- Division of Pulmonary and Critical Care Medicine, Northwestern University, Chicago, IL, United States
Carbon dioxide (CO2) is produced in eukaryotic cells primarily during aerobic respiration, resulting in higher CO2 levels in mammalian tissues than those in the atmosphere. CO2 like other gaseous molecules such as oxygen and nitric oxide, is sensed by cells and contributes to cellular and organismal physiology. In humans, elevation of CO2 levels in tissues and the bloodstream (hypercapnia) occurs during impaired alveolar gas exchange in patients with severe acute and chronic lung diseases. Advances in understanding of the biology of high CO2 effects reveal that the changes in CO2 levels are sensed in cells resulting in specific tissue responses. There is accumulating evidence on the transcriptional response to elevated CO2 levels that alters gene expression and activates signaling pathways with consequences for cellular and tissue functions. The nature of hypercapnia-responsive transcriptional regulation is an emerging area of research, as the responses to hypercapnia in different cell types, tissues, and species are not fully understood. Here, we review the current understanding of hypercapnia effects on gene transcription and consequent cellular and tissue functions.
Introduction
Cells and tissues possess the ability to sense and respond to alterations in the concentration of gaseous molecules. While the understanding of how oxygen (Weir et al., 2005; Cummins et al., 2020) and nitric oxide (Haldar and Stamler, 2013) activate cellular signaling pathways to elicit adaptive responses has been well described, little attention has been given to the mechanisms by which non-excitable cells sense and respond to changes in carbon dioxide (CO2) levels (Shigemura et al., 2017; Cummins et al., 2020). In eukaryotic cells, CO2 is produced during oxidative phosphorylation and physiological CO2 levels in mammalian tissues (∼5%) (Shigemura et al., 2017) are significantly higher than atmospheric levels (∼0.04%)1 (Monastersky, 2013). In humans, severe lung disorders often impair alveolar gas exchange and lead to alveolar hypoventilation resulting in an elevation of CO2 levels in tissues and the bloodstream (hypercapnia) (Shigemura et al., 2017), which affects physiological consequences in the lung and other organs including brain, heart, kidney and skeletal muscles (Barnes et al., 2018). Hypercapnia has been initially proposed to be innocuous or even protective in the lung (Laffey and Kavanagh, 1999; ARDS Network, 2000) and improves outcomes in mechanically ventilated patients with acute lung injury through attenuation of stretch and sheer forces applied to the alveolar wall (volutrauma/barotrauma), cyclic recruitment-derecruitment of atelectatic areas of lung (atelectrauma), and systemic cytokine response (biotrauma) (Slutsky and Ranieri, 2013). The beneficial effects of hypercapnia have been reviewed in detail elsewhere over the past decades (Laffey and Kavanagh, 1999; Contreras et al., 2015). Contrastingly, in recent years, there is accumulating evidence that elevated CO2 conditions are associated with adverse pathophysiological effects on the lung (Doerr et al., 2005; Vadasz et al., 2008; Gates et al., 2013; Shigemura et al., 2018; Bharat et al., 2020) and skeletal muscles (Jaitovich et al., 2015; Korponay et al., 2020). Recent discoveries suggest that high levels of CO2 activate specific signaling pathways with deleterious consequences for organismal functions not only in mammals (Briva et al., 2007; O’Toole et al., 2009; Cummins et al., 2010; Wang et al., 2010; Welch et al., 2010; Vohwinkel et al., 2011; Oliver et al., 2012; Vadasz et al., 2012; Lecuona et al., 2013; Casalino-Matsuda et al., 2015; Dada et al., 2015; Selfridge et al., 2016; Kikuchi et al., 2017), but also the fly Drosophila melanogaster (Helenius et al., 2009; Vadasz et al., 2012), and the nematode Caenorhabditis elegans (Sharabi et al., 2009; Vadasz et al., 2012).
Elevated CO2 has also been reported to affect global gene expression in various cell types, tissues and species (Li et al., 2006; Helenius et al., 2009; Sharabi et al., 2009; Otulakowski et al., 2017; Casalino-Matsuda et al., 2018; Shigemura et al., 2018, 2019). Hypercapnia induces stress responses in animal models (Schaefer et al., 1968; Raff et al., 1983), which can alter gene expression (Zanchi et al., 2010). The increased production of stress hormones in vivo in response to hypercapnia was interpreted as an indirect link between elevated CO2 conditions and gene regulation (Taylor and Cummins, 2011). Increased ventilation stimulated by acute exposure to hypercapnia is also known to affect transcriptional regulations in respiratory muscles (Siafakas et al., 2001). More recent in vitro studies suggest the direct mechanisms by which hypercapnia regulates gene expression in different cell types and species (Helenius et al., 2009; Vohwinkel et al., 2011; Casalino-Matsuda et al., 2015, 2018; Shigemura et al., 2018, 2019; Korponay et al., 2020). However, a systems-level understanding of how hypercapnia effects are integrated into specific signaling pathways, and whether gene programs during hypercapnia are conserved in different cells/tissues and species still remains unclear. Here, we review recent advances in our understanding of hypercapnia-regulated gene transcription and consequent cellular/tissue functions particularly focusing on lung and skeletal muscle functions and innate immune system. We also discuss the global transcriptional response to hypercapnia and conserved alteration in transcription among the different cell types, tissues and species.
Lung
Transcriptomic analyses have been carried out on neonatal and adult mice, as well as human bronchial epithelial cells with different CO2 exposure conditions (Li et al., 2006; Casalino-Matsuda et al., 2018; Shigemura et al., 2018; Bharat et al., 2020). These studies identified changes in the expression of hypercapnia-responsive genes involved in diverse cellular and tissue functions in the lung as described below.
