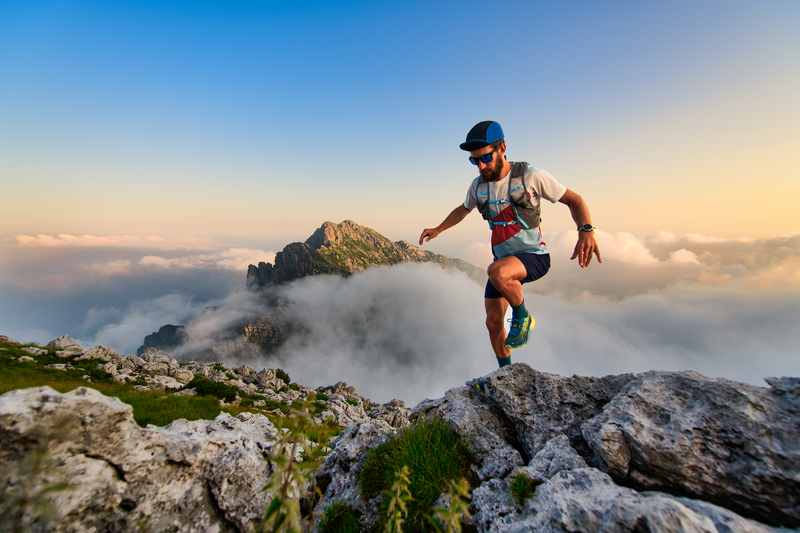
94% of researchers rate our articles as excellent or good
Learn more about the work of our research integrity team to safeguard the quality of each article we publish.
Find out more
OPINION article
Front. Physiol. , 20 November 2020
Sec. Exercise Physiology
Volume 11 - 2020 | https://doi.org/10.3389/fphys.2020.570472
Human responses to hypoxia (i.e., reduced O2 supply) range from immediate adjustments (minutes to hours) to prolonged adaptations (several weeks) within various physiological regulatory systems (Guillemin and Krasnow, 1997). Over the last 50 years, numerous altitude/hypoxic training modalities have been developed to capitalize on these hypoxic responses, with a view to improve athletic performance. Today, the use of hypoxia extends to therapeutic interventions (also known as “hypoxic conditioning”) (Millet et al., 2016b), an application dating back to the former Soviet union era (Serebrovskaya, 2002).
Traditional forms of altitude training include live high-train high, live high-train low, and live low-train high (LLTH) (Wilber, 2007). With the widespread availability of hypoxic chambers and portable hypoxicators, the LLTH paradigm has gained significant popularity over the last decade. This model involves exposure to hypoxia at rest or combined with exercise, while residing near sea level (Wilber, 2007; Girard et al., 2020). Altitude simulation (normobaric hypoxia) with the LLTH method is typically achieved by reducing the inspired oxygen fraction (FiO2), while atmospheric pressure remains unchanged. An example in professional sport, is repeated-sprint training sessions with multiple athletes, conducted at a fixed FiO2 of 0.145 to simulate an altitude equivalent to 3,000 m (Faiss et al., 2013).
Responses to hypoxia vary in magnitude between individuals (Friedmann et al., 2005; Chapman et al., 2011). For example, Friedmann et al. (2005) showed in 16 elite junior swimmers that the increase in erythropoietin concentration after 4 h in normobaric hypoxia (FiO2 0.15) averaged ~58%, but remarkably ranged from 10 to 185%. In this regard, alternative approaches to implementing hypoxia have been proposed (Bassovitch and Serebrovskaya, 2009; Mira et al., 2020). For instance, the “arterial oxygen saturation (SpO2) clamp” approach (Mira et al., 2020), whereby SpO2 is clamped to a target/range by altering the FiO2 presented to each individual has been proposed as a step toward reducing variability in the responses to hypoxia.
This paper first discusses the inter-individual variability in response to hypoxic stress when using “fixed FiO2” as a marker of “dose,” and then examines the “SpO2 clamp” as an alternate approach. We then consider the usefulness of a clinical index that integrates both the external (FiO2) and internal (SpO2) stimuli to characterize individual responses to hypoxia (Rice et al., 2007), and propose its application in exercise and sport science settings.
