- 1Universitätsmedizin Göttingen, Klinik für Anästhesiologie, Georg-August-Universität, Göttingen, Germany
- 2DONAUISAR Klinikum Deggendorf, Deggendorf, Germany
Acute respiratory distress syndrome (ARDS) represents an acute diffuse inflammation of the lungs triggered by different causes, uniformly leading to a noncardiogenic pulmonary edema with inhomogeneous densities in lung X-ray and lung CT scan and acute hypoxemia. Edema formation results in “heavy” lungs, inducing loss of compliance and the need to spend more energy to “move” the lungs. Consequently, an ARDS patient, as long as the patient is breathing spontaneously, has an increased respiratory drive to ensure adequate oxygenation and CO2 removal. One would expect that, once the blood gases get back to “physiological” values, the respiratory drive would normalize and the breathing effort return to its initial status. However, in many ARDS patients, this is not the case; their respiratory drive appears to be upregulated and fully or at least partially detached from the blood gas status. Strikingly, similar alteration of the respiratory drive can be seen in patients suffering from SARS, especially SARS-Covid-19. We hypothesize that alterations of the renin-angiotensin-system (RAS) related to the pathophysiology of ARDS and SARS are involved in this dysregulation of chemosensitive control of breathing.
Introduction
Per definition, acute respiratory distress syndrome (ARDS) is characterized by an inhomogeneously distributed, noncardiogenic pulmonary edema and acute hypoxemia. Its presence is still associated with a high mortality. ARDS is triggered by various stimuli, such as sepsis, major trauma, and pneumonia. The underlying pathophysiology involves activation of the immune system, pneumocyte injury, surfactant dysfunction, and coagulopathies. It markedly impairs adequate exchange and consecutively oxygenation and carbon dioxide removal (Balibrea and Arias-Diaz, 2003; Ranieri et al., 2012; Fanelli and Ranieri, 2015). Patients with ARDS may present with alterations of the breathing pattern, and its regulation might not directly correlate with the O2 or CO2 partial pressures measured in the arterial blood (Spinelli et al., 2020). Of note, despite normalizing arterial pO2 and pCO2 by mechanical ventilation and/or extracorporeal lung support, patients might still present with respiratory rates far higher than expected or needed (Crotti et al., 2017). These patients might require high doses of sedation or even muscle relaxants and controlled ventilation to prevent patient self-inflicted lung injury (P-SILI). Interestingly, in acute cases of COVID-19 pneumonia (SARS), similar observations were made. Despite normalization of the arterial blood gases, COVID-19 patients continued to show forced breathing patterns that might additionally harm the already virus-altered lungs (Cruces et al., 2020; de Vries et al., 2020; Li et al., 2020; Marini and Gattinoni, 2020; Smit et al., 2020).
In this hypothesis and theory paper, we discuss potential mechanisms that might disturb respiratory chemosensitivity in patients with ARDS or SARS.
The Renin-Angiotensin-System in ARDS
The renin-angiotensin-system (RAS; Figure 1) or renin-angiotensin-aldosterone system (RAAS) appears, apart from regulation of blood pressure, to be also involved in the pathogenesis of ARDS (Magalhães et al., 2019). Its main mediator, Angiotensin II (Ang II), is involved in inflammatory and fibrogenic processes in the lungs (Marshall et al., 2004; Hagiwara et al., 2009; Fletcher et al., 2017). Animal experiments in ARDS models demonstrate that the reduction of Ang II formation by inhibition of ACE exerts a protective effect (Imai et al., 2005, 2008; Shen et al., 2009). For example, the ACE inhibitor captopril is able to diminish oleic acid-induced severe acute lung injury in rats (He et al., 2007). Likewise, pharmacological inhibition or genetic deletion of AT1a receptors significantly mitigates lung injury (Raiden et al., 2002; Imai et al., 2005, 2008).
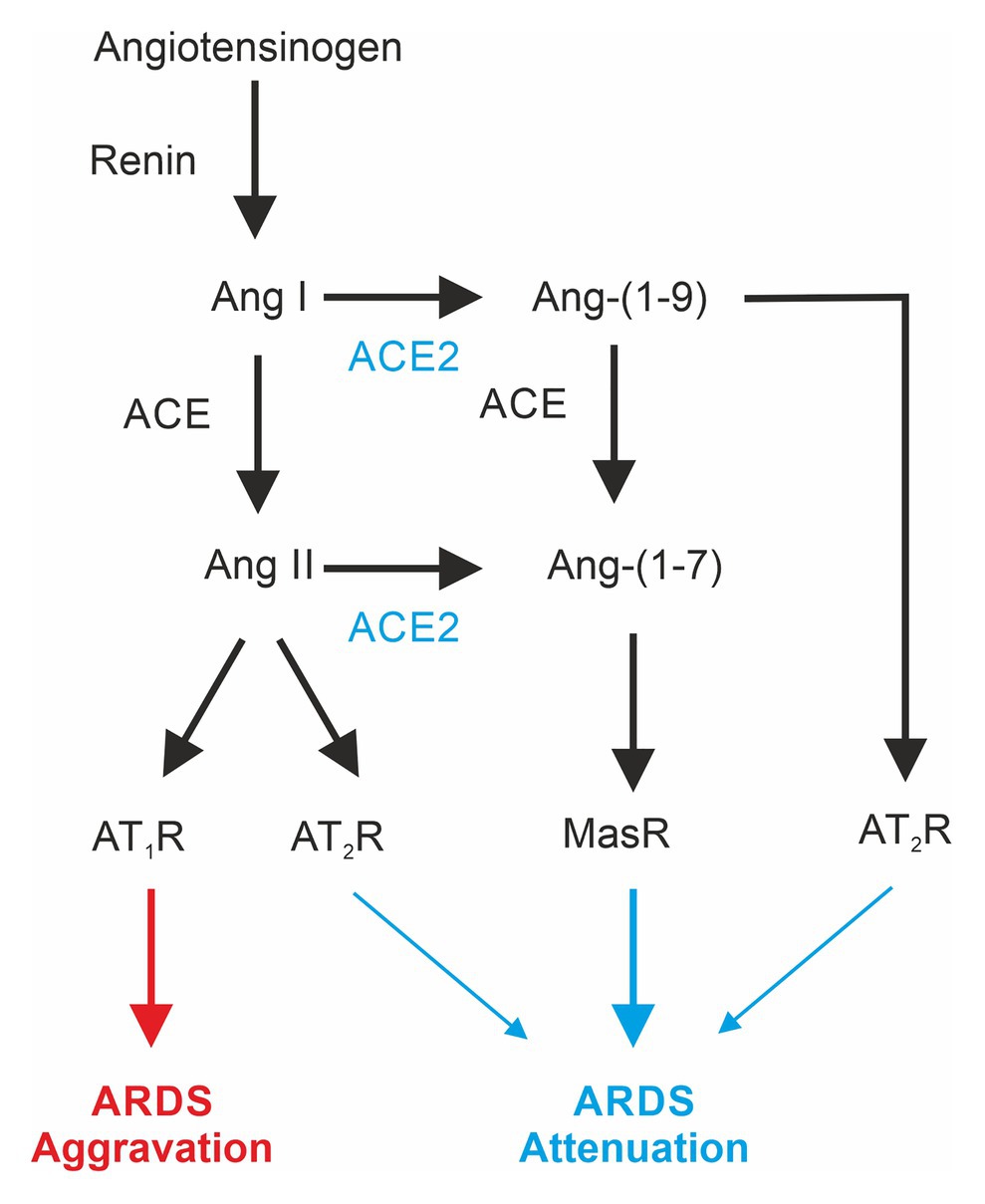
Figure 1. Differential effects of mediators of the renin-angiotensin system involved in acute respiratory distress syndrome (ARDS). ACE, angiotensin-converting enzyme; ACE2, angiotensin-converting enzyme 2; Ang, angiotensin; AT1-R, angiotensin II receptor type 1; AT2-R, angiotensin II receptor type 2; Mas-R, Mas-Receptor.