Lung Epithelial Function
Alveolar epithelial repair is critical for patients to recover from lung injury (Berthiaume et al., 1999). Hypercapnia, particularly hypercapnia-associated acidosis, has been proposed to improve outcomes of patients with acute lung injury, acute respiratory distress syndrome (ARDS) and ventilator-induced lung injury (VILI), which triggered the emergence of “permissive” and even “therapeutic” hypercapnia concepts (Hickling et al., 1994; ARDS Network, 2000; Contreras et al., 2015). The protective ventilation strategy was initially linked with the effects of hypercapnic acidosis on the host immune system, with the attenuation of NF-κB activity, a transcription factor that regulates inflammation, injury and repair (Contreras et al., 2015; Shigemura et al., 2017; Cummins et al., 2020). The transcriptional regulatory role of hypercapnia in anti-inflammation has been described and is discussed in detail (including deleterious effects of hypercapnia) below in section “INNATE IMMUNITY”. A transcriptomic study in a mouse model of VILI reported that hypercapnia increased the expression of α-tocopherol transfer protein, which may contribute to the protection afforded by hypercapnia in VILI (Otulakowski et al., 2017). In contrast, a recent article reported that severe hypercapnia was independently associated with higher intensive care unit (ICU) mortality in patients with ARDS (Nin et al., 2017). Delayed lung healing has been reported in patients with alveolo-pleural fistulae which manifests as a prolonged air leak from the lung surface leading to increased morbidity following surgical lung resections (Okereke et al., 2005; Singhal et al., 2010). A recent clinical study reported that intrapleural hypercapnia was associated with delayed resolution of alveolopleural fistulae in patients after thoracic surgery (Bharat et al., 2016). Interestingly, the study also suggested that the reduction of pleural CO2 levels was associated with faster resolution of air leaks. Alveolar epithelial cells play a role in the response to lung injury and in lung repair (Berthiaume et al., 1999; Barkauskas et al., 2013). Both proliferation and migration of alveolar epithelial cells are important for healing of lung injury (Berthiaume et al., 1999). Hypercapnia, independently of acidosis and hypoxia, has been reported to decrease cell proliferation via microRNA (miR) regulation in human alveolar epithelial cells as well as fibroblasts (Vohwinkel et al., 2011). The impaired cell proliferation by hypercapnia was associated with the miR-183-mediated transcriptional downregulation of the tricarboxylic acid cycle enzyme, isocitrate dehydrogenase-2 and consequent mitochondrial dysfunction. In a different model, hypercapnic acidosis was shown to decrease cell migration and wound repair via inhibition of NF-κB in human alveolar and bronchial epithelial cells (O’Toole et al., 2009). More recently, new evidence obtained through a network analysis of hypercapnia-responsive genes suggests that C-X-C motif chemokine 12 (CXCL12) plays a role in cell migration and wound repair in alveolar and airway epithelial cells during normoxic hypercapnia, independently of extracellular pH (Bharat et al., 2020). CXCL12 is secreted after scratch wound by lung epithelial cells (Ghosh et al., 2012) and promotes alveolar epithelial cell migration by regulating the Rac1-GTPase and cofilin activation (Kanter et al., 2015). In this study, hypercapnia decreased CXCL12 at gene expression level via reduced NF-κB activation following the Rac1-GTPase/cofilin pathway in the epithelial cells of mice and humans.
Airway Function
Persistent hypercapnia is associated with increased disease severity and worse prognosis in obstructive lung diseases such as obesity hypoventilation syndrome (Piper, 2015) and chronic obstructive pulmonary disease (COPD) (Connors et al., 1996; Köhnlein et al., 2014; Murphy et al., 2017). More recent reports show that treating hypercapnic COPD patients with noninvasive ventilation aimed at the reduction of CO2 levels improved mortality (Köhnlein et al., 2014; Murphy et al., 2017) thus, supporting the notion that hypercapnia contributes to airway disease pathogenesis. We have reported that in a mouse model chronic hypercapnia increased airway smooth muscle cell contractility (Shigemura et al., 2018). Our gene network analysis suggested the “miR-133a–related RhoA/myosin light chain (MLC) phosphatase-MLC” pathway as the enriched signaling pathway of airway smooth muscle contraction, which was validated by molecular approaches with studies in mice and cell culture systems. Airway remodeling is an important part of the pathophysiology of obstructive respiratory diseases (Hirota and Martin, 2013; Prakash, 2016). It can be associated with excessive extracellular matrix deposition. In a neonatal mouse model, chronic hypercapnia was suggested to activate genes related to the composition of the extracellular matrix such as collagens and laminins (Li et al., 2006). Hypercapnia may also promote airway smooth muscle contractility altering the expression of genes involved in airway remodeling.
Skeletal Muscles
The detrimental effects of hypercapnia are not limited to the lungs. Lung diseases affect suboptimal function of other metabolic organs such as skeletal muscles (Barreiro et al., 2015). Skeletal muscle wasting, an imbalance between protein degradation and synthesis, is frequently observed as a comorbidity in patients with chronic lung diseases such as COPD and correlates with increased morbidity and mortality (Jaitovich and Barreiro, 2018). In a murine model, normoxic hypercapnia has been shown to cause skeletal muscle atrophy via catabolic activation of the AMP-activated kinase (AMPK)/FoxO3a/E3-ubiquitin ligase muscle-specific RING finger protein-1 (MuRF1) signaling axis (Jaitovich et al., 2015). A transcriptomic study of mouse skeletal muscles revealed that normoxic hypercapnia altered the expression of genes involved in biological processes in diaphragm and soleus muscles (Shigemura et al., 2019). More recently, it has been shown to affect ribosomal biogenesis showing a marked reduction of ribosomal 45S pre-RNA in soleus and extensor digitorum longus (EDL) muscles isolated from mice exposed to hypercapnia and cultured myotubes exposed to high CO2 as well as quadriceps muscles from hypercapnic patients (Jaitovich et al., 2015; Korponay et al., 2020). An unbiased proteomic analysis of EDL muscles in chronic hypercapnia-exposed mice also indicated downregulation of components of “translation initiation” and “structural constituent of ribosome,” suggesting high CO2-mediated regulation of protein anabolism (Korponay et al., 2020). In skeletal muscle physiology, the discoveries of miRs have led to further understanding of the transcriptional complexity. MyomiRs, which represent a suite of miRs such as miR-1, miR-133, miR-208 and miR-499, are highly enriched in skeletal muscles and have distinct roles in modulating skeletal muscle proliferation and differentiation as well as the regulation of the skeletal muscle phenotype and performance (Chen et al., 2006; Williams et al., 2009; McCarthy, 2011). Several studies reported that chronic intermittent hypoxia-hypercapnia leads to slow-to-fast muscle fiber shift via either the increase in the expression of miR-1 and miR-133a (Pan et al., 2016) or the decrease in miR-208b and miR-499 (Huang et al., 2016) modulating the expression of their target transcription factors, which results in significant reduced running capacity (Pan et al., 2016).