The fundamental variables that define the hypoxic “dose” include the severity, duration, frequency, type (normobaric or hypobaric hypoxia) and pattern of hypoxic presentation (Wilber et al., 2007; Navarrete-Opazo and Mitchell, 2014). An optimal “dose” should maximize chronic physiological benefits, whilst minimizing potential harmful consequences (e.g., headaches, dizziness). Currently, there are limited quantitative means to describe the optimal hypoxic “dose” required for planned physiological responses. Further, there is an incomplete understanding of the link between the immediate and chronic responses to hypoxia.
The environmental stress (e.g., elevation) has often been used as a predictor of the total physiological stress imposed on an individual. For example, Garvican-Lewis et al. (2016) introduced the metric termed “kilometer hours” to quantify the overall “external stress” during altitude sojourns based on the terrestrial/simulated altitude level and duration of exposure. One critique is that external load metrics does not consider the physiological stress or internal load imposed on an individual. In response, the “saturation hours” metric was suggested as a measure reflecting internal load (i.e., SpO2) which considers the duration at which a particular SpO2 is sustained during hypoxic exposure (Millet et al., 2016a).
A typical LLTH hypoxic training session entails a group of athletes exercising at a simulated altitude of 2,500–3,500 m (through manipulation of FiO2). Whilst it is tenable to expect that reduced ambient oxygen availability should decrease in vivo oxygenation, regulatory responses to hypoxia (e.g., increased ventilation) can influence events along the oxygen cascade to attenuate the decline in SpO2 (Richardson et al., 2006). Reductions in SpO2 at a fixed FiO2 vary widely due to differences in hypoxic chemosensitivity, pulmonary ventilatory limitation, hypoxic ventilatory response, arterial-venous shunting, ventilatory perfusion mismatch, and/or diffusion limitation (Weil, 2003; Chapman, 2013). Furthermore, determining an ideal hypoxic severity based on FiO2 per se is challenging since the hypoxic range falls on the steep portion of the oxyhemoglobin curve (Chapman, 2013). In other words, a small decline in partial pressure of oxygen (PO2) would result in a disproportionate SpO2 decrease. Remarkably, the variability in SpO2 response becomes more pronounced with increasing hypoxia severity. For instance, the SpO2 response of 15 healthy individuals decreased from 95–98% to 74–95% when FiO2 was lowered from 0.21 to 0.12 (Albert and Swenson, 2014). The heterogeneity in response to a given FiO2 may also result in disparity in exercise performance. For example, at an altitude of 2,100 m, elite athletes who demonstrated greater reductions in SpO2 also experienced larger declines in performance compared with athletes with smaller SpO2 fluctuations (Chapman et al., 2011). Collectively, the variability in SpO2 response at a given FiO2 suggests that some individuals may attain the planned hypoxia-induced response (i.e., those close to the average), whereas others may receive a stimulus either “too small” or “too large.” From a training perspective, a stimulus that is “too large” may inadvertently diminish beneficial gains (i.e., catabolic effect of hypoxia) from exercise training (Etheridge et al., 2011). Further, this variability in hypoxic response is reported within relatively homogenous groups (i.e., healthy and trained). It stands to reason that greater variability in hypoxic responses would be expected in clinical cohorts. This includes type 2 diabetes mellitus and chronic pulmonary obstructive disease, where varying degrees of mitochondrial dysfunction (Lowell and Shulman, 2005; Sangwung et al., 2020) and hypoxic ventilatory response (Weil, 2003) are evident, respectively. Considering the adoption of hypoxia training in clinical cohorts (Verges et al., 2015) along with the established variability in SpO2 responses to hypoxia in non-clinical cohorts, the use of FiO2 as a marker of “dose” requires reconsideration.
