- 1Department of Vascular Pathophysiology, Centro Nacional de Investigaciones Cardiovasculares (CNIC), Madrid, Spain
- 2Department of Molecular Biomedicine, Centro de Investigaciones Biológicas Margarita Salas (CIB-CSIC), Madrid, Spain
The vasculature ensures optimal delivery of nutrients and oxygen throughout the body, and to achieve this function it must continually adapt to varying tissue demands. Newly formed vascular plexuses during development are immature and require dynamic remodeling to generate well-patterned functional networks. This is achieved by remodeling of the capillaries preserving those which are functional and eliminating other ones. A balanced and dynamically regulated capillary remodeling will therefore ensure optimal distribution of blood and nutrients to the tissues. This is particularly important in pathological contexts in which deficient or excessive vascular remodeling may worsen tissue perfusion and hamper tissue repair. Blood flow is a major determinant of microvascular reshaping since capillaries are pruned when relatively less perfused and they split when exposed to high flow in order to shape the microvascular network for optimal tissue perfusion and oxygenation. The molecular machinery underlying blood flow sensing by endothelial cells is being deciphered, but much less is known about how this translates into endothelial cell responses as alignment, polarization and directed migration to drive capillary remodeling, particularly in vivo. Part of this knowledge is theoretical from computational models since blood flow hemodynamics are not easily recapitulated by in vitro or ex vivo approaches. Moreover, these events are difficult to visualize in vivo due to their infrequency and briefness. Studies had been limited to postnatal mouse retina and vascular beds in zebrafish but new tools as advanced microscopy and image analysis are strengthening our understanding of capillary remodeling. In this review we introduce the concept of remodeling of the microvasculature and its relevance in physiology and pathology. We summarize the current knowledge on the mechanisms contributing to capillary regression and to capillary splitting highlighting the key role of blood flow to orchestrate these processes. Finally, we comment the potential and possibilities that microfluidics offers to this field. Since capillary remodeling mechanisms are often reactivated in prevalent pathologies as cancer and cardiovascular disease, all this knowledge could be eventually used to improve the functionality of capillary networks in diseased tissues and promote their repair.
Dynamic Microvascular Remodeling in Physiology and Pathophysiology
The microvasculature constantly adjusts to tissue metabolic demands through functional and structural adaptations (Pries and Secomb, 2014). This is attained by dynamic remodeling of the capillaries preserving and expanding those which are functional and eliminating redundant or poorly efficient ones. We will refer in this review as microvascular or capillary remodeling to the dynamic gain or loss of capillaries for increasing or decreasing the complexity of the microvascular network in order to optimize oxygen and nutrient distribution into the tissue. Two main processes determine microvascular reshaping in several organs and tissues during their growth and development: i) capillary pruning or regression, the elimination of non-functional capillaries to form a functional hierarchically branched network; and ii) duplication of highly perfused capillaries which results in the quick expansion of the microvasculature. Duplication can occur via intussusceptive angiogenesis, recognized as the division of a large lumen within a sinus, or by splitting in tubular capillaries, the process in which we will mainly focus this review (Figure 1).
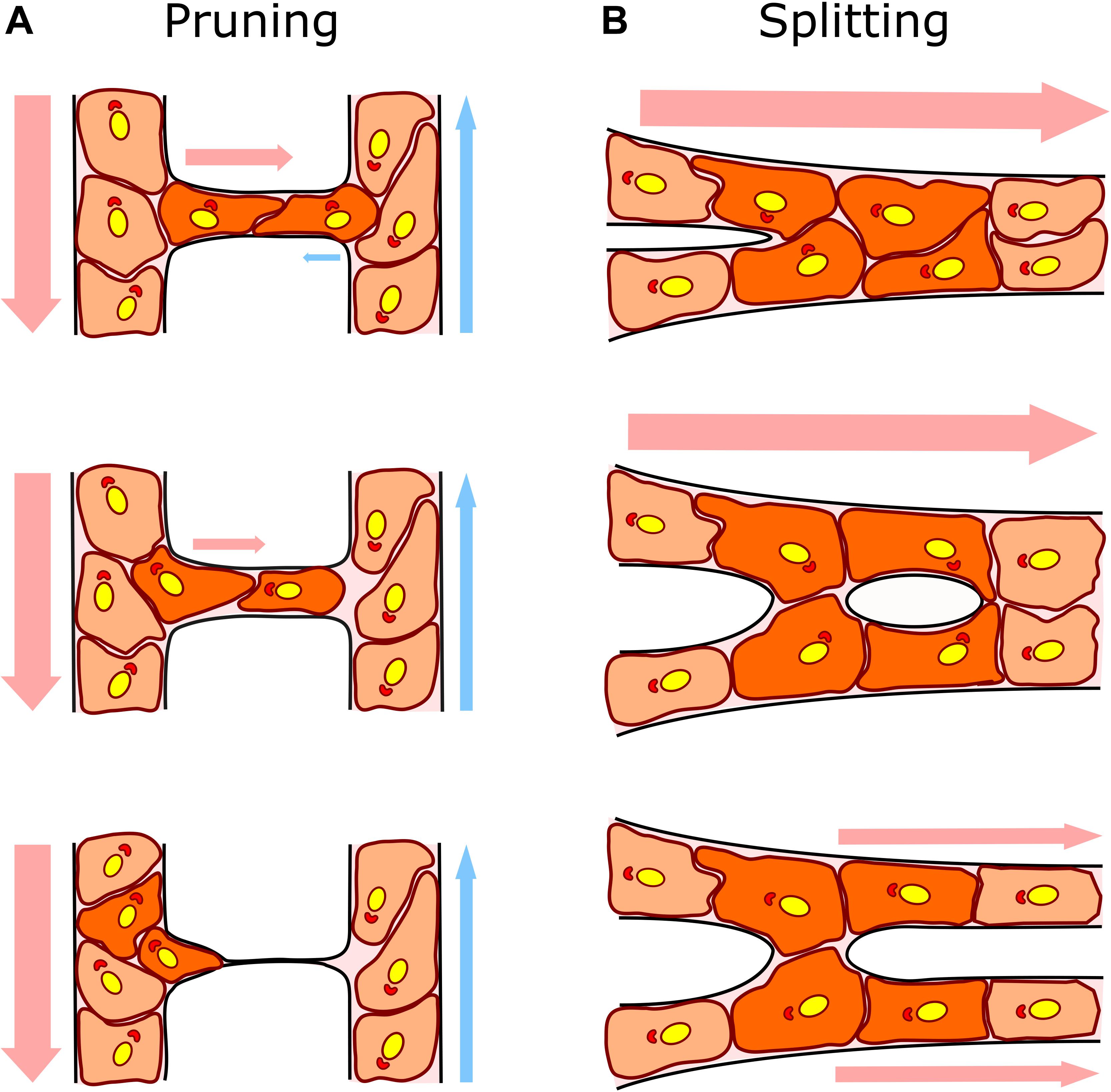
Figure 1. Capillary remodeling by pruning/regression and splitting/duplication. (A) In capillary pruning the poorly perfused vessel is selected to regress, its lumen collapses and the endothelial cells inside (dark orange) polarized against flow and migrate toward the higher flow adjacent vessel. (B) In capillary splitting, highly perfused vessels vasodilate and endothelial cells nearby the intersections (Y bifurcation; dark orange) reorganize their cytoskeleton, migrate toward the lumen and form an intraluminal pillar that will eventually split the vessel forming two daughter vessels. Arrows indicate the direction and intensity of the blood flow (arterial flow in red and venous flow in blue). Endothelial cell nuclei and Golgi are colored in yellow and red, respectively, to show endothelial cell polarization preferentially against the flow.
Once immature vascular plexuses are formed mostly by sprouting angiogenesis, capillary remodeling becomes essential to preserve tissue homeostasis in physiological contexts during development but also in adult tissues. Capillary regression takes place in all developmental stages during embryogenesis after the formation of the first primitive vasculature as in the chicken yolk sac and limb embryo (Feinberg et al., 1986; Lee et al., 2011) or the brain and intersegmental vessels in the zebrafish (Chen et al., 2012; Kochhan et al., 2013; Franco et al., 2015), but also right after birth like in the postnatal mouse retina (Connolly et al., 1988). Vascular regression can also be associated not only to optimization of the blood flow network but also to the elimination of unneeded tissue like in hyaloid regression after birth (Lang et al., 1994; Ito and Yoshioka, 1999; Lobov et al., 2005) or in involution of post-lactation mammary gland and ovarian luteolysis in the adult (Goede et al., 1998; Andres and Djonov, 2010); in these contexts endothelial cell apoptosis is considered the main mechanism and will not be discussed further in this review (Watson et al., 2017). Capillary duplication promotes expansion of the microvasculature during embryo and organ development as observed in the chicken chorioallantoic membrane (Patan et al., 1993) and in a variety of tissues after birth as the lung, heart, intestine, liver or kidney (Patan et al., 1992). It also contributes to capillary growth upon increasing tissue demands as in the exercising skeletal muscle (Prior et al., 2003; Mentzer and Konerding, 2014). Notably these vascular reshaping mechanisms are often reactivated or defective in prevalent pathologies leading generally to a dysfunctional microvasculature which aggravates damage and hinders tissue repair. Although altered capillary pruning has been described in tumors (Holash et al., 1999), it may also occur in ischemic disorders as myocardial infarction (Luttun et al., 2002; Gkontra et al., 2019), hypertension (Boudier, 1999), and age-related neurodegeneration or Alzheimer’s disease (Wu et al., 2005; Bell and Zlokovic, 2009; Sagare et al., 2012) exacerbating the hypo-perfusion of the damaged tissue. Capillary splitting has been observed among others in inflammatory bowel disease (Mori et al., 2005; Ravnic et al., 2007; Esteban et al., 2020), tumors (Patan et al., 1996b; Ribatti and Djonov, 2012), lung dysplasia of prematurity (De Paepe et al., 2015, 2017) and other syndromes (Giacomini et al., 2015) contributing to disease progression. Impaired endothelial cell responses involved in microvascular remodeling can also result in the persistence or arteriovenous shunts, the basis for arteriovenous malformations (AVM) (Red-Horse and Siekmann, 2019).
Capillary remodeling events are dynamic, occurring in hours to a few days and involve active endothelial cell rearrangements and quick morphological changes of the microvasculature, often without compromising its integrity since the basement membrane is preserved (Ricard and Simons, 2015). The current model for capillary pruning proposes a multi-staged model to explain the dynamics of the vessel regression process observed in the postnatal mouse retina and intersegmental vessels in zebrafish (Franco et al., 2015, 2016; Franco and Gerhardt, 2017). Firstly, there is a blood flow-driven selection of the regressing vascular branch which triggers the subsequent morphological alterations. The selected vessel receiving low flow constricts leading to lumen stenosis or even collapse. Then, endothelial cells retract along with junctional remodeling and migrate intraluminally from the regressing segment to the higher-flow adjacent vessel (Franco et al., 2015; Barbacena et al., 2016). The final resolution step involves the complete integration of endothelial cells from the regressing branch into the neighbor vessel leaving an empty basement membrane surrounded by pericytes which is called “empty sleeve” and considered a hallmark of vessel regression (Barbacena et al., 2016). The number of regressed segments increased only slightly and did not accumulate from P4 to P8 during remodeling of the postnatal retinal microvasculature indicating that in spite of the limitation of the analysis in still images, these pruning events seem dynamic and with a limited lifetime (Franco et al., 2015). Accordingly, live microscopy of the intersegmental vessels of zebrafish embryos showed that endothelial cell dynamics during segment regression occur in about 24 h (Franco et al., 2015).
In the context of capillary duplication by splitting or intussusceptive angiogenesis observed for example in the chicken chorioallantoic membrane, the rat skeletal muscle and the mouse inflamed intestine, the division of the vascular plexus takes place through the formation and expansion of intraluminal pillars. Increased blood flow in the capillary bed causes vasodilation of the blood vessels. The endothelial cells of the vessel reorganize their cytoskeleton, develop luminal filopodia mostly from the inter-junctional area and migrate toward the vascular lumen until they join the opposite side and rearrange their junctions (Patan et al., 1996a; Burri et al., 2004; Williams et al., 2006a). The cells form a cell bridge in the center of the vessel, rich in parallel actin filaments. These steps in the nascent pillar have been visualized by microscopy techniques, but it remains unknown how filopodia grow and fuse under high blood flow that would impose a mechanical barrier to the process. Pillars form in vessels with an intact basement membrane (Mentzer and Konerding, 2014), and subsequently, a 1–5 μm-pore forms in the center of the capillary which is invaded by surrounding tissue pericytes and myofibroblasts. These cells deposit collagen fibers stabilizing the formation of the pillar. Finally, the pillars grow in diameter and join other pillars splitting the capillary into two parallel vessels in the form of loops and duplications (Burri et al., 2004; Karthik et al., 2018). Of note, pillar formation in the chorioallantoic membrane initiated 40 min to few hours after vasodilation and in the inflamed ear model full capillary splitting was achieved in 3 days (Styp-Rekowska et al., 2011). Recently a pioneer study has analyzed the dynamics of vessel splitting by live microscopy imaging in the caudal vein plexus of zebrafish and showed that pillar formation and fusion can occur in just a few hours (Karthik et al., 2018). In this study, new data obtained by 3D reconstruction of scanning electron microscopy images have shown with unprecedented resolution the process of formation of intraluminal pillars and their fusion and highlighted the need of understanding the dynamics and cellular events of the loss of endothelial cell polarity, cytoskeleton rearrangement and establishment of new endothelial cell junctions at the pillar (Karthik et al., 2018).
Blood Flow-Driven Capillary Regression and Splitting: Two Sides of the Same Coin?
The dynamic nature of microvascular remodeling closely reflects the dynamically changing nature of blood flow, constantly responding to physicochemical cues in the tissues (Pries and Secomb, 2014). Pioneer studies in the embryo yolk sac established the essential role of blood flow in vascular remodeling (le Noble et al., 2004) and put the foundations about the dynamic responses induced by blood flow in endothelial cells as their directed migration from small capillaries to larger arteries (Udan et al., 2013). In addition to influencing angiogenic endothelial cell sprouting (Campinho et al., 2020), blood flow governs microvascular remodeling by promoting pruning of capillaries when not constantly perfused and expanding them when exposed to high flow in order to shape the microvascular network for optimal blood flow distribution (Figure 2A). However, the concept that low flow drives pruning and high flow triggers splitting is an oversimplification since it is not the magnitude but the gradients of blood flow that rule both remodeling processes as discussed in more detail in the following sections.