Innate Immunity
Hypercapnic patients with pulmonary infections have higher ICU admission and mortality (Afessa et al., 1995; Sin et al., 2005; Laserna et al., 2012), suggesting the role of elevated CO2 per se for immune system function. In studies of experimental organism model, normoxic hypercapnia resulted in altered expression of innate immune genes in C. elegans (Sharabi et al., 2009) and D. melanogaster (Helenius et al., 2009), and hypoxia and hypercapnia in Callosobruchus chinensis (Cui et al., 2019). In flies, hypercapnia suppressed expression of specific antimicrobial peptide genes (ex. diptericin) regulated by Relish which is an ortholog of the mammalian NF-κB, and significantly increased the mortality of the flies inoculated with bacteria (Helenius et al., 2009). A genome-wide RNA interference screen in Drosophila S2 cells reported 16 genes including the zinc finger homeodomain zfh2 with human orthologs whose knockdown restored the suppression of diptericin during hypercapnia (Helenius et al., 2016). Interfering with zfh2 in the flies significantly improved survival from Staphylococcus aureus infection, suggesting zfh2 as a critical regulator of immune suppression by hypercapnia. The transcriptomic studies of hypercapnia in mice and humans revealed that normoxic hypercapnia altered the expression of multiple components of the innate immune system (Li et al., 2006; Casalino-Matsuda et al., 2018). Chronic hypercapnia suppressed the expression of inflammatory mediator genes such as interleukins, tumor necrosis factor (TNF) and chemokines (e.g., Cxcl14) in murine neonatal lung (Li et al., 2006). In human bronchial epithelial cells, hypercapnic acidosis also downregulated the gene expression of interleukin six (IL-6) receptor and chemokines (e.g., CXCL14) (Casalino-Matsuda et al., 2018). CXCL14, C-X-C motif chemokine ligand 14, is conserved between species and possesses chemoattractive activity for activated macrophages and natural killer cells (Hara and Tanegashima, 2012). In immune cells, high CO2 conditions selectively inhibit the gene expression of IL-6 and TNF, and decreases phagocytosis in macrophages including mouse and human alveolar macrophages (Wang et al., 2010). Furthermore, it attenuates Beclin 1 activity by increased expression of anti-apoptotic genes, BCL2 and Bcl-xL, in human macrophages, and inhibits autophagy and macrophage killing of bacteria (Casalino-Matsuda et al., 2015). Hypercapnia was reported to promote anti-inflammatory and immunosuppressive functions via activation of the non-canonical NF-κB component IKKα/RelB/p100 while inhibiting the canonical NF-κB pathway that activates host defense genes (Cummins et al., 2010; Oliver et al., 2012; Keogh et al., 2017). The hypercapnia-mediated immune suppression (impaired regulation of cytokine genes, phagocytosis and autophagy, and NF-κB signaling) occurred independently of hypoxia and pH changes. A transcriptomic study of peripheral blood in elite divers reported alterations of leukocyte gene expression profiles in response to freediving (Eftedal et al., 2016) which can cause acute hypoxia and hypercapnia (Overgaard et al., 2006). Interestingly, deconvolution of transcriptomes indicated a temporary decrease of CD8+ T cells and resting natural killer cells in response to acute hypoxia and hypercapnia. Furthermore, biological pathway analysis showed downregulation of genes coding for components of granule-mediated lymphocyte cytotoxicity.
Global Transcriptional Response to Hypercapnia
We have recently reported a comparative transcriptomic study of hypercapnia (Shigemura et al., 2019) to investigate the interaction/integration/conservation of genes combining multi-tissue microarray analysis in mice with secondary analysis of available datasets in human bronchial epithelial cells (Casalino-Matsuda et al., 2018), D. melanogaster (Helenius et al., 2009) and C. elegans (Sharabi et al., 2009). We found that normoxic hypercapnia transiently increased particularly Wnt ligand and Frizzled genes in lungs and skeletal muscles of mice and in several cell lines of different tissue origin, and activated Wnt pathway homologs, which was also observed in the human bronchial cells, flies and nematodes, suggesting an evolutionarily conserved role of elevated CO2 in regulating Wnt signaling pathways. The Wnt signal plays a critical role in diverse biological processes and is part of a highly conserved pathway in animals (Abiola et al., 2009; Sethi and Vidal-Puig, 2010). The Wnts activate at least two distinct intracellular pathways, canonical Wnt/β-catenin or non-canonical β-catenin-independent (Sethi and Vidal-Puig, 2010). The canonical Wnt signal activates β-catenin-responsive target genes via cytosolic and nuclear β-catenin accumulation. The non-canonical signals are characterized as the calcium/calmodulin-dependent kinase II (CaMKII)-mediated Ca2+ signaling pathway and planar cell polarity pathway via the activation of small GTPase RhoA and Jun N-terminal kinase (JNK). The Wnts are also suggested to activate the metabolic sensor AMPK in myotubes (Abiola et al., 2009) and MuRF-1 in muscle atrophy (Rajasekaran et al., 2017). Intriguingly, we have reported that CaMKII (Vadasz et al., 2008), RhoA (Shigemura et al., 2018), JNK (Vadasz et al., 2012), AMPK (Vadasz et al., 2008; Welch et al., 2010; Jaitovich et al., 2015; Bharat et al., 2020) and MuRF1 (Jaitovich et al., 2015) are responsive to hypercapnia in the above-mentioned pathophysiological contexts. In the large-scale transcriptomic study, we inferred several potentially regulatory transcription factors for the hypercapnia-responsive genes, which are conserved amongst lung and skeletal muscle tissues (Figure 1). Some of the transcription factors, for example c-Myc (Dong et al., 2017; Zhao et al., 2017) and c-Jun (Zhao et al., 2017), are the target genes of the Wnt signaling. Transcriptional regulation of Wnt pathway genes might be of critical importance in the systems-level understanding of hypercapnia effects in organisms.
