amphetamine-regulated transcript peptide in the rat anterior pituitary gland is localized in gonadotrophs and suppresses prolactin secretion. Endocrinology 145, 2542–2550. doi: 10.1210/en.2003-0845
Kwok, A. H., Wang, Y., and Leung, F. C. (2012). Molecular characterization of prostaglandin F receptor (FP) and E receptor subtype 3 (EP3) in chickens. Gen. Comp. Endocrinol. 179, 88–98. doi: 10.1016/j.ygcen.2012.07.019
Larsson, L. I. (1998). On the development of the islets of Langerhans. Microsc. Res. Tech. 43, 284–291. doi: 10.1002/(SICI)1097-0029(19981115)43:4<284::AID-JEMT2<3.0.CO;2-0
Lau, M. T., and Ge, W. (2005). Cloning of Smad2, Smad3, Smad4, and Smad7 from the goldfish pituitary and evidence for their involvement in activin regulation of goldfish FSHbeta promoter activity. Gen. Comp. Endocrinol. 141, 22–38. doi: 10.1016/j.ygcen.2004.10.019
Le Tissier, P. R., Hodson, D. J., Lafont, C., Fontanaud, P., Schaeffer, M., and Mollard, P. (2012). Anterior pituitary cell networks. Front. Neuroendocrinol. 33:252. doi: 10.1016/j.yfrne.2012.08.002
Liu, H., Luo, Q., Zhang, J., Mo, C., Wang, Y., and Li, J. (2019). Endothelins (EDN1, EDN2, EDN3) and their receptors (EDNRA, EDNRB, EDNRB2) in chickens: Functional analysis and tissue distribution. Gen. Comp. Endocrinol. 283:113231. doi: 10.1016/j.ygcen.2019.113231
Lv, C., Mo, C., Liu, H., Wu, C., Li, Z., Li, J., et al. (2018). Dopamine D2-like receptors (DRD2 and DRD4) in chickens: Tissue distribution, functional analysis, and their involvement in dopamine inhibition of pituitary prolactin expression. Gene 651, 33–43. doi: 10.1016/j.gene.2018.01.087
Macnamee, M. C., Sharp, P. J., Lea, R. W., Sterling, R. J., and Harvey, S. (1986). Evidence that vasoactive intestinal polypeptide is a physiological prolactin-releasing factor in the bantam hen. Gen. Comp. Endocrinol. 62, 470–478. doi: 10.1016/0016-6480(86)90057-2
Macosko, E. Z., Basu, A., Satija, R., Nemesh, J., Shekhar, K., Goldman, M., et al. (2015). Highly parallel genome-wide expression profiling of individual cells using nanoliter droplets. Cell 161, 1202–1214. doi: 10.1016/j.cell.2015.05.002
Maliza, R., Fujiwara, K., Azuma, M., Kikuchi, M., and Yashiro, T. (2017). Effect of retinoic acid on midkine gene expression in rat anterior pituitary cells. Endocr. J. 64, 633–638. doi: 10.1507/endocrj.EJ17-0006
McGinnis, C. S., Murrow, L. M., and Gartner, Z. J. (2019). DoubletFinder: doublet detection in single-cell RNA sequencing data using artificial nearest neighbors. Cell Syst 8, 329–337.e324. doi: 10.1016/j.cels.2019.03.003
Meng, F., Huang, G., Gao, S., Li, J., Yan, Z., and Wang, Y. (2014). Identification of the receptors for somatostatin (SST) and cortistatin (CST) in chickens and investigation of the roles of cSST28, cSST14, and cCST14 in inhibiting cGHRH1-27NH2-induced growth hormone secretion in cultured chicken pituitary cells. Mol. Cell. Endocrinol. 384, 83–95. doi: 10.1016/j.mce.2014.01.001
Mikhailova, M. V., Mayeux, P. R., Jurkevich, A., Kuenzel, W. J., Madison, F., Periasamy, A., et al. (2007). Heterooligomerization between vasotocin and corticotropin-releasing hormone (CRH) receptors augments CRH-stimulated 3′,5′-cyclic adenosine monophosphate production. Mol. Endocrinol. 21, 2178–2188. doi: 10.1210/me.2007-0160