The angiotensin-converting enzyme 2 (ACE2; Donoghue et al., 2000; Tipnis et al., 2000), a homolog to the classical ACE, is also expressed in the lung (Hamming et al., 2004; Jia, 2016). The lack of ACE2 expression in ACE2-KO animals increases ARDS susceptibility, and moreover, inactivation of ACE in ACE2-deficient mice attenuates ARDS (Imai et al., 2005). ACE2 catalyzes the formation of angiotensin Ang-(1–7), which acts via the Mas-Receptor (Mas-R; Zambelli et al., 2012). Pharmacological activation of Mas-Rs or administration of recombinant ACE2 has been shown to exert lung-protective effects (Imai et al., 2005; Wosten-van Asperen et al., 2011). In addition, ACE activity is increased in ARDS-lungs, and ACE2 activity is reduced (Li et al., 2008; Wosten-van Asperen et al., 2011).
Taken together, these observations suggest that the ACE2-product Ang-(1–7) via the Mas-Receptor promotes protective effects in the lung, and shifting the RAS toward ACE/Ang II/AT1R has deleterious effects (Wang et al., 2019). Finally, ACE2 also cleaves Ang-(1–10) to angiotensin 1–9 acting via the AT2R, which has been shown to exert protective effects on ARDS development (Imai et al., 2005) and pulmonary hypertension (Cha et al., 2018).
Although in ARDS mice Ang II serum levels are elevated (Imai et al., 2005; Chen et al., 2013; Zou et al., 2014), data for humans are less clear. The Ace gene insertion/deletion (I/D) polymorphisms correlate with the susceptibility for and severity of ARDS (Marshall et al., 2002; Jerng et al., 2006; Adamzik et al., 2007; Tsantes et al., 2013) with those patients carrying a lower risk that are homozygous for the insertion (II) genotype (Adamzik et al., 2007). Since the ACE II genotype is associated with a lower serum ACE concentration (Rigat et al., 1990), one would expect lower ANG II serum levels. However, serum Ang II levels in humans are quite variable in ARDS as well as in control patients. Significantly higher Ang II serum levels in ARDS patients have never been reported (Wiberg-Jorgensen et al., 1983; Reddy et al., 2019). Nevertheless, a significantly higher Ang-(1–7) to Angiotensinogen [Ang-(1–10)] ratio as well as Ang-(1–9) to Ang-(1–10) ratio in ARDS survivors (Reddy et al., 2019) gives a hint of a protective effect of the ACE2. In addition, a pilot clinical trial using recombinant human angiotensin-converting enzyme 2 in ARDS revealed increased Ang-(1–7) levels but “did not result in improvement in physiological or clinical measures of ARDS in this small study” (Khan et al., 2017). Unfortunately, in this study, Ang-(1–9) levels were not tested.
The Renin-Angiotensin-System in SARS
Coronavirus disease 2019 (COVID-191) is a zoonotic disease caused by the novel SARS-CoV2 (Zhu et al., 2020). Although causing, in many cases, only mild symptoms, some patients develop a severe acute respiratory syndrome (SARS), which resembles ARDS in some but not all aspects (Gattinoni et al., 2020b,c; Marini and Gattinoni, 2020). The angiotensin-converting enzyme 2 is the receptor for SARS-CoV (Li et al., 2003) and SARS-CoV2 (Hoffmann et al., 2020).
In the initial phase of the COVID-19 pandemic, concerns about an increased risk for patients treated with ACE-inhibitors or angiotensin-receptor-blockers (ARBs) were raised (Kuster et al., 2020). Meanwhile, this topic has been studied extensively. In brief, no increase in the severity of COVID-19 or SARS-CoV2 infections have been found (Reynolds et al., 2020); in contrast, studies confirm a potential protective effect (Hippisley-Cox et al., 2020).
Interestingly, a considerable number of patients do not experience shortness of breath or dyspnea in the early phase of COVID-19 despite an already markedly impaired gas exchange, a status called silent hypoxia or silent or happy hypoxemia (Couzin-Frankel, 2020; Dhont et al., 2020; Ottestad et al., 2020). This phenomenon appears when lung compliance is still near normal but gas exchange is already impaired by ventilation/perfusion mismatch and functional shunt [non-ARDS type 1 (or type L); Gattinoni et al., 2020b]. SARS-CoV2 does not only infect the pulmonary epithelium, but heavily alters the vascular endothelium, causing impairment of its antithrombotic properties (McFadyen et al., 2020; Teuwen et al., 2020); thus micro-angiopathy and micro-embolisms can explain the alteration of the ventilation/perfusion ratio that is caused (Merrill et al., 2020). Moreover, pulmonary vasoplegia suspending partially or totally hypoxic pulmonary vasoconstriction leads to reasonable functional shunt (Chau et al., 2020).
However, these patients show mostly tachypnea (Chandra et al., 2020; Ottestad et al., 2020), clearly favoring the concept of an already increased respiratory drive and conflicting with the concept of a “failure to trigger the centrally mediated increase in respiratory rate” as put forward by Soliz (Soliz et al., 2020). The nearly normal compliance of the type L lung can explain the lack of dyspnea: As long as breathing efforts are not limited by the lungs’ elastance or external factors (Albashir, 2020). However, the increased respiratory drive can lead to severe hyperventilation with breathing efforts that create large negative pressure swings that lead to self-inflicted lung injury (P-SILI), thus promoting a shift to the H-type of COVID-19 pneumonia (Cruces et al., 2020; Gattinoni et al., 2020a; Smit et al., 2020).
Apart from this clinical alteration, it has been shown that plasma levels of angiotensin II of SARS-CoV2 infected patients were elevated (Liu et al., 2020; Wu et al., 2020), and moreover, plasma levels correlated to the viral load as well as to the degree of lung injury (Liu et al., 2020). An explanation for this is that the binding of SARS-CoV2 to virus-receptor ACE2 led to a downregulation of enzyme ACE2 in the lung tissue (Silhol et al., 2020), a mechanism that had been described already for SARS-CoV1 (Kuba et al., 2005).
RAS and the Regulation Breathing
Ang II and Ang-(1–7) exert differential effects on the carotid body (CB) glomus cells. In CB glomus cells, Ang II increases the respiratory drive by activation of NADPH oxidase (NOX) and mitochondrial-mediated O2-production with the consequence that K+-channels are inhibited and voltage-gated Ca2+ channels are activated (Allen, 1998; Schultz, 2011). In contrast, Ang-(1–7) exerts an inhibitory influence on glomus cells via activation of nNOS and NO-mediated activation of K+ channels (Schultz, 2011; Fung, 2014). It is of note that chronic hypoxia upregulates the expression and function of AT1-receptors in the carotid body (Leung et al., 2000).
However, the stimulation of breathing by i.v. application of Ang II in dogs could not solely be attributed to alterations in the carotid body activity (Potter and McCloskey, 1979), thus suggesting a role of central chemosensory pathways. Injection of Ang II into the nucleus of the solitary tract (NTS), which relays the chemosensitive information from the CB, is able to increase the respiratory rate (Paton and Kasparov, 1999). Moreover, Ang II receptors are expressed on many neurons, including serotonergic neurons in the raphe nuclei (Allen et al., 1991), which contain central CO2-chemosensor neurons (Severson et al., 2003; Richerson, 2004; Bhandare et al., 2020). Although the mechanism of Ang II action in these neurons is not yet completely understood, it is known that Ang II regulates release and synthesis of serotonin in raphe neurons (Nahmod et al., 1978) and that Ang II decreases the resting K+ conductance in other types of brainstem neurons (Li and Guyenet, 1996).