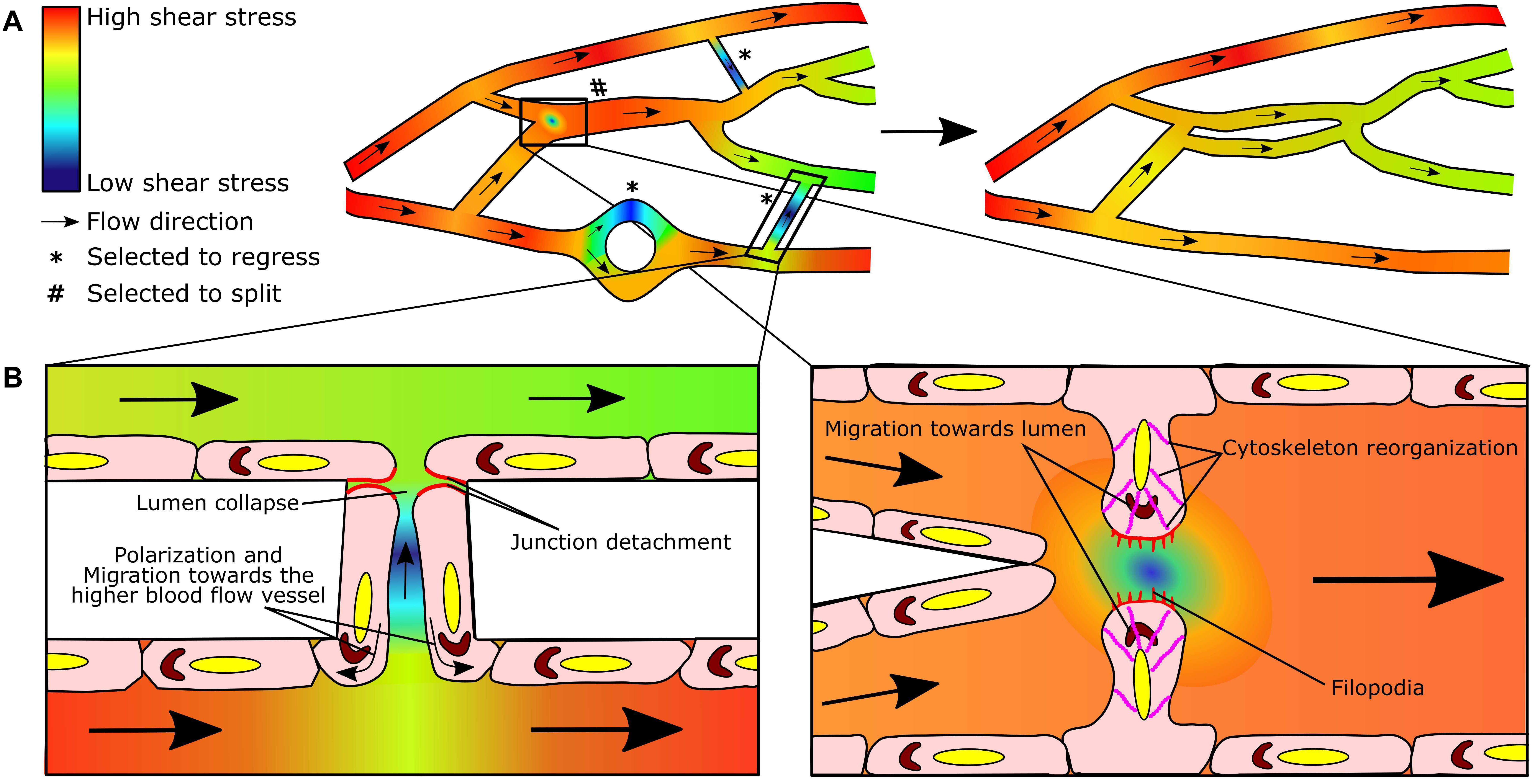
Figure 2. Blood flow-driven capillary pruning and splitting: two sides of the same coin. (A) Heterogeneous flow distribution in dynamic microvascular networks results in shear stress gradients (left). As a consequence, lower perfused segments will be selected to regress (*) and highly perfused segments to split (#) in order to redistribute flow in a more efficient manner in the remodeled network (right). (B) Endothelial cells sense the blood flow and shear stress gradients and respond by elongating and aligning in the direction of flow. In the case of pruning, endothelial cells in the regressing segment (left panel) detach from their neighbors (red) during lumen collapse, polarize (Golgi in brown) and migrate toward the region of higher flow in the adjacent vessel (curved arrows). In capillary splitting (right panel), endothelial cells close to shear stress gradient at the bifurcation, reorganize their cytoskeleton (pink), protrude luminal filopodia (red), and polarize (Golgi in brown) and migrate toward the lumen to form the intraluminal pillar; note the area drawn with low blood flow/shear stress at the nascent pillar which could be permissive for filopodia growth and fusion. Heat map colors indicate the predicted/simulated shear stress values in the modeled microvascular network. Straight arrows indicate the flow direction. Adapted from Chen et al. (2012).
Blood Flow Basics
Blood flow refers to the movement of the blood through the vessels. Flow is typically characterized based on certain physical parameters yielding different types of flow (Chiu and Chien, 2011). The flow is considered laminar, that is smooth and continuous, when all the fluid particles flow in parallel fluid layers. Otherwise, flow is defined as turbulent when it experiences irregularities and chaotic motion as it occurs at high velocities or in certain geometries such as big curvatures, bifurcations, anastomoses or stenotic sites. In general, the microcirculation responds to a steady and laminar flow. However, this assumption cannot be made in larger vessels like arteries where the flow is pulsatile due to the heart beat. Another type of flow observed in the vasculature and maybe relevant for remodeling events is reciprocating flow. In reciprocating or oscillatory flow, the velocity oscillates back and forth with a given frequency, resulting in a minimal forward net blood flow. Impinging flow can be found mainly at arterial bifurcation apices. This type of flow has classically a T-shaped geometry, where the inlet with high velocity collapses against a wall generating a stagnation point (Ostrowski et al., 2014).
Wall shear stress (referred as shear stress hereon) is the force per unit area acting on the vessel wall performed by the blood flowing through the vessel. It has pressure units (Pascal, Pa, in IS; in vascular research dyn/cm2). This force is tangential and depends on two parameters: the viscosity of blood and the rate at which the velocity of blood changes along the vessel radius (shear rate). The shear rate could be in theory correlated with vessel diameter, but only taking into consideration the instantaneous volumetric flow rate and velocity field through that vessel. In addition, since the blood is in reality a non-Newtonian fluid, its viscosity will change depending on this shear rate (Eckmann et al., 2000). Recent computational efforts have also shown the essential role of circulating cells, often disregarded in theoretical in silico simulations, in modulating blood viscosity and regional shear stress (Zhou et al., 2020). Moreover, the geometry of the microvascular network also influences shear stress patterns and values, particularly at curvatures and bifurcations (Chiu and Chien, 2011). Therefore, even though in principle shear stress could be expected to be high in small vessels, in regressing capillaries (with net blood flow practically null) and duplicating capillaries (with altered blood flow related to the geometry), shear stress values are rather low. In fact, shear stress and fluid velocity are simulated together and correlate in the retina and other vascular plexus (Filipovic et al., 2009; Szczerba et al., 2009; Bernabeu et al., 2014). In Table 1 shear stress values at different vascular territories and developmental stages are included as a reference.
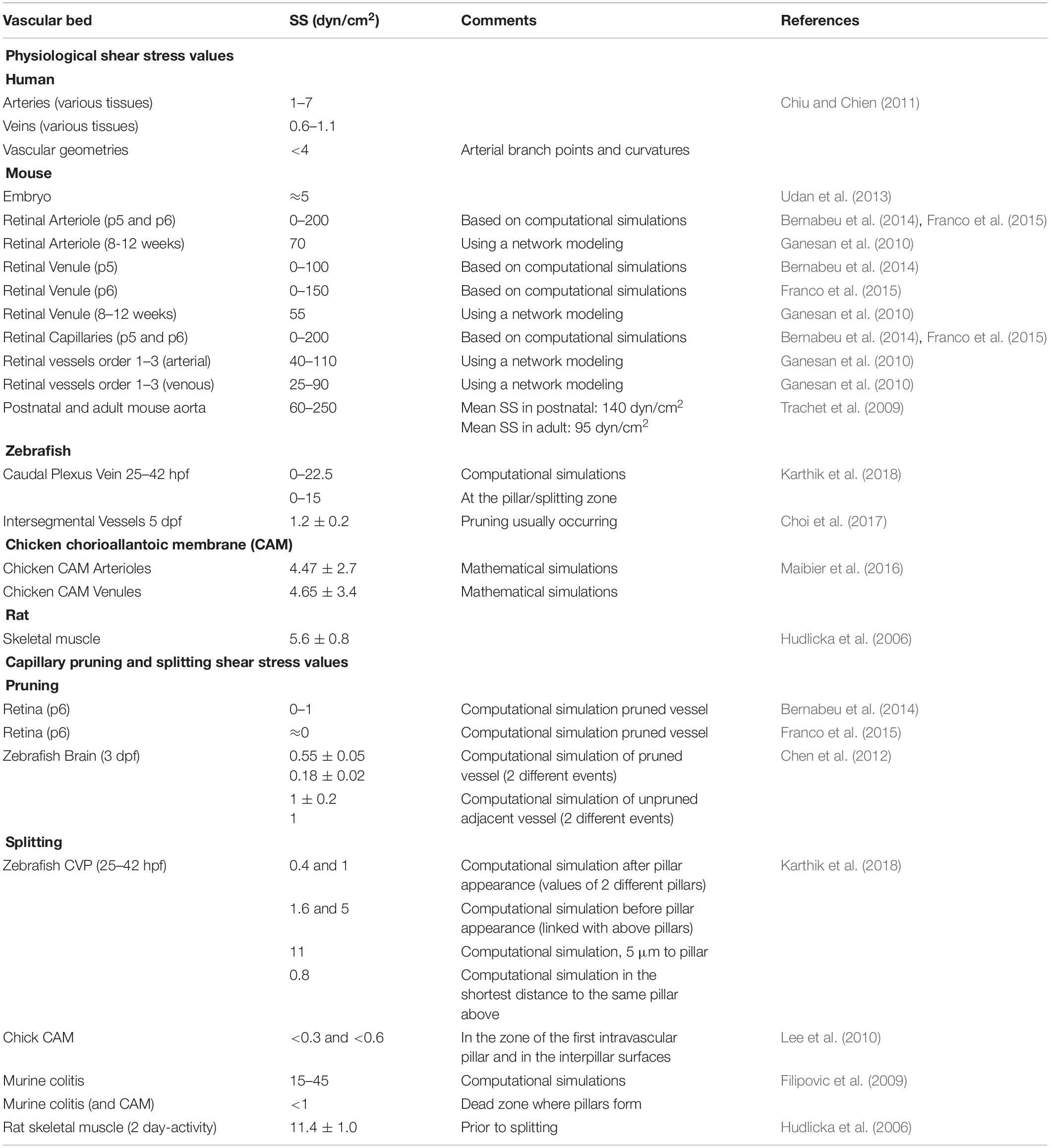
Table 1. Shear stress in microvascular remodeling. Summary of shear stress (SS) values in selected vascular territories under homeostatic and microvascular remodeling conditions in vivo. Note that capillary shear stress values obtained by computational approaches are based on theoretical input pressure values into the simulated network and may not accurately reflect in vivo physiological values.
Shear stress is sensed by the endothelial cells in the inner lining of the vessels and it is a major determinant of endothelial cell behavior (Chiu and Chien, 2011). But it is not only the magnitude of shear stress but its fluctuations that determine the responses of endothelial cells and therefore influence capillary remodeling. Time-related parameters as duration, ramping rate and frequency/oscillations of shear stress have differential impact on endothelial cell morphology and behavior (Yoshino et al., 2017). As an example, long exposure to disturbed instead of laminar flow upregulates pro-inflammatory genes and proliferation, which predisposes to atherosclerosis (Chiu and Chien, 2011; Mack et al., 2017), but short exposure to changing shear stress direction may underlie endothelial cell morphological changes relevant to capillary remodeling (Wang et al., 2013). And abrupt increase in shear stress augments endothelial cell membrane fluidity while gradual increase does not (Butler et al., 2002). Local changes and the 3D geometry of the network also generate shear stress spatial gradients between adjacent or nearby endothelial cells in vitro (Mack et al., 2017) or vascular segments in vivo (Franco et al., 2015). The pattern of shear stress is different in straight, branched and curved regions of the vasculature (Karino and Goldsmith, 1980; Karino et al., 1987; Nerem, 1993; Colangelo et al., 1994; Chien, 2003) and this can be related with the preference for Y and polygonal geometries in capillary splitting (Filipovic et al., 2009; Karthik et al., 2018) and H or O (loop) geometries in capillary regression (Bernabeu et al., 2014; Barbacena et al., 2016).
Blood Flow and Capillary Pruning
Early reports pointed to endothelial cell apoptosis as the main actor in vessel regression (Meeson et al., 1999; Hahn et al., 2005; Lobov et al., 2005; Wang et al., 2011; Simonavicius et al., 2012). More recent works in the mouse yolk sac and postnatal retina among others have shown however that blood flow is critical to induce dynamic endothelial cell responses (le Noble et al., 2004; Udan et al., 2013; Franco et al., 2015). In particular, endothelial cells elongate and align along shear stress axis and gradients of shear stress induce endothelial cell polarization and directed migration toward the higher blood flow adjacent vessel during capillary pruning (Franco et al., 2015; Barbacena et al., 2016) (Figure 2B). Moreover, studies such as those performed in the brain vasculature of zebrafish suggest the existence of a flow threshold for vessel regression (Chen et al., 2012).
This new concept establishes that, contrary to expected, capillary endothelial cells are not quiescent, but subjected to flow changes that regulate their polarization and migratory behavior. It has been proposed that differential blood flow/shear stress patterns in juxtaposed vessels drive asymmetries in cellular movements causing endothelial cells to migrate toward high flow neighboring vessels, thus favoring regression and pruning of the low blood flow capillaries (Lucitti et al., 2007; Chen et al., 2012; Kochhan et al., 2013; Franco et al., 2015). Strong flow/high shear stress seemed to attract endothelial cells making them polarize and migrate against blood flow (Tzima et al., 2002; Franco et al., 2015; Chang et al., 2017). Indeed, linear regression analysis identified a strong correlation between high shear stress values and cell polarization (Franco et al., 2015). But, how is blood flow and shear stress in the regressing segment? Quantification of blood flow and shear stress in vivo is challenging in particular in mouse models, and most information about shear stress values and patterns in vivo, in particular in capillaries, is derived from computational analysis and simulations. These models provide cues about these values in vascular networks with active capillary pruning as the mouse postnatal retina or the brain and the intersegmental vessels in zebrafish (Chen et al., 2012; Franco et al., 2015, 2016). In the postnatal mouse retina simulations predicted shear stress values up to 200 dyn/cm2 at arterioles, 100–150 dyn/cm2 at venules and 200 dyn/cm2 in normal capillaries to values near 0 dyn/cm2 in regressing capillaries which correlated with the percentage of cells aligned and polarized in each of these territories (Franco et al., 2015). Indeed, although dynamic quantification of shear stress cannot be analyzed, comparison of simulated distribution of blood flow and shear stress between P5 and P6 neonatal retinas suggest the presence of spatial and temporal gradients that could underlie pruning events (Bernabeu et al., 2014). Moreover, in the brain zebrafish vasculature capillaries with blood flow below a certain value were selected to regress (Chen et al., 2012). Table 1 shows shear stress values observed or simulated in the context of vessel regression. Of note, live-imaging in zebrafish brain vasculature demonstrated that regressing vessel segments exhibit low or reciprocating flow, which decreased irreversibly prior to the onset of regression (Chen et al., 2012). The shear stress threshold to enter the regression program seems variable and depends on relative levels on juxtaposed vessel segments indicating the need for spatial shear stress gradients (Chen et al., 2012). In relation to this, vascular segments with lower shear stress segments in a range between 2.5 and 10 dyn/cm2 often contained endothelial cells with very low axial or misaligned polarity vectors in the intersegmental vessels of zebrafish (Franco et al., 2015). However, in this state, endothelial cells were able to sense the onset of flow and responded dynamically polarizing against the direction of flow and migrating toward the highly perfused neighboring vessel. This paradoxical phenomenon, also observed in capillary splitting, underscores the relevance of shear stress ramping and gradients to trigger endothelial cell responses leading to microvascular remodeling. Finally, it has been observed that pruning of capillary segments often occurs at locations with H or O (loop) geometries, usually in flat vascular networks, but how bifurcations and intersections affect shear stress and thus endothelial cell migration and how capillary pruning may occur in 3D complex networks remains poorly characterized.
Blood Flow and Capillary Splitting
Tiny holes representative of intraluminal pillars during intussusceptive angiogenesis were visible in vascular segments that were dilated and at triple or quadruple branching points in several tissues and organs (Patan et al., 1992), already indicating that blood flow and shear stress magnitude or gradients were relevant for this remodeling process. Indeed, a sustained increase in blood flow of between 50 and 60% in the chorioallantoic membrane of chicken was reported to increase shear stress and stimulate the creation of intraluminal pillars, leading to the division of the vascular sinus (Burri et al., 2004). Moreover, the increased vascular volume in the skeletal muscle induced by exercise or the administration of vasodilators as prazosin also led to capillary splitting (Zhou et al., 1998). In the zebrafish caudal vein plexus, blood flow is an essential requirement for vessel duplication as demonstrated by its occurrence only in perfused areas and by enhanced or reduced pillar formation observed with pharmacological strategies that increase (adrenergic agonist) or decrease (muscle contractility inhibitor) blood flow (Karthik et al., 2018). In models of experimental colitis, vasodilation of the intestine feeding arterioles has been reported (Mori et al., 2005) and impairing vasodilation prevents capillary splitting in the inflamed intestine (Esteban et al., 2020). Therefore, increased blood flow is considered a prerequisite for initiation of capillary splitting aimed to restore blood flow and shear stress in the duplicated segments (Egginton, 2011). Indeed shear stress will promote cellular events such as loss of apical-basal endothelial cell polarity, cytoskeleton and membrane rearrangements and filopodia protrusion to form the intraluminal pillars (Karthik et al., 2018) (Figure 2B). Although this review is focused on the role of shear stress in capillary remodeling, it is worth mentioning that increased blood flow may trigger splitting by elevating not only shear stress but also circumferential wall stress, which is similarly sensed by capillary endothelial cells (Lu and Kassab, 2011). How the increased blood flow induces the endothelial cell intraluminal rearrangements needed for the formation of pillars is far from being understood yet.