Support for the use of SpO2 in setting the hypoxic “dose” comes from research demonstrating that many hypoxia-induced outcomes (e.g., angiogenesis, neuromuscular adaptations) are ultimately governed by downstream events of the oxygen cascade (Ameln et al., 2005; Manimmanakorn et al., 2013). Consequently, these physiological outcomes occur in response to decreased arterial oxygen saturation, measured using SpO2, rather than FiO2 per se (Manimmanakorn et al., 2013). Indeed, elevated skeletal muscle adaptations (e.g., transcript expression of mitochondria biogenesis) to hypoxic training are proportional to the magnitude of SpO2 decrease (Schmutz et al., 2010). Methods of clamping SpO2 include prior oxygen titration to predetermine the optimal FiO2 (McKeown et al., 2019) and manual (Mira et al., 2020) or automatic adjustments (Ng et al., 2016; Bayer et al., 2017) (requiring a biofeedback mode) during the actual session. A possible concern of the “SpO2 clamp” approach—particularly when oxygen delivery is manually adjusted—is the accuracy of SpO2 responses. This is because SpO2 does not decrease proportionally with FiO2, due to the sigmoidal relationship between PO2 and SpO2. That said, studies which have attempted to clamp SpO2 to a specific target, or within a 3–10% range, report standard deviation values of <5% during both passive (Törpel et al., 2019) and active (Mira et al., 2020; Törpel et al., 2020) hypoxic exposure.
Oxygen therapy is routinely prescribed for patients with lung conditions (e.g., in severe COVID-19 cases) experiencing hypoxemia (Alhazzani et al., 2020). To mitigate risks associated with hypoxemia and hyperoxia-related lung injury, oxygen delivery is individually titrated within a tight range. The calculation of the pulmonary shunt fraction is the preferred clinical assessment of the oxygenating capacity of the lungs, although arterial oxygen partial pressure (PaO2) and SpO2 have been proposed as surrogate measurements of oxygenation (Zetterstrom, 1988). In order to assess the severity of hypoxemia in ventilated patients (where supplemental oxygen is used to maintain SpO2 within a normal/safe range) the PaO2 to FiO2 ratio, and later the SpO2 to FiO2 ratio (SF), were proposed (Horovitz et al., 1974; Rice et al., 2007). To illustrate, a healthy individual at sea level with a SpO2 of 98% would have a SF value of 467 (i.e., 98/0.21). Lower SF values are indicative of reduced oxygenating capacity, and is used, for instance, to diagnose patients with acute respiratory distress syndrome (SF values ≤ 235) and acute lung injury (SF values ≤ 315) (Rice et al., 2007). Unlike previous approaches, the SF ratio considers both the internal and external stimuli which allows for comparison between individuals/groups. Furthermore, the SF index is readily accessible and easy to interpret, which therefore represents an appealing tool for the early assessment of patients with potential respiratory disorders.
Moving beyond the conventional “fixed FiO2” approach, an individualized approach to administering hypoxia may consist of a combination of strategies such as (1) a prior hypoxia test to elucidate variability in responsiveness to hypoxia, (2) altering severity of hypoxia individually to regulate SpO2 within a tightly defined range, and (3) reporting the inter-individual variability based on the SF index.
A hypoxia test can be used to estimate the trajectory of SpO2 to hypoxia, and in turn, inform decisions on the hypoxic “dose.” Figure 1 depicts the hypothetical SpO2 responses of participants A, B, and C during a decremental titration using FiO2 of 0.17, 0.15, and 0.13. As illustrated, the corresponding responses form an abbreviated individual-specific oxyhemoglobin curve. In this example, with a lower SpO2 response to a given FiO2, participant C displays the highest response to hypoxia compared to participants A and B; this is represented by rightward and downward shifts of the abbreviated oxyhemoglobin curve. Participant C would likely require a higher FiO2 (i.e., milder hypoxia) to record similar SpO2 values as participants A and B. For instance, if the target SpO2 is 85%, the approximate FiO2 for participants A, B, and C would be 0.11 (SF: 85/0.11 = 773), 0.15 (85/0.15 = 567), and 0.16 (85/0.16 = 531), respectively. The corresponding SF values may then provide clarity on the inter-individual variability in response to hypoxia, wherein a low SF value indicates a high sensitivity to hypoxia.
Figure 1. Individual arterial oxygen saturation (SpO2) response of participants A, B, and C at fractional inspired oxygen (FiO2) of ~0.11. Hypothetical SpO2 response to a hypoxia test at FiO2 of 0.13, 0.15, and 0.17. Corresponding SpO2 to FiO2 ratio (SF) are presented at each data point.
Where a “fixed FiO2” approach is used to administer hypoxia, the SF index may also provide similar information about inter-individual variability. At a FiO2 of ~0.11, for instance, the SpO2 response of participants A, B, and C are 85, 78, and 71%, equating to SF values of 773, 709, and 645, respectively (Figure 1). By establishing threshold values for SF, distinct groups can be identified and clustered for training purposes, to increase likelihood of achieving similar physiological responses.