ACE2 is also expressed in the mouse brainstem (Lin et al., 2008), particularly in raphe neurons (Doobay et al., 2007). The functional role of the Ang II or Ang-(1–7) in primary respiratory neurons of pre-Bötzinger Complex in the medulla has not been investigated yet, but solid evidence exists that Ang II or Ang-(1–7) modulate the activity of cardiac neurons neighboring the respiratory neurons in the ventral lateral medulla (de Moura et al., 2010) as well as neurons in the nucleus of the solitary tract (Diz et al., 2002). Several recent studies demonstrate that ACE2/Ang-(1–7)/MasR interacts in the CNS with different neurotransmitter systems, including GABA, dopamine, and norepinephrine (Gironacci et al., 2004; Stragier et al., 2005; Wang et al., 2016). MasR are robustly expressed in GABAergic neurons in the basolateral amygdala (BLA), and ACE2 overexpression increases the spontaneous postsynaptic inhibitory currents in this region (Wang et al., 2016).
A Novel Hypothesis: Synthesis of the Obvious
Based on the literature reviewed above, we suggest the following hypothesis: In acute respiratory distress syndrome (ARDS) and in severe acute respiratory syndrome (SARS/COVID-19), alterations of the renin-angiotensin-system (RAS) signal a change of the chemosensitive reflex control of breathing, which results in an increase of the respiratory drive, which becomes independent from alterations of blood gases. Our hypothesis is based on the following key observation: In ARDS and especially in SARS/COVID-19, the RAS is dysregulated and shifted toward the ACE/Ang II/AT1R axis. This dysregulation is expected to stimulate, apart from any potential effect on the lung tissue, chemosensitive neurons in the brainstem and also chemosensitive cells in the carotid body (Figure 2), making them more sensitive to changes of CO2 and O2 and, thus, shifting their baseline activity and response curves to higher values.
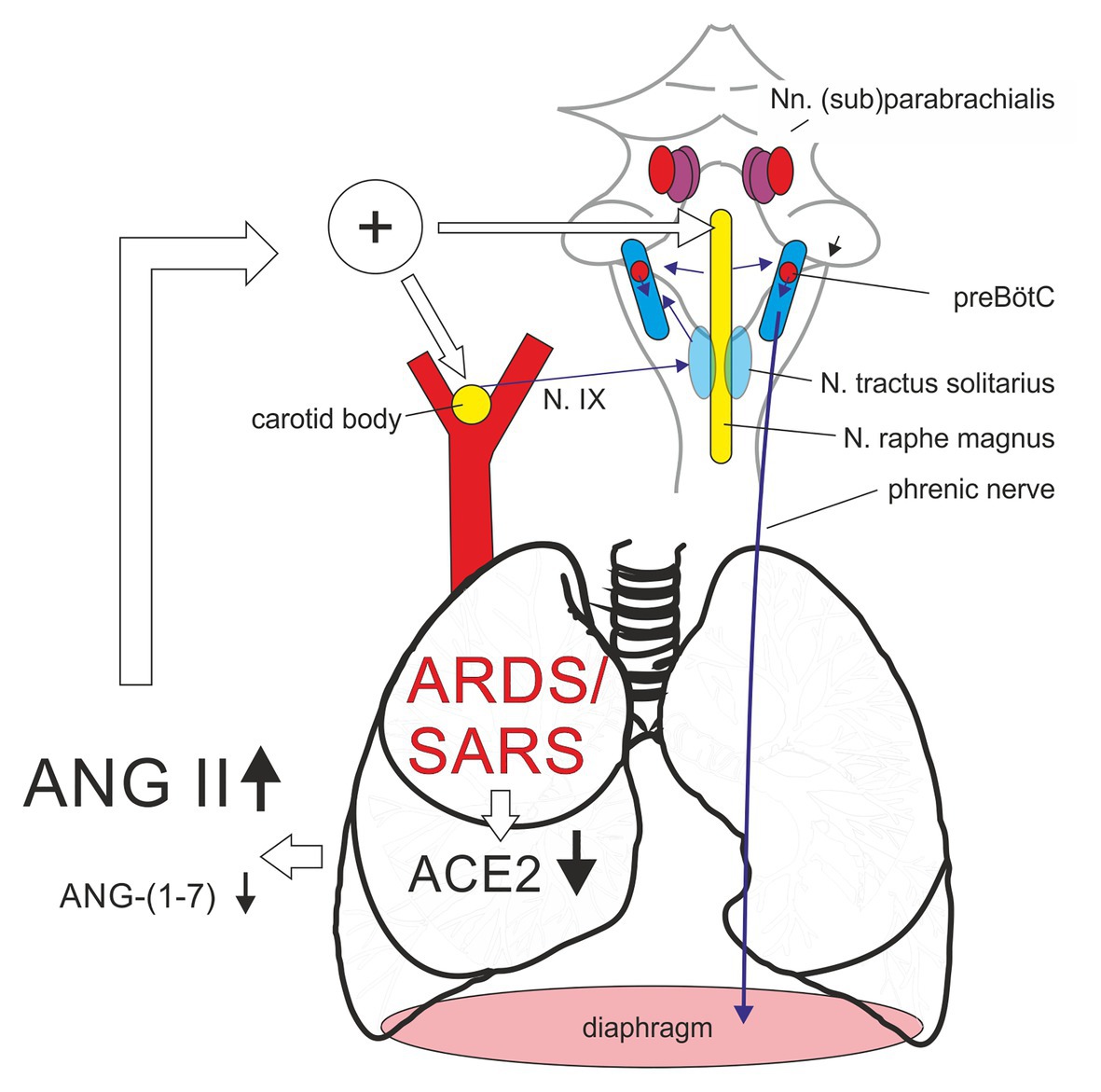
Figure 2. Alterations of renin-angiotensin-system (RAS) in the patient with acute respiratory distress syndrome or coronavirus-induced severe respiratory syndrome (SARS) led to an increase of respiratory chemosensitivity by an Angiotensin II (Ang II) mediated shift of the activity of the chemosensitive cell population in the carotid body and the medullary raphe. ACE2, angiotensin-converting enzyme 2; N. IX, glossopharyngeal nerve; preBötC, pre-Bötzinger complex.
Discussion
Confirmation of this hypothesis requires a joint effort of clinical and basic scientists with broad knowledge in physiology and neurosciences. Experimental approaches should include in vivo and ex vivo studies in animal models of ARDS.
What Types of Animal Models Are Available?
In general, so far, only animal models for the “classical” ARDS have been established and used, trying to mimic the uniform pathophysiology of this syndrome, characterized by a marked shunt volume and heavy, hard-to-move lungs. A COVID-19 affliction might – in the early phase – present with nearly normally compliant lungs but a heavily altered ventilation/perfusion (V/Q) ratio and a marked functional shunt volume, leading to severe hypoxia. The classical ARDS models have their clear limitations with regard to their transferability to clinical practice; they are what they are: models. To the best of our knowledge, a model for mimicking low V/Q and functional shunt does not exist and seems difficult to develop (Matute-Bello et al., 2008). Some of the “classical” ARDS models require intravenous application of agents, e.g., oleic acid (Schuster, 1994), and in others, the lung injury is induced by intratracheal application of the toxic agent, e.g., of acid (Imai et al., 2005) or bleomycin (Moore and Hogaboam, 2008). Data about alteration of respiratory control in animal models of acute lung injury and ARDS are limited. In the bleomycin model, alteration of the respiratory drive is described, which is independent of the impairment of oxygen exchange in the lung tissue (Jacono et al., 2006; Hsieh et al., 2020; Litvin et al., 2020). Alteration of Ang II serum levels have yet not been analyzed in the bleomycin model but are confirmed, among others, in the acid-instillation model (Imai et al., 2005; Chen et al., 2013; Zou et al., 2014).
Mouse models for COVID-19 that allow the analysis of breathing regulation are more complicated to develop, not only because the animal experiments are hindered by the need of laboratories with high biosafety levels, but because the spike proteins of SARS-CoV and SARS-CoV2 have a much lower binding affinity to the murine ACE2 than to its human homolog (Lutz et al., 2020). However, transgenic mice have been developed that express the human ACE2 (McCray et al., 2007; Bao et al., 2020; Sun et al., 2020). To our knowledge, no experiments on chemosensitivity have been performed in later mouse models yet.
How to Test Change of Chemosensitivity in ARDS Models?