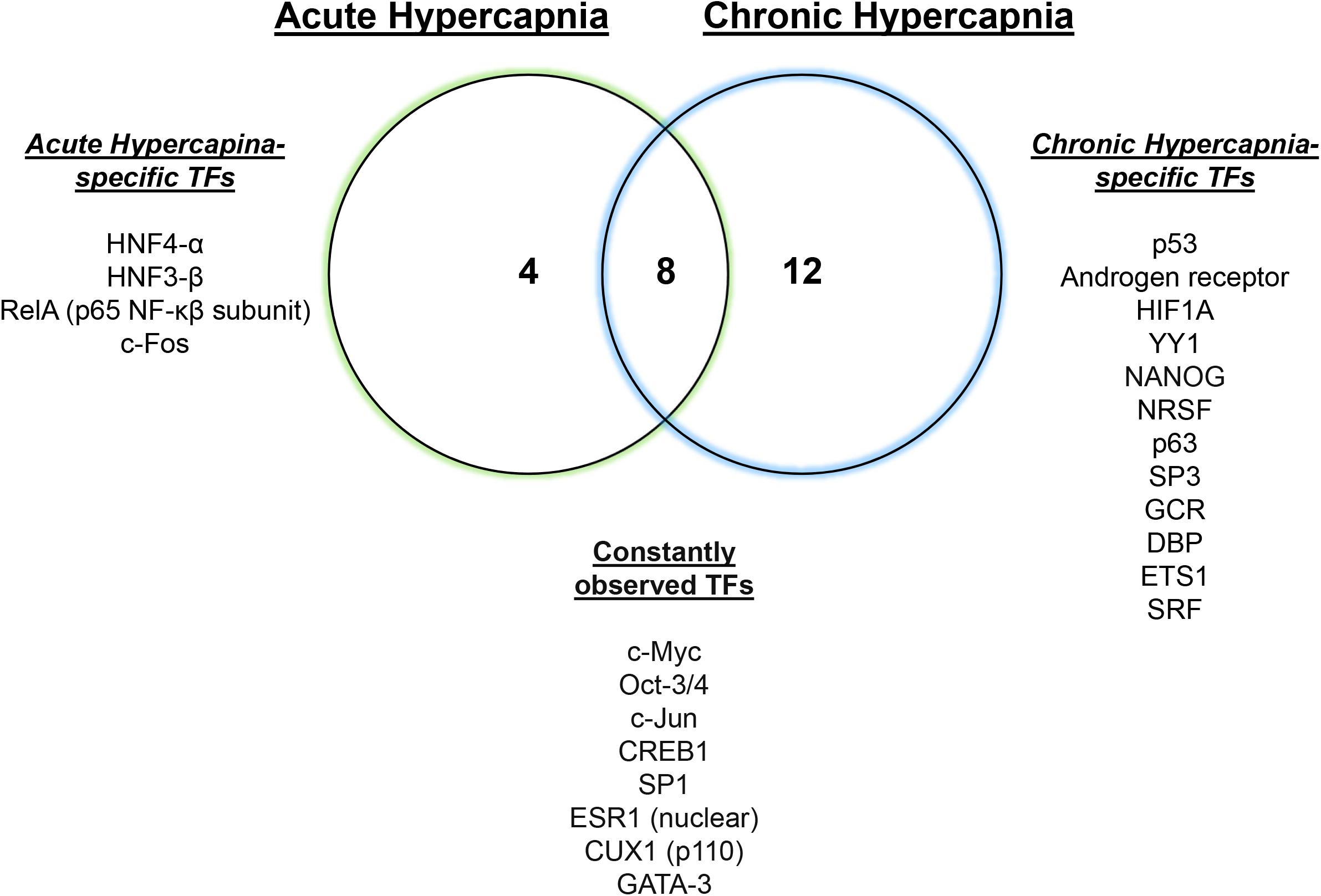
Figure 1. Hypercapnia-responsive transcription factors conserved in mouse tissues. Twelve or twenty hypercapnia-responsive transcription factors (TFs) were observed during acute or chronic hypercapnia conditions in mouse lung, diaphragm and soleus, respectively. Eight conserved TFs consistently inferred gene expression signatures in the tissues during hypercapnia. Modified from Shigemura et al. (2019).
Conclusion
Cells, tissues and organisms possess a complex transcriptional program that selectively promotes certain genes while simultaneously attenuating translational activity in response to elevated CO2 levels (Figure 2). It has become evident that some of the hypercapnia-responsive genes, particularly involved in innate immune system and Wnt pathways, is evolutionarily conserved in different cell types, tissues and species. The changes in gene expression during hypercapnia appear to be mostly maladaptive in the lung, skeletal muscle and innate immune functions, which likely underlies the negative effects of elevated CO2 in patients with lung diseases. Our understanding of the transcriptional regulatory role of hypercapnia in organisms is still limited, but much research is being conducted in order to identify molecular CO2 sensing and downstream effects. Additional research is warranted to identify transcriptional regulators and how these regulators interact in physiological and pathophysiological contexts in hypercapnia.
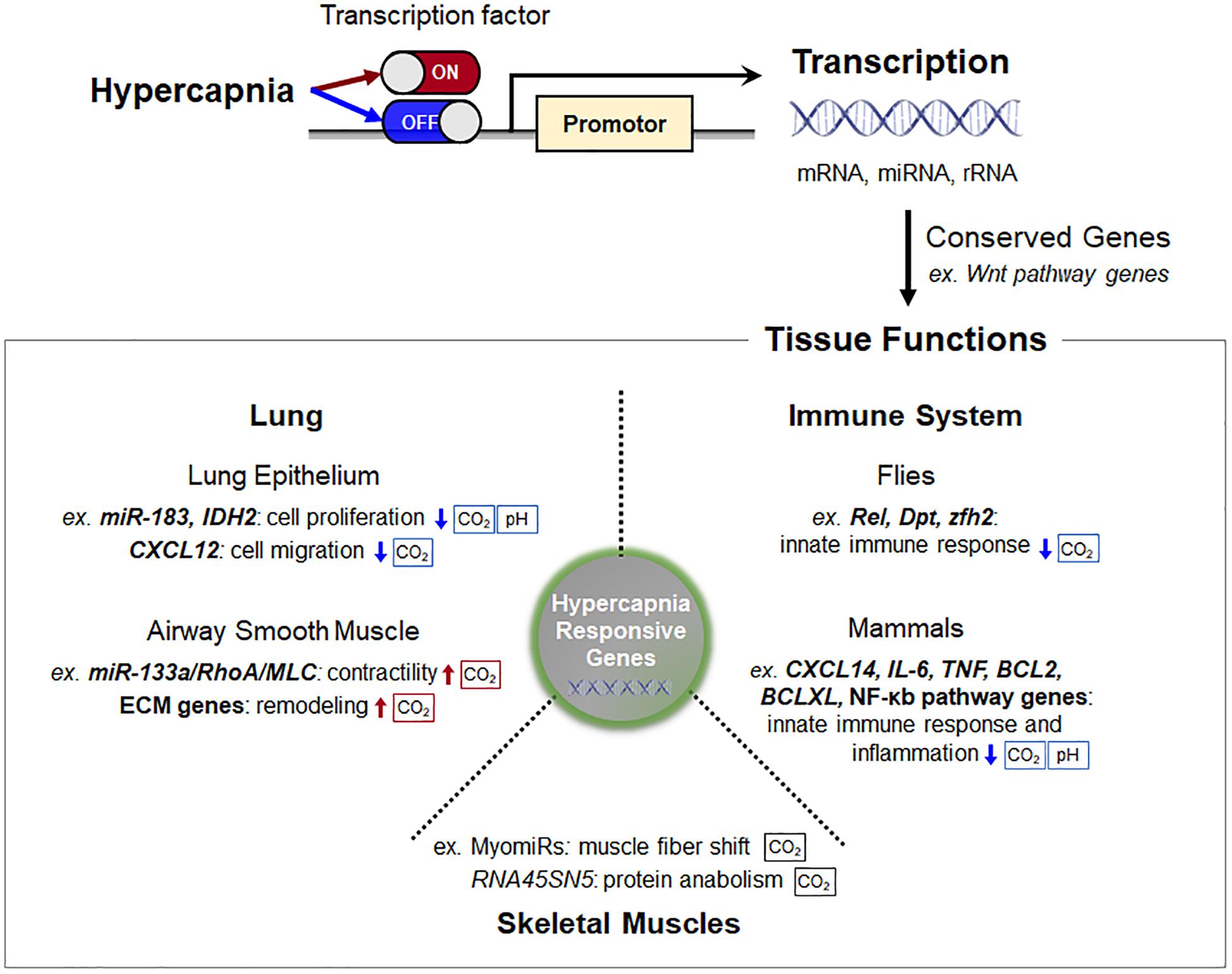
Figure 2. Schematic of transcription regulation and consequent tissue functions in hypercapnia. Cells, tissues, and organisms possess a complex transcriptional program that selectively promotes certain genes while simultaneously attenuating translational activity in response to elevated CO2 levels. Some of the hypercapnia-responsive genes, particularly Wnt pathway genes, are evolutionarily conserved in different cell types, tissues, and species. The sensing pathways that are pH-dependent are indicated. Abbreviations: IDH2, isocitrate dehydrogenase-2; CXCL12, C-X-C motif chemokine 12; MLC, myosin light chain; ECM, extracellular matrix; RNA45SN45, ribosomal 45S pre-RNA; Rel, Relish; Dpt, diptericin; zfh2, Zn finger homeodomain 2; TNF, tumor necrosis factor.