Since blood flow and shear stress cannot be quantified in the capillaries of the mucosa vascular plexus in the intestine, computational modeling has helped understand their contribution to capillary splitting. Previous computational studies of shear stress maps indicated that formation of new intravascular pillars was limited to regions of lower shear stress, less than 1 dyn/cm2, that were constrained between high shear stress in the pillar midportion and the lateral vessel wall (Filipovic et al., 2009) (Table 1). In parallel, flow modeling showed that these pillars were favored in areas with high flow (Szczerba et al., 2009). Consequently, the accepted paradigm is that development of the pillars is caused by increased flow but it occurs in low shear/turbulent flow areas (Djonov et al., 2002; Lee et al., 2010). This paradox (similar to capillary pruning) could be explained if the high flow was not continuous or whether it occurred after a period of decreased flow as observed in the rabbit ear (Styp-Rekowska et al., 2011). These observations would suggest a role for shear stress gradients and ramping in capillary splitting, so that, perhaps, sharp gradients of both shear stress and flow promote capillary duplication in areas which normally have very low shear stress. Indeed, this has been confirmed in an elegant study performed in zebrafish in which blood flow velocity and shear stress profiles have been simulated from live microscopy videos of the caudal vein plexus (Karthik et al., 2018). The authors observed that there was a steep drop in shear stress (about 2.5–5 dyn/cm2) in the region of pillar formation and initial fusion and then, as the pillar grows and splitting occurs, shear stress increased gradually (between 10 and 12.5 dyn/cm2).
Beyond Blood Flow in Capillary Remodeling
At first, it was unclear how hemodynamic forces promoted endothelial cell migration in the mouse yolk sac (Udan et al., 2013), but it was proposed that endothelial cells could be responding to shear stress or substrate tension gradients (mechanotaxis) (Chiu and Chien, 2011) and/or to chemical signals released by cells sensing the force (chemotaxis). In microvascular remodeling, previous reports indicated that although blood flow is a critical driver, it needs to be coupled with other physical, chemical and cellular cues (Georgieva et al., 2019).
In the context of capillary pruning in the mouse postnatal retina, computational modeling studies stated that soluble signals such as VEGF (induced in low perfused regions) will complement blood flow actions in the vascular regression process (Watson et al., 2012). In addition to its role in optimizing network functionality, microvascular remodeling also aims at preventing the formation and persistence of preferential arteriovenous shunts which would negatively impact oxygen distribution (Pries and Secomb, 2014). In computational simulations during the remodeling of pillars into a differentiated network, it was predicted the need of a chemical inhibitor to avoid arteriovenous shunts (Szczerba et al., 2009). More recently, blood flow has been shown to act in coordination with endothelial cell collective migration for maintenance or avoidance of bifurcations which together with molecular signals and network geometry will contribute to capillary remodeling (Edgar et al., 2020).
In mouse experimental colitis, computational models pointed to the relevance of mechanical and chemical cues such as oxygen, metabolites and growth factors to fully explain capillary splitting together with blood flow and shear stress (Szczerba et al., 2009). It was proposed that tissue mechanical properties would regulate shear stress and lead to endothelial responses (recently reviewed in Gordon et al., 2020), but also to the secretion of chemical signals that will contribute to the splitting process and to the crosstalk with supporting cells (Szczerba et al., 2009; Egginton, 2011). These secreted chemical signals could promote or inhibit pillar growth fine-tuning the remodeling of the network. Most interestingly, they predicted the properties of such soluble factors which should have a diffusion coefficient between 10–9 and 10–12 m2/s and a molecular mass of 1 to 100 kD. The influence of mechanical tension on the process of capillary splitting has directly been demonstrated in the chorioallantoic membrane in which stretching stimulated capillary splitting (Belle et al., 2014).
Interstitial flow also leads to shear stress and other pressure forces on blood vessels that endothelial cells are able to sense and that may be contributing to some extent to the remodeling of the vascular tree. The role of interstitial flow has mostly been studied in the context of vascular morphogenesis and sprouting angiogenesis. However, although shear stress produced by interstitial flow is in the order of 10–3 dyn/cm2 (Rutkowski and Swartz, 2007; Shirure et al., 2017), orders of magnitude below those produced by blood flow, significant endothelial cell elongation and actin filament rearrangement has been reported for shear stress between 5.15 × 10–2 and 2.15 × 10–1 dyn/cm2 (Vickerman and Kamm, 2012), suggesting that interstitial flow may also contribute to capillary remodeling events. The effect of interstitial flow through the vessel wall (transmural) or around the vessel wall through the surrounding endothelial cells has been analyzed in different angiogenic contexts and in combination with VEGF actions (Song and Munn, 2011; Vickerman and Kamm, 2012). Interstitial flow was concluded to drive endothelial cell migration toward vessels that have higher microvascular pressure, a phenomenon reminding of capillary regressing events. Another relevant effect is the elimination of morphogen gradients since physiological values of interstitial fluid could dissipate morphogen gradients within hours in a magnitude-dependent manner (Shirure et al., 2017). Through these actions on endothelial cell migration and on the distribution of the biomolecular endothelial cell environment, interstitial flow may constitute an underestimated player in capillary remodeling.
Common Features in Capillary Pruning and Splitting
Therefore, both modes of capillary remodeling rely on blood flow forces. In particular, on spatial-temporal gradients of shear stress established at particular network geometries as the intersections between capillaries and adjacent larger vessels (in pruning) or between adjacent capillaries (in splitting). These gradients coupled with capillary caliber changes drive endothelial cell rearrangements leading to cell migration toward the high flow/shear stress region either the adjacent highly perfused larger vessel (in pruning) or the lumen (in splitting). In addition, although both pruning and splitting events occur at sites of local lower shear stress, the observations point to high blood flow as the main driver, indicating that dynamics and ramping of shear stress changes are critical drivers. Finally, chemical signals as oxygen or VEGF and mechanical cues as tissue stiffness or interstitial flow can also contribute to both capillary pruning and splitting.
The co-existence of both remodeling processes can be envisioned in the entity called intussusceptive pruning in which pillar extension occurs in non-axial directions (Mentzer and Konerding, 2014); this has been observed in the extraembryonic vessels of chick embryos (Lee et al., 2011) and at the branch angles of bifurcating vessels in experimental mouse colitis (Ackermann et al., 2013). Moreover, reported images of the vascular plexus behind the sprouting front in the postnatal mouse retina show holes that could remind of intraluminal pillars (Turner et al., 2017), supporting the possible co-occurrence of both remodeling processes in this capillary plexus. Nevertheless, capillary splitting and pruning are still often considered independent and distinct processes. However, common hemodynamic regulatory cues together with the subsequent changes in the number and perfusion of microvascular segments lead us to propose that pruning and splitting could be the two sides of the same coin. That is, two different mechanisms of microvascular remodeling that are coordinated in time and space in response to changes in tissue oxygen demand and in blood flow distribution with the final aim to achieve an optimized and refined vascular network. It would not be unexpected that molecular mechanisms are also shared.
Mechanisms in Blood Flow-Driven Capillary Remodeling
Shear stress sensed by endothelial cells induces different cellular responses that would finally lead to capillary remodeling in vivo (Davies, 1995; Wang et al., 2013). In vitro studies showed firstly that endothelial cells elongate and align in a shear stress-dependent manner with alignment requiring longer exposure times (Levesque and Nerem, 1985), and secondly that the higher the laminar flow and shear stress the larger the proportion of endothelial cells that polarize and migrate against flow direction (Table 2). These findings would suggest that endothelial cell elongation, alignment and polarization and migration against blood flow are sequential responses to higher and longer shear stress values, but the reality is more complex and depends on the endothelial cell type, the onset of blood flow (in vitro is usually abrupt) and the kinetics of the process under investigation (Dieterich et al., 2000) (Table 2). And what is it known about values of shear stress and endothelial cell responses in vivo? Without disregarding the potential effect of vessel caliber and geometry as well as matrix composition in endothelial cell elongation and orientation in vivo (Campinho et al., 2020; Gordon et al., 2020), previous reports (Tkachenko et al., 2013; Franco et al., 2015, 2016; Chang et al., 2017; Poduri et al., 2017) and our own observations indicate that endothelial cell elongation and polarization against the flow direction positively correlate with the shear stress magnitude estimated in different arterial beds and in capillaries during pruning (Table 1). These findings emphasize the influence of spatiotemporal regulation of blood flow/shear stress and the possible existence of threshold shear stress values for differential endothelial cell responses in vivo (Table 2).
Molecular actors involved in blood flow-mediated endothelial cell responses, in particular the mechanosensor complex formed by PECAM-1/VE-cadherin/VEGFR2 at the junctions, have already been reviewed (Korn and Augustin, 2015; Campinho et al., 2020; Gordon et al., 2020). In this review, we will comment only some of the molecular pathways known to be regulated by shear stress and that seem to be relevant to endothelial cell responses in the context of blood flow-driven pruning and splitting (Tables 3, 4).
The new concepts in capillary remodeling predict that the molecular pathways related to sensing blood flow/shear stress by endothelial cells and its transduction into extracellular and intracellular gradients will be of special relevance to modulate the process. The non-canonical Wnt signaling pathway (Wnt11 and Wnt5a) regulates vessel regression in the mouse retina by affecting the sensitivity of the vasculature to shear stress (Franco et al., 2016). The role of BMP signals can be more complex since BMP9 cooperates with the primary cilium to prevent vessel regression under low shear stress (Vion et al., 2018) but the absence of endothelial BMP-SMAD1/5 signals results in reduced vessel regression with aberrant vascular loops and arteriovenous malformations in areas with high blood flow in the mouse retina (Benn et al., 2020). Endoglin, a TGFβ/BMP co-receptor, is required to couple flow-mediated mechanical cues with endothelial cell migration and shape modulating final vessel remodeling (Lobov et al., 2011; Boriushkin et al., 2019). The role of novel mechanosensors (in addition to the well-known PECAM-1/VE-cadherin/VEGFR2 complex) in driving polarized endothelial cell responses is also an active field of research (see review by Campinho et al., 2020). Among these mechanosensors, Piezo 1 increases intracellular calcium in response to shear stress and regulates endothelial cell migration via nitric oxide production and its absence leads to defects in embryonic vascular remodeling suggestive of defective pruning (Li et al., 2014; Ranade et al., 2014). Downstream of Piezo 1, the transcriptional factor Yap/Taz, responsive to both shear stress and endothelial cell stretching (Nakajima et al., 2017; Neto et al., 2018), has been proved to be active during capillary remodeling by inducing actin polymerization; this resulted in a decrease in vascular regression when Yap/Taz pathway is silenced (Nagasawa-Masuda and Terai, 2017). Endothelial K+ channel Kir2.1 has recently been identified as a shear stress sensor whose absence led to decreased endothelial alignment in retinal endothelium and reduced capillary pruning near the angiogenic front of postnatal retinas (Boriushkin et al., 2019). The quick response of these mechanosensors to shear stress (Table 3) underscores their role as initial upstream regulators of early endothelial cell responses as alignment and polarization required for capillary pruning (Table 4). Of interest is the transcription factor Dach 1 that is regulated by laminar flow specifically, being expressed in arteries subjected to low flow in which it stimulates endothelial cell migration against blood flow (Chang et al., 2017) suggesting a similar not-yet investigated role during capillary pruning. The actions of Notch pathway in capillary pruning seem more complex and pleiotropic; inhibition of Dll4/Notch was shown to prevent retinal capillary regression in the mouse retina by regulating vasoconstriction and blood flow (Lobov et al., 2011) and deletion or loss of its inhibitor Nrapr resulted in enhanced vessel regression, likely by the additional modulation of Wnt signaling (Phng et al., 2009). Moreover, since lower Notch activity correlated with more mobile VE-cadherin at endothelial junctions (Bentley et al., 2014a), differential Notch activity may coordinate endothelial cell arrangements during polarized migration in capillary regression (Franco et al., 2015). Accordingly, endothelial Notch1 was demonstrated to be responsive to shear stress and necessary for the maintenance of junctional integrity induced by laminar shear stress (Mack et al., 2017). However, shear stress sensing needs to be transduced into polarized intracellular signals to promote alignment, polarization (with the Golgi in front of the nucleus toward the migrating edge) and directed migration. In this line, the small GTPase Rac1, regulator of actin polymerization and cell migration, is required for vessel regression in the zebrafish brain (Chen et al., 2012) and polarized Rac1 subcellular gradients are induced by laminar (15 dyn/cm2) but not disturbed flow in endothelial cells in vitro (Shao et al., 2017). Similarly, phosphoinositide gradients are required for a balanced capillary pruning induced by VEGF since the absence of CDP-diacylglycerol synthase-2 (CDS2), a metabolic enzyme that controls phosphoinositide recycling, in mouse and zebrafish showed increased endothelial cell migration and vessel regression (Zhao et al., 2019). VEGF was considered an essential player in vascular regression occurring after deprivation of VEGF or VEGF-induced signals which led to endothelial cell apoptosis in different tissues and contexts (Meeson et al., 1999; Tang et al., 2004; Baffert et al., 2006). Previous in vivo and in silico analysis in the postnatal mouse retina pointed to VEGF as a complementary actor together with blood flow in capillary pruning in this context (Watson et al., 2012). However, how VEGF precisely participates in endothelial cell migration against blood flow during capillary pruning remains undefined.
Much less is known about the mechanisms underlying capillary splitting or duplication since there is no optimal in vitro model for its study and the endothelial cell processes involved are less understood. In a pioneer attempt to understand the molecular pathways involved in capillary splitting, Egginton’s group aimed at identifying genes differentially expressed in the context of capillary splitting (vasodilator-treated) versus sprouting (agonist-excised) in the skeletal muscle and they found that endothelial nitric oxide synthase (eNOS) and neuropilin-1 were upregulated (Egginton, 2011). Nitric oxide is a downstream target of the laminar flow-induced transcription factor Klf2 (Nayak et al., 2011) but also by shear stress-stimulated glycocalyx on endothelial apical side (Bartosch et al., 2017). Indeed, nitric oxide produced by the endothelial cells is essential not only to induce the endothelial cell rearrangements required for intraluminal pillar formation and splitting, in particular luminal filopodia protruding mostly from the inter-junctional regions, but for capillary splitting to occur as demonstrated in the skeletal muscle of vasodilator-treated eNOS-deficient mice (Baum et al., 2004; Williams et al., 2006a). A recent report from our group has added a layer to nitric oxide regulation showing that TSP1 cleavage by the protease MT1-MMP promotes endothelial cell production of nitric oxide favoring vasodilation and capillary splitting during experimental colitis (Esteban et al., 2020); whether in this model nitric oxide mainly acts by inducing vasodilation of the feeding arterioles or has additional actions in the endothelial cell rearrangements to form the pillars during capillary splitting still needs to be established. Of note, nitric oxide is a soluble factor with properties close to those predicted for the soluble factor required for refining capillary splitting according to in silico models (Filipovic et al., 2009). The role of VEGF in the process is supported by its expression in the chorioallantoic membrane microvasculature and the delay in capillary splitting when VEGF was inhibited (Baum et al., 2010) and by the induction at high doses of dysfunctional capillaries by splitting (Gianni-Barrera et al., 2013); VEGF actions in this context can be modulated by PDGFB and EphrinB2/EphB4 pathways (Gianni-Barrera et al., 2018; Groppa et al., 2018). Novel actors include the inhibitor of proteases RECK which regulates non-sprouting (likely splitting) vascular remodeling during embryonic development (Chandana et al., 2010) and Notch (Dimova et al., 2013) and endoglin, whose inhibition led to enhanced capillary splitting by distinct mechanisms (Hlushchuk et al., 2017).
