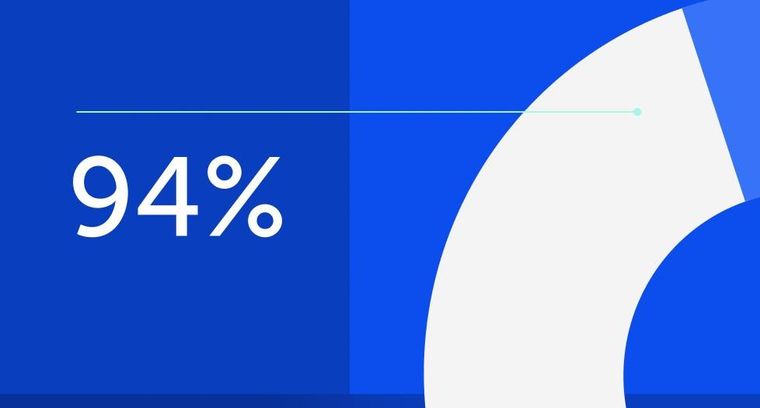
94% of researchers rate our articles as excellent or good
Learn more about the work of our research integrity team to safeguard the quality of each article we publish.
Find out more
MINI REVIEW article
Front. Physiol., 20 January 2021
Sec. Clinical and Translational Physiology
Volume 11 - 2020 | https://doi.org/10.3389/fphys.2020.583191
This article is part of the Research TopicNon-coding RNA as Therapeutic Target: A Game Changer in Cardiac Regenerative Strategies?View all 10 articles
Aging imposes a barrier for tissue regeneration. In the heart, aging leads to a severe rearrangement of the cardiac structure and function and to a subsequent increased risk of heart failure. An intricate network of distinct pathways contributes to age-related alterations during healthy heart aging and account for a higher susceptibility of heart disease. Our understanding of the systemic aging process has already led to the design of anti-aging strategies or to the adoption of protective interventions. Nevertheless, our understanding of the molecular determinants operating during cardiac aging or repair remains limited. Here, we will summarize the molecular and physiological alterations that occur during aging of the heart, highlighting the potential role for long non-coding RNAs (lncRNAs) as novel and valuable targets in cardiac regeneration/repair.
Worldwide, cardiovascular diseases are the leading cause of death, causing nearly 18 million deaths in 2017. Cardiovascular diseases comprise several pathological conditions, including heart failure (Yusuf et al., 2001; Lloyd-Jones et al., 2009, 2010; Mensah et al., 2019). Aging is probably the most important risk factor for heart failure (Li et al., 2020a). As opposed to the neonatal heart, adult mammalian hearts lose their capacity to fully regenerate after an exogenous or endogenous harm (Lam and Sadek, 2018). This may be mediated through several interconnected processes, including cellular senescence and secreted factors, telomere attrition, mitochondrial damage, cell death, or inflammation (for a comprehensive review on age-related pathways affecting the heart, see Li et al., 2020a). Although a partial myocyte turnover has been observed in adult heart after damage (e.g., myocardial infarction), it only partially and slightly restores heart function. For instance, it has been recently demonstrated that manipulation of telomere length through the expression of telomerase, whose expression is silenced in the mouse heart from day 5 to 7 (Blasco et al., 1995; Borges and Liew, 1997; Richardson et al., 2012), may be beneficial in heart healing and healthspan (Bernardes de Jesus and Blasco, 2011; Bar et al., 2014).
Long non-coding RNAs (lncRNAs) have emerged as important regulators of epigenetic modulation and gene expression. Although deprived from coding potential, lncRNAs have been associated with several biological processes, including dosage compensation, genomic imprinting, aging, and cell differentiation (Mercer et al., 2009; Rinn and Chang, 2012; Sousa-Franco et al., 2019; Yao et al., 2019). Furthermore, lncRNAs have been linked to several diseases including cardiovascular diseases (Hobuß et al., 2019; Abbas et al., 2020). In this review, we will discuss the cardiac regeneration properties of neonatal and adult hearts. We will focus on lncRNAs and their potential role in cardiac regeneration briefly discussing the potential of fibroblasts as a source of cardiomyocytes for regenerative medicine purposes.
There is a general consensus on the capacity of neonatal hearts to regenerate, after distinct types of damage (Supplementary Figure 1 – Porrello et al., 2011, 2013; Haubner et al., 2012, 2016; Jesty et al., 2012; Mahmoud et al., 2013, 2014, 2015; Rubin et al., 2013; Andersen et al., 2014, 2016; Aurora et al., 2014; Sadek et al., 2014; Bryant et al., 2015; Darehzereshki et al., 2015; Han et al., 2015; Jiang et al., 2015; Konfino et al., 2015; Aix et al., 2016; Blom et al., 2016; Kang et al., 2016; Tao et al., 2016; Valiente-Alandi et al., 2016; Xiong and Hou, 2016; Yu et al., 2016; Ai et al., 2017; Bassat et al., 2017; Malek Mohammadi et al., 2017; Zebrowski et al., 2017; Ahmed et al., 2018; Ingason et al., 2018; Sampaio-Pinto et al., 2018; Sereti et al., 2018; Cai et al., 2019; Elhelaly et al., 2019; Wang et al., 2019b; Fan et al., 2020; Pei et al., 2020; Li et al., 2020b, 2020c). A comprehensive overview of neonatal heart regeneration studies has been previously and elegantly detailed by Lam and Sadek (2018). Heart regeneration seems to be dependent on the type of injury that causes loss of cardiomyocytes. For example, cryoinjury does not induce the same level of regeneration as apical resection or myocardial infarction. Furthermore, it is likely that neonatal heart regeneration is mediated by the proliferation of pre-existing cardiomyocytes, and not by cardiac stem or progenitor cells. This regenerative state occurs in an extremely short time frame (<10days; Eschenhagen et al., 2017) and, just a few days after birth, cardiomyocytes exit the cell cycle resulting in a decline in heart regeneration capacity. This is accompanied by other alterations in the cardiomyocytes, including their metabolic needs or changes in the expression of both coding and non-coding genes. Subsequently, several strategies have been designed for regenerating the adult heart. Those approaches may include the forced re-entering in the cell cycle of the pre-existing cardiomyocytes, or may include cell transdifferentiation strategies, in which somatic cells can be converted into functional cardiomyocytes for cell replacement therapy (Qian et al., 2012; Addis and Epstein, 2013; Nam et al., 2013; Wada et al., 2013; Ghiroldi et al., 2017; Amin et al., 2018; Engel and Ardehali, 2018a).
The importance of lncRNAs in heart regeneration has been brought to light recently (Bar et al., 2016; Abbas et al., 2020). LncRNAs are a vast category of non-coding, poorly conserved, and tissue- and developmental stage-specific transcripts with distinct functions in several biological processes, including epigenetic, transcriptional, and post-transcriptional regulation. Regarding the role of lncRNAs in heart regeneration, we will discuss some recent studies describing lncRNAs directly acting (promoting or inhibiting) on heart regeneration (Table 1).
