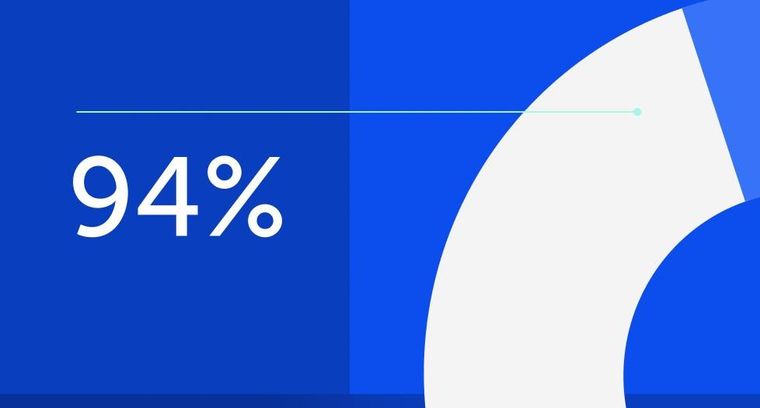
94% of researchers rate our articles as excellent or good
Learn more about the work of our research integrity team to safeguard the quality of each article we publish.
Find out more
ORIGINAL RESEARCH article
Front. Physiol., 22 October 2020
Sec. Aquatic Physiology
Volume 11 - 2020 | https://doi.org/10.3389/fphys.2020.559348
Neuropeptides are endogenous active substances that are present in nervous tissues and participate in behavioral and physiological processes of the animal system. Locomotor behavior is basic to predation, escape, reproduction in animals, and neuropeptides play an important role in locomotion. In this study, the function of pedal peptide-type neuropeptide (PDP) in the process of locomotor behavior of the sea cucumber Apostichopus japonicus was evaluated. The locomotor behavior of A. japonicus was recorded by infrared camera before and after PDP administration, and muscle physiology was studied by ultra performance liquid chromatography and quadrupole time-off-light mass spectrometry (UPLC-Q-TOF-MS) to clarify the potential physiological mechanisms. The results showed that PDP enhanced the cumulative duration of moving significantly at the 7th h after injection, and reduced the mean and maximum velocity by 16.90 and 14.22% in A. japonicus. The data of muscle metabolomics suggested that some significantly changed metabolites were related to locomotor behavior of sea cucumbers. The decreases of phosphatidylethanolamine (PE) and phosphatidylcholine (PC) might result in the increases of lysophosphatidylcholines (lysoPC) and lysophosphatidylethanolamine (lysoPE), and suggested the change of fluidity and permeability in the muscle cell membrane, which would affect the physiology and function of muscle cells, and finally alter the locomotor behavior. In addition, the increased level of arachidonic acid (ARA) might activate K+ ion channels and then affect the signaling of muscle cells, or promote the sensitivity of muscle cells to Ca2+ and then result in the contractility of longitudinal muscles in sea cucumbers. ARA was also involved in the linoleic acid metabolism which was the only pathway that disturbed significantly after PDP administration. In conclusion, PDP participated in the regulation of locomotor behavior in the sea cucumber, and the decreased PE and PC, increased lysoPC, lysoPE and ARA might be the potential physiological mechanisms that responsible for behavioral effects of PDP in A. japonicus.
Neuropeptides generally refer to endogenous active substances that are present in nervous tissues and participate in the functions of animal nervous system. They are characterized by low contents, high activities, extensive functions and complex mechanisms. In evolution, neuropeptides are ancient neuronal signaling molecules that play a key role in the regulation of various physiological processes (homeostasis, energy metabolism) and behaviors (locomotion, feeding, and reproduction). Locomotor behavior is basic to predation, escape, habitat dominance, reproduction in animals, and a number of studies were focused on the functions and mechanisms of neuropeptides in locomotion (He et al., 2013; Pauls et al., 2019). Although the locomotor behavior of A. japonicus was studied thoroughly before (Pan et al., 2015), the mechanisms underlying locomotor behavior in this echinoderm species are still unclear. With the decoding of whole-genome and the application of transcriptomics and proteomics in A. japonicus, the neuropeptides in A. japonicus were recently identified (Chen et al., 2019), which provide the foundation to study the functions of neuropeptides in locomotor behavior in this species.