Of course, the cellular and molecular understanding of capillary remodeling and its regulation is far from being complete. Other possible cues not much investigated yet, but with possible roles in regulation of endothelial cell morphology, include endothelial cell junction components that may be a critical factor to explain rearrangements during both regression and splitting. In particular, VE-cadherin forms part of the mechanosensory complex together with PECAM-1 and VEGFR2 (Tzima et al., 2005) and, it has been reported that short-term reciprocating flow reduces VE-cadherin localization at the junctions while sustained exposure to pulsatile flow reinforces it (Miao et al., 2005). Since reciprocating flow is observed at the regressing segment (Chen et al., 2012), subsequent reduction in junctional VE-cadherin may favor destabilization of endothelial junctions and allow endothelial cell migration to the adjacent high flow vessels. Cues from the basement membrane on which directed endothelial cell migration takes place toward the adjacent vessel can also be important as suggested by recent studies with gradients of a collagen IV peptide in vitro (Du and Gao, 2019). Actin cytoskeleton may be an additional actor since short exposure to laminar flow induces apical F-actin reorganization and filopodia (Galbraith et al., 1998; Zeng and Tarbell, 2014) (Table 2) and actin filaments are visualized in the pillars during capillary splitting although their ability to generate the required force for intraluminal protrusions under blood flow is still unclear (Paku et al., 2011; Mentzer and Konerding, 2014). Finally, other players in endothelial directed migration as the Apelin GPCR (Kwon et al., 2016), the actin cytoskeleton regulators cdc42 (Lavina et al., 2018) and Nck (Chaki and Rivera, 2013), the atypical MAP kinase Erk5 (Spiering et al., 2009), the TGFβ/BMP receptor Alk1 (Rochon et al., 2016) or the adapter p130Cas (Evans et al., 2017) could also be good candidates to participate in capillary remodeling in vivo (Tables 3, 4).
New Methodological Approaches and Tools to Understand Capillary Remodeling in vivo and in vitro
It is clear that capillary remodeling events depend largely on local spatiotemporal gradients of blood flow, but also cellular gradients (molecular signals) and luminal and tissue gradients (soluble factors), which forces us to change the paradigm of how to analyze cellular and molecular actors involved in these processes. Remodeling cannot be analyzed in bulk approaches but at single cell level as also recently reviewed (Campinho et al., 2020). This need demands advanced imaging techniques (with reporters and probes to track possible actors and signals) in vitro, ex vivo, and in vivo but also screening for new players by single-cell and spatial techniques for protein changes and modifications since some of these events occur in a time-scale that would not allow major gene changes (Lundberg and Borner, 2019).
Single-Vessel and Single-Cell Image Analysis
Although in vitro models have provided relevant cues about cellular and molecular mechanisms involved in endothelial cell responses to changes in blood flow gradients, in vivo models allow a closer look at the endothelial cell behavior in the real physiological context. In vivo models used in the context of microvascular remodeling have typically focused on either immature microvascular networks during development or in pathological scenarios. Most advances have been made by the use of animal models such as the postnatal mouse retina (Connolly et al., 1988) and the intersegmental vessels and the brain vasculature in the transparent zebrafish (Chen et al., 2012; Franco et al., 2015) for segment pruning and the choriollantoic membrane of the chicken (Feinberg et al., 1986), the caudal venous plexus of the zebrafish (Karthik et al., 2018), the skeletal muscle (Hudlicka et al., 2006) and the experimental mouse colitis (Esteban et al., 2020) for capillary duplication.
Once endothelial cell migration triggered by changes in the blood flow sensing was established as the main actor in the vascular remodeling, markers implicated in the migration of the cells became an ideal target to monitor the behavior of these cells and also identify the potential vessels involved in this process. Thus, transgenic reporter animals became an essential tool in the investigation of vascular remodeling. Transgenic lines carrying endothelial cell fluorescence reporters have allowed live-imaging and visualization of the dynamics of the process in zebrafish (Scheer and Campos-Ortega, 1999; Lawson and Weinstein, 2002; Chen et al., 2012). More recently, Franco’s group has generated the GNrep transgenic mouse line with a double fluorescence reporter for the Golgi apparatus and the endothelial cell nucleus which allows a direct analysis of endothelial cell polarization in vascular territories undergoing remodeling (Barbacena et al., 2019). Notably, the use of the Raichu Rac1 FRET sensor established the role for the activity of this small GTPase in regulating directed endothelial cell migration during capillary pruning in the brain vasculature of zebrafish (Chen et al., 2012). LifeAct reporter has recently been used for visualization of endothelial cell filopodia and dynamics in the postnatal mouse retina ex vivo (Riedl et al., 2008; Prahst et al., 2020) and it could be a useful tool to analyze cytoskeletal rearrangements of endothelial cells during capillary remodeling. A better knowledge of the molecular actors involved in each step of segment pruning or splitting could help designing new fluorescent reporter animal lines.
In addition to time-lapse microscopy in amenable models as zebrafish, confocal microscopy and 3D image analysis have largely advanced our understanding of capillary remodeling events (Campinho et al., 2020). Confocal microscopy in the quasi-2D flat mount postnatal retina has allowed precise quantification and characterization of “empty sleeves” (collagen IV positive, endothelial cell negative), the hallmark of capillary pruning events (Figure 3A). The challenge of exploring capillary pruning in more complex 3D vascular plexus remains and novel confocal microscopy-based 3D image processing tools and algorithms may help comprehend this process (Gkontra et al., 2018, 2019). Likewise, confocal microscopy of vessel-stained tissues such as whole-mount inflamed intestine combined with 3D-image rendering has turned into an approach that provides sufficient resolution to identify and quantify capillary holes/pillars, loops, and duplications (Figure 3B) (Karthik et al., 2018; Esteban et al., 2020), all hallmarks of capillary splitting, circumventing the limitations of previously used corrosion cast techniques and electron microscopy (Nowak-Sliwinska et al., 2018). Light-sheet fluorescent microscopy (LSFM) has recently been applied to the quantitative 3D and 4D analysis of endothelial cell motility and filopodia dynamics in blood flow-free ex vivo postnatal mouse retina (Prahst et al., 2020). Advanced microscopy techniques will undoubtedly improve our knowledge about capillary remodeling in more complex vascular beds what combined with the use of fluorescent reporter animal lines may provide unprecedented visualization and information about these events.
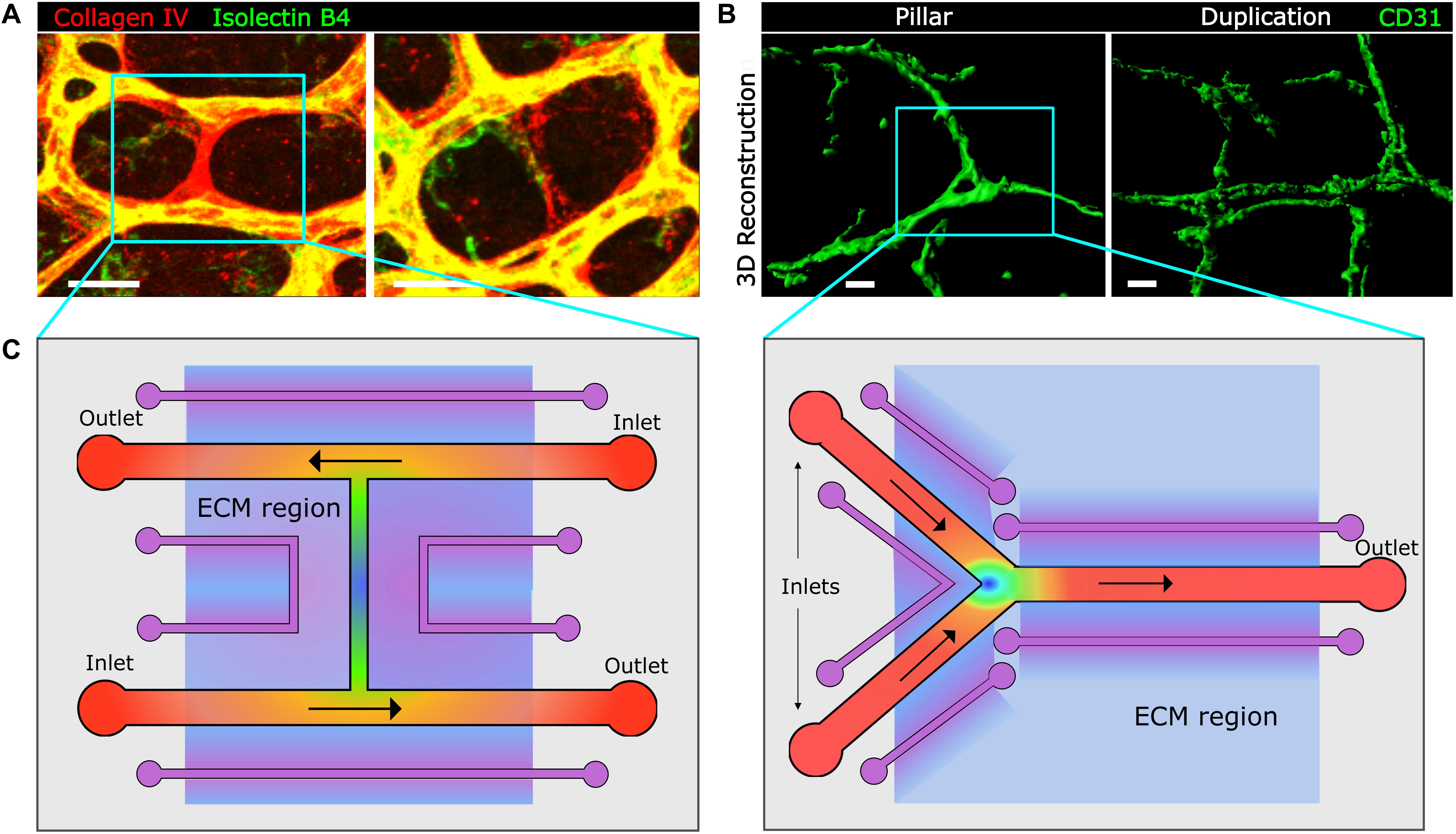
Figure 3. Image-based approaches to capillary remodeling in vivo and proposed device-based simulation in vitro. (A, B) Examples of whole-mount staining and confocal microscopy for the visualization and analysis of capillary pruning in the mouse postnatal retina (A) and of capillary splitting in the inflamed mouse intestine by means of 3D reconstruction with Imaris software. Scale bar, 20 μm. (C) Top view of proposed microfluidic chips for the study of capillary regression in an H-type geometry (left panel) and of capillary splitting in Y-type geometry (right panel), mimicking the geometries found in microvascular networks (blue boxes in panels A and B). Inlets and outlets for flow are indicated and are exchangeable depending on the preferred direction of flow in the different segments. Vessel channels are covered with a basement membrane and endothelial cells. In purple auxiliary channels for creating biochemical gradients along the extracellular matrix (ECM)-like hydrogel region (light blue). This hydrogel could also incorporate cells for co-culture analysis. Heat map color represent theoretical shear stress values (as in Figure 2) for established flow gradient profiles and shading represent biochemical gradients along the ECM. The rest of the microfluidic chip would be made of PDMS.
Novel mathematical and computational tools can also be of great value to understand the changes and actions of blood flow and shear stress, specially their gradients and dynamic changes. Although continuous blood flow models can provide some information about blood flow fluctuations and drop pressure changes in the microvascular network by means of permeability tensors (Gkontra et al., 2019), capillary remodeling research will most benefit from discontinuous or segment-based models and simulations. This has been the base for the efforts made to simulate the complexity of the hemodynamic forces even in simple networks as the postnatal retina vasculature (Bernabeu et al., 2014) and the development of new tools such as PolNet (an open-source software; Bernabeu et al., 2018) which aimed at facilitating the combined analysis of blood flow and shear stress with cell polarization in order to understand their influence in capillary remodeling by comparison of values between neighbor segments and at different developmental stages. This tool is available and useful for the study of blood flow in low complexity network as retinas. It is worth mentioning that computational modelization of particles has just uncovered the essential role of circulating red blood cells in generating regional shear stress differences and thus in regulating vascular remodeling by modulation of blood viscosity (Zhou et al., 2020). Of note, new advances in mathematical approaches in the multidisciplinary field of Adaptive Systems could also help decipher the dynamic changes of endothelial cells responding to a variety of environmental signals in order to adopt the best network architecture possible (Bentley et al., 2014b).
In vitro Systems for Analysis of Microvascular Remodeling Under Flow: Microfluidics as a Promising Technology
In vitro flow chambers could be considered the preludes of microfluidic-based devices as they mimic hemodynamic shear stress in macroscale flow chambers with 2D endothelial cell monolayers. The two main models of in vitro flow chambers are the parallel plate flow chamber and the cone and plate viscometer with its corresponding modifications; in both cases, cells are cultured on a two-dimensional stiff surface and fluid is flown over the culture. While the parallel plate system has several advantages as its simplicity or its compatibility with imaging modalities, the effects of shear stress cannot be discriminated from those due to hydrostatic pressure. On the other hand, the cone and plate viscometer does allow to separate both effects but it has other limitations as its difficult combination with imaging and the need of continuously supply the culture with fresh medium (Papadaki and McLntire, 1999). The most popular commercial versions of flow chambers are the family products Bioflux (multi-well format with small fluid reservoir) and Ibidi (varied plate formats with larger fluid reservoir for longer experiments) which are widely used among researchers in the field. They are relatively easy to implement and offer advantages as its high reproducibility, good cell viability and technical support on-hand. But they come with limitations since they are not very versatile (fixed geometries and fluid properties) and they are expensive (disposable and non-reusable plates and consumables) (Roest et al., 2011). These type of 2D systems are mostly interesting to examine the role of shear stress exerted by different types of flow including laminar, pulsatile and reciprocating flow (Usami et al., 1993; Chien, 2007) but, in addition to the limitations already discussed, they do not incorporate the remodeling of the extracellular matrix (ECM) nor its mechanical properties, failing to fully recapitulate the complex 3D environment, geometries or the shear stress gradients found in vivo (Coluccio et al., 2019; Gordon et al., 2020).
Microfluidic chips are characterized by having channel sizes of tens to thousands of micrometers and dealing with volumes in the microliter to picoliter range; in fact, they are considered such when one or more channels have at least one dimension smaller than 1 mm. Switching from the macroscale to the microscale offers new advantages for researchers including a big reduction in the amount of reagents but more importantly a huge versatility for exploring new geometries and flow conditions (van Duinen et al., 2015). The main advantages of this technology are that it provides the possibility of isolating and controlling fluid properties, physical, chemical and biological stimuli while being compatible with several imaging modalities including time-lapse microscopy (Hsu et al., 2013). They can also be used in a high throughput manner so several conditions can be tested simultaneously (Hsu et al., 2013). Among the applications of interest in the miocrovascular remodeling field is the analysis of vascular cell responses to shear stress (Wong et al., 2012). Even though microfluidic technology is usually applied for quasi-2D models with laminar flow, in the last years new techniques implemented fully 3D models that offer a more physiological environment.
Microfluidic devices are typically built in PDMS (polydimethylsiloxane) because being it transparent, permeable to oxygen and compatible with soft-lithography and rapid prototyping techniques, it is a good option to build biocompatible devices for research with custom-made geometries. Nevertheless, further demands for deeper understanding of vascular phenomena made PDMS-hydrogel hybrid devices emerge as a better option for the study of angiogenesis and vascular remodeling (Bogorad et al., 2015; Gordon et al., 2020). This type of devices incorporates a hydrogel region surrounding the endothelial cell-covered microchannels which can be remodeled by cells and whose mechanical properties resemble much better the endothelial microenvironment. In addition, with the hydrogel regions, different types of biochemical gradients can be incorporated throughout the device. The possibility to control and model computationally both the biochemical and physical gradients (Nguyen et al., 2013) and the flow and shear stress distribution, timing and ramping along the device (Chiu and Chien, 2011) makes it possible to establish correlations between the different types of stimuli and the endothelial cell response. Given that intraluminal endothelial migration occurs in contact with the preserved basement membrane during capillary remodeling, it is worth mentioning that microfluidic systems including a basement membrane have been implemented for the study of endothelial cell permeability or cancer metastasis (Han et al., 2015; Kim et al., 2019; Coughlin and Kamm, 2020).