P7 mice subjected to LAD ligation and injected with adenovirus containing NR_045363 exhibited improved left ventricular ejection fraction and reduced infarct size compared to the control-injected group (Wang et al., 2019a). Mice overexpressing NR_045363 showed higher expression of cardiomyocyte mitotic markers, such as Ki67 and phosphorylated histone H3 (pH3), suggesting that improved heart function after MI was due to cardiomyocyte proliferation. The authors reported that NR_045363 acted as a competing endogenous RNA (ceRNA), binding to miR-216a (Wang et al., 2019a). miR-216a is known to repress JAK2, leading to decreased levels of phosphorylation of STAT3 (Hou et al., 2015). Furthermore, deletion of STAT3 was shown to impair cardiomyocyte proliferation after apical resection (Kurdi et al., 2018), suggesting that NR_045363 promoted cardiomyocyte proliferation by modulating the JAK2-STAT3 pathway. So, the absence of NR_045363 (which results in an upregulation of miR216a) led to reduced activity of the JAK2-STAT3, whilst NR_045363 overexpression (which leads to a downregulation of miR-216a) resulted in an increase of the phosphorylation levels of JAK2 and STAT3, thus promoting cardiomyocyte proliferation (Wang et al., 2019a). More recently, NR_045363 was associated with cardiomyocyte apoptosis. Chen et al. (2020) reported that loss of NR_045363 led to the activation of the p53 signaling pathway, promoting apoptosis. On the other hand, overexpressing NR_045363 inhibited apoptosis and improved cardiac function after MI, thus potentially mediating the cardiac functions observed after NR_045363 modulation.
Long non-coding RNA endogenous cardiac regeneration-associated regulator (ECRAR) was found to be upregulated in the fetal heart, and its expression gradually decreased in postnatal hearts. Overexpression of ECRAR in postnatal rat cardiomyocytes, both in vitro and in vivo, resulted in an increase of DNA synthesis, and an increase of cytokinesis (pH3 and aurora B kinase), suggesting a direct involvement in cardiomyocytes proliferation (Chen et al., 2019). Overexpression of ECRAR resulted in the phosphorylation of ERK1/2, their subsequent translocation to the nucleus and the transcription of cell proliferation and cell cycle-related genes (Chen et al., 2019). Li et al. (2018a) identified Sirt1 antisense lncRNA (Sirt1-as), whose expression was high during heart development. Overexpression of this lncRNA resulted in an increase of Ki67- and pH3-positive cardiomyocytes. On the other hand, silencing of Sirt1-as, both in vitro and in vivo, led to a decrease of Ki67- and pH3-positive cardiomyocytes, indicating a potential decline in cell division (Li et al., 2018a). Furthermore, overexpression of Sirt1-as after MI in adult mice resulted in an increased expression of cell-cycle specific factors Ki67 and pH3, thus suggesting a potential implication in cardiac health (Li et al., 2018a).
More recently, Wilson et al. (2020) described BANCR, a lncRNA exclusively expressed in primate fetal cardiomyocytes. BANCR promotes cardiomyocyte migration in vitro and ventricular enlargement in vivo. To elucidate the regulation of BANCR in cardiomyocytes, the authors suggested that TBX5 binding was responsible for the fetal heart-specific expression of BANCR. Additionally, the authors identified TEAD4 and YAP1 (two factors involved in the HIPPO pathway) in the same enhancer, promoting BANCR expression. Finally, the authors identified a role for BANCR in heart disease, demonstrating higher expression in pediatric but not adult dilated cardiomyopathy (Nelakanti and Xiao, 2020; Wilson et al., 2020). Other lncRNAs associated with aged hearts include lncRNA H19 (downregulated in aged or ischemic heart; Hofmann et al., 2019), and MALAT1 a lncRNA which, itself, is regulated by an antisense lncRNA transcript (TALAM1; Zong et al., 2016; Gomes et al., 2019), was also shown to be decreased in aged hearts (Bink et al., 2019; Gomes et al., 2019), and this decrease was shown to be involved in cardiac dysfunction (Zhu et al., 2019; Li et al., 2020a).
Cai et al. (2018) explored the role of lncRNAs during heart regeneration after ischemic injury, in both neonatal and adult mice. CAREL, a lncRNA whose expression gradually increased in the neonatal hearts from P1 to P10 mice, with P7 corresponding to the time point at which the heart regenerative capacity is lost in mice (Cai et al., 2018). Cardiac-specific overexpression of CAREL led to a decrease of cardiomyocyte proliferation and reduced heart regeneration in neonatal mice after injury. On the contrary, silencing CAREL promoted cardiac regeneration and improved heart functional parameters after myocardial infarction in neonatal and adult mice (Cai et al., 2018). CAREL was found to be a ceRNA, sequestering miR-296. It was suggested that the CAREL-miR-296 interaction led to the activation of Trp53inp1 and Itm2a, leading to a decrease in cardiomyocyte proliferation, thus resulting in a reduction of regeneration. Intramyocardial administration of CAREL to p1 neonatal mice inhibited cardiomyocyte mitosis and increased the formation of cardiac scar and, on the other hand, overexpression of miR-256 promoted cardiomyocyte proliferation and cardiac regeneration after injury. Similarly, lncRNA cardiomyocyte proliferation regulator (CPR) was shown to be a negative regulator of cardiomyocyte proliferation and cardiac repair. Ponnusamy et al. (2019) observed that higher levels of CPR hampered cardiomyocyte proliferation, whilst silencing CPR resulted in cardiomyocyte proliferation in postnatal and adult hearts. CPR expression levels were found to be higher in the adult heart, which is consistent with their lack of regeneration. The authors reported that CPR recruits DNMT3A to several locus leading, in particular, to increased levels of methylation in the MCM3 promoter (Ponnusamy et al., 2019). In dividing tissues, MCM3 promotes the initiation of DNA replication and cell cycle progression (Lin et al., 2008), something halted by CPR in the heart and leading to the inhibition of cardiomyocytes proliferation.
Another lncRNA that negatively regulates cardiac regeneration is LncDACH1. This lncRNA was found to be gradually upregulated in postnatal hearts, which is in accordance with the loss of myocardial regenerative capacity soon after birth (Cai et al., 2020). The authors suggest that LncDACH1 binds protein phosphatase 1 catalytic subunit alpha (PP1A), reducing its dephosphorylation capacity, and increases the phosphorylation of yes-associated protein 1 (YAP1), preventing its translocation to the nucleus and, thus, the activation of cell proliferation-related genes (von Gise et al., 2012; Cai et al., 2020). Cardiac-specific overexpression of LncDACH1 resulted in the suppression of neonatal heart regeneration and aggravation of cardiac function after apical resection. These phenotypes were accompanied by a decrease in the number of cardiac-cells expressing proliferative markers (Cai et al., 2020). Cardiomyocyte regeneration-related lncRNA (CRRL) was also found to be involved in heart regeneration. CRRL silencing was associated with an increased expression of EdU, Ki67, and pH3 in P1 and P7 rat cardiomyocytes (Chen et al., 2018). Similar results were obtained in neonatal rats post-MI, concomitantly with better prognosis such as reduction of the fibrotic length of the infarct wall and fibrosis area in the non-infarct zone. Instead, overexpression of CRRL leads to a decrease in pH3-positive cardiomyocytes and inhibition of functional recovery post-MI. CRRL function seemed to be mediated through the binding to miR-199a-3p, resulting in an increased expression of Hopx, which is a negative regulator of cardiomyocyte proliferation (Trivedi et al., 2010).