Based on this hypothesis, it will be necessary to determine how the shift of the RAS toward the ACE/Ang II/AT1R axis influences the target cell population of the chemosensitive reflex. Therefore, experiments in animal models of ARDS and SARS are necessary to establish the cellular basis of alteration of neuronal control of breathing. There is a wide range of experimental tools available that allow addressing chemosensitivity of the respiratory network at different levels. Experiments could be performed in acutely isolated brainstem slices, allowing measurement of the direct response of cells to alteration of CO2 or O2 (Gourine et al., 2010; Rajani et al., 2018).
Alteration of chemosensitivity in mice with ARDS can also be tested in vivo using whole body plethysmography, where alteration of tidal volume and respiratory rate can be analyzed in animals exposed to different levels of CO2 or and/or O2 (Bissonnette and Knopp, 2004; Hsieh et al., 2020). Moreover, the whole respiratory network can be analyzed in an arterially perfused preparation [the working heart brainstem preparation, WHBP (Paton, 1996; Dhingra et al., 2019)], which has the advantage that it allows testing for alterations of the chemosensitivity and respiratory drive that are independent from the injury of the lung since blood gas can be controlled via the perfusate.
Alternative Mechanisms of Modulation of Respiratory Drive in ARDS
Ang II might increase respiratory drive via activation of carotid body (CB) glomus cells (Allen, 1998; Schultz, 2011, chemosensitive neurons of the raphe Severson et al., 2003 #3721; Richerson, 2004 #4146; Bhandare et al., 2020 #304), and in the relay nucleus of the solitary tract (NTS; Paton and Kasparov, 1999 #14118). However, further experiential effort is necessary to identify ARDS-dependent changes in other areas of the respiratory network, whether RAS may be involved directly or indirectly. This includes retrotrapezoid body (RTN) and the parafacial respiratory group, the pontine parabrachial/Kölliker-Fuse complex (pB/KF) as well as the ventrolateral medulla with BötC, preBötC, and VRG (Li and Guyenet, 1995).
Apart from its action on neurons, Ang II might be involved in alterations of astrocytes-dependent modulation of the respiratory network. Indeed, in many regions of the brain, AT-receptors have been found to be expressed on astrocytes (Sumners et al., 1991; Tallant et al., 1991; Gebke et al., 1998). Moreover, sequencing data indicate MasR-expression in astrocytes at least in older animals (Clarke et al., 2018). Whether the O2-sensitive astrocyte population in the medulla (Gourine and Funk, 2017; Rajani et al., 2018) or the population of CO2-sensitive astrocytes in the retrotrapezoid nucleus [RTN; (Gourine et al., 2010)] also expresses AT1R, AT2R, or MasR remains to be investigated.
From the beginning of the 1970s, it has been postulated that lung fibrosis can change breathing by alteration of lung reflexes (Guz and Widdicombe, 1970; Mansoor et al., 1997; Schelegle, 2003). Recently, lung reflex receptors, e.g., J-reflex, head deflation reflex, and Hering-Breuer inflation reflex, were again suggested to contribute to ARDS- and SARS-induced modulation of ventilatory response in patients (de Vries et al., 2020).
Are There any Potential Secondary Effects of Elevated Angiotensin II?
Focus of the research should be extended beyond the direct effects of, e.g., Ang II on the target cells. Since Ang II is involved in the inflammatory response of the body, secondary neuroinflammatory effects that might modulate the neural control of breathing have to be considered as well (Pena-Ortega, 2019). Indeed, the elevated level of pro-inflammatory cytokines in critically ill COVID-19 patients sheds new light on this topic (Herold et al., 2020; Huang et al., 2020; Schett et al., 2020). Many of these mediators have also be found to be elevated in classical ARDS (Tzouvelekis et al., 2005), and their expression is often stimulated by Ang II (Han et al., 1999; Nakamura et al., 2002; Luther et al., 2006; Qi et al., 2011). For IL 6, IL-1β, and TNF-α, stimulatory effects in the carotid body have been demonstrated (Fan et al., 2009; Del Rio et al., 2012), and there is little doubt that these three cytokines can have potentially stimulating effects also on respiratory and chemosensitive neurons in the brainstem (Kawasaki et al., 2008; Pena-Ortega, 2019). Along with this, it has been recently shown that ARDS is associated with a specific modulation of the post-hypoxic frequency decline, a component of the respiratory chemoreflex (Hsieh et al., 2020). Further, it has been previously shown that carotid body chemosensitivity is upregulated even before the presence of severe lung injury pathology (Jacono et al., 2006). Similarly, 2nd-order NTS neurons have also been implicated in mediating a sensory-plasticity after lung injury (Getsy et al., 2019).
Conclusion
In summary, imbalance of the renin-angiotensin-system in ARDS and SARS is expected to have substantial impact on the neuronal control of breathing and the chemosensitive reflex of the human body. While our hypothesis awaits experimental confirmation, it might lead to new therapeutic concepts and treatment options for intensive care patients with acute lung injury.
Author Contributions
SH and MQ conceptualization, writing – review and editing. SK and KM writing – review and editing. All authors contributed to the article and approved the submitted version.
Funding
SH received funding from VolkswagenStiftung and DFG.
Conflict of Interest
The authors declare that the research was conducted in the absence of any commercial or financial relationships that could be construed as a potential conflict of interest.
Footnotes
1. Novel Coronavirus (2019-nCoV). Situation Report – 22, WHO, February 12th, 2020, PDF downloaded July 28th 2020.
References
Adamzik, M., Frey, U., Sixt, S., Knemeyer, L., Beiderlinden, M., Peters, J., et al. (2007). ACE I/D but not AGT (−6)A/G polymorphism is a risk factor for mortality in ARDS. Eur. Respir. J. 29, 482–488. doi: 10.1183/09031936.00046106
Albashir, A. A. D. (2020). The potential impacts of obesity on COVID-19. Clin. Med. 20, e109–e113. doi: 10.7861/clinmed.2020-0239
Allen, A. M. (1998). Angiotensin AT1 receptor-mediated excitation of rat carotid body chemoreceptor afferent activity. J. Physiol. 510, 773–781.
Allen, A. M., Paxinos, G., Mckinley, M. J., Chai, S. Y., and Mendelsohn, F. A. (1991). Localization and characterization of angiotensin II receptor binding sites in the human basal ganglia, thalamus, midbrain pons, and cerebellum. J. Comp. Neurol. 312, 291–298.
Balibrea, J. L., and Arias-Diaz, J. (2003). Acute respiratory distress syndrome in the septic surgical patient. World J. Surg. 27, 1275–1284. doi: 10.1007/s00268-003-7258-3
Bao, L., Deng, W., Huang, B., Gao, H., Liu, J., Ren, L., et al. (2020). The pathogenicity of SARS-CoV-2 in hACE2 transgenic mice. Nature 583, 830–833. doi: 10.1038/s41586-020-2312-y
Bhandare, A., van de Wiel, J., Roberts, R., Braren, I., Huckstepp, R., and Dale, N. (2020). Analyzing the brainstem circuits for respiratory chemosensitivity in freely moving mice.