One drawback of lithographic techniques commonly used to produce the PDMS structure is the limitation to rectangular cross-sections rather than the natural cylindrical shape (Akbari et al., 2017), that could influence the outcome of the experiments due to pulling and traction forces at the corners of polygonal shapes (Rauff et al., 2019). Cylindrical cross-sections have been achieved with 3D microvessel templates by cross-linking the hydrogel around a needle or rod that is then removed. However, this technique is limited to linear geometries and larger diameters (60–200 μm). The report of a grid geometry for microvessel networks that resemble the highly branched native plexus, where blood passes from capillaries to arterioles and venules, could be of interest as a retina model since in both cases blood flow diverges and decelerates as it branches, and consequently it creates different shear stress patterns (Zheng et al., 2015). Going even further, fully hydrogel embedded systems that can incorporate other cell types such as pericytes in co-culture, are the most accurate at resembling the three-dimensional physiological microenvironment.
PDMS-hydrogel hybrid devices can incorporate branching and bifurcating regions of special interest for capillary splitting and other remodeling events due to the different flow patterns occurring at these sites. This type of devices have recently been used to produce impinging bifurcating fluid flow, laminar shear stress and transvascular fluid flow (Akbari et al., 2018) and also controllable disturbed flow patterns for the study of its effects on actin stress fiber and endothelial cell orientation (Tovar-Lopez et al., 2019). But how exactly does the direction, slope and average magnitude of shear stress gradients affect endothelial cell biological and molecular responses needs still to be understood. Due to the geometrical versatility and high reproducibility and control that microfluidic devices offer, this technology springs as a promising tool for a better understanding of these events. For example, a recent microfluidic device was able to produce areas with three different constant shear stress values and six different shear stress gradients and showed that human endothelial cell upstream orientation depends on gradient direction (Sonmez et al., 2020). Following a top-down approach (Hesh et al., 2019), we propose two prototypes of hybrid devices that on one hand are inspired on real microvascular networks in which capillary pruning and splitting occurs (Figure 3A,B), mimicking their preferential H and Y geometries; and on the other, they incorporate predicted shear stress values and spatiotemporal gradients, as discussed along this review, whose tuning and dynamics could only be recapitulated in these systems (Figure 3C). These prototypes could even represent better the in vivo microvascular remodeling context by including a physiologically relevant ECM, a basement membrane and supporting cells in co-culture.
Microfluidic devices offer several advantages. The influence of the environment on the microvasculature, in particular chemical factors, can be tested not only controlling its concentration but its gradients for example of WNTs, Notch-related molecules, VEGF, all with important roles in vessel pruning (Wong et al., 2012; Korn and Augustin, 2015). The possibility of time-lapse imaging allows to study the dynamics of microvascular remodeling events (Wong et al., 2012). Some devices can incorporate supporting cells such as pericytes (Rauff et al., 2019), contributors to microvascular remodeling. In vitro microfluidic models designed using solely human components may serve as a translational bridge between experimental animal models and human applications given the much larger shear stress values found in mouse compared to human vessels (Chiu and Chien, 2011; Zheng et al., 2015). Microfluidic models can also offer a platform to test and refine computational models. Mathematical and computational models require tight boundary conditions (like flow parameters at the inlet and outlet or pre-defined values at certain locations) that are impossible to control or measure in vivo. Since flow parameters can be tuned by the user in microfluidic chips and they are compatible with several imaging modalities and measuring techniques, microfluidic platforms could be a great complement to feed these theoretical models.
A bit aside from the classical microfluidic chips that have been described so far, but that may be relevant in the field, are organ mimetic microdevices and organ-on-a-chip devices. These technologies combine microfluidic technology with other culture systems as bioreactors and microchambers and computer control systems (Huh et al., 2011).
Even though the advantages of microfluidics versus macroscale flow chambers are vast, the fact that it has recently started to be exploited for biological research poses some difficulties related to their implementation by researchers. Protocols for biochemical assays and cellular cultures are established for macroscale conditions where the surface to volume ratio is small and the culture media to cell ratio is large. These conditions have to be carefully adjusted to the microscale to avoid artifacts and cell death and comparison with the results obtained in macroscale systems and classical biochemical assays have to be made with care. For PDMS devices, attention has to be paid with the surface coating for proper cell attachment (which is different to that in glass or polystyrene). Also, CO2 concentration and pH needs to be checked since, even though it is permeable to gases, too thick PDMS layers can hinder proper ventilation of the culture. The perfusion system (that can be gravity-driven, external syringes or on-chip peristaltic pumps, all of them having been proved to allow more than 1 week perfusion) has to be carefully configured to ensure both adequate nutrient flow at the flow rate required for the shear stress pattern to be studied and bubble-free fluid flow (Kim et al., 2007). Fluid control can become specially challenging in self-organized microvessels due to their random nature. Lastly, it has to be mentioned that often microfluidic control systems are rather complex to use and researchers may not be familiar with its handling (Halldorsson et al., 2015).
All in all, we believe that the microfluidic technology needs still to be exploited to reach its full potential in the study of vascular remodeling events because of the many advantages it offers in comparison to other in vitro systems and that standardization of this type of experiments will overcome many of the difficulties it may pose nowadays.
Conclusions and Perspectives
Tissues continuously adjust their oxygen and nutrient demands and for that they require the dynamic adaption of the microvasculature in order to optimize blood flow distribution. This is achieved by means of capillary remodeling, eliminating poorly functional or redundant segments by pruning, or duplicating segments to expand the microvasculature by splitting. Defective or excessive capillary remodeling will deprive tissue regions of appropriate perfusion leading to tissue damage or limiting its repair. This process has therefore attracted a lot of recent interest not only to understand vascular homeostasis but also to propose new strategies devoted to prevent disease and promote tissue repair (Georgieva et al., 2019).
Active research on the basic mechanisms of capillary remodeling beyond blood flow-driven endothelial cell migration can help identifying novel actors and modulators with potential use in preventing or ameliorating diseases related to excessive or defective capillary splitting or pruning as for example inflammatory bowel diseases, lung dysplasia, myocardial infarction or Alzheimer’s disease among others. As an example, the small GTPase Rac1 has been involved in capillary pruning in the brain zebrafish microvasculature and it is tempting to speculate that Rac1 inhibitors may help prevent excessive brain capillary pruning associated for example with aging or Alzheimer’s disease (Garkavtsev et al., 2011; Chen et al., 2012). On the other hand, excessive capillary splitting has been shown to contribute to the progression of inflammatory bowel disease and targeting recently identified actors as the molecular axis MT1-MMP/TSP1/eNOS by delivery of inhibitory antibodies or competitive peptides was shown to ameliorate the progression of the disease in mice (Esteban et al., 2020). Since failed microvascular remodeling can also lead to the presence of undesired arteriovenous shunts, recent identification of endoglin as a key regulator of endothelial cell responses for preventing AVM, can shed light about mechanistic links between endothelial cell responses to blood flow and disease. Furthermore, provocatively considering capillary pruning and splitting as two coordinated processes which continuously translate blood flow/shear stress fluctuations into microvascular adaptations can also help advance our understanding of these vascular events.
Finally, research efforts should be encouraged for the development and implementation of approaches and tools which more closely recapitulate the dynamics, geometries and flow conditions and the biochemical microenvironment of in vivo capillary remodeling in order to guarantee a wider comprehension of this process in near future.
Author Contributions
RS and SE obtained microscopy images. RS and MG-Á prepared the figures. RS, MG-Á, and AGA wrote the text and all authors revised it. All authors contributed to the article and approved the submitted version.
Funding
The work in this manuscript has been funded by a grant from the Spanish Ministry of Science and Innovation (SAF2017-83229-R to AGA) and a fellowship from the FPI-Severo Ochoa program (to RS). The CNIC is supported by the Instituto de Salud Carlos III (ISCIII), the Ministerio de Ciencia e Innovación (MCIN) and the Pro CNIC Foundation, and was a Severo Ochoa Center of Excellence (SEV-2015-0505). We acknowledge support of the publication fee by the CSIC Open Access Publication Support Initiative through its Unit of Information Resources for Research (URICI).
Conflict of Interest
The authors declare that the research was conducted in the absence of any commercial or financial relationships that could be construed as a potential conflict of interest.
References
Ackermann, M., Tsuda, A., Secomb, T. W., Mentzer, S. J., and Konerding, M. A. (2013). Intussusceptive remodeling of vascular branch angles in chemically-induced murine colitis. Microvasc. Res. 87, 75–82. doi: 10.1016/j.mvr.2013.02.002
Akbari, E., Spychalski, G. B., Rangharajan, K. K., Prakash, S., and Song J. W. (2018). Flow dynamics control endothelial permeability in a microfluidic vessel bifurcation model. Lab. Chip. 18, 1084–1093. doi: 10.1039/C8LC00130H
Akbari, E., Spychalski, G. B., and Song, J. W. (2017). Microfluidic approaches to the study of angiogenesis and the microcirculation. Microcirculation 24:e12363. doi: 10.1111/micc.12363
Andres, A. C., and Djonov, V. (2010) The mammary gland vasculature revisited. J. Mammary Gland Biol. Neoplasia 15, 319–328. doi: 10.1007/s10911-010-9186-9
Baeyens, N., Bandyopadhyay, C., Coon, B. G., Yun, S., and Schwartz, M. A. (2016). Endothelial fluid shear stress sensing in vascular health and disease. J. Clin. Invest. 126, 821–828. doi: 10.1172/JCI83083
Baeyens, N., Nicoli, S., Coon, B. G., Ross, T. D., Van den Dries, K., Han, J., et al. (2015). Vascular remodeling is governed by a VEGFR3-dependent fluid shear stress set point. eLife 4:e04645. doi: 10.7554/eLife.04645
Baffert, F., Le, T., Sennino, B., Thurston, G., Kuo, C. J., Hu-Lowe, D., et al. (2006). Cellular changes in normal blood capillaries undergoing regression after inhibition of VEGF signaling. Am. J. Physiol. Heart Circ. Physiol. 290, H547–H559. doi: 10.1152/ajpheart.00616.2005
Barbacena, P., Carvalho, J. R., and Franco, C. A. (2016). Endothelial cell dynamics in vascular remodelling. Clin. Hemorheol. Microcirc. 64, 557–563. doi: 10.3233/CH-168006
Barbacena, P., Ouarne, M., Haigh, J. J., Vasconcelos, F. F., Pezzarossa, A., and Franco, C. A. (2019). GNrep mouse: a reporter mouse for front-rear cell polarity. Genesis 57:e23299. doi: 10.1002/dvg.23299
Bartosch, A. M. W., Mathews, R., and Tarbell, J. M. (2017). Endothelial glycocalyx-mediated nitric oxide production in response to selective AFM pulling. Biophys. J. 113, 101–108. doi: 10.1016/j.bpj.2017.05.033
Baum, O., Da Silva-Azevedo, L., Willerding, G., Wockel, A., Planitzer, G., Gossrau, R., et al. (2004). Endothelial NOS is main mediator for shear stress-dependent angiogenesis in skeletal muscle after prazosin administration. Am. J. Physiol. Heart Circ. Physiol. 287, H2300–H2308. doi: 10.1152/ajpheart.00065.2004
Baum, O., Suter, F., Gerber, B., Tschanz, S. A., Buergy, R., Blank, F., et al. (2010). VEGF-A promotes intussusceptive angiogenesis in the developing chicken chorioallantoic membrane. Microcirculation 17, 447–457. doi: 10.1111/j.1549-8719.2010.00043.x
Bell, R. D., and Zlokovic, B. V. (2009). Neurovascular mechanisms and blood-brain barrier disorder in Alzheimer’s disease. Acta Neuropathol. 118, 103–113. doi: 10.1007/s00401-009-0522-3
Belle, J., Ysasi, A., Bennett, R. D., Filipovic, N., Nejad, M. I., Trumper, D. L., et al. (2014). Stretch-induced intussuceptive and sprouting angiogenesis in the chick chorioallantoic membrane. Microvasc. Res. 95, 60–67. doi: 10.1016/j.mvr.2014.06.009
Benn, A., Alonso, F., Mangelschots, J., Genot, E., Lox, M., and Zwijsen, A. (2020). BMP-SMAD1/5 signaling regulates retinal vascular development. Biomolecules 10:488. doi: 10.3390/biom10030488
Bentley, K., Franco, C. A., Philippides, A., Blanco, R., Dierkes, M., Gebala, V., et al. (2014a). The role of differential VE-cadherin dynamics in cell rearrangement during angiogenesis. Nat. Cell Biol. 16, 309–321. doi: 10.1038/ncb2926
Bentley, K., Philippides, A., and Ravasz Regan, E. (2014b). Do endothelial cells dream of eclectic shape? Dev. Cell. 29, 146–158. doi: 10.1016/j.devcel.2014.03.019
Bernabeu, M. O., Jones, M. L., Nash, R. W., Pezzarossa, A., Coveney, P. V., Gerhardt, H., et al. (2018). PolNet: a tool to quantify network-level cell polarity and blood flow in vascular remodeling. Biophys. J. 114, 2052–2058. doi: 10.1016/j.bpj.2018.03.032
Bernabeu, M. O., Jones, M. L., Nielsen, J. H., Kruger, T., Nash, R. W., Groen, D., et al. (2014). Computer simulations reveal complex distribution of haemodynamic forces in a mouse retina model of angiogenesis. J. R. Soc. Interface 11:20140543. doi: 10.1098/rsif.2014.0543
Bhagyalakshmi, A., Berthiaume, F., Reich, K. M., and Frangos, J. A. (1992). Fluid shear stress stimulates membrane phospholipid metabolism in cultured human endothelial cells. J. Vasc. Res. 29, 443–449. doi: 10.1159/000158963
Blackman, B. R., Garcia-Cardena, G., and Gimbrone, M. A. Jr. (2002). A new in vitro model to evaluate differential responses of endothelial cells to simulated arterial shear stress waveforms. J. Biomech. Eng. 124, 397–407. doi: 10.1115/1.1486468
Bogorad, M. I., DeStefano, J., Karlsson, J., Wong, A. D., Gerecht, S., and Searson, P. C. (2015). Review: in vitro microvessel models. Lab. Chip. 15, 4242–4255. doi: 10.1039/C5LC00832H