LncRNA AZIN2-sv, a splice variant of the AZIN2 gene, was found to be upregulated in human adult hearts. AZIN2-sv was reported to negatively regulate cardiomyocyte proliferation, both in vitro and in vivo (Li et al., 2018b). Overexpression of AZIN2-sv led to an anti-proliferative phenotype, marked by decreased levels of EdU-, Ki67-, pH3-, and Aurora-B. On the other hand, silencing AZIN2-sv promoted cardiomyocyte proliferation and improved cardiac function after MI. AZIN2-sv sequesters miR-214, leading to the release of its target PTEN, resulting in a decrease in the phosphorylation of Akt and Cyclin-D, therefore inhibiting cardiomyocyte proliferation. Reduced levels of AZIN2-sv allow miR-214 to repress PTEN, leading to increased levels of phosphorylated Akt and Cyclin-D1, thus promoting cardiomyocyte proliferation.
More recently, Trembinski et al. (2020) identified lncRNA SCOT1-antisense RNA regulated during aging in the heart (SARRAH), whose expression declines during aging. Inhibition of Sarrah induces caspase activity in mouse and human cardiomyocytes, promoting apoptosis. Gene set enrichment analysis after SARRAH silencing showed enrichment of apoptosis-related pathways, corroborating previous observations (Trembinski et al., 2020). SARRAH was also found to directly bind to the promoters through RNA-DNA triplex helix structures, suggesting that its binding may activate gene expression. Indeed, it was reported that SARRAH interacted with cardiac transcription factor cysteine-rich protein 2 (CRIP2) and p300, which acetylates histone H3 lysine 27 to activate transcription (Trembinski et al., 2020). On the other hand, overexpression of SARRAH led to a decrease in caspase activity. In adult mice a decline in apoptosis was observed after overexpressing SARRAH, suggesting that reduced expression levels of this lncRNA in aged mice might contribute to cardiomyocyte cell death in vivo. Furthermore, reduced levels of Sarrah were observed in the infarcted and border regions after acute MI (Trembinski et al., 2020).
Furthermore, several lncRNAs have been identified as promoters of cardiac fibrosis (Liang et al., 2018; Wang et al., 2018; Hao et al., 2019; Zhang et al., 2019a). Aged tissues accumulate signals that promote the epithelial-mesenchymal transition (EMT), inducing the transdifferentiation of epithelial cells to mesenchymal cells, such as fibroblasts, which are the main mediators of fibrosis through the deposition of extracellular matrix. In the heart, many fibroblasts derive from endothelial cells, leading to excessive deposition of extracellular matrix and causing cardiac fibrosis, which is common in patients with heart failure (reviewed by Santos et al., 2019). Thus, targeting these lncRNAs may also be considered for improving heart function.
Cell reprogramming has emerged as a novel strategy for regenerative medicine and cell-based therapy. The reprogramming of mouse and human fibroblasts into induced pluripotent stem cells (iPSCs) using transcription factors (TFs) known to play key roles in the maintenance of embryonic stem cell identity suggested that patient-derived iPSCs could be produced from somatic cells. This strategy allowed the conversion of fully differentiated cells into cells with the potency to be differentiated in tissues from different development lineages (Takahashi and Yamanaka, 2006; Takahashi et al., 2007; Yamanaka, 2009; Abad et al., 2013). Additionally, many of the reprogramming barriers, such as the obstacles imposed by aging, have been addressed through the direct manipulation of tumor suppressor genes, p53 or Ink4a/ARF (Li et al., 2009; Marion et al., 2009), or the EMT-promoting factor ZEB2 (Bernardes de Jesus et al., 2018; Santos et al., 2019).
The reprogramming of fibroblasts into iPSCs opened doors to direct cell reprogramming. Direct cardiac reprogramming of fibroblasts into cardiomyocytes [usually termed induced cardiomyocytes (iCMs)] has emerged as an attractive strategy for replacing lost or damaged cells in the heart. Mouse postnatal cardiac and dermal fibroblasts have been transdifferentiated into iCMs through the combined expression of three different cardiac-specific TFs: Gata4, Mef2c, and Tbx5 (GMT). The ectopic expression of GMT activates a cardiac-like gene expression program and promotes the conversion of fibroblasts into iCMs (Ieda et al., 2010; Qian et al., 2012). Comparative gene expression analyses reported that iCMs generated in vitro exhibited bona fide adult cardiomyocyte-like features, such as fatty acid oxidation or cell cycle exit (Muraoka et al., 2019). Remarkably, this approach has been adapted in vivo, where cardiac fibroblasts have been transdifferentiated into iCMs (Song et al., 2012; Zhang et al., 2019b, 2019c), bypassing the need to revert fibroblasts to a pluripotent state (Liu et al., 2017; Muraoka et al., 2019). Endogenous cardiac fibroblasts comprise about 50% of all the cells in the heart, making them a potential source of cardiomyocytes for regenerative therapy (Ieda et al., 2010). In fact, iCMs reprogrammed from endogenous cardiac fibroblasts enhanced cardiac function after myocardial infarction, fully demonstrating the potential of this strategy for cardiac repair (Miyamoto et al., 2018; Bektik and Fu, 2019; Lee et al., 2020).
Despite several encouraging results, current reprogramming methodologies remain somewhat inefficient, as very few fibroblasts are fully converted into functional iCMs. Differential expression patterns of lncRNAs have been observed in several developmental stages, including cardiogenesis, and involve the expression of lncRNAs Braveheart, Fendrr, and Carmen. In fact, lncRNA ZEB2-NAT has been modulated in order to improve the reprogramming of fibroblasts into iPSCs (Bernardes de Jesus et al., 2018). Having these concepts in mind, it seems reasonable to expect that modulating lncRNAs might improve the efficiency of direct cardiac reprogramming.
Regarding phenoconversion of cardiac cells, it is important to mention, however, that many of the current protocols depend on viral vectors for gene delivery. There are a few safety issues associated with the use of lentiviral and retroviral vectors, as they integrate their genome in the host cell. They could potentially disturb endogenous gene expression and are associated with the risk of insertional mutagenesis, hampering the clinical application of this method. However, non-integrative viruses, such as Sendai virus, and non-viral reprogramming systems have emerged as safer alternatives for clinical application (Engel and Ardehali, 2018b; Miyamoto et al., 2018; Tani et al., 2018; Chang et al., 2019).