Bissonnette, J. M., and Knopp, S. J. (2004). Hypercapnic ventilatory response in mice lacking the 65 kDa isoform of glutamic acid decarboxylase (GAD65). Respir. Res. 5:3. doi: 10.1186/1465-9921-5-3
Cha, S. A., Park, B. M., and Kim, S. H. (2018). Angiotensin-(1-9) ameliorates pulmonary arterial hypertension via angiotensin type II receptor. Korean J. Physiol. Pharmacol. 22, 447–456. doi: 10.4196/kjpp.2018.22.4.447
Chandra, A., Chakraborty, U., Pal, J., and Karmakar, P. (2020). Silent hypoxia: a frequently overlooked clinical entity in patients with COVID-19. BMJ Case Rep. 13:e237207. doi: 10.1136/bcr-2020-237207
Chau, V. Q., Giustino, G., Mahmood, K., Oliveros, E., Neibart, E., Oloomi, M., et al. (2020). Cardiogenic shock and hyperinflammatory syndrome in young males with COVID-19. Circ. Heart Fail. 13:e007485. doi: 10.1161/CIRCHEARTFAILURE.120.007485
Chen, L. N., Yang, X. H., Nissen, D. H., Chen, Y. Y., Wang, L. J., Wang, J. H., et al. (2013). Dysregulated renin-angiotensin system contributes to acute lung injury caused by hind-limb ischemia-reperfusion in mice. Shock 40, 420–429. doi: 10.1097/SHK.0b013e3182a6953e
Clarke, L. E., Liddelow, S. A., Chakraborty, C., Munch, A. E., Heiman, M., and Barres, B. A. (2018). Normal aging induces A1-like astrocyte reactivity. Proc. Natl. Acad. Sci. U. S. A. 115, E1896–E1905. doi: 10.1073/pnas.1800165115
Couzin-Frankel, J. (2020). The mystery of the pandemic’s ‘happy hypoxia’. Science 368, 455–456. doi: 10.1126/science.368.6490.455
Crotti, S., Bottino, N., Ruggeri, G. M., Spinelli, E., Tubiolo, D., Lissoni, A., et al. (2017). Spontaneous breathing during extracorporeal membrane oxygenation in acute respiratory failure. Anesthesiology 126, 678–687. doi: 10.1097/ALN.0000000000001546
Cruces, P., Retamal, J., Hurtado, D. E., Erranz, B., Iturrieta, P., Gonzalez, C., et al. (2020). A physiological approach to understand the role of respiratory effort in the progression of lung injury in SARS-CoV-2 infection. Crit. Care 24:494. doi: 10.1186/s13054-020-03197-7
de Moura, M. M., Dos Santos, R. A., Campagnole-Santos, M. J., Todiras, M., Bader, M., Alenina, N., et al. (2010). Altered cardiovascular reflexes responses in conscious angiotensin-(1-7) receptor mas-knockout mice. Peptides 31, 1934–1939. doi: 10.1016/j.peptides.2010.06.030
de Vries, H., Endeman, H., van der Hoeven, J., and Heunks, L. (2020). Lung-protective mechanical ventilation in patients with COVID-19. Neth. J. Crit. Care 28, 120–124.
Del Rio, R., Moya, E. A., Parga, M. J., Madrid, C., and Iturriaga, R. (2012). Carotid body inflammation and cardiorespiratory alterations in intermittent hypoxia. Eur. Respir. J. 39, 1492–1500. doi: 10.1183/09031936.00141511
Dhingra, R. R., Furuya, W. I., Bautista, T. G., Dick, T. E., Galan, R. F., and Dutschmann, M. (2019). Increasing local excitability of brainstem respiratory nuclei reveals a distributed network underlying respiratory motor pattern formation. Front. Physiol. 10:887. doi: 10.3389/fphys.2019.00887
Dhont, S., Derom, E., Van Braeckel, E., Depuydt, P., and Lambrecht, B. N. (2020). The pathophysiology of ‘happy’ hypoxemia in COVID-19. Respir. Res. 21:198. doi: 10.1186/s12931-020-01462-5
Diz, D. I., Jessup, J. A., Westwood, B. M., Bosch, S. M., Vinsant, S., Gallagher, P. E., et al. (2002). Angiotensin peptides as neurotransmitters/neuromodulators in the dorsomedial medulla. Clin. Exp. Pharmacol. Physiol. 29, 473–482. doi: 10.1046/j.1440-1681.2002.03659.x
Donoghue, M., Hsieh, F., Baronas, E., Godbout, K., Gosselin, M., Stagliano, N., et al. (2000). A novel angiotensin-converting enzyme-related carboxypeptidase (ACE2) converts angiotensin I to angiotensin 1-9. Circ. Res. 87, E1–E9. doi: 10.1161/01.res.87.5.e1
Doobay, M. F., Talman, L. S., Obr, T. D., Tian, X., Davisson, R. L., and Lazartigues, E. (2007). Differential expression of neuronal ACE2 in transgenic mice with overexpression of the brain renin-angiotensin system. Am. J. Phys. Regul. Integr. Comp. Phys. 292, R373–R381. doi: 10.1152/ajpregu.00292.2006
Fan, J., Zhang, B., Shu, H. F., Zhang, X. Y., Wang, X., Kuang, F., et al. (2009). Interleukin-6 increases intracellular Ca2+ concentration and induces catecholamine secretion in rat carotid body glomus cells. J. Neurosci. Res. 87, 2757–2762. doi: 10.1002/jnr.22107
Fanelli, V., and Ranieri, V. M. (2015). Mechanisms and clinical consequences of acute lung injury. Ann. Am. Thorac. Soc. 12(Suppl. 1), S3–S8. doi: 10.1513/AnnalsATS.201407-340MG
Fletcher, A. N., Molteni, A., Ponnapureddy, R., Patel, C., Pluym, M., and Poisner, A. M. (2017). The renin inhibitor aliskiren protects rat lungs from the histopathological effects of fat embolism. J. Trauma Acute Care Surg. 82, 338–344. doi: 10.1097/TA.0000000000001278
Fung, M. L. (2014). The role of local renin-angiotensin system in arterial chemoreceptors in sleep-breathing disorders. Front. Physiol. 5:336. doi: 10.3389/fphys.2014.00336
Gattinoni, L., Chiumello, D., Caironi, P., Busana, M., Romitti, F., Brazzi, L., et al. (2020a). COVID-19 pneumonia: different respiratory treatments for different phenotypes? Intensive Care Med. 46, 1099–1102. doi: 10.1007/s00134-020-06033-2
Gattinoni, L., Coppola, S., Cressoni, M., Busana, M., Rossi, S., and Chiumello, D. (2020b). COVID-19 does not Lead to a "typical" acute respiratory distress syndrome. Am. J. Respir. Crit. Care Med. 201, 1299–1300. doi: 10.1164/rccm.202003-0817LE
Gattinoni, L., Meissner, K., and Marini, J. J. (2020c). The baby lung and the COVID-19 era. Intensive Care Med. 46, 1438–1440. doi: 10.1007/s00134-020-06103-5
Gebke, E., Müller, A. R., Jurzak, M., and Gerstberger, R. (1998). Angiotensin II-induced calcium signalling in neurons and astrocytes of rat circumventricular organs. Neuroscience 85, 509–520.
Getsy, P. M., Mayer, C. A., Macfarlane, P. M., Jacono, F. J., and Wilson, C. G. (2019). Acute lung injury in neonatal rats causes postsynaptic depression in nucleus tractus solitarii second-order neurons. Respir. Physiol. Neurobiol. 269:103250. doi: 10.1016/j.resp.2019.103250
Gironacci, M. M., Valera, M. S., Yujnovsky, I., and Pena, C. (2004). Angiotensin-(1-7) inhibitory mechanism of norepinephrine release in hypertensive rats. Hypertension 44, 783–787. doi: 10.1161/01.HYP.0000143850.73831.9d
Gourine, A. V., and Funk, G. D. (2017). On the existence of a central respiratory oxygen sensor. J. Appl. Physiol. 123, 1344–1349. doi: 10.1152/japplphysiol.00194.2017
Gourine, A. V., Kasymov, V., Marina, N., Tang, F., Figueiredo, M. F., Lane, S., et al. (2010). Astrocytes control breathing through pH-dependent release of ATP. Science 329, 571–575. doi: 10.1126/science.1190721
Guz, A., and Widdicombe, J. (1970). “Pattern of breathing during hypercapnia before and after vagal blockade in man” in Ciba foundation symposium-breathing: Hering-Breuer centenary symposium. ed. R. Porter (Wiley Online Library), 41–52.