Bongrazio, M., Da Silva-Azevedo, L., Bergmann, E. C., Baum, O., Hinz, B., Pries, A. R., et al. (2006). Shear stress modulates the expression of thrombospondin-1 and CD36 in endothelial cells in vitro and during shear stress-induced angiogenesis in vivo. Int. J. Immunopathol. Pharmacol. 19, 35–48. doi: 10.1177/205873920601900104
Boriushkin, E., Fancher, I. S., and Levitan, I. (2019). Shear-stress sensitive inwardly-rectifying K(+) channels regulate developmental retinal angiogenesis by vessel regression. Cell Physiol. Biochem. 52, 1569–1583. doi: 10.33594/000000109
Burri, P. H., Hlushchuk, R., and Djonov, V. (2004). Intussusceptive angiogenesis: its emergence, its characteristics, and its significance. Dev. Dyn. 231, 474–488. doi: 10.1002/dvdy.20184
Busch, R., Strohbach, A., Pennewitz, M., Lorenz, F., Bahls, M., Busch, M. C., et al. (2015). Regulation of the endothelial apelin/APJ system by hemodynamic fluid flow. Cell Signal. 27, 1286–1296. doi: 10.1016/j.cellsig.2015.03.011
Butler, P. J., Tsou, T. C., Li, J. Y., Usami, S., and Chien, S. (2002). Rate sensitivity of shear-induced changes in the lateral diffusion of endothelial cell membrane lipids: a role for membrane perturbation in shear-induced MAPK activation. FASEB J. 16, 216–218. doi: 10.1096/fj.01-0434fje
Campinho, P., Vilfan, A., and Vermot, J. (2020). Blood flow forces in shaping the vascular system: a focus on endothelial cell behavior. Front. Physiol. 11:552. doi: 10.3389/fphys.2020.00552
Chaki, S. P., and Rivera, G. M. (2013). Integration of signaling and cytoskeletal remodeling by Nck in directional cell migration. Bioarchitecture 3, 57–63. doi: 10.4161/bioa.25744
Chandana, E. P., Maeda, Y., Ueda, A., Kiyonari, H., Oshima, N., Yamamoto, M., et al. (2010). Involvement of the Reck tumor suppressor protein in maternal and embryonic vascular remodeling in mice. BMC Dev. Biol. 10:84. doi: 10.1186/1471-213X-10-84
Chang, A. H., Raftrey, B. C., D’Amato, G., Surya, V. N., Poduri, A., Chen, H. I., et al. (2017). DACH1 stimulates shear stress-guided endothelial cell migration and coronary artery growth through the CXCL12-CXCR4 signaling axis. Genes Dev. 31, 1308–1324. doi: 10.1101/gad.301549.117
Chen, Q., Jiang, L., Li, C., Hu, D., Bu, J. W., Cai, D., et al. (2012). Haemodynamics-driven developmental pruning of brain vasculature in zebrafish. PLoS Biol. 10:e1001374. doi: 10.1371/journal.pbio.1001374
Chien, S. (2003). Molecular and mechanical bases of focal lipid accumulation in arterial wall. Prog. Biophys. Mol. Biol. 83, 131–151. doi: 10.1016/S0079-6107(03)00053-1
Chien, S. (2007). Mechanotransduction and endothelial cell homeostasis: the wisdom of the cell. Am. J. Physiol. Heart Circ. Physiol. 292, H1209–H1224. doi: 10.1152/ajpheart.01047.2006
Chien, S. (2008). Effects of disturbed flow on endothelial cells. Ann. Biomed. Eng. 36, 554–562. doi: 10.1007/s10439-007-9426-3
Chiu, J. J., and Chien, S. (2011). Effects of disturbed flow on vascular endothelium: pathophysiological basis and clinical perspectives. Physiol. Rev. 91, 327–387. doi: 10.1152/physrev.00047.2009
Choi, W., Kim, H. M., Park, S., Yeom, E., Doh, J., and Lee SJ. (2017). Variation in wall shear stress in channel networks of zebrafish models. J. R. Soc. Interface 14:20160900. doi: 10.1098/rsif.2016.0900
Chu, T. J., and Peters, D. G. (2008). Serial analysis of the vascular endothelial transcriptome under static and shear stress conditions. Physiol. Genomics 34, 185–192. doi: 10.1152/physiolgenomics.90201.2008
Colangelo, S., Langille, B. L., and Gotlieb AI. (1994). Three patterns of distribution characterize the organization of endothelial microfilaments at aortic flow dividers. Cell Tissue Res. 278, 235–242. doi: 10.1007/BF00414165
Coluccio, M. L., D’Attimo, M. A., Cristiani, C. M., Candeloro, P., Parrotta, E., Dattola, E., et al. (2019). A passive microfluidic device for chemotaxis studies. Micromachines 10:551 doi: 10.3390/mi10080551
Connolly, S. E., Hores, T. A., Smith, L. E., and D’Amore, P. A. (1988). Characterization of vascular development in the mouse retina. Microvasc. Res. 36, 275–290. doi: 10.1016/0026-2862(88)90028-3
Coughlin, M. F., and Kamm, R. D. (2020). The use of microfluidic platforms to probe the mechanism of cancer cell extravasation. Adv. Health Mater. 9:e1901410. doi: 10.1002/adhm.201901410
Davies, P. F. (1995). Flow-mediated endothelial mechanotransduction. Physiol. Rev. 75, 519–560. doi: 10.1152/physrev.1995.75.3.519
De Paepe, M. E., Benny, M. K. V., Priolo, L., Luks, F. I., and Shapiro, S. (2017). Florid intussusceptive-like microvascular dysangiogenesis in a preterm lung. Pediatr. Dev. Pathol. 20, 432–439. doi: 10.1177/1093526616686455
De Paepe, M. E., Chu, S., Hall, S. J., McDonnell-Clark, E., Heger, N. E, Schorl, C., et al. (2015). Intussusceptive-like angiogenesis in human fetal lung xenografts: link with bronchopulmonary dysplasia-associated microvascular dysangiogenesis? Exp. Lung Res. 41, 477–488. doi: 10.3109/01902148.2015.1080321
dela Paz, N. G., Walshe, T. E., Leach, L. L., Saint-Geniez, M., and D’Amore, P. A. (2012). Role of shear-stress-induced VEGF expression in endothelial cell survival. J. Cell Sci. 125(Pt 4), 831–843. doi: 10.1242/jcs.084301
Dieterich, P., Odenthal-Schnittler, M., Mrowietz, C., Kramer, M., Sasse, L., Oberleithner, H., et al. (2000). Quantitative morphodynamics of endothelial cells within confluent cultures in response to fluid shear stress. Biophys. J. 79, 1285–1297. doi: 10.1016/S0006-3495(00)76382-X
Dimova, I., Hlushchuk, R., Makanya, A., Styp-Rekowska, B., Ceausu, A., Flueckiger, S., et al. (2013). Inhibition of Notch signaling induces extensive intussusceptive neo-angiogenesis by recruitment of mononuclear cells. Angiogenesis 16, 921–937. doi: 10.1007/s10456-013-9366-5
Djonov, V. G., Kurz, H., and Burri, P. H. (2002). Optimality in the developing vascular system: branching remodeling by means of intussusception as an efficient adaptation mechanism. Dev. Dyn. 224, 391–402. doi: 10.1002/dvdy.10119
Du, W., and Gao, C. (2019). Selective adhesion and directional migration of endothelial cells guided by Cys-Ala-Gly peptide density gradient on antifouling polymer brushes. Macromol. Biosci. 19:e1900292. doi: 10.1002/mabi.201900292
Dubrac, A., Genet, G., Ola, R., Zhang, F., Pibouin-Fragner, L., and Han J, et al. (2016). Targeting NCK-mediated endothelial cell front-rear polarity inhibits neovascularization. Circulation 133, 409–421. doi: 10.1161/CIRCULATIONAHA.115.017537
Eckmann, D. M., Bowers, S., Stecker, M., and Cheung, A. T. (2000). Hematocrit, volume expander, temperature, and shear rate effects on blood viscosity. Anesth. Analg. 91, 539–545. doi: 10.1213/00000539-200009000-00007
Edgar, L. T., Franco, C. A., Gerhardt, H., and Bernabeu, M. O. (2020). On the preservation of vessel bifurcations during flow-mediated angiogenic remodelling. bioRxiv [Preprint] doi: 10.1101/2020.02.07.938522
Egginton, S. (2011). In vivo shear stress response. Biochem. Soc. Trans. 39, 1633–1638. doi: 10.1042/BST20110715
Esteban, S., Clemente, C., Koziol, A., Gonzalo, P., Rius, C., Martinez, F., et al. (2020). Endothelial MT1-MMP targeting limits intussusceptive angiogenesis and colitis via TSP1/nitric oxide axis. Embo Mol Med. 12:e10862. doi: 10.15252/emmm.201910862
Evans, I. M., Kennedy, S. A., Paliashvili, K., Santra, T., Yamaji, M., Lovering, R. C., et al. (2017). Vascular endothelial growth factor (VEGF) promotes assembly of the p130Cas interactome to drive endothelial chemotactic signaling and angiogenesis. Mol. Cell. Proteomics 16, 168–180. doi: 10.1074/mcp.M116.064428
Fantin, A., Lampropoulou, A., Gestri, G., Raimondi, C., Senatore, V., Zachary, I., et al. (2015). NRP1 regulates CDC42 activation to promote filopodia formation in endothelial tip cells. Cell Rep. 11, 1577–1590. doi: 10.1016/j.celrep.2015.05.018
Feinberg, R. N., Latker, C. H., and Beebe, D. C. (1986). Localized vascular regression during limb morphogenesis in the chicken embryo. I. Spatial and temporal changes in the vascular pattern. Anat. Rec. 214, 405–409. doi: 10.1002/ar.1092140411
Filipovic, N., Tsuda, A., Lee, G. S., Miele, L. F., Lin, M., Konerding, M. A., et al. (2009). Computational flow dynamics in a geometric model of intussusceptive angiogenesis. Microvasc. Res. 78, 286–293. doi: 10.1016/j.mvr.2009.08.004
Franco, C. A., and Gerhardt, H. (2017). Morph or move? How distinct endothelial cell responses to blood flow shape vascular networks. Dev. Cell. 41, 574–576. doi: 10.1016/j.devcel.2017.06.008
Franco, C. A., Jones, M. L., Bernabeu, M. O., Geudens, I., Mathivet, T., Rosa, A., et al. (2015). Dynamic endothelial cell rearrangements drive developmental vessel regression. PLoS Biol. 13:e1002125. doi: 10.1371/journal.pbio.1002125
Franco, C. A., Jones, M. L., Bernabeu, M. O., Vion, A. C., Barbacena, P., Fan, J., et al. (2016). Non-canonical Wnt signalling modulates the endothelial shear stress flow sensor in vascular remodelling. eLife 5:e07727. doi: 10.7554/eLife.07727
Galbraith, C. G., Skalak, R., and Chien, S. (1998). Shear stress induces spatial reorganization of the endothelial cell cytoskeleton. Cell Motil. Cytoskeleton 40, 317–330. doi: 10.1002/(SICI)1097-0169(1998)40:4<317::AID-CM1>3.0.CO;2-8
Gan, L., Miocic, M., Doroudi, R., Selin-Sjogren, L., and Jern, S. (2000). Distinct regulation of vascular endothelial growth factor in intact human conduit vessels exposed to laminar fluid shear stress and pressure. Biochem. Biophys. Res. Commun. 272, 490–496. doi: 10.1006/bbrc.2000.2663
Ganesan, P., He, S., and Xu, H. (2010). Development of an image-based network model of retinal vasculature. Ann. Biomed. Eng. 38, 1566–1585. doi: 10.1007/s10439-010-9942-4
Garkavtsev, I., Chauhan, V. P., Wong, H. K., Mukhopadhyay, A., Glicksman, M. A., Peterson, R. T., et al. (2011). Dehydro-alpha-lapachone, a plant product with antivascular activity. Proc. Natl. Acad. Sci. U.S.A. 108, 11596–11601. doi: 10.1073/pnas.1104225108
Georgieva, P. B., Marchuk, D. A., Gerhardt, H., and Leducq, A. C. (2019). Attract. Circ. Res. 125, 262–264. doi: 10.1161/CIRCRESAHA.119.315198
Giacomini, A., Ackermann, M., Belleri, M., Coltrini, D., Nico, B., Ribatti, D., et al. (2015). Brain angioarchitecture and intussusceptive microvascular growth in a murine model of Krabbe disease. Angiogenesis 18, 499–510. doi: 10.1007/s10456-015-9481-6
Gianni-Barrera, R., Butschkau, A., Uccelli, A., Certelli, A., Valente, P., Bartolomeo, M., et al. (2018). PDGF-BB regulates splitting angiogenesis in skeletal muscle by limiting VEGF-induced endothelial proliferation. Angiogenesis 21, 883–900. doi: 10.1007/s10456-018-9634-5
Gianni-Barrera, R., Trani, M., Fontanellaz, C., Heberer, M., Djonov, V., Hlushchuk, R., et al. (2013). VEGF over-expression in skeletal muscle induces angiogenesis by intussusception rather than sprouting. Angiogenesis 16, 123–136. doi: 10.1007/s10456-012-9304-y
Gkontra, P., El-Bouri, W. K., Norton, K. A., Santos, A., Popel, A. S., Payne, S. J., et al. (2019). Dynamic changes in microvascular flow conductivity and perfusion after myocardial infarction shown by image-based modeling. J. Am. Heart Assoc. 8:e011058. doi: 10.1161/JAHA.118.011058
Gkontra, P., Norton, K. A., Zak, M. M., Clemente, C., Agüero, J., Ibáñez, B., et al. (2018). Deciphering microvascular changes after myocardial infarction through 3D fully automated image analysis. Sci. Rep. 8:1854. doi: 10.1038/s41598-018-32598-6
Goede, V., Schmidt, T., Kimmina, S., Kozian, D., and Augustin, H. G. (1998). Analysis of blood vessel maturation processes during cyclic ovarian angiogenesis. Lab. Invest. 78, 1385–1394.
Gomes, N., Legrand, C., and Fauvel-Lafeve, F. (2005). Shear stress induced release of von Willebrand factor and thrombospondin-1 in HUVEC extracellular matrix enhances breast tumour cell adhesion. Clin. Exp Metast. 22, 215–223. doi: 10.1007/s10585-005-7359-5
Gordon, E., Schimmel, L., and Frye, M. (2020). The importance of mechanical forces for in vitro endothelial cell biology. Front. Physiol. 11:684. doi: 10.3389/fphys.2020.00684
Groppa, E., Brkic, S., Uccelli, A., Wirth, G., Korpisalo-Pirinen, P., Filippova, M., et al. (2018). EphrinB2/EphB4 signaling regulates non-sprouting angiogenesis by VEGF. Embo Rep. 19:e45054. doi: 10.15252/embr.201745054
Hahn, P., Lindsten, T., Tolentino, M., Thompson, C. B., Bennett, J., and Dunaief, J. L. (2005). Persistent fetal ocular vasculature in mice deficient in bax and bak. Arch. Ophthalmol. 123, 797–802. doi: 10.1001/archopht.123.6.797
Han, S., Shin, Y., Jeong, H. E., Jeon, J. S., Kamm, R. D., Huh, D., et al. (2015). Constructive remodeling of a synthetic endothelial extracellular matrix. Sci. Rep. 5:18290. doi: 10.1038/srep18290
Halldorsson, S., Lucumi, E., Gomez-Sjoberg, R., and Fleming, R. M. T. (2015). Advantages and challenges of microfluidic cell culture in polydimethylsiloxane devices. Biosens. Bioelectron. 63, 218–231. doi: 10.1016/j.bios.2014.07.029
Hamm, M. J., Kirchmaier, B. C., and Herzog, W. (2016). Sema3d controls collective endothelial cell migration by distinct mechanisms via Nrp1 and PlxnD1. J. Cell Biol. 215, 415–430. doi: 10.1083/jcb.201603100
Hesh, C. A., Qiu, Y., and Lam, W. A. (2019). Vascularized microfluidics and the blood-endothelium interface. Micromachines 11:18. doi: 10.3390/mi11010018
Hlushchuk, R., Styp-Rekowska, B., Dzambazi, J., Wnuk, M., Huynh-Do, U., Makanya, A., et al. (2017). Endoglin inhibition leads to intussusceptive angiogenesis via activation of factors related to COUP-TFII signaling pathway. PLoS One 12:e0182813. doi: 10.1371/journal.pone.0182813
Holash, J., Maisonpierre, P. C., Compton, D., Boland, P., Alexander, C. R., Zagzag, D., et al. (1999). Vessel cooption, regression, and growth in tumors mediated by angiopoietins and VEGF. Science 284, 1994–1998. doi: 10.1126/science.284.5422.1994
Hsu, Y. H., Moya, M. L., Hughes, C. C., George, S. C., and Lee, A. P. (2013). A microfluidic platform for generating large-scale nearly identical human microphysiological vascularized tissue arrays. Lab. Chip. 13, 2990–2998. doi: 10.1039/c3lc50424g
Hudlicka, O., Brown, M. D., May, S., Zakrzewicz, A., and Pries, A. R. (2006). Changes in capillary shear stress in skeletal muscles exposed to long-term activity: role of nitric oxide. Microcirculation 13, 249–259. doi: 10.1080/10739680600556951
Huh, D., Hamilton, G. A., and Ingber, D. E. (2011). From 3D cell culture to organs-on-chips. Trends Cell Biol. 21, 745–754. doi: 10.1016/j.tcb.2011.09.005
Ito, M., and Yoshioka, M. (1999). Regression of the hyaloid vessels and pupillary membrane of the mouse. Anat. Embryol. 200, 403–411. doi: 10.1007/s004290050289
Jacobs, E. R., Cheliakine, C., Gebremedhin, D., Birks, E. K., Davies, P. F., and Harder, D. R. (1995). Shear activated channels in cell-attached patches of cultured bovine aortic endothelial cells. Pflugers Arch. 431, 129–131. doi: 10.1007/BF00374386
Jin, Y., Muhl, L., Burmakin, M., Wang, Y., Duchez, A. C., Betsholtz, C., et al. (2017). Endoglin prevents vascular malformation by regulating flow-induced cell migration and specification through VEGFR2 signalling. Nat. Cell Biol. 19, 639–652. doi: 10.1038/ncb3534
Kang, H., Kwak, H. I., Kaunas, R., and Bayless, K. J. (2011). Fluid shear stress and sphingosine 1-phosphate activate calpain to promote membrane type 1 matrix metalloproteinase (MT1-MMP) membrane translocation and endothelial invasion into three-dimensional collagen matrices. J. Biol. Chem. 286, 42017–42026. doi: 10.1074/jbc.M111.290841
Karino, T., and Goldsmith, H. L. (1980). Disturbed flow in models of branching vessels. Trans. Am. Soc. Artif. Intern. Organs. 26, 500–506.