The appeal of the “fixed FiO2” approach, is the ease of implementation, for example, in an environmental chamber where a group of athletes can train together. Comparatively, whilst an individualized approach may produce a more consistent hypoxic response, such an approach would likely require personalized equipment and/or prior preparations (e.g., titration of “dose”). That said, an individualized approach to administering hypoxia would be applicable across the spectrum from clinical cohorts to elite-level athletes. However, it should be highlighted that the SF index is a measurement that does not consider the type of hypoxia exposure (i.e., hypobaric vs. normobaric). Since greater desaturation is associated with hypobaric than normobaric hypoxia for a matched inspired PO2 (Saugy et al., 2014), SF values may not be strictly equivalent between terrestrial and simulated hypoxia, and therefore should not be used interchangeably.
Traditionally, hypoxic training has adopted a universal approach, wherein all individuals receive the same absolute hypoxia stress (i.e., FiO2). Whilst highly practical, substantial inter-individual variability in response to a given FiO2 is indisputable. The implication being, that some individuals attain the appropriate hypoxia-related adaptations, whereas others may receive potentially harmful or ineffective stimuli. Similar to the individual tailoring of training variables, we suggest that the administration of hypoxia requires an individualized approach. We therefore propose that the SF index (i.e., SpO2 to FiO2 ratio)—which is already widely adopted in clinical settings—can also be used by exercise physiologists and sport scientists to gauge an individual's response to hypoxia. This may ultimately offer a more pragmatic approach toward defining physiologically distinct groups of individuals and enable a tailored level of FiO2.
All authors listed have made a substantial, direct and intellectual contribution to the work, and approved it for publication.
The authors declare that the research was conducted in the absence of any commercial or financial relationships that could be construed as a potential conflict of interest.
The handling editor declared a past co-authorship with the authors JS and OG.
Albert, T. J., and Swenson, E. R. (2014). Peripheral chemoreceptor responsiveness and hypoxic pulmonary vasoconstriction in humans. High Alt. Med. Biol. 15, 15–20. doi: 10.1089/ham.2013.1072
Alhazzani, W., Møller, M. H., Arabi, Y. M., Loeb, M., Gong, M. N., Fan, E., et al. (2020). Surviving sepsis campaign: guidelines on the management of critically ill adults with coronavirus disease 2019 (COVID-19). Intensive Care Med. 46, 854–887.
Ameln, H., Gustafsson, T., Sundberg, C. J., Okamoto, K., Jansson, E., Poellinger, L., et al. (2005). Physiological activation of hypoxia inducible factor-1 in human skeletal muscle. FASEB J. 19, 1009–1011. doi: 10.1096/fj.04-2304fje
Bassovitch, O., and Serebrovskaya, T. V., (eds.). (2009). “Equipment and regimes for intermittent hypoxia therapy,” in Intermittent Hypoxia: From Molecular Mechanisms to Clinical Applications (New York, NY: Nova Science Publishers), 561–572.