Millar, R. P. (2005). GnRHs and GnRH receptors. Anim. Reprod. Sci. 88, 5–28. doi: 10.1016/j.anireprosci.2005.05.032
Mo, C., Huang, L., Cui, L., Lv, C., Lin, D., Song, L., et al. (2017). Characterization of NMB, GRP and their receptors (BRS3, NMBR and GRPR) in chickens. J. Mol. Endocrinol. 59, 61–79. doi: 10.1530/JME-17-0020
Mo, C., Lv, C., Huang, L., Li, Z., Zhang, J., Li, J., et al. (2019). Regulation of pituitary cocaine- and amphetamine-regulated transcript expression and secretion by hypothalamic gonadotropin-releasing hormone in chickens. Front Physiol 10:882. doi: 10.3389/fphys.2019.00882
Normanno, N., De Luca, A., Bianco, C., Strizzi, L., Mancino, M., Maiello, M. R., et al. (2006). Epidermal growth factor receptor (EGFR) signaling in cancer. Gene 366, 2–16. doi: 10.1016/j.gene.2005.10.018
Pinelli, C., Fiorentino, M., D’Aniello, B., Tanaka, S., and Rastogi, R. K. (1996). Immunohistochemical demonstration of FSH and LH in the pituitary of the developing frog, Rana esculenta. Gen. Comparat. Endocrinol. 104, 189–196. doi: 10.1006/gcen.1996.0161
Proudman, J. A., Vandesande, F., and Berghman, L. R. (1999). Immunohistochemical evidence that follicle-stimulating hormone and luteinizing hormone reside in separate cells in the chicken pituitary. Biol. Reprod. 60, 1324–1328. doi: 10.1095/biolreprod60.6.1324
Puebla-Osorio, N., Proudman, J., Compton, A., Clements, K., Decuypere, E., Vandesande, F., et al. (2002). FSH-and LH-cells originate as separate cell populations and at different embryonic stages in the chicken embryo. Gen. Compar. Endocrinol. 127, 242–248. doi: 10.1016/s0016-6480(02)00054-0
Ren, Y. A., Monkkonen, T., Lewis, M. T., Bernard, D. J., Christian, H. C., Jorgez, C. J., et al. (2019). S100a4-Cre-mediated deletion of Patched1 causes hypogonadotropic hypogonadism: role of pituitary hematopoietic cells in endocrine regulation. JCI Insight 5:325. doi: 10.1172/jci.insight.126325
Rizzoti, K. (2015). Genetic regulation of murine pituitary development. J. Mol. Endocrinol. 54, R55–R73. doi: 10.1530/JME-14-0237
Sansom, S. N., Griffiths, D. S., Faedo, A., Kleinjan, D. J., Ruan, Y., Smith, J., et al. (2009). The level of the transcription factor Pax6 is essential for controlling the balance between neural stem cell self-renewal and neurogenesis. PLoS Genet. 5:e1000511. doi: 10.1371/journal.pgen.1000511
Scanes, C. G. (2016). Opening a New Door: Neuropeptide W (NPW) Is a Novel Inhibitory Secretagogue for GH and Prolactin Acting via the Gi Protein-Coupled NPBWR2. Endocrinology 157, 3394–3397. doi: 10.1210/en.2016-1518
Scanes, C. G., Sharp, P. J., Harvey, S., Godden, P. M., Chadwick, A., and Newcomer, W. S. (1979). Variations in plasma prolactin, thyroid hormones, gonadal steroids and growth hormone in turkeys during the induction of egg laying and moult by different photoperiods. Br. Poult. Sci. 20, 143–148. doi: 10.1080/00071667908416561
Schwartz, J. (2000). Intercellular communication in the anterior pituitary. Endocr. Rev. 21, 488–513. doi: 10.1210/edrv.21.5.0408
Selvam, R., Jurkevich, A., Kang, S. W., Mikhailova, M. V., Cornett, L. E., and Kuenzel, W. J. (2013). Distribution of the vasotocin subtype four receptor (VT4R) in the anterior pituitary gland of the chicken, Gallus gallus, and its possible role in the avian stress response. J. Neuroendocrinol. 25, 56–66. doi: 10.1111/j.1365-2826.2012.02370.x