Pedal neuropeptides and orcokinin-type (PP/OK) neuropeptides are two structurally related neuropeptides that belong to the family of bilateral symmetric animal neuropeptides (Rowe and Elphick, 2012; Jekely, 2013). These two neuropeptides have been identified in many animals, including protostomia (such as nematodes and annelids) and deuterostome (such as echinoderms). Pedal peptide (PP) was originally found in the mollusk sea hare Aplysia californica and was predominately synthesized in the pedal ganglia of this species (Lloyd and Connolly, 1989). In particular, PP could cause pedal muscle contraction (Hall and Lloyd, 1990) and foot-related ciliary oscillation (Longley and Peterman, 2013), which indicated that it might play a role in the locomotor behavior of sea hares. In addition, orcokinin (OK) neuropeptides were firstly isolated from the crayfish Orconectes limosus nerve extract (Stangier et al., 1992), and OK-type neuropeptides could influence the circadian activity rhythm in Leucophaea maderae (Hofer and Homberg, 2006; Soehler et al., 2011; Wei and Stengl, 2011).
In recent years, studies on the PP/OK neuropeptides in echinoderms have been carried out widely. The echinoderm PP/OK neuropeptide was first discovered by analyzing the transcriptome data of the sea urchin Strongylocentrotus purpuratus (Rowe and Elphick, 2012). Subsequently, it was also demonstrated to be present in the sea cucumber (Rowe et al., 2014). Besides, a kind of muscle relaxant (SMP) was proved to be a PP/OK neuropeptide in the starfish Patina pectinifera (Kim et al., 2016). Five neuropeptides (ArPPLN1a-e) were identified in the SMP precursor (PP-type neuropeptide precursor 1; ArPPLNP1) of the starfish Asterias rubens, and ArPPLNP1 and neuropeptides in this precursor were widely expressed in sacral nerve cord, nerve ring, digestive system (such as cardiac stomach), body wall muscle and appendages (such as tube feet and spines) (Lin et al., 2017). This study showed that PP neuropeptides were present in the lateral motor nerves and the nerves that innervate the internal muscles, and caused relaxation of the body wall muscles, tube feet and cardiac stomach in this species (Lin et al., 2017). In addition, the distribution of the second PP/OK neuropeptide (ArPPLNP2) is extremely broad in the tissues of the starfish, and it can efficiently cause relaxation of the cardiac stomach (Lin et al., 2018).
Metabolomics is an important tool in systems biology research, which can detect the concentration of endogenous small molecules in tissues and shows the changes of metabolites’ concentrations in organisms under specific physiological conditions (Nicholson et al., 1999; Sun et al., 2017). The techniques of metabolomics include high performance liquid chromatography (HPLC) (Onchoi et al., 2008), gas chromatography-mass spectrometry (GC-MS) (Plumb et al., 2003), liquid chromatography-mass spectrometry (LC- MS) (Luo et al., 2007) and Nuclear Magnetic Resonance (NMR) (Robertson et al., 2000; Ji et al., 2013). Among them, ultra performance liquid chromatography and quadrupole time-off-light mass spectrometry (UPL-Q-TOF-MS) have the features of high resolution and high sensitivity, which can detect changes in differential metabolites in biological fluids or tissues quickly and effectively (Wilson et al., 2005; O’Connor and Mortishire-Smith, 2006). At present, this technology has been successfully applied to evaluate the metabolic physiology of sea cucumber muscle in breeding and non-propagation stages (Ru et al., 2017), and to study the effects of melatonin on muscle physiology in sea cucumbers (Ding et al., 2019).
The sea cucumber A. japonicus is the most important commercial species in echinoderms, and it is widely distributed along the coasts of north-west Pacific Ocean (35°N – 44°N) (Yang et al., 2015). To date, this species is cultured extensively in China. According to the China Fishery Statistical Yearbook 2020, the total marine aquaculture area and yield of sea cucumbers reached 246,745 ha and 171,700 tons in 2019. In present study, A. japonicus was used as a model system to study the function of PDP in the process of locomotor behavior. A pedal peptide-type neuropeptide (C-terminal serine is amidated, as determined from mass spec data.) from the Ajnp7 precursor protein (Rowe and Elphick, 2012; Chen et al., 2019) was synthesized artificially by biological techniques. The EthoVision XT software was used to analysis the changes of locomotor behavior in A. japonicus after PDP administration. In addition, the key metabolites and pathways are identified by UPL-Q-TOF-MS metabolomics to clarify the potential mechanisms underlying the effect of PDP on locomotor behavior.