Bayer, U., Likar, R., Pinter, G., Stettner, H., Demschar, S., Trummer, B., et al. (2017). Intermittent hypoxic–hyperoxic training on cognitive performance in geriatric patients. Alzheimers Dement. 3, 114–122. doi: 10.1016/j.trci.2017.01.002
Chapman, R. F. (2013). The individual response to training and competition at altitude. Br. J. Sports Med. 47, i40–i44. doi: 10.1136/bjsports-2013-092837
Chapman, R. F., Stager, J. M., Tanner, D. A., Stray-Gundersen, J., and Levine, B. D. (2011). Impairment of 3000-m run time at altitude is influenced by arterial oxyhemoglobin saturation. Med. Sci. Sports Exerc. 43, 1649–1656. doi: 10.1249/MSS.0b013e318211bf45
Etheridge, T., Atherton, P. J., Wilkinson, D., Selby, A., Rankin, D., Webborn, N., et al. (2011). Effects of hypoxia on muscle protein synthesis and anabolic signaling at rest and in response to acute resistance exercise. Am. J. Physiol. Endocrinol. Metab. 301, E697–702. doi: 10.1152/ajpendo.00276.2011
Faiss, R., Girard, O., and Millet, G. P. (2013). Advancing hypoxic training in team sports: from intermittent hypoxic training to repeated sprint training in hypoxia. Br. J. Sports Med. 47(Suppl 1), i45–50. doi: 10.1136/bjsports-2013-092741
Friedmann, B., Frese, F., Menold, E., Kauper, F., Jost, J., and Bartsch, P. (2005). Individual variation in the erythropoietic response to altitude training in elite junior swimmers. Br. J. Sports Med. 39, 148–153. doi: 10.1136/bjsm.2003.011387
Garvican-Lewis, L. A., Sharpe, K., and Gore, C. J. (2016). Time for a new metric for hypoxic dose? J. Appl. Physiol. 121, 352–355. doi: 10.1152/japplphysiol.00579.2015
Girard, O., Brocherie, F., Goods, P. S. R., and Millet, G. P. (2020). An updated panorama of “living low-training high” altitude/hypoxic methods. Front. Sports Active Liv. 2:26. doi: 10.3389/fspor.2020.00026
Guillemin, K., and Krasnow, M. A. (1997). The hypoxic response: huffing and hifing. Cell 89, 9–12. doi: 10.1016/S0092-8674(00)80176-2
Horovitz, J. H., Carrico, C. J., and Shires, G. T. (1974). Pulmonary response to major injury. Arch. Surg. 108, 349–355. doi: 10.1001/archsurg.1974.01350270079014
Lowell, B. B., and Shulman, G. I. (2005). Mitochondrial dysfunction and type 2 diabetes. Science 307, 384–387. doi: 10.1126/science.1104343
Manimmanakorn, A., Manimmanakorn, N., Taylor, R., Draper, N., Billaut, F., Shearman, J. P., et al. (2013). Effects of resistance training combined with vascular occlusion or hypoxia on neuromuscular function in athletes. Eur. J. Appl. Physiol. 113, 1767–1774. doi: 10.1007/s00421-013-2605-z
McKeown, D. J., Simmonds, M. J., and Kavanagh, J. J. (2019). Reduced blood oxygen levels induce changes in low-threshold motor unit firing that align with the individual's tolerance to hypoxia. J. Neurophysiol. 121, 1664–1671. doi: 10.1152/jn.00071.2019
Millet, G. P., Brocherie, F., Girard, O., Wehrlin, J. P., Troesch, S., Hauser, A., et al. (2016a). Commentaries on Viewpoint: Time for a new metric for hypoxic dose? J. Appl. Physiol. 121:356. doi: 10.1152/japplphysiol.00460.2016
Millet, G. P., Debevec, T., Brocherie, F., Malatesta, D., and Girard, O. (2016b). Therapeutic use of exercising in hypoxia: promises and limitations. Front. Physiol. 7:224. doi: 10.3389/fphys.2016.00224
Mira, J., Floreani, M., Savoldelli, A., Amery, K., Koral, J., Oranchuk, D. J., et al. (2020). Neuromuscular fatigue of cycling exercise in hypoxia. Med. Sci. Sports Exerc. 52, 1888–1899. doi: 10.1249/MSS.0000000000002331
Navarrete-Opazo, A., and Mitchell, G. S. (2014). Therapeutic potential of intermittent hypoxia: a matter of dose. Am. J. Physiol. Regul. Integr. Comp. Physiol. 307, R1181–1197. doi: 10.1152/ajpregu.00208.2014
Ng, I., Fairchild, T., Greene, W., and Hoyne, G. (2016). Preferential mobilization and egress of type 1 and Type 3 innate lymphocytes in response to exercise and hypoxia. Immun. Res. 12:2. doi: 10.4172/1745-7580.10000123
Rice, T. W., Wheeler, A. P., Bernard, G. R., Hayden, D. L., Schoenfeld, D. A., Ware, L. B., et al. (2007). Comparison of the SpO2/FIO2 ratio and the PaO2/FIO2 ratio in patients with acute lung injury or ARDS. Chest 132, 410–417. doi: 10.1378/chest.07-0617