As previously discussed, several lncRNAs are expressed during the development of the heart and during heart pathologies. Targeting lncRNAs may be a novel strategy against heart diseases (Bar et al., 2016). Technically, the development of specific and deliverable antisense transcripts (e.g., LNA-GapmeRs) has been proved powerful and efficient carriers for in vivo targeting and RNase H-mediated degradation of specific targets (Bernardes de Jesus et al., 2018). Similar approaches may be designed for expression of selected lncRNAs, downregulated in cardiac diseases. We have to face, however, that most human lncRNAs are non-conserved between species, making it extremely challenging to identify the functional lncRNAs in vivo. The lack of sequence conservation poses a challenge for the translational application of human lncRNAs. Since lncRNAs are species-specific, we often can only visualize their impact when studied in their specific system. This challenge may only be addressed through a humanized experimental model where the detailed function of non-conserved lncRNAs may be tested. In conclusion, understand lncRNAs specific profiles in dividing vs. non-dividing cardiomyocytes may allow the detection of potentially druggable targets for adult heart repair.
FS, MC, SN-P, and BB planned, wrote, and discussed the paper. SN-P and BB revised the paper. All authors contributed to the article and approved the submitted version.
This work was supported by Fundação para a Ciência e Tecnologia (FCT; ERA-CVD 2018 / 3599-PPCDT - ERA-CVD/0001/2018 - INNOVATION).
The authors declare that the research was conducted in the absence of any commercial or financial relationships that could be construed as a potential conflict of interest.
The Supplementary Material for this article can be found online at: https://www.frontiersin.org/articles/10.3389/fphys.2020.583191/full#supplementary-material
Supplementary Figure 1 | Studies on neonatal heart regeneration depicting different methodologies. The studies with blue background observed heart regeneration whether the studies in red could not detect heart healing.
Abad, M., Mosteiro, L., Pantoja, C., Canamero, M., Rayon, T., Ors, I., et al. (2013). Reprogramming in vivo produces teratomas and iPS cells with totipotency features. Nature 502, 340–345. doi: 10.1038/nature12586
Abbas, N., Perbellini, F., and Thum, T. (2020). Non-coding RNAs: emerging players in cardiomyocyte proliferation and cardiac regeneration. Basic Res. Cardiol. 115:52. doi: 10.1007/s00395-020-0816-0
Addis, R. C., and Epstein, J. A. (2013). Induced regeneration--the progress and promise of direct reprogramming for heart repair. Nat. Med. 19, 829–836. doi: 10.1038/nm.3225
Ahmed, A., Wang, T., and Delgado-Olguin, P. (2018). Ezh2 is not required for cardiac regeneration in neonatal mice. PLoS One 13:e0192238. doi: 10.1371/journal.pone.0192238
Ai, S., Yu, X., Li, Y., Peng, Y., Li, C., Yue, Y., et al. (2017). Divergent requirements for EZH1 in heart development versus regeneration. Circ. Res. 121, 106–112. doi: 10.1161/CIRCRESAHA.117.311212
Aix, E., Gutierrez-Gutierrez, O., Sanchez-Ferrer, C., Aguado, T., and Flores, I. (2016). Postnatal telomere dysfunction induces cardiomyocyte cell-cycle arrest through p21 activation. J. Cell Biol. 213, 571–583. doi: 10.1083/jcb.201510091
Amin, M., Kushida, Y., Wakao, S., Kitada, M., Tatsumi, K., and Dezawa, M. (2018). Cardiotrophic growth factor-driven induction of human muse cells into cardiomyocyte-like phenotype. Cell Transplant. 27, 285–298. doi: 10.1177/0963689717721514
Andersen, D. C., Ganesalingam, S., Jensen, C. H., and Sheikh, S. P. (2014). Do neonatal mouse hearts regenerate following heart apex resection? Stem Cell Rep. 2, 406–413. doi: 10.1016/j.stemcr.2014.02.008
Andersen, D. C., Jensen, C. H., Baun, C., Hvidsten, S., Zebrowski, D. C., Engel, F. B., et al. (2016). Persistent scarring and dilated cardiomyopathy suggest incomplete regeneration of the apex resected neonatal mouse myocardium--a 180 days follow up study. J. Mol. Cell. Cardiol. 90, 47–52. doi: 10.1016/j.yjmcc.2015.11.031
Aurora, A. B., Porrello, E. R., Tan, W., Mahmoud, A. I., Hill, J. A., Bassel-Duby, R., et al. (2014). Macrophages are required for neonatal heart regeneration. J. Clin. Invest. 124, 1382–1392. doi: 10.1172/JCI72181
Bar, C., Bernardes de Jesus, B., Serrano, R., Tejera, A., Ayuso, E., Jimenez, V., et al. (2014). Telomerase expression confers cardioprotection in the adult mouse heart after acute myocardial infarction. Nat. Commun. 5:5863. doi: 10.1038/ncomms6863
Bar, C., Chatterjee, S., and Thum, T. (2016). Long noncoding RNAs in cardiovascular pathology, diagnosis, and therapy. Circulation 134, 1484–1499. doi: 10.1161/CIRCULATIONAHA.116.023686
Bassat, E., Mutlak, Y. E., Genzelinakh, A., Shadrin, I. Y., Baruch Umansky, K., Yifa, O., et al. (2017). The extracellular matrix protein agrin promotes heart regeneration in mice. Nature 547, 179–184. doi: 10.1038/nature22978
Bektik, E., and Fu, J. -D. (2019). Ameliorating the fibrotic remodeling of the heart through direct cardiac reprogramming. Cell 8:679. doi: 10.3390/cells8070679
Bernardes de Jesus, B., and Blasco, M. A. (2011). Aging by telomere loss can be reversed. Cell Stem Cell 8, 3–4. doi: 10.1016/j.stem.2010.12.013
Bernardes de Jesus, B., Marinho, S. P., Barros, S., Sousa-Franco, A., Alves-Vale, C., Carvalho, T., et al. (2018). Silencing of the lncRNA Zeb2-NAT facilitates reprogramming of aged fibroblasts and safeguards stem cell pluripotency. Nat. Commun. 9:94. doi: 10.1038/s41467-017-01921-6
Bink, D. I., Lozano-Vidal, N., and Boon, R. A. (2019). Long non-coding RNA in vascular disease and aging. Noncoding RNA 5:26. doi: 10.3390/ncrna5010026