Author Contributions
MS, LW, and JS conceived, designed the review and wrote the manuscript. All authors read and approved the manuscript.
Funding
This review was supported in part by the United States National Institutes of Health (HL-147070 and HL-071643).
Conflict of Interest
The authors declare that the research was conducted in the absence of any commercial or financial relationships that could be construed as a potential conflict of interest.
The reviewer CT declared a past co-authorship with one of the authors, JS, to the handling editor.
Footnotes
References
Abiola, M., Favier, M., Christodoulou-Vafeiadou, E., Pichard, A. L., Martelly, I., and Guillet-Deniau, I. (2009). Activation of Wnt/beta-catenin signaling increases insulin sensitivity through a reciprocal regulation of Wnt10b and SREBP-1c in skeletal muscle cells. PLoS One 4:e8509. doi: 10.1371/journal.pone.0008509
Afessa, B., Greaves, W. L., and Frederick, W. R. (1995). Pneumococcal bacteremia in adults: a 14-year experience in an inner-city university hospital. Clin. Infect. Dis. 21, 345–351. doi: 10.1093/clinids/21.2.345
ARDS Network (2000). Ventilation with lower tidal volumes as compared with traditional tidal volumes for acute lung injury and the acute respiratory distress syndrome. The acute respiratory distress syndrome network. N. Engl. J. Med. 342, 1301–1308. doi: 10.1056/nejm200005043421801
Barkauskas, C. E., Cronce, M. J., Rackley, C. R., Bowie, E. J., Keene, D. R., Stripp, B. R., et al. (2013). Type 2 alveolar cells are stem cells in adult lung. J. Clin. Invest. 123, 3025–3036.
Barnes, T., Zochios, V., and Parhar, K. (2018). Re-examining permissive hypercapnia in ARDS: a narrative review. Chest 154, 185–195. doi: 10.1016/j.chest.2017.11.010
Barreiro, E., Sznajder, J. I., Nader, G. A., and Budinger, G. R. (2015). Muscle dysfunction in patients with lung diseases: a growing epidemic. Am. J. Respir. Crit. Care Med. 191, 616–619. doi: 10.1164/rccm.201412-2189oe
Berthiaume, Y., Lesur, O., and Dagenais, A. (1999). Treatment of adult respiratory distress syndrome: plea for rescue therapy of the alveolar epithelium. Thorax 54, 150–160. doi: 10.1136/thx.54.2.150
Bharat, A., Angulo, M., Sun, H., Akbarpour, M., Alberro, A., Cheng, Y., et al. (2020). High CO2 levels impair lung wound healing. Am. J. Respir. Cell Mol. Biol. 63, 244–254. doi: 10.1165/rcmb.2019-0354oc
Bharat, A., Graf, N., Mullen, A., Kanter, J., Andrei, A. C., Sporn, P. H., et al. (2016). Pleural hypercarbia after lung surgery is associated with persistent alveolopleural fistulae. Chest 149, 220–227. doi: 10.1378/chest.15-1591
Briva, A., Vadasz, I., Lecuona, E., Welch, L. C., Chen, J., Dada, L. A., et al. (2007). High CO2 levels impair alveolar epithelial function independently of pH. PLoS One 2:e1238. doi: 10.1371/journal.pone.0001238
Casalino-Matsuda, S. M., Nair, A., Beitel, G. J., Gates, K. L., and Sporn, P. H. (2015). Hypercapnia inhibits autophagy and bacterial killing in human macrophages by increasing expression of Bcl-2 and Bcl-xL. J. Immunol. 194, 5388–5396. doi: 10.4049/jimmunol.1500150
Casalino-Matsuda, S. M., Wang, N., Ruhoff, P. T., Matsuda, H., Nlend, M. C., Nair, A., et al. (2018). Hypercapnia alters expression of immune response, nucleosome assembly and lipid metabolism genes in differentiated human bronchial epithelial cells. Sci. Rep. 8:13508.