Karino, T., Goldsmith, H. L., Motomiya, M., Mabuchi, S., and Sohara, Y. (1987). Flow patterns in vessels of simple and complex geometries. Ann. N. Y. Acad. Sci. 516, 422–441. doi: 10.1111/j.1749-6632.1987.tb33063.x
Karthik, S., Djukic, T., Kim, J. D., Zuber, B., Makanya, A., Odriozola, A., et al. (2018). Synergistic interaction of sprouting and intussusceptive angiogenesis during zebrafish caudal vein plexus development. Sci. Rep. 8:9840. doi: 10.1038/s41598-018-27791-6
Kim, L., Toh, Y. C., Voldman, J., and Yu, H. (2007). A practical guide to microfluidic perfusion culture of adherent mammalian cells. Lab. Chip. 7, 681–694. doi: 10.1039/b704602b
Kim, H., Chung, H., Kim, J., Choi, D. H., Shin, Y., Kang, Y. G., et al. (2019). Macrophages-triggered sequential remodeling of endothelium-interstitial matrix to form pre-metastatic niche in microfluidic tumor microenvironment. Adv. Sci. 6:1900195. doi: 10.1002/advs.201900195
Kochhan, E., Lenard, A., Ellertsdottir, E., Herwig, L., Affolter, M., Belting, H. G., et al. (2013). Blood flow changes coincide with cellular rearrangements during blood vessel pruning in zebrafish embryos. PLoS One 8:e75060. doi: 10.1371/journal.pone.0075060
Korn, C., and Augustin, H. G. (2015). Mechanisms of vessel pruning and regression. Dev. Cell. 34, 5–17. doi: 10.1016/j.devcel.2015.06.004
Kwon, H. B., Wang, S., Helker, C. S., Rasouli, S. J., Maischein, H. M., and Offermanns, S. (2016). In vivo modulation of endothelial polarization by Apelin receptor signalling. Nat Commun. 7:11805. doi: 10.1038/ncomms11805
Lang, R., Lustig, M., Francois, F., Sellinger, M., and Plesken, H. (1994). Apoptosis during macrophage-dependent ocular tissue remodelling. Development 120, 3395–3403.
Larrivee, B., Prahst, C., Gordon, E., del Toro, R., Mathivet, T., Duarte, A., et al. (2012). ALK1 signaling inhibits angiogenesis by cooperating with the Notch pathway. Dev Cell. 22, 489–500. doi: 10.1016/j.devcel.2012.02.005
Lavina, B., Castro, M., Niaudet, C., Cruys, B., Alvarez-Aznar, A., Carmeliet, P., et al. (2018). Defective endothelial cell migration in the absence of Cdc42 leads to capillary-venous malformations. Development 145: dev161182. doi: 10.1242/dev.161182
Lawson, N. D., and Weinstein, B. M. (2002). In vivo imaging of embryonic vascular development using transgenic zebrafish. Dev Biol. 248, 307–318. doi: 10.1006/dbio.2002.0711
le Noble, F., Moyon, D., Pardanaud, L., Yuan, L., Djonov, V., Matthijsen, R., et al. (2004). Flow regulates arterial-venous differentiation in the chick embryo yolk sac. Development 131, 361–375. doi: 10.1242/dev.00929
Lee, G. S., Filipovic, N., Lin, M., Gibney, B. C., Simpson, D. C., Konerding, M. A., et al. (2011). Intravascular pillars and pruning in the extraembryonic vessels of chick embryos. Dev. Dyn. 240, 1335–1343. doi: 10.1002/dvdy.22618
Lee, G. S., Filipovic, N., Miele, L. F., Lin, M., Simpson, D. C., Giney, B., et al. (2010). Blood flow shapes intravascular pillar geometry in the chick chorioallantoic membrane. J. Angiogenes Res. 2:11. doi: 10.1186/2040-2384-2-11
Levesque, M. J., and Nerem, R. M. (1985). The elongation and orientation of cultured endothelial cells in response to shear stress. J. Biomech. Eng. 107, 341–347. doi: 10.1115/1.3138567
Li, J., Hou, B., Tumova, S., Muraki, K., Bruns, A., Ludlow, M. J., et al. (2014). Piezo1 integration of vascular architecture with physiological force. Nature 515, 279–282. doi: 10.1038/nature13701
Lobov, I. B., Cheung, E., Wudali, R., Cao, J., Halasz, G., Wei, Y., et al. (2011). The Dll4/Notch pathway controls postangiogenic blood vessel remodeling and regression by modulating vasoconstriction and blood flow. Blood 117, 6728–6737. doi: 10.1182/blood-2010-08-302067
Lobov, I. B., Rao, S., Carroll, T. J., Vallance, J. E., Ito, M., Ondr, J. K., et al. (2005). WNT7b mediates macrophage-induced programmed cell death in patterning of the vasculature. Nature 437, 417–421. doi: 10.1038/nature03928
Lu, D., and Kassab, G. S. (2011). Role of shear stress and stretch in vascular mechanobiology. J. R. Soc. Interface 8, 1379–1385. doi: 10.1098/rsif.2011.0177
Lucitti, J. L., Jones, E. A., Huang, C., Chen, J., Fraser, S. E., and Dickinson, M. E. (2007). Vascular remodeling of the mouse yolk sac requires hemodynamic force. Development 134, 3317–3326. doi: 10.1242/dev.02883
Lundberg, E., and Borner, G. H. H. (2019). Spatial proteomics: a powerful discovery tool for cell biology. Nat. Rev. Mol. Cell Biol. 20, 285–302. doi: 10.1038/s41580-018-0094-y
Luttun, A., Tjwa, M., Moons, L., Wu, Y., Angelillo-Scherrer, A., and Liao, F., (2002). Revascularization of ischemic tissues by PlGF treatment, and inhibition of tumor angiogenesis, arthritis and atherosclerosis by anti-Flt1. Nat Med. 8, 831–840. doi: 10.1038/nm731
Mack, J. J., Mosqueiro, T. S., Archer, B. J., Jones, W. M., Sunshine, H., Faas, G. C., et al. (2017). NOTCH1 is a mechanosensor in adult arteries. Nat. Commun. 8:1620. doi: 10.1038/s41467-017-01741-8
Magid, R., Martinson, D., Hwang, J., Jo, H., and Galis, Z. S. (2003). Optimization of isolation and functional characterization of primary murine aortic endothelial cells. Endothelium 10, 103–109. doi: 10.1080/10623320303364
Maibier, M., Reglin, B., Nitzsche, B., Xiang, W., Rong, W. W., Hoffmann, B., et al. (2016). Structure and hemodynamics of vascular networks in the chorioallantoic membrane of the chicken. Am. J. Physiol. Heart Circ. Physiol. 311, H913–H926. doi: 10.1152/ajpheart.00786.2015
Meeson, A. P., Argilla, M., Ko, K., Witte, L., and Lang, R. A. (1999). VEGF deprivation-induced apoptosis is a component of programmed capillary regression. Development 126, 1407–1415.
Mehta, V., Pang, K. L., Rozbesky, D., Nather, K., Keen, A., Lachowski, D., et al. (2020). The guidance receptor plexin D1 is a mechanosensor in endothelial cells. Nature 578, 290–295. doi: 10.1038/s41586-020-1979-4
Mentzer, S. J., and Konerding, M. A. (2014). Intussusceptive angiogenesis: expansion and remodeling of microvascular networks. Angiogenesis 17, 499–509. doi: 10.1007/s10456-014-9428-3
Miao, H., Hu, Y. L., Shiu, Y. T., Yuan, S., Zhao, Y., Kaunas, R., et al. (2005). Effects of flow patterns on the localization and expression of VE-cadherin at vascular endothelial cell junctions: in vivo and in vitro investigations. J. Vasc. Res. 42, 77–89. doi: 10.1159/000083094
Mori, M., Stokes, K. Y., Vowinkel, T., Watanabe, N., Elrod, J. W., Harris, N. R., et al. (2005). Colonic blood flow responses in experimental colitis: time course and underlying mechanisms. Am. J. Physiol. Gastrointest. Liver Physiol. 289, G1024–G1029. doi: 10.1152/ajpgi.00247.2005
Nagasawa-Masuda, A., and Terai, K. (2017). Yap/Taz transcriptional activity is essential for vascular regression via Ctgf expression and actin polymerization. PLoS One 12:e0174633. doi: 10.1371/journal.pone.0174633
Nakajima, H., Yamamoto, K., Agarwala, S., Terai, K., Fukui, H., Fukuhara, S., et al. (2017). Flow-dependent endothelial YAP regulation contributes to vessel maintenance. Dev Cell. 40, 523.e6–536.e6. doi: 10.1016/j.devcel.2017.02.019
Nayak, L., Lin, Z., and Jain, M. K. (2011). “Go with the flow”: how Kruppel-like factor 2 regulates the vasoprotective effects of shear stress. Antioxid. Redox Signal. 15, 1449–1461. doi: 10.1089/ars.2010.3647
Nerem, R. M. (1993). Hemodynamics and the vascular endothelium. J. Biomech. Eng. 115, 510–514. doi: 10.1115/1.2895532
Neto, F., Klaus-Bergmann, A., Ong, Y. T., Alt, S., Vion, A. C., Szymborska, A., et al. (2018). YAP and TAZ regulate adherens junction dynamics and endothelial cell distribution during vascular development. eLife 7:e31037. doi: 10.7554/eLife.31037
Nguyen, D. H., Stapleton, S. C., Yang, M. T., Cha, S. S., Choi, C. K., Galie, P. A., et al. (2013). Biomimetic model to reconstitute angiogenic sprouting morphogenesis in vitro. Proc. Natl. Acad. Sci. U.S.A. 110, 6712–6717. doi: 10.1073/pnas.1221526110
Nithianandarajah-Jones, G. N., Wilm, B., Goldring, C. E., Muller, J., and Cross, M. J. (2014). The role of ERK5 in endothelial cell function. Biochem. Soc. Trans. 42, 1584–1589. doi: 10.1042/BST20140276
Nollert, M. U., Eskin, S. G., and McIntire, L. V. (1990). Shear stress increases inositol trisphosphate levels in human endothelial cells. Biochem. Biophys. Res. Commun. 170, 281–287. doi: 10.1016/0006-291X(90)91271-S
Noria, S., Xu, F., McCue, S., Jones, M., Gotlieb, A. I., and Langille, B. L. (2004). Assembly and reorientation of stress fibers drives morphological changes to endothelial cells exposed to shear stress. Am. J. Pathol. 164, 1211–1223. doi: 10.1016/S0002-9440(10)63209-9
Noris, M., Morigi, M., Donadelli, R., Aiello, S., Foppolo, M., Todeschini, M., et al. (1995). Nitric oxide synthesis by cultured endothelial cells is modulated by flow conditions. Circ. Res. 76, 536–543. doi: 10.1161/01.RES.76.4.536
Nowak-Sliwinska, P., Alitalo, K., Allen, E., Anisimov, A., Aplin, A. C., Auerbach, R., et al. (2018). Consensus guidelines for the use and interpretation of angiogenesis assays. Angiogenesis 21, 425–532 doi: 10.1007/s10456-018-9613-x
Ohta, S., Inasawa, S., and Yamaguchi, Y. (2015). Alignment of vascular endothelial cells as a collective response to shear flow. J. Phys. D Appl. Phys. 48, 245–401. doi: 10.1088/0022-3727/48/24/245401
Okuda, M., Takahashi, M., Suero, J., Murry, C. E., Traub, O., Kawakatsu, H., et al. (1999). Shear stress stimulation of p130(cas) tyrosine phosphorylation requires calcium-dependent c-Src activation. J. Biol. Chem. 274, 26803–26809. doi: 10.1074/jbc.274.38.26803
Ostrowski, M. A., Huang, N. F., Walker, T. W., Verwijlen, T., Poplawski, C., Khoo, A. S., et al. (2014). Microvascular endothelial cells migrate upstream and align against the shear stress field created by impinging flow. Biophys. J. 106, 366–374. doi: 10.1016/j.bpj.2013.11.4502
Paku, S., Dezso, K., Bugyik, E., Tovari, J., Timar, J., and Nagy, P, et al. (2011). A new mechanism for pillar formation during tumor-induced intussusceptive angiogenesis: inverse sprouting. Am. J. Pathol. 179, 1573–1585. doi: 10.1016/j.ajpath.2011.05.033
Papadaki, M., and McLntire, L. V. (1999). Quantitative measurement of shear-stress effects on endothelial cells. Methods Mol. Med. 18577–593. doi: 10.1385/0-89603-516-6:577
Patan, S., Alvarez, M. J., Schittny, J. C., and Burri, P. H. (1992). Intussusceptive microvascular growth: a common alternative to capillary sprouting. Arch. Histol. Cytol. 55, 65–75. doi: 10.1679/aohc.55.Suppl_65
Patan, S., Haenni, B., and Burri, P. H. (1993). Evidence for intussusceptive capillary growth in the chicken chorio-allantoic membrane (CAM). Anat. Embryol. 187, 121–130. doi: 10.1007/BF00171743
Patan, S., Haenni, B., and Burri, PH. (1996a). Implementation of intussusceptive microvascular growth in the chicken chorioallantoic membrane (CAM): 1. pillar formation by folding of the capillary wall. Microvasc. Res. 51, 80–98. doi: 10.1006/mvre.1996.0009
Patan, S., Munn, L. L., and Jain, R. K. (1996b). Intussusceptive microvascular growth in a human colon adenocarcinoma xenograft: a novel mechanism of tumor angiogenesis. Microvasc. Res. 51, 260–272. doi: 10.1006/mvre.1996.0025
Phng, L. K., Potente, M., Leslie, J. D., Babbage, J., Nyqvist, D., Lobov, I., et al. (2009). Nrarp coordinates endothelial Notch and Wnt signaling to control vessel density in angiogenesis. Dev. Cell. 16, 70–82. doi: 10.1016/j.devcel.2008.12.009
Poduri, A., Chang, A. H., Raftrey, B., Rhee, S., Van, M., and Red-Horse, K. (2017). Endothelial cells respond to the direction of mechanical stimuli through SMAD signaling to regulate coronary artery size. Development 144, 3241–3252. doi: 10.1242/dev.150904
Prahst, C., Ashrafzadeh, P., Mead, T., Figueiredo, A., Chang, K., Richardson, D., et al. (2020). Mouse retinal cell behaviour in space and time using light sheet fluorescence microscopy. eLife 9: e49779. doi: 10.7554/eLife.49779
Pries, A. R., and Secomb, T. W. (2014). Making microvascular networks work: angiogenesis, remodeling, and pruning. Physiology 29, 446–455. doi: 10.1152/physiol.00012.2014
Prior, B. M., Lloyd, P. G., Yang, H. T., and Terjung, R. L. (2003). Exercise-induced vascular remodeling. Exerc. Sport Sci. Rev. 31, 26–33. doi: 10.1097/00003677-200301000-00006
Ranade, S. S., Qiu, Z., Woo, S. H., Hur, S. S., Murthy, S. E., Cahalan, S. M., et al. (2014). Piezo1, a mechanically activated ion channel, is required for vascular development in mice. Proc. Natl. Acad. Sci. U.S.A. 111, 10347–10352. doi: 10.1073/pnas.1409233111
Rauff, A., LaBelle, S. A., Strobel, H. A., Hoying, J. B., and Weiss, J. A. (2019). Imaging the dynamic interaction between sprouting microvessels and the extracellular matrix. Front. Physiol. 10:1011. doi: 10.3389/fphys.2019.01011