Blasco, M. A., Funk, W., Villeponteau, B., and Greider, C. W. (1995). Functional characterization and developmental regulation of mouse telomerase RNA. Science 269, 1267–1270. doi: 10.1126/science.7544492
Blom, J. N., Lu, X., Arnold, P., and Feng, Q. (2016). Myocardial infarction in neonatal mice, a model of cardiac regeneration. J. Vis. Exp. 54100. doi: 10.3791/54100
Borges, A., and Liew, C. C. (1997). Telomerase activity during cardiac development. J. Mol. Cell. Cardiol. 29, 2717–2724. doi: 10.1006/jmcc.1997.0503
Bryant, D. M., O’meara, C. C., Ho, N. N., Gannon, J., Cai, L., and Lee, R. T. (2015). A systematic analysis of neonatal mouse heart regeneration after apical resection. J. Mol. Cell. Cardiol. 79, 315–318. doi: 10.1016/j.yjmcc.2014.12.011
Cai, B., Ma, W., Ding, F., Zhang, L., Huang, Q., Wang, X., et al. (2018). The long noncoding RNA CAREL controls cardiac regeneration. J. Am. Coll. Cardiol. 72, 534–550. doi: 10.1016/j.jacc.2018.04.085
Cai, B., Ma, W., Wang, X., Sukhareva, N., Hua, B., Zhang, L., et al. (2020). Targeting LncDACH1 promotes cardiac repair and regeneration after myocardium infarction. Cell Death Differ. 27, 2158–2175. doi: 10.1038/s41418-020-0492-5
Cai, W., Tan, J., Yan, J., Zhang, L., Cai, X., Wang, H., et al. (2019). Limited regeneration potential with minimal Epicardial progenitor conversions in the neonatal mouse heart after injury. Cell Rep. 28, 190–201.e3. doi: 10.1016/j.celrep.2019.06.003
Chang, Y., Lee, E., Kim, J., Kwon, Y. W., and Kwon, Y. (2019). Efficient in vivo direct conversion of fibroblasts into cardiomyocytes using a nanoparticle-based gene carrier. Biomaterials 192, 500–509. doi: 10.1016/j.biomaterials.2018.11.034
Chen, G., Li, H., Li, X., Li, B., Zhong, L., Huang, S., et al. (2018). Loss of long non-coding RNA CRRL promotes cardiomyocyte regeneration and improves cardiac repair by functioning as a competing endogenous RNA. J. Mol. Cell. Cardiol. 122, 152–164. doi: 10.1016/j.yjmcc.2018.08.013
Chen, Y., Li, X., Li, B., Wang, H., Li, M. S., Huang, S., et al. (2019). Long non-coding RNA ECRAR triggers post-natal myocardial regeneration by activating ERK1/2 signaling. Mol. Ther. 27, 29–45. doi: 10.1016/j.ymthe.2018.10.021
Chen, X., Wang, J., Nie, Y., and Chu, M. (2020). The long noncoding RNA NR_045363 involves cardiomyocyte apoptosis and cardiac repair via p53 signal pathway. Cell Biol. Int. 44, 1957–1965. doi: 10.1002/cbin.11374
Darehzereshki, A., Rubin, N., Gamba, L., Kim, J., Fraser, J., Huang, Y., et al. (2015). Differential regenerative capacity of neonatal mouse hearts after cryoinjury. Dev. Biol. 399, 91–99. doi: 10.1016/j.ydbio.2014.12.018
Elhelaly, W. M., Cardoso, A. C., Pereira, A. H. M., Elnawasany, A., Ebrahimi, S., Nakada, Y., et al. (2019). C-kit cells do not significantly contribute to cardiomyogenesis during neonatal heart regeneration. Circulation 139, 559–561. doi: 10.1161/CIRCULATIONAHA.117.033150
Engel, J. L., and Ardehali, R. (2018a). Direct cardiac reprogramming: progress and promise. Stem Cells Int. 2018:1435746. doi: 10.1155/2018/1435746
Engel, J. L., and Ardehali, R. (2018b). Sendai virus based direct cardiac reprogramming: what lies ahead? Stem Cell Investig. 5:37. doi: 10.21037/sci.2018.10.02
Eschenhagen, T., Bolli, R., Braun, T., Field, L. J., Fleischmann, B. K., Frisen, J., et al. (2017). Cardiomyocyte regeneration: a consensus statement. Circulation 136, 680–686. doi: 10.1161/CIRCULATIONAHA.117.029343
Fan, Y., Cheng, Y., Li, Y., Chen, B., Wang, Z., Wei, T., et al. (2020). Phosphoproteomic analysis of neonatal regenerative myocardium revealed important roles of checkpoint kinase 1 via activating mammalian target of rapamycin C1/ribosomal protein S6 kinase b-1 pathway. Circulation 141, 1554–1569. doi: 10.1161/CIRCULATIONAHA.119.040747
Ghiroldi, A., Piccoli, M., Ciconte, G., Pappone, C., and Anastasia, L. (2017). Regenerating the human heart: direct reprogramming strategies and their current limitations. Basic Res. Cardiol. 112:68. doi: 10.1007/s00395-017-0655-9
Gomes, C. P., Nobrega-Pereira, S., Domingues-Silva, B., Rebelo, K., Alves-Vale, C., Marinho, S. P., et al. (2019). An antisense transcript mediates MALAT1 response in human breast cancer. BMC Cancer 19:771. doi: 10.1186/s12885-019-5962-0
Han, C., Nie, Y., Lian, H., Liu, R., He, F., Huang, H., et al. (2015). Acute inflammation stimulates a regenerative response in the neonatal mouse heart. Cell Res. 25, 1137–1151. doi: 10.1038/cr.2015.110
Hao, K., Lei, W., Wu, H., Wu, J., Yang, Z., Yan, S., et al. (2019). LncRNA-safe contributes to cardiac fibrosis through safe-Sfrp2-HuR complex in mouse myocardial infarction. Theranostics 9, 7282–7297. doi: 10.7150/thno.33920
Haubner, B. J., Adamowicz-Brice, M., Khadayate, S., Tiefenthaler, V., Metzler, B., Aitman, T., et al. (2012). Complete cardiac regeneration in a mouse model of myocardial infarction. Aging 4, 966–977. doi: 10.18632/aging.100526
Haubner, B. J., Schuetz, T., and Penninger, J. M. (2016). A reproducible protocol for neonatal ischemic injury and cardiac regeneration in neonatal mice. Basic Res. Cardiol. 111:64. doi: 10.1007/s00395-016-0580-3
Hobuß, L., Bär, C., and Thum, T. (2019). Long non-coding RNAs: at the heart of cardiac dysfunction? Front. Physiol. 10:30. doi: 10.3389/fphys.2019.00030
Hofmann, P., Sommer, J., Theodorou, K., Kirchhof, L., Fischer, A., Li, Y., et al. (2019). Long non-coding RNA H19 regulates endothelial cell aging via inhibition of STAT3 signalling. Cardiovasc. Res. 115, 230–242. doi: 10.1093/cvr/cvy206