Chen, J. F., Mandel, E. M., Thomson, J. M., Wu, Q., Callis, T. E., Hammond, S. M., et al. (2006). The role of microRNA-1 and microRNA-133 in skeletal muscle proliferation and differentiation. Nat. Genet. 38, 228–233. doi: 10.1038/ng1725
Connors, A. F., Dawson, N. V., Thomas, C., Harrell, F. E., Desbiens, N., Fulkerson, W. J., et al. (1996). Outcomes following acute exacerbation of severe chronic obstructive lung disease. The SUPPORT investigators (study to understand prognoses and preferences for outcomes and risks of treatments). Am. J. Respir. Crit. Care Med. 154, 959–967. doi: 10.1164/ajrccm.154.4.8887592
Contreras, M., Masterson, C., and Laffey, J. G. (2015). Permissive hypercapnia: what to remember. Curr. Opin. Anaesthesiol. 28, 26–37. doi: 10.1097/aco.0000000000000151
Cui, S. F., Wang, L., Ma, L., Wang, Y. L., Qiu, J. P., Liu, Z. C., et al. (2019). Comparative transcriptome analyses of adzuki bean weevil (Callosobruchus chinensis) response to hypoxia and hypoxia/hypercapnia. Bull. Entomol. Res. 109, 266–277. doi: 10.1017/s0007485318000512
Cummins, E. P., Oliver, K. M., Lenihan, C. R., Fitzpatrick, S. F., Bruning, U., Scholz, C. C., et al. (2010). NF-kappaB links CO2 sensing to innate immunity and inflammation in mammalian cells. J. Immunol. 185, 4439–4445. doi: 10.4049/jimmunol.1000701
Cummins, E. P., Strowitzki, M. J., and Taylor, C. T. (2020). Mechanisms and consequences of oxygen and carbon dioxide sensing in mammals. Physiol. Rev. 100, 463–488. doi: 10.1152/physrev.00003.2019
Dada, L. A., Trejo Bittar, H. E., Welch, L. C., Vagin, O., Deiss-Yehiely, N., Kelly, A. M., et al. (2015). High CO2 leads to Na,K-ATPase endocytosis via c-Jun amino-terminal kinase-induced LMO7b phosphorylation. Mol. Cell. Biol. 35, 3962–3973. doi: 10.1128/mcb.00813-15
Doerr, C. H., Gajic, O., Berrios, J. C., Caples, S., Abdel, M., Lymp, J. F., et al. (2005). Hypercapnic acidosis impairs plasma membrane wound resealing in ventilator-injured lungs. Am. J. Respir. Crit. Care Med. 171, 1371–1377. doi: 10.1164/rccm.200309-1223oc
Dong, L., Hao, H., Liu, J., Ti, D., Tong, C., Hou, Q., et al. (2017). A conditioned medium of umbilical cord mesenchymal stem cells overexpressing Wnt7a promotes wound repair and regeneration of hair follicles in mice. Stem Cells Int. 2017:3738071.
Eftedal, I., Flatberg, A., Drvis, I., and Dujic, Z. (2016). Immune and inflammatory responses to freediving calculated from leukocyte gene expression profiles. Physiol. Genomics 48, 795–802. doi: 10.1152/physiolgenomics.00048.2016
Gates, K. L., Howell, H. A., Nair, A., Vohwinkel, C. U., Welch, L. C., Beitel, G. J., et al. (2013). Hypercapnia impairs lung neutrophil function and increases mortality in murine Pseudomonas pneumonia. Am. J. Respir. Cell Mol. Biol. 49, 821–828. doi: 10.1165/rcmb.2012-0487oc
Ghosh, M. C., Makena, P. S., Gorantla, V., Sinclair, S. E., and Waters, C. M. (2012). CXCR4 regulates migration of lung alveolar epithelial cells through activation of Rac1 and matrix metalloproteinase-2. Am. J. Physiol. Lung Cell. Mol. Physiol. 302, L846–L856.
Haldar, S. M., and Stamler, J. S. (2013). S-nitrosylation: integrator of cardiovascular performance and oxygen delivery. J. Clin. Invest. 123, 101–110. doi: 10.1172/jci62854
Hara, T., and Tanegashima, K. (2012). Pleiotropic functions of the CXC-type chemokine CXCL14 in mammals. J. Biochem. 151, 469–476. doi: 10.1093/jb/mvs030
Helenius, I. T., Haake, R. J., Kwon, Y. J., Hu, J. A., Krupinski, T., Casalino-Matsuda, S. M., et al. (2016). Identification of Drosophila Zfh2 as a mediator of hypercapnic immune regulation by a genome-wide RNA interference screen. J. Immunol. 196, 655–667. doi: 10.4049/jimmunol.1501708
Helenius, I. T., Krupinski, T., Turnbull, D. W., Gruenbaum, Y., Silverman, N., Johnson, E. A., et al. (2009). Elevated CO2 suppresses specific Drosophila innate immune responses and resistance to bacterial infection. Proc. Natl. Acad. Sci. U.S.A. 106, 18710–18715. doi: 10.1073/pnas.0905925106
Hickling, K. G., Walsh, J., Henderson, S., and Jackson, R. (1994). Low mortality rate in adult respiratory distress syndrome using low-volume, pressure-limited ventilation with permissive hypercapnia: a prospective study. Crit. Care Med. 22, 1568–1578. doi: 10.1097/00003246-199422100-00011
Hirota, N., and Martin, J. G. (2013). Mechanisms of airway remodeling. Chest 144, 1026–1032. doi: 10.1378/chest.12-3073
Huang, S., Jin, L., Shen, J., Shang, P., Jiang, X., and Wang, X. (2016). Electrical stimulation influences chronic intermittent hypoxia-hypercapnia induction of muscle fibre transformation by regulating the microRNA/Sox6 pathway. Sci. Rep. 6:26415.
Jaitovich, A., Angulo, M., Lecuona, E., Dada, L. A., Welch, L. C., Cheng, Y., et al. (2015). High CO2 levels cause skeletal muscle atrophy via AMP-activated kinase (AMPK), FoxO3a protein, and muscle-specific Ring finger protein 1 (MuRF1). J. Biol. Chem. 290, 9183–9194. doi: 10.1074/jbc.m114.625715
Jaitovich, A., and Barreiro, E. (2018). Skeletal muscle dysfunction in chronic obstructive pulmonary disease. what we know and can do for our patients. Am. J. Respir. Crit. Care Med. 198, 175–186. doi: 10.1164/rccm.201710-2140ci
Kanter, J. A., Sun, H., Chiu, S., Decamp, M. M., Sporn, P. H., Sznajder, J. I., et al. (2015). Decreased CXCL12 is associated with impaired alveolar epithelial cell migration and poor lung healing after lung resection. Surgery 158, 1073–1080; discussion 1080–1082.