The PDP sequence was derived from the PP/OK neuropeptide precursor protein obtained by Rowe et al. (2014) by analyzing the transcriptome data of the sea cucumber. The C-terminal serine amidation of the FGSSQIMDPLRYSLVS sequence was finally determined by mass spec data (Chen et al., 2019). Pedal peptide-type neuropeptide was synthesized by GL Biochem (Shanghai) Peptide Ltd., using peptide solid phase syntheses, and the molecular formula is C80H127N21O24S, molecular mass is 1790.09 g/mol and purity is 99.80%. The product of PDP was stored at −20°C until use.
The sea cucumbers were collected from the outdoor aquaculture pond in Zhuwang Port, Laizhou, Yantai (37°15.656′N, 119°53.985′E). After taking sea cucumbers back to the Qingdao laboratory, they were placed in a tank that was prepared in advance. The tank had a capacity of 1,500L, and was filled with filtered seawater and oxygenated by an air pump (dissolved oxygen >6.0 mg/L), the water temperature was maintained at 15 ± 0.5°C, the salinity was 30 ppt, and the pH was 8.0. Sea cucumbers were fed by the self-made diet (70% sea mud and 30% Sargassum powder), and food debris and feces were removed every morning, then half of the water was replaced once a day at 8 o’clock. The holding time lasts for 2 weeks.
Twenty-four healthy sea cucumbers were selected from the holding tank for behavioral experiments. They were randomly divided into 2 groups (98.4 ± 16.2g, n = 12 sea cucumber/group), one of them was set as PDP group, and sea cucumbers in this group were injected with 0.1% (v/w) 10–5 M PDP solution into their coeloms (the PDP was dissolved in 1xPBS solution and diluted to 10–5 M by sterilized sea water) (Kato et al., 2009). The other one was the CON group, and sea cucumbers were injected with an equal amount of 1xPBS solution. The locomotor behavior of each individual sea cucumber was recorded separately in one glass tank (50 × 50 × 50 cm), and 24 identical tanks were used in this study. A white acrylic plate was placed at the bottom of the glass tank to facilitate the movement tracking with EthoVision XT (version 10.1) software (Noldus Inc., Netherlands). The water level in the glass tank was about 30 cm. An infrared camera (Hikvision DS-2CD3310D-I, 4MM, Hangzhou) was fixed by a plastic bracket above each tank. In order to ensure the uniformity of the experimental conditions, the experiment was carried out under full dark conditions. Before the experiment, the individual sea cucumber was placed in each tank for 24 h to familiarize the experimental environment (Ding et al., 2019). After that, all sea cucumbers in the tank were recorded for 3 h; then, twelve of them were injected with PDP solution, others were administrated by the 1xPBS solution. The injections were performed under dim red light with a 1 mL ICO syringe (JIANSHI®, Luohe, China) and a 0.3 mm microneedle (Pinillos et al., 2001).
The Hikvision infrared camera was used to record the locomotor behavior of each sea cucumber for 12 h (3 h before injection, 9 h after injection). The videos of locomotor behavior were analyzed by EthoVision software to quantify the locomotor behavior, and the behavioral indicators, including total distance traveled, cumulative duration of movement, mean and maximum velocity, were obtained. Continuous alternation of body contraction and relaxation makes the sea cucumber move ahead (Pan et al., 2015). Therefore, we defined one contraction and relaxation of body as one step, and the moving distance of one step is the stride length. The number of movement steps was also counted, and average stride length, stride frequency, and stride velocity were calculated in this study.
One-way analysis of variance and Tukey’s post hoc multiple comparison tests (SPSS 20.0 software) were used to analyze the data of sea cucumber locomotor behavior. A probability level of p < 0.05 was considered to be statistically significant.
The behavioral data indicating that the time point of the significant difference of locomotor behavior between the treatment group and control group was the 7th h after PDP injection. 48 healthy sea cucumbers were selected from the holding water tanks and randomly divided into 2 groups (n = 24). The control group (CON) and the administrated group (PDP) were treated in the same way of behavioral study, respectively. About 2 g of longitudinal muscle tissue was sheared off from each sea cucumber at the 7th h after administration, and was washed with ultrapure water, dried with absorbent paper, placed in a sterile tube and stored in a refrigerator at −80°C.
Every 3 samples in both groups were combined in 1 tube for metabolomic detection. UPLC-Q-TOF-MS method was used in this study, and the detailed steps and statistical analysis were the same as previous research (Ding et al., 2019).