Hagiwara, S., Iwasaka, H., Matumoto, S., Hidaka, S., and Noguchi, T. (2009). Effects of an angiotensin-converting enzyme inhibitor on the inflammatory response in in vivo and in vitro models. Crit. Care Med. 37, 626–633. doi: 10.1097/CCM.0b013e3181958d91
Hamming, I., Timens, W., Bulthuis, M. L., Lely, A. T., Navis, G., and van Goor, H. (2004). Tissue distribution of ACE2 protein, the functional receptor for SARS coronavirus. A first step in understanding SARS pathogenesis. J. Pathol. 203, 631–637. doi: 10.1002/path.1570
Han, Y., Runge, M. S., and Brasier, A. R. (1999). Angiotensin II induces interleukin-6 transcription in vascular smooth muscle cells through pleiotropic activation of nuclear factor-kappa B transcription factors. Circ. Res. 84, 695–703. doi: 10.1161/01.res.84.6.695
He, X., Han, B., Mura, M., Xia, S., Wang, S., Ma, T., et al. (2007). Angiotensin-converting enzyme inhibitor captopril prevents oleic acid-induced severe acute lung injury in rats. Shock 28, 106–111. doi: 10.1097/SHK.0b013e3180310f3a
Herold, T., Jurinovic, V., Arnreich, C., Lipworth, B. J., Hellmuth, J. C., Bergwelt-Baildon, M. V., et al. (2020). Elevated levels of IL-6 and CRP predict the need for mechanical ventilation in COVID-19. J. Allergy Clin. Immunol. 46, 128.e4–136.e4. doi: 10.1016/j.jaci.2020.05.008
Hippisley-Cox, J., Young, D., Coupland, C., Channon, K. M., Tan, P. S., Harrison, D. A., et al. (2020). Risk of severe COVID-19 disease with ACE inhibitors and angiotensin receptor blockers: cohort study including 8.3 million people. Heart 106, 1503–1511. doi: 10.1136/heartjnl-2020-317393
Hoffmann, M., Kleine-Weber, H., Schroeder, S., Kruger, N., Herrler, T., Erichsen, S., et al. (2020). SARS-CoV-2 cell entry depends on ACE2 and TMPRSS2 and is blocked by a clinically proven protease inhibitor. Cell 181, 271.e278–280.e278. doi: 10.1016/j.cell.2020.02.052
Hsieh, Y. H., Litvin, D. G., Zaylor, A. R., Nethery, D. E., Dick, T. E., and Jacono, F. J. (2020). Brainstem inflammation modulates the ventilatory pattern and its variability after acute lung injury in rodents. J. Physiol. 598, 2791–2811. doi: 10.1113/JP279177
Huang, C., Wang, Y., Li, X., Ren, L., Zhao, J., Hu, Y., et al. (2020). Clinical features of patients infected with 2019 novel coronavirus in Wuhan, China. Lancet 395, 497–506. doi: 10.1016/S0140-6736(20)30183-5
Imai, Y., Kuba, K., and Penninger, J. M. (2008). The discovery of angiotensin-converting enzyme 2 and its role in acute lung injury in mice. Exp. Physiol. 93, 543–548. doi: 10.1113/expphysiol.2007.040048
Imai, Y., Kuba, K., Rao, S., Huan, Y., Guo, F., Guan, B., et al. (2005). Angiotensin-converting enzyme 2 protects from severe acute lung failure. Nature 436, 112–116. doi: 10.1038/nature03712
Jacono, F. J., Peng, Y. J., Nethery, D., Faress, J. A., Lee, Z., Kern, J. A., et al. (2006). Acute lung injury augments hypoxic ventilatory response in the absence of systemic hypoxemia. J. Appl. Physiol. 101, 1795–1802. doi: 10.1152/japplphysiol.00100.2006
Jerng, J. S., Yu, C. J., Wang, H. C., Chen, K. Y., Cheng, S. L., and Yang, P. C. (2006). Polymorphism of the angiotensin-converting enzyme gene affects the outcome of acute respiratory distress syndrome. Crit. Care Med. 34, 1001–1006. doi: 10.1097/01.CCM.0000206107.92476.39
Jia, H. (2016). Pulmonary angiotensin-converting enzyme 2 (ACE2) and inflammatory lung disease. Shock 46, 239–248. doi: 10.1097/SHK.0000000000000633
Kawasaki, Y., Zhang, L., Cheng, J. K., and Ji, R. R. (2008). Cytokine mechanisms of central sensitization: distinct and overlapping role of interleukin-1beta, interleukin-6, and tumor necrosis factor-alpha in regulating synaptic and neuronal activity in the superficial spinal cord. J. Neurosci. 28, 5189–5194. doi: 10.1523/JNEUROSCI.3338-07.2008
Khan, A., Benthin, C., Zeno, B., Albertson, T. E., Boyd, J., Christie, J. D., et al. (2017). A pilot clinical trial of recombinant human angiotensin-converting enzyme 2 in acute respiratory distress syndrome. Crit. Care 21:234. doi: 10.1186/s13054-017-1823-x
Kuba, K., Imai, Y., Rao, S., Gao, H., Guo, F., Guan, B., et al. (2005). A crucial role of angiotensin converting enzyme 2 (ACE2) in SARS coronavirus-induced lung injury. Nat. Med. 11, 875–879. doi: 10.1038/nm1267
Kuster, G. M., Pfister, O., Burkard, T., Zhou, Q., Twerenbold, R., Haaf, P., et al. (2020). SARS-CoV2: should inhibitors of the renin-angiotensin system be withdrawn in patients with COVID-19? Eur. Heart J. 41, 1801–1803. doi: 10.1093/eurheartj/ehaa235
Leung, P. S., Lam, S. Y., and Fung, M. L. (2000). Chronic hypoxia upregulates the expression and function of AT(1) receptor in rat carotid body. J. Endocrinol. 167, 517–524. doi: 10.1677/joe.0.1670517
Li, Y. W., and Guyenet, P. G. (1995). Neuronal excitation by angiotensin II in the rostral ventrolateral medulla of the rat in vitro. Am. J. Phys. 268, R272–R277. doi: 10.1152/ajpregu.1995.268.1.R272
Li, Y. W., and Guyenet, P. G. (1996). Angiotensin II decreases a resting K+ conductance in rat bulbospinal neurons of the C1 area. Circ. Res. 78, 274–282. doi: 10.1161/01.res.78.2.274
Li, X., Molina-Molina, M., Abdul-Hafez, A., Uhal, V., Xaubet, A., and Uhal, B. D. (2008). Angiotensin converting enzyme-2 is protective but downregulated in human and experimental lung fibrosis. Am. J. Phys. Lung Cell. Mol. Phys. 295, L178–L185. doi: 10.1152/ajplung.00009.2008
Li, W. H., Moore, M. J., Vasilieva, N., Sui, J. H., Wong, S. K., Berne, M. A., et al. (2003). Angiotensin-converting enzyme 2 is a functional receptor for the SARS coronavirus. Nature 426, 450–454. doi: 10.1038/nature02145
Li, T., Yin, P. -F., Li, A., Shen, M. R., and Yao, Y. -X. (2020). Acute respiratory distress syndrome treated with awake extracorporeal membrane oxygenation in a patient with COVID-19 pneumonia. J. Cardiothorac. Vasc. Anesth. doi: 10.1053/j.jvca.2020.11.017 [Epub ahead of print]
Lin, Z., Chen, Y., Zhang, W., Chen, A. F., Lin, S., and Morris, M. (2008). RNA interference shows interactions between mouse brainstem angiotensin AT1 receptors and angiotensin-converting enzyme 2. Exp. Physiol. 93, 676–684. doi: 10.1113/expphysiol.2007.041657
Litvin, D. G., Denstaedt, S. J., Borkowski, L. F., Nichols, N. L., Dick, T. E., Smith, C. B., et al. (2020). Peripheral-to-central immune communication at the area postrema glial-barrier following bleomycin-induced sterile lung injury in adult rats. Brain Behav. Immun. 87, 610–633. doi: 10.1016/j.bbi.2020.02.006
Liu, Y., Yang, Y., Zhang, C., Huang, F., Wang, F., Yuan, J., et al. (2020). Clinical and biochemical indexes from 2019-nCoV infected patients linked to viral loads and lung injury. Sci. China Life Sci. 63, 364–374. doi: 10.1007/s11427-020-1643-8
Luther, J. M., Gainer, J. V., Murphey, L. J., Yu, C., Vaughan, D. E., Morrow, J. D., et al. (2006). Angiotensin II induces interleukin-6 in humans through a mineralocorticoid receptor–dependent mechanism. Hypertension 48, 1050–1057. doi: 10.1161/01.HYP.0000248135.97380.76
Lutz, C., Maher, L., Lee, C., and Kang, W. (2020). COVID-19 preclinical models: human angiotensin-converting enzyme 2 transgenic mice. Hum. Genomics 14:20. doi: 10.1186/s40246-020-00272-6
Magalhães, G. S., Campagnole-Santos, M. J., and da Glória Rodrigues-Machado, M. (2019). “Lung” in Angiotensin-(1-7). ed. R. Santos (Cham: Springer), 131–152.