Seuntjens, E., Hauspie, A., Roudbaraki, M., Vankelecom, H., and Denef, C. (2002). Combined expression of different hormone genes in single cells of normal rat and mouse pituitary. Arch. Physiol. Biochem. 110, 12–15. doi: 10.1076/apab.110.1.12.904
Sharp, P. J., Dawson, A., and Lea, R. W. (1998). Control of luteinizing hormone and prolactin secretion in birds. Comp. Biochem. Physiol. C Pharmacol. Toxicol. Endocrinol. 119, 275–282. doi: 10.1016/s0742-8413(98)00016-4
Sharp, P. J., Dunn, I. C., and Talbot, R. T. (1987). Sex differences in the LH responses to chicken LHRH-I and -II in the domestic fowl. J. Endocrinol. 115, 323–331. doi: 10.1677/joe.0.1150323
Sharp, P. J., Scanes, C. G., Williams, J. B., Harvey, S., and Chadwick, A. (1979). Variations in concentrations of prolactin, luteinizing hormone, growth hormone and progesterone in the plasma of broody bantams (Gallus domesticus). J. Endocrinol. 80, 51–57. doi: 10.1677/joe.0.0800051
Shen, X., Bai, X., Xu, J., Zhou, M., Xu, H., Nie, Q., et al. (2016). Transcriptome sequencing reveals genetic mechanisms underlying the transition between the laying and brooding phases and gene expression changes associated with divergent reproductive phenotypes in chickens. Mol. Biol. Rep. 43, 977–989. doi: 10.1007/s11033-016-4033-8
Siddique, K., Ager-Wick, E., Fontaine, R., Weltzien, F.-A., and Henkel, C. V. (2020). Characterization of hormone-producing cell types in the teleost pituitary gland using single-cell RNA-seq. bioRxiv [Preprint]. doi: 10.1101/2020.12.14.422690
Soneson, C., Love, M. I., and Robinson, M. D. (2015). Differential analyses for RNA-seq: transcript-level estimates improve gene-level inferences. F1000Research 4:1521. doi: 10.12688/f1000research.7563.2
Sreekumar, K. P., and Sharp, P. J. (1998). Ontogeny of the photoperiodic control of prolactin and luteinizing hormone secretion in male and female bantams (Gallus domesticus). Gen. Comp. Endocrinol. 109, 69–74. doi: 10.1006/gcen.1997.7009
Srivastava, A., Malik, L., Smith, T., Sudbery, I., and Patro, R. (2019). Alevin efficiently estimates accurate gene abundances from dscRNA-seq data. Genome Biol. 20:65. doi: 10.1186/s13059-019-1670-y
Stuart, T., Butler, A., Hoffman, P., Hafemeister, C., Papalexi, E., and Mauck, W. M. III, et al. (2019). Comprehensive integration of single-cell data. Cell 177, 1888–1902.e1821. doi: 10.1016/j.cell.2019.05.031
Suh, H., Gage, P. J., Drouin, J., and Camper, S. A. (2002). Pitx2 is required at multiple stages of pituitary organogenesis: pituitary primordium formation and cell specification. Development 129, 329–337. doi: 10.1242/dev.129.2.329
Tiong, K. H., Mah, L. Y., and Leong, C. O. (2013). Functional roles of fibroblast growth factor receptors (FGFRs) signaling in human cancers. Apoptosis 18, 1447–1468. doi: 10.1007/s10495-013-0886-7
Tomita, H., Tanaka, K., Tanaka, T., and Hara, A. (2016). Aldehyde dehydrogenase 1A1 in stem cells and cancer. Oncotarget 7, 11018–11032. doi: 10.18632/oncotarget.6920
Trapnell, C., Roberts, A., Goff, L., Pertea, G., Kim, D., Kelley, D. R., et al. (2012). Differential gene and transcript expression analysis of RNA-seq experiments with TopHat and Cufflinks. Nat. Protoc. 7, 562–578. doi: 10.1038/nprot.2012.016