In Figure 1, the results of EthoVision software analysis showed the total moving distance, cumulative duration of moving, average and maximum velocity from CON and PDP groups. The distances of sea cucumbers moved per hour ranged from 115.05 to 176.84 cm in the CON group and 120.57 to 188.31 cm in the PDP group, and they increased to some extent after PDP administration, but were not statistically significant (Figure 1A; F = 7.235, p > 0.05). The average total distance moved per hour after injection was 12.50 ± 1.29 m in the CON group and 14.05 ± 1.85 m in the PDP group. However, the cumulative duration of moving within each hour increased in the PDP group, and the significant difference between CON and PDP groups occurred at 7 h after injection (Figure 1B; F = 10.364, p < 0.05). The hourly duration of moving was between 17.81 and 27.15 min in the CON group, while in the PDP group, it was between 23.32 and 36.35 min (Figure 1B). The total cumulative duration of moving after injection was 205.30 ± 19.35 min (CON group) and 278.67 ± 24.63 min (PDP group). Moreover, the mean and maximum velocities of the PDP group were 16.90 and 14.22% lower than that of the CON group, but they were not statistically significant (Figures 1C,D; p > 0.05).
Figure 1. Total distance traveled per hour (A), cumulative duration of movement per hour (B), and mean and maximum velocity (C,D) for A. japonicus in the control (CON) and pedal peptide-type neuropeptide injected (PDP) groups. Each symbol or bar and vertical line represents the mean ± SEM (N = 12, p < 0.05).
Figure 2 shows results of other measures of locomotor performance, including the number of steps moving, average stride, mean duration of each step and step velocity in each hour. The average number of steps moving within each hour ranged from 24.83 to 36.67 in the control and 29.08 to 45.08 in the treatment, and almost all of them in the PDP group were higher than that in the CON group, despite none of them were statistically significant (Figure 2A; F = 7.869, p > 0.05). The number of total steps taken after injection was 281.58 ± 23.49 in the CON group and 343.67 ± 26.58 in the PDP group. In addition, the average stride in the PDP group decreased to some extent, but it was no statistically significant, neither the mean duration of steps taken and stride frequency (Figures 2B–D).
Figure 2. Average total number of steps taken per hour (A), stride (B), stride frequency (C), and stride velocity (D) of A. japonicus in the control (CON) and pedal peptide-type neuropeptide injected (PDP) groups. Each vertical line represents the mean ± SEM (N = 12, p < 0.05).
PLS-DA and OPLS-DA were used to identify the metabolic alterations of muscle tissues between CON and PDP groups. In the PLS-DA plot, the abscissa represents the first principal component PC1 (t[1]), and the ordinate represents the second principal component PC2 (t[2]) (Figure 3A). While in the OPLS-DA plot, the abscissa represents the predictive principal component, and the ordinate represents the orthogonal principal component (Figure 3B). Each spot in the figure represents one sample. Both plots reveal that the CON (green spot) group and PDP (red spot) group are clearly separated from each other. Besides, Figure 4 shows the heat map of overall differential metabolites from CON and PDP groups. Each transverse line represents a differential metabolite and each cross represents a muscle sample. Different colors represent different higher abundance intensity (mean value acquired from all detected samples of the same group). The correlation analysis of overall differential metabolites from CON and PDP groups is shown in Figure 5. The color of each dot represents the Pearson’s correlation coefficient of two differential metabolites. Red for positive correlation and blue for negative correlation.
Figure 3. The PLS-DA (A) and OPLS-DA (B) scores plot of muscle metabolites from the control (CON) and pedal peptide-type neuropeptide injected (PDP) groups. The abscissa and ordinate represent the first principal component (PC1) and the second principal component (PC2), respectively.
Figure 4. The heat maps of overall differential metabolites from the control (CON) and pedal peptide-type neuropeptide injected (PDP) groups. Each line represents a differential metabolite and each cross represents a muscle sample. Different colors represent different higher abundance intensity (mean value acquired from all detected samples of the same group).
Figure 5. The correlation analysis of overall differential metabolites from the control (CON) and pedal peptide-type neuropeptide injected (PDP) groups. The color of each line represents the Pearson correlation coefficient of two differential metabolites. Red for positive correlation and blue for negative correlation.