Hou, B. H., Jian, Z. X., Cui, P., Li, S. J., Tian, R. Q., and Ou, J. R. (2015). miR-216a may inhibit pancreatic tumor growth by targeting JAK2. FEBS Lett. 589, 2224–2232. doi: 10.1016/j.febslet.2015.06.036
Ieda, M., Fu, J. D., Delgado-Olguin, P., Vedantham, V., Hayashi, Y., Bruneau, B. G., et al. (2010). Direct reprogramming of fibroblasts into functional cardiomyocytes by defined factors. Cell 142, 375–386. doi: 10.1016/j.cell.2010.07.002
Ingason, A. B., Goldstone, A. B., Paulsen, M. J., Thakore, A. D., Truong, V. N., Edwards, B. B., et al. (2018). Angiogenesis precedes cardiomyocyte migration in regenerating mammalian hearts. J. Thorac. Cardiovasc. Surg. 155, 1118–1127.e1. doi: 10.1016/j.jtcvs.2017.08.127
Jesty, S. A., Steffey, M. A., Lee, F. K., Breitbach, M., Hesse, M., Reining, S., et al. (2012). c-kit+ precursors support postinfarction myogenesis in the neonatal, but not adult, heart. Proc. Natl. Acad. Sci. U. S. A. 109, 13380–13385. doi: 10.1073/pnas.1208114109
Jiang, J., Burgon, P. G., Wakimoto, H., Onoue, K., Gorham, J. M., O’meara, C. C., et al. (2015). Cardiac myosin binding protein C regulates postnatal myocyte cytokinesis. Proc. Natl. Acad. Sci. U. S. A. 112, 9046–9051. doi: 10.1073/pnas.1511004112
Kang, J., Hu, J., Karra, R., Dickson, A. L., Tornini, V. A., Nachtrab, G., et al. (2016). Modulation of tissue repair by regeneration enhancer elements. Nature 532, 201–206. doi: 10.1038/nature17644
Konfino, T., Landa, N., Ben-Mordechai, T., and Leor, J. (2015). The type of injury dictates the mode of repair in neonatal and adult heart. J. Am. Heart Assoc. 4:e001320. doi: 10.1161/JAHA.114.001320
Kurdi, M., Zgheib, C., and Booz, G. W. (2018). Recent developments on the crosstalk between STAT3 and inflammation in heart function and disease. Front. Immunol. 9:3029. doi: 10.3389/fimmu.2018.03029
Lam, N. T., and Sadek, H. A. (2018). Neonatal heart regeneration: comprehensive literature review. Circulation 138, 412–423. doi: 10.1161/CIRCULATIONAHA.118.033648
Lee, S., Park, B. -W., Lee, Y. J., Ban, K., and Park, H. -J. (2020). In vivo combinatory gene therapy synergistically promotes cardiac function and vascular regeneration following myocardial infarction. J. Tissue Eng. 11:2041731420953413. doi: 10.1177/2041731420953413
Li, H., Collado, M., Villasante, A., Strati, K., Ortega, S., Canamero, M., et al. (2009). The Ink4/Arf locus is a barrier for iPS cell reprogramming. Nature 460, 1136–1139. doi: 10.1038/nature08290
Li, Y., Feng, J., Hu, S., and Nie, Y. (2020b). Achieving stable myocardial regeneration after apical resection in neonatal mice. J. Cell. Mol. Med. 24, 6500–6504. doi: 10.1111/jcmm.15223
Li, Y., Feng, J., Song, S., Li, H., Yang, H., Zhou, B., et al. (2020c). Gp130 controls cardiomyocyte proliferation and heart regeneration. Circulation 142, 967–982. doi: 10.1161/CIRCULATIONAHA.119.044484
Li, H., Hastings, M. H., Rhee, J., Trager, L. E., Roh, J. D., and Rosenzweig, A. (2020a). Targeting age-related pathways in heart failure. Circ. Res. 126, 533–551. doi: 10.1161/CIRCRESAHA.119.315889
Li, X., He, X., Wang, H., Li, M., Huang, S., Chen, G., et al. (2018b). Loss of AZIN2 splice variant facilitates endogenous cardiac regeneration. Cardiovasc. Res. 114, 1642–1655. doi: 10.1093/cvr/cvy075
Li, B., Hu, Y., Li, X., Jin, G., Chen, X., Chen, G., et al. (2018a). Sirt1 antisense long noncoding RNA promotes cardiomyocyte proliferation by enhancing the stability of Sirt1. J. Am. Heart Assoc. 7:e009700. doi: 10.1161/JAHA.118.009700
Liang, H., Pan, Z., Zhao, X., Liu, L., Sun, J., Su, X., et al. (2018). LncRNA PFL contributes to cardiac fibrosis by acting as a competing endogenous RNA of let-7d. Theranostics 8, 1180–1194. doi: 10.7150/thno.20846
Lin, D. I., Aggarwal, P., and Diehl, J. A. (2008). Phosphorylation of MCM3 on Ser-112 regulates its incorporation into the MCM2-7 complex. Proc. Natl. Acad. Sci. U. S. A. 105, 8079–8084. doi: 10.1073/pnas.0800077105
Liu, Z., Wang, L., Welch, J. D., Ma, H., Zhou, Y., Vaseghi, H. R., et al. (2017). Single-cell transcriptomics reconstructs fate conversion from fibroblast to cardiomyocyte. Nature 551, 100–104. doi: 10.1038/nature24454
Lloyd-Jones, D., Adams, R. J., Brown, T. M., Carnethon, M., Dai, S., De Simone, G., et al. (2010). Heart disease and stroke statistics--2010 update: a report from the American Heart Association. Circulation 121, e46–e215. doi: 10.1161/CIRCULATIONAHA.109.192667
Lloyd-Jones, D., Adams, R., Carnethon, M., De Simone, G., Ferguson, T. B., Flegal, K., et al. (2009). Heart disease and stroke statistics--2009 update: a report from the American Heart Association statistics committee and stroke statistics subcommittee. Circulation 119, e21–e181. doi: 10.1161/CIRCULATIONAHA.108.191259
Mahmoud, A. I., Kocabas, F., Muralidhar, S. A., Kimura, W., Koura, A. S., Thet, S., et al. (2013). Meis1 regulates postnatal cardiomyocyte cell cycle arrest. Nature 497, 249–253. doi: 10.1038/nature12054
Mahmoud, A. I., O’meara, C. C., Gemberling, M., Zhao, L., Bryant, D. M., Zheng, R., et al. (2015). Nerves regulate cardiomyocyte proliferation and heart regeneration. Dev. Cell 34, 387–399. doi: 10.1016/j.devcel.2015.06.017
Mahmoud, A. I., Porrello, E. R., Kimura, W., Olson, E. N., and Sadek, H. A. (2014). Surgical models for cardiac regeneration in neonatal mice. Nat. Protoc. 9, 305–311. doi: 10.1038/nprot.2014.021
Malek Mohammadi, M., Kattih, B., Grund, A., Froese, N., Korf-Klingebiel, M., Gigina, A., et al. (2017). The transcription factor GATA4 promotes myocardial regeneration in neonatal mice. EMBO Mol. Med. 9, 265–279. doi: 10.15252/emmm.201606602
Marion, R. M., Strati, K., Li, H., Murga, M., Blanco, R., Ortega, S., et al. (2009). A p53-mediated DNA damage response limits reprogramming to ensure iPS cell genomic integrity. Nature 460, 1149–1153. doi: 10.1038/nature08287