Traverso, V., Christian, H. C., Morris, J. F., and Buckingham, J. C. (1999). Lipocortin 1 (annexin 1): a candidate paracrine agent localized in pituitary folliculo-stellate cells. Endocrinology 140, 4311–4319. doi: 10.1210/endo.140.9.7008
Treier, M., Gleiberman, A. S., O’Connell, S. M., Szeto, D. P., McMahon, J. A., McMahon, A. P., et al. (1998). Multistep signaling requirements for pituitary organogenesis in vivo. Genes Dev. 12, 1691–1704. doi: 10.1101/gad.12.11.1691
Van Nassauw, L., Harrisson, F., Cras, P., and Callebaut, M. (1987). Immunohistochemical localization of S-100 protein, glial fibrillary acidic protein, and neuron-specific enolase in the pars distalis of quail, rat, and human hypophyses. Histochemistry 86, 353–358. doi: 10.1007/BF00494992
Vankelecom, H. (2007). Non-hormonal cell types in the pituitary candidating for stem cell. Semin. Cell Dev. Biol. 18, 559–570. doi: 10.1016/j.semcdb.2007.04.006
Vankelecom, H. (2010). Pituitary stem/progenitor cells: embryonic players in the adult gland? Eur. J. Neurosci. 32, 2063–2081. doi: 10.1111/j.1460-9568.2010.07523.x
Vankelecom, H., and Chen, J. (2014). Pituitary stem cells: where do we stand? Mol. Cell. Endocrinol. 385, 2–17. doi: 10.1016/j.mce.2013.08.018
Vazquez-Borrego, M. C., Gahete, M. D., Martinez-Fuentes, A. J., Fuentes-Fayos, A. C., Castano, J. P., Kineman, R. D., et al. (2018). Multiple signaling pathways convey central and peripheral signals to regulate pituitary function: Lessons from human and non-human primate models. Mol. Cell. Endocrinol. 463, 4–22. doi: 10.1016/j.mce.2017.12.007
Villalobos, C., Nunez, L., and Garcia-Sancho, J. (2004). Phenotypic characterization of multi-functional somatotropes, mammotropes and gonadotropes of the mouse anterior pituitary. Pflugers Arch. 449, 257–264. doi: 10.1007/s00424-004-1337-7
Wang, Y., Li, J., Ying Wang, C., Yan Kwok, A. H., and Leung, F. C. (2007). Epidermal growth factor (EGF) receptor ligands in the chicken ovary: I. Evidence for heparin-binding EGF-like growth factor (HB-EGF) as a potential oocyte-derived signal to control granulosa cell proliferation and HB-EGF and kit ligand expression. Endocrinology 148, 3426–3440. doi: 10.1210/en.2006-1383
Waters, S. T., and Lewandoski, M. (2006). A threshold requirement for Gbx2 levels in hindbrain development. Development 133, 1991–2000. doi: 10.1242/dev.02364
Wen, S., Ai, W., Alim, Z., and Boehm, U. (2010). Embryonic gonadotropin-releasing hormone signaling is necessary for maturation of the male reproductive axis. Proc. Natl. Acad. Sci. U.S.A. 107, 16372–16377. doi: 10.1073/pnas.1000423107
Wu, C., Lv, C., Wan, Y., Li, X., Zhang, J., Li, J., et al. (2019). Arginine vasotocin (AVT)/mesotocin (MT) receptors in chickens: Evidence for the possible involvement of AVT-AVPR1 signaling in the regulation of oviposition and pituitary prolactin expression. Gen. Comp. Endocrinol. 281, 91–104. doi: 10.1016/j.ygcen.2019.05.013
Yavropoulou, M. P., Maladaki, A., and Yovos, J. G. (2015). The role of Notch and Hedgehog signaling pathways in pituitary development and pathogenesis of pituitary adenomas. Hormones (Athens) 14, 5–18. doi: 10.1007/BF03401377
Ye, P., Ge, K., Li, M., Yang, L., Jin, S., Zhang, C., et al. (2019). Egg-laying and brooding stage-specific hormonal response and transcriptional regulation in pituitary of Muscovy duck (Cairina moschata). Poult. Sci. 98, 5287–5296. doi: 10.3382/ps/pez433