Mansoor, J. K., Hyde, D. M., and Schelegle, E. S. (1997). Contribution of vagal afferents to breathing pattern in rats with lung fibrosis. Respir. Physiol. 108, 45–61. doi: 10.1016/s0034-5687(96)02505-4
Marini, J. J., and Gattinoni, L. (2020). Management of COVID-19 respiratory distress. JAMA 323, 2329–2330. doi: 10.1001/jama.2020.6825
Marshall, R. P., Gohlke, P., Chambers, R. C., Howell, D. C., Bottoms, S. E., Unger, T., et al. (2004). Angiotensin II and the fibroproliferative response to acute lung injury. Am. J. Phys. Lung Cell. Mol. Phys. 286, L156–L164. doi: 10.1152/ajplung.00313.2002
Marshall, R. P., Webb, S., Bellingan, G. J., Montgomery, H. E., Chaudhari, B., Mcanulty, R. J., et al. (2002). Angiotensin converting enzyme insertion /deletion polymorphism is associated with susceptibility and outcome in acute respiratory distress syndrome. Am. J. Respir. Crit. Care Med. 166, 646–650. doi: 10.1164/rccm.2108086
Matute-Bello, G., Frevert, C. W., and Martin, T. R. (2008). Animal models of acute lung injury. Am. J. Phys. Lung Cell. Mol. Phys. 295, L379–L399. doi: 10.1152/ajplung.00010.2008
McCray, P. B. Jr., Pewe, L., Wohlford-Lenane, C., Hickey, M., Manzel, L., Shi, L., et al. (2007). Lethal infection of K18-hACE2 mice infected with severe acute respiratory syndrome coronavirus. J. Virol. 81, 813–821. doi: 10.1128/JVI.02012-06
McFadyen, J. D., Stevens, H., and Peter, K. (2020). The emerging threat of (micro)thrombosis in COVID-19 and its therapeutic implications. Circ. Res. 127, 571–587. doi: 10.1161/CIRCRESAHA.120.317447
Merrill, J. T., Erkan, D., Winakur, J., and James, J. A. (2020). Emerging evidence of a COVID-19 thrombotic syndrome has treatment implications. Nat. Rev. Rheumatol. 16, 581–589. doi: 10.1038/s41584-020-0474-5
Moore, B. B., and Hogaboam, C. M. (2008). Murine models of pulmonary fibrosis. Am. J. Phys. Lung Cell. Mol. Phys. 294, L152–L160. doi: 10.1152/ajplung.00313.2007
Nahmod, V. E., Finkielman, S., Benarroch, E. E., and Pirola, C. J. (1978). Angiotensin regulates release and synthesis of serotonin in brain. Science 202, 1091–1093. doi: 10.1126/science.152460
Nakamura, A., Johns, E. J., Imaizumi, A., Niimi, R., Yanagawa, Y., and Kohsaka, T. (2002). Role of angiotensin II-induced cAMP in mesangial TNF-alpha production. Cytokine 19, 47–51. doi: 10.1006/cyto.2002.1040
Ottestad, W., Seim, M., and Maehlen, J. O. (2020). COVID-19 with silent hypoxemia. Tidsskr. Nor. Laegeforen. 140. doi: 10.4045/tidsskr.20.0299
Paton, J. F. R. (1996). A working heart-brainstem preparation of the mouse. J. Neurosci. Methods 65, 63–68. doi: 10.1016/0165-0270(95)00147-6
Paton, J. F. R., and Kasparov, S. (1999). Differential effects of angiotensin II on cardiorespiratory reflexes mediated by nucleus tractus solitarii a microinjection study in the rat. J. Physiol. 521, 213–225. doi: 10.1111/j.1469-7793.1999.00213.x
Pena-Ortega, F. (2019). Clinical and experimental aspects of breathing modulation by inflammation. Auton. Neurosci. 216, 72–86. doi: 10.1016/j.autneu.2018.11.002
Potter, E. K., and Mccloskey, D. I. (1979). Respiratory stimulatiion by angiotensin II. Respir. Physiol. 36, 367–373. doi: 10.1016/0034-5687(79)90048-3
Qi, G., Jia, L., Li, Y., Bian, Y., Cheng, J., Li, H., et al. (2011). Angiotensin II infusion-induced inflammation, monocytic fibroblast precursor infiltration, and cardiac fibrosis are pressure dependent. Cardiovasc. Toxicol. 11, 157–167. doi: 10.1007/s12012-011-9109-z
Raiden, S., Nahmod, K., Nahmod, V., Semeniuk, G., Pereira, Y., Alvarez, C., et al. (2002). Nonpeptide antagonists of AT1 receptor for angiotensin II delay the onset of acute respiratory distress syndrome. J. Pharmacol. Exp. Ther. 303, 45–51. doi: 10.1124/jpet.102.037382
Rajani, V., Zhang, Y., Jalubula, V., Rancic, V., Sheikhbahaei, S., Zwicker, J. D., et al. (2018). Release of ATP by pre-Botzinger complex astrocytes contributes to the hypoxic ventilatory response via a Ca2+-dependent P2Y1 receptor mechanism. J. Physiol. 596, 3245–3269. doi: 10.1113/JP274727
Ranieri, V. M., Rubenfeld, G. D., Thompson, B. T., Ferguson, N. D., Caldwell, E., Fan, E., et al. (2012). ARDS definition task force. Acute respiratory distress syndrome: the Berlin definition. JAMA 307, 2526–2533. doi: 10.1001/jama.2012.5669
Reddy, R., Asante, I., Liu, S., Parikh, P., Liebler, J., Borok, Z., et al. (2019). Circulating angiotensin peptides levels in acute respiratory distress syndrome correlate with clinical outcomes: a pilot study. PLoS One 14:e0213096. doi: 10.1371/journal.pone.0213096
Reynolds, H. R., Adhikari, S., Pulgarin, C., Troxel, A. B., Iturrate, E., Johnson, S. B., et al. (2020). Renin-angiotensin-aldosterone system inhibitors and risk of Covid-19. N. Engl. J. Med. 382, 2441–2448. doi: 10.1056/NEJMoa2008975
Richerson, G. B. (2004). Serotonergic neurons as carbon dioxide sensors that maintain pH homeostasis. Nat. Rev. Neurosci. 5, 449–461. doi: 10.1038/nrn1409
Rigat, B., Hubert, C., Alhenc-Gelas, F., Cambien, F., Corvol, P., and Soubrier, F. (1990). An insertion/deletion polymorphism in the angiotensin I-converting enzyme gene accounting for half the variance of serum enzyme levels. J. Clin. Invest. 86, 1343–1346. doi: 10.1172/JCI114844
Schelegle, E. S. (2003). Functional morphology and physiology of slowly adapting pulmonary stretch receptors. Anat. Rec. A Discov. Mol. Cell. Evol. Biol. 270, 11–16. doi: 10.1002/ar.a.10004
Schett, G., Sticherling, M., and Neurath, M. F. (2020). COVID-19: risk for cytokine targeting in chronic inflammatory diseases? Nat. Rev. Immunol. 20, 271–272. doi: 10.1038/s41577-020-0312-7
Schultz, H. D. (2011). Angiotensin and carotid body chemoreception in heart failure. Curr. Opin. Pharmacol. 11, 144–149. doi: 10.1016/j.coph.2010.12.004
Schuster, D. P. (1994). ARDS: clinical lessons from the oleic acid model of acute lung injury. Am. J. Respir. Crit. Care Med. 149, 245–260. doi: 10.1164/ajrccm.149.1.8111590
Severson, C. A., Wang, W., Pieribone, V. A., Dohle, C. I., and Richerson, G. B. (2003). Midbrain serotonergic neurons are central pH chemoreceptors. Nat. Neurosci. 6, 1139–1140. doi: 10.1038/nn1130
Shen, L., Mo, H., Cai, L., Kong, T., Zheng, W., Ye, J., et al. (2009). Losartan prevents sepsis-induced acute lung injury and decreases activation of nuclear factor kappaB and mitogen-activated protein kinases. Shock 31, 500–506. doi: 10.1097/SHK.0b013e318189017a
Silhol, F., Sarlon, G., Deharo, J. C., and Vaisse, B. (2020). Downregulation of ACE2 induces overstimulation of the renin-angiotensin system in COVID-19: should we block the renin-angiotensin system? Hypertens. Res. 43, 854–856. doi: 10.1038/s41440-020-0476-3
Smit, F., Oelofse, A., Linegar, A., Botes, L., and Turton, E. (2020). Supplemental oxygen therapy in COVID-19. SA Heart J. 17, 324–328.