Mensah, G. A., Roth, G. A., and Fuster, V. (2019). The global burden of cardiovascular diseases and risk factors: 2020 and beyond. J. Am. Coll. Cardiol. 74, 2529–2532. doi: 10.1016/j.jacc.2019.10.009
Mercer, T. R., Dinger, M. E., and Mattick, J. S. (2009). Long non-coding RNAs: insights into functions. Nat. Rev. Genet. 10, 155–159. doi: 10.1038/nrg2521
Miyamoto, K., Akiyama, M., Tamura, F., Isomi, M., Yamakawa, H., Sadahiro, T., et al. (2018). Direct in vivo reprogramming with Sendai virus vectors improves cardiac function after myocardial infarction. Cell Stem Cell 22, 91–103.e5. doi: 10.1016/j.stem.2017.11.010
Muraoka, N., Nara, K., Tamura, F., Kojima, H., Yamakawa, H., Sadahiro, T., et al. (2019). Role of cyclooxygenase-2-mediated prostaglandin E2-prostaglandin E receptor 4 signaling in cardiac reprogramming. Nat. Commun. 10:674. doi: 10.1038/s41467-019-08626-y
Nam, Y. J., Song, K., Luo, X., Daniel, E., Lambeth, K., West, K., et al. (2013). Reprogramming of human fibroblasts toward a cardiac fate. Proc. Natl. Acad. Sci. U. S. A. 110, 5588–5593. doi: 10.1073/pnas.1301019110
Nelakanti, R. V., and Xiao, A. Z. (2020). A new link to primate heart development. Dev. Cell 54, 685–686. doi: 10.1016/j.devcel.2020.09.009
Pei, J., Wang, F., Pei, S., Bai, R., Cong, X., Nie, Y., et al. (2020). Hydrogen sulfide promotes cardiomyocyte proliferation and heart regeneration via ROS scavenging. Oxidative Med. Cell. Longev. 2020:1412696. doi: 10.1155/2020/1412696
Ponnusamy, M., Liu, F., Zhang, Y. H., Li, R. B., Zhai, M., Liu, F., et al. (2019). Long noncoding RNA CPR (cardiomyocyte proliferation regulator) regulates cardiomyocyte proliferation and cardiac repair. Circulation 139, 2668–2684. doi: 10.1161/CIRCULATIONAHA.118.035832
Porrello, E. R., Mahmoud, A. I., Simpson, E., Hill, J. A., Richardson, J. A., Olson, E. N., et al. (2011). Transient regenerative potential of the neonatal mouse heart. Science 331, 1078–1080. doi: 10.1126/science.1200708
Porrello, E. R., Mahmoud, A. I., Simpson, E., Johnson, B. A., Grinsfelder, D., Canseco, D., et al. (2013). Regulation of neonatal and adult mammalian heart regeneration by the miR-15 family. Proc. Natl. Acad. Sci. U. S. A. 110, 187–192. doi: 10.1073/pnas.1208863110
Qian, L., Huang, Y., Spencer, C. I., Foley, A., Vedantham, V., Liu, L., et al. (2012). In vivo reprogramming of murine cardiac fibroblasts into induced cardiomyocytes. Nature 485, 593–598. doi: 10.1038/nature11044
Richardson, G. D., Breault, D., Horrocks, G., Cormack, S., Hole, N., and Owens, W. A. (2012). Telomerase expression in the mammalian heart. FASEB J. 26, 4832–4840. doi: 10.1096/fj.12-208843
Rinn, J. L., and Chang, H. Y. (2012). Genome regulation by long noncoding RNAs. Annu. Rev. Biochem. 81, 145–166. doi: 10.1146/annurev-biochem-051410-092902
Rubin, N., Darehzereshki, A., Bellusci, S., Kaartinen, V., and Ling Lien, C. (2013). FGF10 signaling enhances epicardial cell expansion during neonatal mouse heart repair. J. Cardiovasc. Dis. Diagn. 1:101. doi: 10.4172/2329-9517.1000101
Sadek, H. A., Martin, J. F., Takeuchi, J. K., Leor, J., Nie, Y., Giacca, M., et al. (2014). Multi-investigator letter on reproducibility of neonatal heart regeneration following apical resection. Stem Cell Rep. 3:1. doi: 10.1016/j.stemcr.2014.06.009
Sampaio-Pinto, V., Rodrigues, S. C., Laundos, T. L., Silva, E. D., Vasques-Novoa, F., Silva, A. C., et al. (2018). Neonatal apex resection triggers cardiomyocyte proliferation, neovascularization and functional recovery despite local fibrosis. Stem Cell Rep. 10, 860–874. doi: 10.1016/j.stemcr.2018.01.042
Santos, F., Moreira, C., Nobrega-Pereira, S., and Bernardes de Jesus, B. (2019). New insights into the role of epithelial(−)mesenchymal transition during aging. Int. J. Mol. Sci. 20:891. doi: 10.3390/ijms20040891
Sereti, K. I., Nguyen, N. B., Kamran, P., Zhao, P., Ranjbarvaziri, S., Park, S., et al. (2018). Analysis of cardiomyocyte clonal expansion during mouse heart development and injury. Nat. Commun. 9:754. doi: 10.1038/s41467-018-02891-z
Song, K., Nam, Y. J., Luo, X., Qi, X., Tan, W., Huang, G. N., et al. (2012). Heart repair by reprogramming non-myocytes with cardiac transcription factors. Nature 485, 599–604. doi: 10.1038/nature11139
Sousa-Franco, A., Rebelo, K., Da Rocha, S. T., and Bernardes de Jesus, B. (2019). LncRNAs regulating stemness in aging. Aging Cell 18:e12870. doi: 10.1111/acel.12870
Takahashi, K., Tanabe, K., Ohnuki, M., Narita, M., Ichisaka, T., Tomoda, K., et al. (2007). Induction of pluripotent stem cells from adult human fibroblasts by defined factors. Cell 131, 861–872. doi: 10.1016/j.cell.2007.11.019
Takahashi, K., and Yamanaka, S. (2006). Induction of pluripotent stem cells from mouse embryonic and adult fibroblast cultures by defined factors. Cell 126, 663–676. doi: 10.1016/j.cell.2006.07.024
Tani, H., Sadahiro, T., and Ieda, M. (2018). Direct cardiac reprogramming: a novel approach for heart regeneration. Int. J. Mol. Sci. 19:2629. doi: 10.3390/ijms19092629
Tao, G., Kahr, P. C., Morikawa, Y., Zhang, M., Rahmani, M., Heallen, T. R., et al. (2016). Pitx2 promotes heart repair by activating the antioxidant response after cardiac injury. Nature 534, 119–123. doi: 10.1038/nature17959
Trembinski, D. J., Bink, D. I., Theodorou, K., Sommer, J., Fischer, A., Van Bergen, A., et al. (2020). Aging-regulated anti-apoptotic long non-coding RNA Sarrah augments recovery from acute myocardial infarction. Nat. Commun. 11:2039. doi: 10.1038/s41467-020-15995-2