Ravnic, D. J., Konerding, M. A., Tsuda, A., Huss, H. T., Wolloscheck, T., Pratt, J. P., et al. (2007). Structural adaptations in the murine colon microcirculation associated with hapten-induced inflammation. Gut 56, 518–523. doi: 10.1136/gut.2006.101824
Red-Horse, K., and Siekmann, A. F. (2019). Veins and arteries build hierarchical branching patterns differently: bottom-up versus top-down. Bioessays 41:e1800198. doi: 10.1002/bies.201800198
Ribatti, D., and Djonov, V. (2012). Intussusceptive microvascular growth in tumors. Cancer Lett. 316, 126–131. doi: 10.1016/j.canlet.2011.10.040
Ricard, N., and Simons, M. (2015). When it is better to regress: dynamics of vascular pruning. PLoS Biol. 13:e1002148. doi: 10.1371/journal.pbio.1002148
Riedl, J., Crevenna, A. H., Kessenbrock, K., Yu, J. H., Neukirchen, D., Bista, M., et al. (2008). Lifeact: a versatile marker to visualize F-actin. Nat. Methods 5, 605–607. doi: 10.1038/nmeth.1220
Rochon, E. R., Menon, P. G., and Roman, B. L. (2016). Alk1 controls arterial endothelial cell migration in lumenized vessels. Development 143, 2593–2602. doi: 10.1242/dev.135392
Roest, M., Reininger, A., Zwaginga, J. J., King, M. R., and Heemskerk, J. W. Biorheology Subcommittee of the SSCotI (2011). Flow chamber-based assays to measure thrombus formation in vitro: requirements for standardization. J. Thromb. Haemost. 9, 2322–2324. doi: 10.1111/j.1538-7836.2011.04492.x
Rutkowski, J. M., and Swartz, M. A. (2007). A driving force for change: interstitial flow as a morphoregulator. Trends Cell Biol. 17, 44–50. doi: 10.1016/j.tcb.2006.11.007
Sagare, A. P., Bell, R. D., and Zlokovic, B. V. (2012). Neurovascular dysfunction and faulty amyloid beta-peptide clearance in Alzheimer disease. Cold Spring Harb. Perspect. Med. 2:a011452 doi: 10.1101/cshperspect.a011452
Scheer, N., and Campos-Ortega, J. A. (1999). Use of the Gal4-UAS technique for targeted gene expression in the zebrafish. Mech. Dev. 80, 153–158. doi: 10.1016/S0925-4773(98)00209-3
Shalaby, S. Y., Chitragari, G., Sumpio, B. J., and Sumpio, B. E. (2017). Shear stress induces change in extracellular signal-regulated kinase 5 levels with sustained activation under disturbed and continuous laminar flow. Int. J. Angiol. 26, 109–115. doi: 10.1055/s-0037-1599057
Shao, S., Xiang, C., Qin, K., Ur Rehman Aziz, A., Liao, X., and Liu, B. (2017). Visualizing the spatiotemporal map of Rac activation in bovine aortic endothelial cells under laminar and disturbed flows. PLoS One 12:e0189088. doi: 10.1371/journal.pone.0189088
Shirure, V. S., Lezia, A., Tao, A., Alonzo, L. F., and George, S. C. (2017). Low levels of physiological interstitial flow eliminate morphogen gradients and guide angiogenesis. Angiogenesis 20, 493–504. doi: 10.1007/s10456-017-9559-4
Simonavicius, N., Ashenden, M., van Weverwijk, A., Lax, S., Huso, D. L., Buckley, C. D., et al. (2012). Pericytes promote selective vessel regression to regulate vascular patterning. Blood 120, 1516–1527. doi: 10.1182/blood-2011-01-332338
Song, J. W., and Munn, L. L. (2011). Fluid forces control endothelial sprouting. Proc. Natl. Acad. Sci. U.S.A. 108, 15342–15347. doi: 10.1073/pnas.1105316108
Sonmez, U. M., Cheng, Y.-W., Simon, C. W., Beth, L. R., and Lance, A. D. (2020). Endothelial cell polarization and orientation to flow in a novel microfluidic multimodal shear stress generator. bioRxiv [Preprint] doi: 10.1101/2020.07.10.197244
Spiering, D., Schmolke, M., Ohnesorge, N., Schmidt, M., Goebeler, M., Wegener, J., et al. (2009). MEK5/ERK5 signaling modulates endothelial cell migration and focal contact turnover. J. Biol. Chem. 284, 24972–24980. doi: 10.1074/jbc.M109.042911
Steward, R. Jr., Tambe, D., Hardin, C. C., Krishnan, R., and Fredberg, JJ. (2015). Fluid shear, intercellular stress, and endothelial cell alignment. Am. J. Physiol. Cell Physiol. 308, C657–C664. doi: 10.1152/ajpcell.00363.2014
Styp-Rekowska, B., Hlushchuk, R., Pries, A. R., and Djonov, V. (2011). Intussusceptive angiogenesis: pillars against the blood flow. Acta Physiol. 202, 213–223. doi: 10.1111/j.1748-1716.2011.02321.x
Sugden, W. W., Meissner, R., Aegerter-Wilmsen, T., Tsaryk, R., Leonard, E. V., Bussmann, J., et al. (2017). Endoglin controls blood vessel diameter through endothelial cell shape changes in response to haemodynamic cues. Nat. Cell Biol. 19, 653–665. doi: 10.1038/ncb3528
Szczerba, D., Kurz, H., and Szekely, G. (2009). A computational model of intussusceptive microvascular growth and remodeling. J. Theor. Biol. 261, 570–583. doi: 10.1016/j.jtbi.2009.09.018
Tang, K., Breen, E. C., Gerber, H. P., Ferrara, N. M., and Wagner, P. D. (2004). Capillary regression in vascular endothelial growth factor-deficient skeletal muscle. Physiol. Genomics 18, 63–69. doi: 10.1152/physiolgenomics.00023.2004
Tkachenko, E., Gutierrez, E., Saikin, S. K., Fogelstrand, P., Kim, C., Groisman, A., et al. (2013). The nucleus of endothelial cell as a sensor of blood flow direction. Biol. Open. 2, 1007–1012. doi: 10.1242/bio.20134622
Tovar-Lopez, F., Thurgood, P., Gilliam, C., Nguyen, N., Pirogova, E., Khoshmanesh, K., et al. (2019). A microfluidic system for studying the effects of disturbed flow on endothelial cells. Front. Bioeng. Biotechnol. 7:81. doi: 10.3389/fbioe.2019.00081
Trachet, B., Swillens, A., Van Loo, D., Casteleyn, C., De Paepe, A., Loeys, B., et al. (2009). The influence of aortic dimensions on calculated wall shear stress in the mouse aortic arch. Comput. Methods Biomech. Biomed. Engin. 12, 491–499. doi: 10.1080/10255840802695445
Turner, C. J., Badu-Nkansah, K., and Hynes, R. O. (2017). Endothelium-derived fibronectin regulates neonatal vascular morphogenesis in an autocrine fashion. Angiogenesis 20, 519–531. doi: 10.1007/s10456-017-9563-8
Tzima, E., Del Pozo, M. A., Kiosses, W. B., Mohamed, S. A., Li, S., Chien, S., et al. (2002). Activation of Rac1 by shear stress in endothelial cells mediates both cytoskeletal reorganization and effects on gene expression. Embo J. 21, 6791–6800. doi: 10.1093/emboj/cdf688
Tzima, E., Irani-Tehrani, M., Kiosses, W. B., Dejana, E., Schultz, D. A., Engelhardt, B., et al. (2005). A mechanosensory complex that mediates the endothelial cell response to fluid shear stress. Nature 437, 426–431. doi: 10.1038/nature03952
Udan, R. S., Vadakkan, T. J., and Dickinson, M. E. (2013). Dynamic responses of endothelial cells to changes in blood flow during vascular remodeling of the mouse yolk sac. Development 140, 4041–4050. doi: 10.1242/dev.096255
Usami, S., Chen, H. H., Zhao, Y., Chien, S., and Skalak, R. (1993). Design and construction of a linear shear stress flow chamber. Ann. Biomed. Eng. 21, 77–83. doi: 10.1007/BF02368167
van Duinen, V., Trietsch, S. J., Joore, J., Vulto, P., and Hankemeier, T. (2015). Microfluidic 3D cell culture: from tools to tissue models. Curr. Opin. Biotechnol. 35, 118–126. doi: 10.1016/j.copbio.2015.05.002
Vickerman, V., and Kamm, R. D. (2012). Mechanism of a flow-gated angiogenesis switch: early signaling events at cell-matrix and cell-cell junctions. Integr. Biol. 4, 863–874. doi: 10.1039/c2ib00184e
Vion, A. C., Alt, S., Klaus-Bergmann, A., Szymborska, A., Zheng, T., Perovic, T., et al. (2018). Primary cilia sensitize endothelial cells to BMP and prevent excessive vascular regression. J. Cell Biol. 217, 1651–1665. doi: 10.1083/jcb.201706151
Voyvodic, P. L., Min, D., and Baker, A. B. (2012). A multichannel dampened flow system for studies on shear stress-mediated mechanotransduction. Lab. Chip. 12, 3322–3330. doi: 10.1039/c2lc40526a
Wang, C., Baker, B. M., Chen, C. S., and Schwartz, M. A. (2013). Endothelial cell sensing of flow direction. Arterioscler. Thromb. Vasc. Biol. 33, 2130–2136. doi: 10.1161/ATVBAHA.113.301826
Wang, S., Park, S., Fei, P., and Sorenson, C. M. (2011). Bim is responsible for the inherent sensitivity of the developing retinal vasculature to hyperoxia. Dev. Biol. 349, 296–309. doi: 10.1016/j.ydbio.2010.10.034
Wang, Y., Chang, J., Chen, K. D., Li, S., Li, J. Y., Wu, C., et al. (2007). Selective adapter recruitment and differential signaling networks by VEGF vs. shear stress. Proc. Natl. Acad. Sci. U.S.A. 104, 8875–8879. doi: 10.1073/pnas.0703088104
Watson, E. C., Grant, Z. L., and Coultas, L. (2017). Endothelial cell apoptosis in angiogenesis and vessel regression. Cell Mol. Life Sci. 74, 4387–4403. doi: 10.1007/s00018-017-2577-y
Watson, M. G., McDougall, S. R., Chaplain, M. A., Devlin, A. H., and Mitchell, C. A. (2012). Dynamics of angiogenesis during murine retinal development: a coupled in vivo and in silico study. J. R. Soc Interface 9, 2351–2364. doi: 10.1098/rsif.2012.0067
Williams, J. L., Cartland, D., Hussain, A., and Egginton, S. (2006a). A differential role for nitric oxide in two forms of physiological angiogenesis in mouse. J. Physiol. 570(Pt 3):445–454. doi: 10.1113/jphysiol.2005.095596
Williams, J. L., Weichert, A., Zakrzewicz, A., Da Silva-Azevedo, L., Pries, A. R., Baum, O., et al. (2006b). Differential gene and protein expression in abluminal sprouting and intraluminal splitting forms of angiogenesis. Clin. Sci. 110, 587–595. doi: 10.1042/CS20050185
Wojciak-Stothard, B., and Ridley, A. J. (2003). Shear stress-induced endothelial cell polarization is mediated by Rho and Rac but not Cdc42 or PI 3-kinases. J. Cell Biol. 161, 429–439. doi: 10.1083/jcb.200210135
Wong, K. H., Chan, J. M., Kamm, R. D., and Tien, J. (2012). Microfluidic models of vascular functions. Annu. Rev. Biomed. Eng. 14, 205–230. doi: 10.1146/annurev-bioeng-071811-150052
Wu, Z., Guo, H., Chow, N., Sallstrom, J., Bell, R. D., and Deane, R. (2005). Role of the MEOX2 homeobox gene in neurovascular dysfunction in Alzheimer disease. Nat Med. 11, 959–965. doi: 10.1038/nm1287
Yan, C., Takahashi, M., Okuda, M., Lee, J. D., and Berk, B. C. (1999). Fluid shear stress stimulates big mitogen-activated protein kinase 1 (BMK1) activity in endothelial cells. Dependence on tyrosine kinases and intracellular calcium. J. Biol. Chem. 274, 143–150. doi: 10.1074/jbc.274.1.143
Yoshino, D., Sakamoto, N., and Sato, M. (2017). Fluid shear stress combined with shear stress spatial gradients regulates vascular endothelial morphology. Integr. Biol. 9, 584–594. doi: 10.1039/C7IB00065K
Yun, S., Dardik, A., Haga, M., Yamashita, A., Yamaguchi, S., Koh, Y., et al. (2002). Transcription factor Sp1 phosphorylation induced by shear stress inhibits membrane type 1-matrix metalloproteinase expression in endothelium. J. Biol. Chem. 277, 34808–34814. doi: 10.1074/jbc.M205417200
Zaidel-Bar, R., Kam, Z., and Geiger, B. (2005). Polarized downregulation of the paxillin-p130CAS-Rac1 pathway induced by shear flow. J. Cell Sci. 118(Pt 17), 3997–4007. doi: 10.1242/jcs.02523
Zeng, Y., and Tarbell, J. M. (2014). The adaptive remodeling of endothelial glycocalyx in response to fluid shear stress. PLoS One 9:e86249. doi: 10.1371/journal.pone.0086249
Zhao, W., Cao, L., Ying, H., Zhang, W., Li, D., Zhu, X., et al. (2019). Endothelial CDS2 deficiency causes VEGFA-mediated vascular regression and tumor inhibition. Cell Res. 29, 895–910. doi: 10.1038/s41422-019-0229-5
Zheng, Y., Chen, J., and Lopez, J. A. (2015). Flow-driven assembly of VWF fibres and webs in in vitro microvessels. Nat. Commun. 6:7858. doi: 10.1038/ncomms8858
Zhou, A., Egginton, S., Hudlicka, O., and Brown, M. D. (1998). Internal division of capillaries in rat skeletal muscle in response to chronic vasodilator treatment with alpha1-antagonist prazosin. Cell Tissue Res. 293, 293–303. doi: 10.1007/s004410051121
Keywords: microvascular remodeling, capillary pruning, capillary splitting, blood flow, shear stress, endothelial cells, 3D-confocal microscopy, microfluidics
Citation: Santamaría R, González-Álvarez M, Delgado R, Esteban S and Arroyo AG (2020) Remodeling of the Microvasculature: May the Blood Flow Be With You. Front. Physiol. 11:586852. doi: 10.3389/fphys.2020.586852
Received: 24 July 2020; Accepted: 09 September 2020;
Published: 15 October 2020.
Edited by:
Anna Rita Cantelmo, Université Lille Nord de France, FranceReviewed by:
Oliver Baum, Charité – Universitätsmedizin Berlin, GermanyAnne-Clémence Vion, INSERM U1087 L’Unité de Recherche de l’Institut Duthorax, France
Copyright © 2020 Santamaría, González-Álvarez, Delgado, Esteban and Arroyo. This is an open-access article distributed under the terms of the Creative Commons Attribution License (CC BY). The use, distribution or reproduction in other forums is permitted, provided the original author(s) and the copyright owner(s) are credited and that the original publication in this journal is cited, in accordance with accepted academic practice. No use, distribution or reproduction is permitted which does not comply with these terms.
*Correspondence: Alicia G. Arroyo, YWdhcnJveW9AY2liLmNzaWMuZXM=
†These authors have contributed equally to this work