Keogh, C. E., Scholz, C. C., Rodriguez, J., Selfridge, A. C., Von Kriegsheim, A., and Cummins, E. P. (2017). Carbon dioxide-dependent regulation of NF-kappaB family members RelB and p100 gives molecular insight into CO2-dependent immune regulation. J. Biol. Chem. 292, 11561–11571. doi: 10.1074/jbc.m116.755090
Kikuchi, R., Tsuji, T., Watanabe, O., Yamaguchi, K., Furukawa, K., Nakamura, H., et al. (2017). Hypercapnia accelerates adipogenesis: a novel role of high CO2 in exacerbating obesity. Am. J. Respir. Cell Mol. Biol. 57, 570–580. doi: 10.1165/rcmb.2016-0278oc
Köhnlein, T., Windisch, W., Köhler, D., Drabik, A., Geiseler, J., Hartl, S., et al. (2014). Non-invasive positive pressure ventilation for the treatment of severe stable chronic obstructive pulmonary disease: a prospective, multicentre, randomised, controlled clinical trial. Lancet Respir. Med. 2, 698–705. doi: 10.1016/s2213-2600(14)70153-5
Korponay, T. C., Balnis, J., Vincent, C. E., Singer, D. V., Chopra, A., Adam, A. P., et al. (2020). High CO2 downregulates skeletal muscle protein anabolism via AMP-activated protein kinase alpha2-mediated depressed ribosomal biogenesis. Am. J. Respir. Cell Mol. Biol. 62, 74–86. doi: 10.1165/rcmb.2019-0061oc
Laffey, J. G., and Kavanagh, B. P. (1999). Carbon dioxide and the critically ill–too little of a good thing? Lancet 354, 1283–1286. doi: 10.1016/s0140-6736(99)02388-0
Laserna, E., Sibila, O., Aguilar, P. R., Mortensen, E. M., Anzueto, A., Blanquer, J. M., et al. (2012). Hypocapnia and hypercapnia are predictors for ICU admission and mortality in hospitalized patients with community-acquired pneumonia. Chest 142, 1193–1199. doi: 10.1378/chest.12-0576
Lecuona, E., Sun, H., Chen, J., Trejo, H. E., Baker, M. A., and Sznajder, J. I. (2013). Protein kinase A-Ialpha regulates Na,K-ATPase endocytosis in alveolar epithelial cells exposed to high CO(2) concentrations. Am. J. Respir. Cell Mol. Biol. 48, 626–634. doi: 10.1165/rcmb.2012-0373oc
Li, G., Zhou, D., Vicencio, A. G., Ryu, J., Xue, J., Kanaan, A., et al. (2006). Effect of carbon dioxide on neonatal mouse lung: a genomic approach. J. Appl. Physiol. 101, 1556–1564. doi: 10.1152/japplphysiol.01031.2005
McCarthy, J. J. (2011). The MyomiR network in skeletal muscle plasticity. Exerc. Sport Sci. Rev. 39, 150–154. doi: 10.1097/jes.0b013e31821c01e1
Monastersky, R. (2013). Global carbon dioxide levels near worrisome milestone. Nature 497, 13–14. doi: 10.1038/497013a
Murphy, P. B., Rehal, S., Arbane, G., Bourke, S., Calverley, P. M. A., Crook, A. M., et al. (2017). Effect of home noninvasive ventilation with oxygen therapy vs oxygen therapy alone on hospital readmission or death after an acute COPD exacerbation: a randomized clinical trial. JAMA 317, 2177–2186. doi: 10.1001/jama.2017.4451
Nin, N., Muriel, A., Penuelas, O., Brochard, L., Lorente, J. A., Ferguson, N. D., et al. (2017). Severe hypercapnia and outcome of mechanically ventilated patients with moderate or severe acute respiratory distress syndrome. Intensive Care Med. 43, 200–208.
Okereke, I., Murthy, S. C., Alster, J. M., Blackstone, E. H., and Rice, T. W. (2005). Characterization and importance of air leak after lobectomy. Ann. Thorac. Surg. 79, 1167–1173. doi: 10.1016/j.athoracsur.2004.08.069
Oliver, K. M., Lenihan, C. R., Bruning, U., Cheong, A., Laffey, J. G., Mcloughlin, P., et al. (2012). Hypercapnia induces cleavage and nuclear localization of RelB protein, giving insight into CO2 sensing and signaling. J. Biol. Chem. 287, 14004–14011. doi: 10.1074/jbc.m112.347971
O’Toole, D., Hassett, P., Contreras, M., Higgins, B. D., Mckeown, S. T., Mcauley, D. F., et al. (2009). Hypercapnic acidosis attenuates pulmonary epithelial wound repair by an NF-kappaB dependent mechanism. Thorax 64, 976–982. doi: 10.1136/thx.2008.110304
Otulakowski, G., Engelberts, D., Arima, H., Hirate, H., Bayir, H., Post, M., et al. (2017). alpha-Tocopherol transfer protein mediates protective hypercapnia in murine ventilator-induced lung injury. Thorax 72, 538–549. doi: 10.1136/thoraxjnl-2016-209501
Overgaard, K., Friis, S., Pedersen, R. B., and Lykkeboe, G. (2006). Influence of lung volume, glossopharyngeal inhalation and P(ET) O2 and P(ET) CO2 on apnea performance in trained breath-hold divers. Eur. J. Appl. Physiol. 97, 158–164. doi: 10.1007/s00421-006-0156-2
Pan, L. L., Ke, J. Q., Zhao, C. C., Huang, S. Y., Shen, J., Jiang, X. X., et al. (2016). Electrical stimulation improves rat muscle dysfunction caused by chronic intermittent hypoxia-hypercapnia via regulation of miRNA-related signaling pathways. PLoS One 11:e0152525. doi: 10.1371/journal.pone.0152525
Piper, A. (2015). Obesity hypoventilation syndrome: weighing in on therapy options. Chest 149, 856–868.
Prakash, Y. S. (2016). Emerging concepts in smooth muscle contributions to airway structure and function: implications for health and disease. Am. J. Physiol. Lung Cell. Mol. Physiol. 311, L1113–L1140.
Raff, H., Shinsako, J., Keil, L. C., and Dallman, M. F. (1983). Vasopressin, ACTH, and corticosteroids during hypercapnia and graded hypoxia in dogs. Am. J. Physiol. 244, E453–E458.
Rajasekaran, M. R., Kanoo, S., Fu, J., Nguyen, M. L., Bhargava, V., and Mittal, R. K. (2017). Age-related external anal sphincter muscle dysfunction and fibrosis: possible role of Wnt/beta-catenin signaling pathways. Am. J. Physiol. Gastrointest. Liver Physiol. 313, G581–G588.