Trivedi, C. M., Zhu, W., Wang, Q., Jia, C., Kee, H. J., Li, L., et al. (2010). Hopx and Hdac2 interact to modulate Gata4 acetylation and embryonic cardiac myocyte proliferation. Dev. Cell 19, 450–459. doi: 10.1016/j.devcel.2010.08.012
Valiente-Alandi, I., Albo-Castellanos, C., Herrero, D., Sanchez, I., and Bernad, A. (2016). Bmi1+ cardiac progenitor cells contribute to myocardial repair following acute injury. Stem Cell Res. Ther. 7:100. doi: 10.1186/s13287-016-0355-7
von Gise, A., Lin, Z., Schlegelmilch, K., Honor, L. B., Pan, G. M., Buck, J. N., et al. (2012). YAP1, the nuclear target of Hippo signaling, stimulates heart growth through cardiomyocyte proliferation but not hypertrophy. Proc. Natl. Acad. Sci. U. S. A. 109, 2394–2399. doi: 10.1073/pnas.1116136109
Wada, R., Muraoka, N., Inagawa, K., Yamakawa, H., Miyamoto, K., Sadahiro, T., et al. (2013). Induction of human cardiomyocyte-like cells from fibroblasts by defined factors. Proc. Natl. Acad. Sci. U. S. A. 110, 12667–12672. doi: 10.1073/pnas.1304053110
Wang, J., Chen, X., Shen, D., Ge, D., Chen, J., Pei, J., et al. (2019a). A long noncoding RNA NR_045363 controls cardiomyocyte proliferation and cardiac repair. J. Mol. Cell. Cardiol. 127, 105–114. doi: 10.1016/j.yjmcc.2018.12.005
Wang, Z., Cui, M., Shah, A. M., Ye, W., Tan, W., Min, Y. L., et al. (2019b). Mechanistic basis of neonatal heart regeneration revealed by transcriptome and histone modification profiling. Proc. Natl. Acad. Sci. U. S. A. 116, 18455–18465. doi: 10.1073/pnas.1905824116
Wang, X., Yong, C., Yu, K., Yu, R., Zhang, R., Yu, L., et al. (2018). Long noncoding RNA (lncRNA) n379519 promotes cardiac fibrosis in post-infarct myocardium by targeting miR-30. Med. Sci. Monit. 24, 3958–3965. doi: 10.12659/MSM.910000
Wilson, K. D., Ameen, M., Guo, H., Abilez, O. J., Tian, L., Mumbach, M. R., et al. (2020). Endogenous retrovirus-derived lncRNA BANCR promotes cardiomyocyte migration in humans and non-human primates. Dev. Cell 54, 694–709.e699. doi: 10.1016/j.devcel.2020.07.006
Xiong, J., and Hou, J. (2016). Apical resection mouse model to study early mammalian heart regeneration. J. Vis. Exp. e53488. doi: 10.3791/53488
Yao, R. W., Wang, Y., and Chen, L. L. (2019). Cellular functions of long noncoding RNAs. Nat. Cell Biol. 21, 542–551. doi: 10.1038/s41556-019-0311-8
Yu, W., Huang, X., Tian, X., Zhang, H., He, L., Wang, Y., et al. (2016). GATA4 regulates Fgf16 to promote heart repair after injury. Development 143, 936–949. doi: 10.1242/dev.130971
Yusuf, S., Reddy, S., Ounpuu, S., and Anand, S. (2001). Global burden of cardiovascular diseases: part I: general considerations, the epidemiologic transition, risk factors, and impact of urbanization. Circulation 104, 2746–2753. doi: 10.1161/hc4601.099487
Zebrowski, D. C., Jensen, C. H., Becker, R., Ferrazzi, F., Baun, C., Hvidsten, S., et al. (2017). Cardiac injury of the newborn mammalian heart accelerates cardiomyocyte terminal differentiation. Sci. Rep. 7:8362. doi: 10.1038/s41598-017-08947-2
Zhang, S. Y., Huang, S. H., Gao, S. X., Wang, Y. B., Jin, P., and Lu, F. J. (2019a). Upregulation of lncRNA RMRP promotes the activation of cardiac fibroblasts by regulating miR-613. Mol. Med. Rep. 20, 3849–3857. doi: 10.3892/mmr.2019.10634
Zhang, Z., Zhang, A. D., Kim, L. J., and Nam, Y. J. (2019b). Ensuring expression of four core cardiogenic transcription factors enhances cardiac reprogramming. Sci. Rep. 9:6362. doi: 10.1038/s41598-019-42945-w
Zhang, Z., Zhang, W., and Nam, Y. J. (2019c). Stoichiometric optimization of Gata4, Hand2, Mef2c, and Tbx5 expression for contractile cardiomyocyte reprogramming. Sci. Rep. 9:14970. doi: 10.1038/s41598-019-51536-8
Zhu, B., Zhang, L., Liang, C., Liu, B., Pan, X., Wang, Y., et al. (2019). Stem cell-derived exosomes prevent aging-induced cardiac dysfunction through a novel exosome/lncRNA MALAT1/NF-kappaB/TNF-alpha signaling pathway. Oxidative Med. Cell. Longev. 2019:9739258. doi: 10.1155/2019/9739258
Keywords: lncRNAs, heart, regeneration, reprogramming, transdifferentiation
Citation: Santos F, Correia M, Nóbrega-Pereira S and Bernardes de Jesus B (2021) Age-Related Pathways in Cardiac Regeneration: A Role for lncRNAs? Front. Physiol. 11:583191. doi: 10.3389/fphys.2020.583191
Received: 14 July 2020; Accepted: 16 December 2020;
Published: 20 January 2021.
Edited by:
George Grant, University of Aberdeen, United KingdomReviewed by:
Meijing Wang, Indiana University Bloomington School of Medicine, United StatesCopyright © 2021 Santos, Correia, Nóbrega-Pereira and Bernardes de Jesus. This is an open-access article distributed under the terms of the Creative Commons Attribution License (CC BY). The use, distribution or reproduction in other forums is permitted, provided the original author(s) and the copyright owner(s) are credited and that the original publication in this journal is cited, in accordance with accepted academic practice. No use, distribution or reproduction is permitted which does not comply with these terms.
*Correspondence: Sandrina Nóbrega-Pereira, c2FuZHJpbmEucGVyZWlyYUB1YS5wdA==; Bruno Bernardes de Jesus, YnJ1bm9iLmplc3VzQHVhLnB0
†These authors have contributed equally to this work and share first authorship
Disclaimer: All claims expressed in this article are solely those of the authors and do not necessarily represent those of their affiliated organizations, or those of the publisher, the editors and the reviewers. Any product that may be evaluated in this article or claim that may be made by its manufacturer is not guaranteed or endorsed by the publisher.
Research integrity at Frontiers
Learn more about the work of our research integrity team to safeguard the quality of each article we publish.