Soliz, J., Schneider-Gasser, E. M., Arias-Reyes, C., Aliaga-Raduan, F., Poma-Machicao, L., Zubieta-Calleja, G., et al. (2020). Coping with hypoxemia: could erythropoietin (EPO) be an adjuvant treatment of COVID-19? Respir. Physiol. Neurobiol. 279:103476. doi: 10.1016/j.resp.2020.103476
Spinelli, E., Mauri, T., Beitler, J. R., Pesenti, A., and Brodie, D. (2020). Respiratory drive in the acute respiratory distress syndrome: pathophysiology, monitoring, and therapeutic interventions. Intensive Care Med. 46, 606–618. doi: 10.1007/s00134-020-05942-6
Stragier, B., Hristova, I., Sarre, S., Ebinger, G., and Michotte, Y. (2005). In vivo characterization of the angiotensin-(1-7)-induced dopamine and gamma-aminobutyric acid release in the striatum of the rat. Eur. J. Neurosci. 22, 658–664. doi: 10.1111/j.1460-9568.2005.04188.x
Sumners, C., Tang, W., Zelezna, B., and Raizada, M. K. (1991). Angiotensin II receptor subtypes are coupled with distinct signal-transduction mechanisms in neurons and astrocytes from rat brain. Proc. Natl. Acad. Sci. U. S. A. 88, 7567–7571. doi: 10.1073/pnas.88.17.7567
Sun, S. -H., Chen, Q., Gu, H. -J., Yang, G., Wang, Y. -X., Huang, X. -Y., et al. (2020). A mouse model of SARS-CoV-2 infection and pathogenesis. Cell Host Microbe 28, 124–133.e4. doi: 10.1016/j.chom.2020.05.020
Tallant, E. A., Jaiswal, N., Diz, D. I., and Ferrario, C. M. (1991). Human astrocytes contain two distinct angiotensin receptor subtypes. Hypertension 18, 32–39. doi: 10.1161/01.hyp.18.1.32
Teuwen, L. A., Geldhof, V., Pasut, A., and Carmeliet, P. (2020). COVID-19: the vasculature unleashed. Nat. Rev. Immunol. 20, 389–391. doi: 10.1038/s41577-020-0343-0
Tipnis, S. R., Hooper, N. M., Hyde, R., Karran, E., Christie, G., and Turner, A. J. (2000). A human homolog of angiotensin-converting enzyme. Cloning and functional expression as a captopril-insensitive carboxypeptidase. J. Biol. Chem. 275, 33238–33243. doi: 10.1074/jbc.M002615200
Tsantes, A. E., Kopterides, P., Bonovas, S., Bagos, P., Antonakos, G., Nikolopoulos, G. K., et al. (2013). Effect of angiotensin converting enzyme gene I/D polymorphism and its expression on clinical outcome in acute respiratory distress syndrome. Minerva Anestesiol. 79, 861–870.
Tzouvelekis, A., Pneumatikos, I., and Bouros, D. (2005). Serum biomarkers in acute respiratory distress syndrome an ailing prognosticator. Respir. Res. 6:62. doi: 10.1186/1465-9921-6-62
Wang, D., Chai, X. Q., Magnussen, C. G., Zosky, G. R., Shu, S. H., Wei, X., et al. (2019). Renin-angiotensin-system, a potential pharmacological candidate, in acute respiratory distress syndrome during mechanical ventilation. Pulm. Pharmacol. Ther. 58:101833. doi: 10.1016/j.pupt.2019.101833
Wang, L., De Kloet, A. D., Pati, D., Hiller, H., Smith, J. A., Pioquinto, D. J., et al. (2016). Increasing brain angiotensin converting enzyme 2 activity decreases anxiety-like behavior in male mice by activating central mas receptors. Neuropharmacology 105, 114–123. doi: 10.1016/j.neuropharm.2015.12.026
Wiberg-Jorgensen, F., Klausen, N. O., Hald, A., Qvist, J., Giese, J., and Damkjaer Nielsen, M. (1983). Pulmonary angiotensin II production in respiratory failure. Clin. Physiol. 3, 59–67. doi: 10.1111/j.1475-097x.1983.tb00699.x
Wosten-van Asperen, R. M., Lutter, R., Specht, P. A., Moll, G. N., van Woensel, J. B., van der Loos, C. M., et al. (2011). Acute respiratory distress syndrome leads to reduced ratio of ACE/ACE2 activities and is prevented by angiotensin-(1-7) or an angiotensin II receptor antagonist. J. Pathol. 225, 618–627. doi: 10.1002/path.2987
Wu, Z., Hu, R., Zhang, C., Ren, W., Yu, A., and Zhou, X. (2020). Elevation of plasma angiotensin II level is a potential pathogenesis for the critically ill COVID-19 patients. Crit. Care 24:290. doi: 10.1186/s13054-020-03015-0
Zambelli, V., Grassi, A., and Bellani, G. (2012). “Role of the renin-angiotensin system in ARDS” in Annual update in intensive care and emergency medicine 2012. ed. J.-L. Vincent (Berlin, Heidelberg: Springer)
Zhu, N., Zhang, D., Wang, W., Li, X., Yang, B., Song, J., et al. (2020). A novel coronavirus from patients with pneumonia in China, 2019. N. Engl. J. Med. 382, 727–733. doi: 10.1056/NEJMoa2001017
Keywords: acute lung damage, respiratory chemoreflexes, neuronal control of breathing, brainstem, homeostasis
Citation: Hülsmann S, Khabbazzadeh S, Meissner K and Quintel M (2021) A Potential Role of the Renin-Angiotensin-System for Disturbances of Respiratory Chemosensitivity in Acute Respiratory Distress Syndrome and Severe Acute Respiratory Syndrome. Front. Physiol. 11:588248. doi: 10.3389/fphys.2020.588248
Edited by:
Deborah Schechtman, University of São Paulo, BrazilReviewed by:
Carmen Silvia Valente Barbas, University of São Paulo, BrazilRishi R. Dhingra, University of Melbourne, Australia
Copyright © 2021 Hülsmann, Khabbazzadeh, Meissner and Quintel. This is an open-access article distributed under the terms of the Creative Commons Attribution License (CC BY). The use, distribution or reproduction in other forums is permitted, provided the original author(s) and the copyright owner(s) are credited and that the original publication in this journal is cited, in accordance with accepted academic practice. No use, distribution or reproduction is permitted which does not comply with these terms.
*Correspondence: Swen Hülsmann, c2h1ZWxzbTJAdW5pLWdvZXR0aW5nZW4uZGU=