Schaefer, K. E., Mccabe, N., and Withers, J. (1968). Stress response in chronic hypercapnia. Am. J. Physiol. 214, 543–548. doi: 10.1152/ajplegacy.1968.214.3.543
Selfridge, A. C., Cavadas, M. A., Scholz, C. C., Campbell, E. L., Welch, L. C., Lecuona, E., et al. (2016). Hypercapnia suppresses the HIF-dependent adaptive response to hypoxia. J. Biol. Chem. 291, 11800–11808. doi: 10.1074/jbc.m116.713941
Sethi, J. K., and Vidal-Puig, A. (2010). Wnt signalling and the control of cellular metabolism. Biochem. J. 427, 1–17. doi: 10.1042/bj20091866
Sharabi, K., Hurwitz, A., Simon, A. J., Beitel, G. J., Morimoto, R. I., Rechavi, G., et al. (2009). Elevated CO2 levels affect development, motility, and fertility and extend life span in Caenorhabditis elegans. Proc. Natl. Acad. Sci. U.S.A. 106, 4024–4029. doi: 10.1073/pnas.0900309106
Shigemura, M., Lecuona, E., Angulo, M., Dada, L. A., Edwards, M. B., Welch, L. C., et al. (2019). Elevated CO2 regulates the Wnt signaling pathway in mammals, Drosophila melanogaster and Caenorhabditis elegans. Sci. Rep. 9:18251.
Shigemura, M., Lecuona, E., Angulo, M., Homma, T., Rodriguez, D. A., Gonzalez-Gonzalez, F. J., et al. (2018). Hypercapnia increases airway smooth muscle contractility via caspase-7-mediated miR-133a-RhoA signaling. Sci. Transl. Med. 10:eaat1662. doi: 10.1126/scitranslmed.aat1662
Shigemura, M., Lecuona, E., and Sznajder, J. I. (2017). Effects of hypercapnia on the lung. J. Physiol. 595, 2431–2437. doi: 10.1113/jp273781
Siafakas, N. M., Jordan, M., Wagner, H., Breen, E. C., Benoit, H., and Wagner, P. D. (2001). Diaphragmatic angiogenic growth factor mRNA responses to increased ventilation caused by hypoxia and hypercapnia. Eur. Respir. J. 17, 681–687. doi: 10.1183/09031936.01.17406810
Sin, D. D., Man, S. F., and Marrie, T. J. (2005). Arterial carbon dioxide tension on admission as a marker of in-hospital mortality in community-acquired pneumonia. Am. J. Med. 118, 145–150. doi: 10.1016/j.amjmed.2004.10.014
Singhal, S., Ferraris, V. A., Bridges, C. R., Clough, E. R., Mitchell, J. D., Fernando, H. C., et al. (2010). Management of alveolar air leaks after pulmonary resection. Ann. Thorac. Surg. 89, 1327–1335. doi: 10.1016/j.athoracsur.2009.09.020
Slutsky, A. S., and Ranieri, V. M. (2013). Ventilator-induced lung injury. N. Engl. J. Med. 369, 2126–2136.
Taylor, C. T., and Cummins, E. P. (2011). Regulation of gene expression by carbon dioxide. J. Physiol. 589, 797–803. doi: 10.1113/jphysiol.2010.201467
Vadasz, I., Dada, L. A., Briva, A., Helenius, I. T., Sharabi, K., Welch, L. C., et al. (2012). Evolutionary conserved role of c-Jun-N-terminal kinase in CO2-induced epithelial dysfunction. PLoS One 7:e46696. doi: 10.1371/journal.pone.0046696
Vadasz, I., Dada, L. A., Briva, A., Trejo, H. E., Welch, L. C., Chen, J., et al. (2008). AMP-activated protein kinase regulates CO2-induced alveolar epithelial dysfunction in rats and human cells by promoting Na,K-ATPase endocytosis. J. Clin. Invest. 118, 752–762.
Vohwinkel, C. U., Lecuona, E., Sun, H., Sommer, N., Vadász, I., Chandel, N. S., et al. (2011). Elevated CO(2) levels cause mitochondrial dysfunction and impair cell proliferation. J. Biol. Chem. 286, 37067–37076. doi: 10.1074/jbc.m111.290056
Wang, N., Gates, K. L., Trejo, H., Favoreto, S. Jr., Schleimer, R. P., Sznajder, J. I., et al. (2010). Elevated CO2 selectively inhibits interleukin-6 and tumor necrosis factor expression and decreases phagocytosis in the macrophage. FASEB J. 24, 2178–2190. doi: 10.1096/fj.09-136895
Weir, E. K., Lopez-Barneo, J., Buckler, K. J., and Archer, S. L. (2005). Acute oxygen-sensing mechanisms. N. Engl. J. Med. 353, 2042–2055. doi: 10.1056/nejmra050002
Welch, L. C., Lecuona, E., Briva, A., Trejo, H. E., Dada, L. A., and Sznajder, J. I. (2010). Extracellular signal-regulated kinase (ERK) participates in the hypercapnia-induced Na,K-ATPase downregulation. FEBS Lett. 584, 3985–3989. doi: 10.1016/j.febslet.2010.08.002
Williams, A. H., Liu, N., Van Rooij, E., and Olson, E. N. (2009). MicroRNA control of muscle development and disease. Curr. Opin. Cell Biol. 21, 461–469. doi: 10.1016/j.ceb.2009.01.029
Zanchi, N. E., Filho, M. A., Felitti, V., Nicastro, H., Lorenzeti, F. M., and Lancha, A. H. Jr. (2010). Glucocorticoids: extensive physiological actions modulated through multiple mechanisms of gene regulation. J. Cell. Physiol. 224, 311–315. doi: 10.1002/jcp.22141
Keywords: carbon dioxide, hypercapnia, transcriptional regulation, cellular and tissue function, lung, skeletal muscles, innate immune system
Citation: Shigemura M, Welch LC and Sznajder JI (2020) Hypercapnia Regulates Gene Expression and Tissue Function. Front. Physiol. 11:598122. doi: 10.3389/fphys.2020.598122
Received: 23 August 2020; Accepted: 26 October 2020;
Published: 20 November 2020.
Edited by:
Peter Markus Spieth, University Hospital Carl Gustav Carus, GermanyReviewed by:
Vincent Joseph, Laval University, CanadaMatt Robert Hodges, Medical College of Wisconsin, United States
Cormac Taylor, University College Dublin, Ireland
Copyright © 2020 Shigemura, Welch and Sznajder. This is an open-access article distributed under the terms of the Creative Commons Attribution License (CC BY). The use, distribution or reproduction in other forums is permitted, provided the original author(s) and the copyright owner(s) are credited and that the original publication in this journal is cited, in accordance with accepted academic practice. No use, distribution or reproduction is permitted which does not comply with these terms.
*Correspondence: Jacob I. Sznajder, ai1zem5hamRlckBub3J0aHdlc3Rlcm4uZWR1