Ying, Q. L., Nichols, J., Chambers, I., and Smith, A. (2003). BMP induction of Id proteins suppresses differentiation and sustains embryonic stem cell self-renewal in collaboration with STAT3. Cell 115, 281–292. doi: 10.1016/s0092-8674(03)00847-x
Yoshida, S., Kato, T., Yako, H., Susa, T., Cai, L. Y., Osuna, M., et al. (2011). Significant quantitative and qualitative transition in pituitary stem / progenitor cells occurs during the postnatal development of the rat anterior pituitary. J. Neuroendocrinol. 23, 933–943. doi: 10.1111/j.1365-2826.2011.02198.x
You, S., Foster, L. K., Silsby, J. L., el Halawani, M. E., and Foster, D. N. (1995). Sequence analysis of the turkey LH beta subunit and its regulation by gonadotrophin-releasing hormone and prolactin in cultured pituitary cells. J. Mol. Endocrinol. 14, 117–129. doi: 10.1677/jme.0.0140117
Zhang, J., Li, X., Zhou, Y., Cui, L., Li, J., Wu, C., et al. (2017). The interaction of MC3R and MC4R with MRAP2, ACTH, α-MSH and AgRP in chickens. J. Endocrinol. 234, 155–174. doi: 10.1530/joe-17-0131
Zhang, J., Zhou, Y., Wu, C., Wan, Y., Fang, C., Li, J., et al. (2018). Characterization of the Apelin/Elabela receptors (APLNR) in chickens, turtles, and zebrafish: identification of a novel apelin-specific receptor in teleosts. Front Endocrinol (Lausanne) 9:756. doi: 10.3389/fendo.2018.00756
Zhang, S., Cui, Y., Ma, X., Yong, J., Yan, L., Yang, M., et al. (2020). Single-cell transcriptomics identifies divergent developmental lineage trajectories during human pituitary development. Nat. Commun. 11:5275. doi: 10.1038/s41467-020-19012-4
Zhu, G., Fang, C., Li, J., Mo, C., Wang, Y., and Li, J. (2019). Transcriptomic diversification of granulosa cells during Follicular development in chicken. Sci. Rep. 9:5462. doi: 10.1038/s41598-019-41132-1
Zhu, X., Gleiberman, A. S., and Rosenfeld, M. G. (2007). Molecular physiology of pituitary development: signaling and transcriptional networks. Physiol. Rev. 87, 933–963. doi: 10.1152/physrev.00006.2006
Keywords: chickens, anterior pituitary, endocrine cell, folliculo-stellate cell, scRNA sequencing, gene expression
Citation: Zhang J, Lv C, Mo C, Liu M, Wan Y, Li J and Wang Y (2021) Single-Cell RNA Sequencing Analysis of Chicken Anterior Pituitary: A Bird’s-Eye View on Vertebrate Pituitary. Front. Physiol. 12:562817. doi: 10.3389/fphys.2021.562817
Received: 16 May 2020; Accepted: 21 May 2021;
Published: 29 June 2021.
Edited by:
Krystyna Pierzchala-Koziec, University of Agriculture in Krakow, PolandReviewed by:
Kazuyoshi Ukena, Hiroshima University, JapanMatan Golan, Agricultural Research Organization (ARO), Israel
Copyright © 2021 Zhang, Lv, Mo, Liu, Wan, Li and Wang. This is an open-access article distributed under the terms of the Creative Commons Attribution License (CC BY). The use, distribution or reproduction in other forums is permitted, provided the original author(s) and the copyright owner(s) are credited and that the original publication in this journal is cited, in accordance with accepted academic practice. No use, distribution or reproduction is permitted which does not comply with these terms.
*Correspondence: Yajun Wang, Y2R3eWpoa0BnbWFpbC5jb20=; Juan Li, bGlqdWFuc2N1aGtAMTYzLmNvbQ==
†These authors have contributed equally to this work