Richardson, R. S., Duteil, S., Wary, C., Wray, D. W., Hoff, J., and Carlier, P. G. (2006). Human skeletal muscle intracellular oxygenation: the impact of ambient oxygen availability. J. Physiol. 571, 415–424. doi: 10.1113/jphysiol.2005.102327
Sangwung, P., Petersen, K. F., Shulman, G. I., and Knowles, J. W. (2020). Mitochondrial dysfunction, insulin resistance, and potential genetic implications: potential role of alterations in mitochondrial function in the pathogenesis of insulin resistance and type 2 diabetes. Endocrinology 161:bqaa017. doi: 10.1210/endocr/bqaa017
Saugy, J. J., Schmitt, L., Cejuela, R., Faiss, R., Hauser, A., Wehrlin, J. P., et al. (2014). Comparison of “live high-train low” in normobaric versus hypobaric hypoxia. PLoS ONE 9:e114418. doi: 10.1371/journal.pone.0114418
Schmutz, S., Däpp, C., Wittwer, M., Durieux, A. C., Mueller, M., Weinstein, F., et al. (2010). A hypoxia complement differentiates the muscle response to endurance exercise. Exp. Physiol. 95, 723–735. doi: 10.1113/expphysiol.2009.051029
Serebrovskaya, T. V. (2002). Intermittent hypoxia research in the former soviet union and the commonwealth of independent states: history and review of the concept and selected applications. High Alt. Med. Biol. 3, 205–221. doi: 10.1089/15270290260131939
Törpel, A., Peter, B., Hamacher, D., and Schega, L. (2019). Dose–response relationship of intermittent normobaric hypoxia to stimulate erythropoietin in the context of health promotion in young and old people. Eur. J. Appl. Physiol. 119, 1065–1074. doi: 10.1007/s00421-019-04096-8
Törpel, A., Peter, B., and Schega, L. (2020). Effect of resistance training under normobaric hypoxia on physical performance, hematological parameters, and body composition in young and older people. Front. Physiol. 11:335. doi: 10.3389/fphys.2020.00335
Verges, S., Chacaroun, S., Godin-Ribuot, D., and Baillieul, S. (2015). Hypoxic conditioning as a new therapeutic modality. Front. Pediatr. 3:58. doi: 10.3389/fped.2015.00058
Weil, J. (2003). Variation in human ventilatory control—genetic influence on the hypoxic ventilatory response. Respir. Physiol. Neurobiol. 135, 239–246. doi: 10.1016/S1569-9048(03)00048-X
Wilber, R. L. (2007). Application of altitude/hypoxic training by elite athletes. Med. Sci. Sports Exerc. 39, 1610–1624. doi: 10.1249/mss.0b013e3180de49e6
Wilber, R. L., Stray-Gundersen, J., and Levine, B. D. (2007). Effect of hypoxic “dose" on physiological responses and sea-level performance. Med. Sci. Sports Exerc. 39, 1590–1599. doi: 10.1249/mss.0b013e3180de49bd
Keywords: hypoxia, hypoxemia, simulated altitude, hypoxic training, oxygen saturation
Citation: Soo J, Girard O, Ihsan M and Fairchild T (2020) The Use of the SpO2 to FiO2 Ratio to Individualize the Hypoxic Dose in Sport Science, Exercise, and Health Settings. Front. Physiol. 11:570472. doi: 10.3389/fphys.2020.570472
Received: 08 June 2020; Accepted: 09 October 2020;
Published: 20 November 2020.
Edited by:
François Billaut, Laval University, CanadaReviewed by:
Martin Burtscher, University of Innsbruck, AustriaCopyright © 2020 Soo, Girard, Ihsan and Fairchild. This is an open-access article distributed under the terms of the Creative Commons Attribution License (CC BY). The use, distribution or reproduction in other forums is permitted, provided the original author(s) and the copyright owner(s) are credited and that the original publication in this journal is cited, in accordance with accepted academic practice. No use, distribution or reproduction is permitted which does not comply with these terms.
*Correspondence: Timothy Fairchild, dC5mYWlyY2hpbGRAbXVyZG9jaC5lZHUuYXU=
Disclaimer: All claims expressed in this article are solely those of the authors and do not necessarily represent those of their affiliated organizations, or those of the publisher, the editors and the reviewers. Any product that may be evaluated in this article or claim that may be made by its manufacturer is not guaranteed or endorsed by the publisher.
Research integrity at Frontiers
Learn more about the work of our research integrity team to safeguard the quality of each article we publish.