Combining t-test (p < 0.05) and OPLS-DA model (VIP > 1.0) results, 31 key differential metabolites between CON and PDP groups were identified, and 15 of them were positive ion patterns and 16 of them were negative ion patterns (Table 1). Twenty-two metabolites such as piperidine, phosphatidylethanolamine (PE), phosphatidylcholine (PC), neoporrigenin B, and L-3-Aminodihydro-2(3H)-furanone decreased significantly, and 9 metabolites such as lysophosphatidylethanolamine (LysoPE), lysophosphatidylcholine (LysoPC), carindone, and arachidonic acid increased significantly in the PDP group. Correlation analysis of 50 differential metabolites illustrated that the correlation between LysoPC and PC were negative, as well as LysoPE and PE (Figure 5). Metabolic pathway enrichment analysis indicated that these differential metabolites were involved linoleic acid metabolism, drug metabolism – other enzymes, galactose metabolism, biosynthesis of unsaturated fatty acids and arachidonic acid metabolism, in which the linoleic acid metabolism pathway was disturbed significantly after PDP administration (Figure 6, p < 0.05). The dataset of metabolomics in this study was uploaded in figshare (https://doi.org/10.6084/m9.figshare.12436949.v1).
Table 1. Muscle metabolites with concentrations that differed significantly between the control (CON) and pedal peptide-type neuropeptide injected (PDP) groups, including the ion mode [positive (pos) or negative (neg)], mass (compound molecular weight), RT [retention time (min)], VIP (variable importance in the projection), FC (fold change, PDP/CON) and p value of these metabolites.
Figure 6. The KEGG pathway enrichment of overall differential metabolites from the control (CON) and pedal peptide-type neuropeptide injected (PDP) groups. Horizontal axis for enriched pathway; vertical axis for the significance level of pathway enrichment. Above the red and blue dashed lines represents p < 0.01 and p < 0.05.
The results of this study indicated that PDP was involved in the regulation of locomotor behavior of the sea cucumber A. japonicus, especially the locomotor endurance. To date, the effects of neuropeptides on animal locomotor behavior have been widely reported in mice, rats, locusts, fruit flies, sea hares, nematodes and other organisms (Pañeda et al., 2009; Kahsai et al., 2010; Yang et al., 2016; Hou et al., 2017; Pauls et al., 2019). Both Nocll neuropeptide and neuropeptide S (NPS) can stimulate the locomotor behavior in mice, and NPS induce this effect through corticotropin-releasing factor receptor 1 (Florin et al., 1997; Pañeda et al., 2009). Besides, neuropeptide F (NPF, including NPF1a, and NPF2) can suppress locomotor behavior, and NPS/nitric oxide pathway is essential for the plasticity of locomotor behavior during phase transition in swarming locust (Hou et al., 2017). GdFFD neuropeptide can significantly reduce the locomotor activity and induce a foot curl in the marine mollusc Aplysia californica (Yang et al., 2016). In drosophila, neuropeptide Drosophila tachykinin (DTK) participates in the regulation of spatial orientation, and the deficiency of DTK resulted in the decrease of locomotor behavior, while short neuropeptide F (sNPF) takes part in the fine regulation of locomotor performance (Kahsai et al., 2010). Sensory neurons trigger locomotor behavior by secreting neuropeptide (PDF-1) and glutamate, while neuropeptide (FLP-2) induces locomotor activity via an orexin-like receptor (FRPR-18) in the nematode Caenorhabditis elegans (Chen et al., 2016). Although the effects of different neuropeptides on locomotor behavior were widely studied in various animals, few studies focused on the pedal neuropeptide. Pedal neuropeptide can stimulate the feet muscle of Aplysia to increase the amplitude and relaxation rate of contractions driven by neuronal or intracellular stimulation of pedal motor neurons, and pedal neurons have the function of modulating foot muscle contractility during locomotor behavior in Aplysia californica (Hall and Lloyd, 1990). The results of this study indicated that the stride of sea cucumbers decreased to some extent after PDP injection, indicating that PDP might participate in the regulation of muscle contraction during locomotor activity. In addition, the distance moved, number of steps taken and cumulative duration of moving were increased after PDP administration, while the cumulative duration of moving in each hour was significantly higher in PDP group at the 7th h, indicating that PDP can enhance the locomotor endurance of sea cucumbers. The decrease of mean velocity, maximum velocity and mean step velocity, and the increase of average duration of moving, indicated that PDP may reduce the efficiency of locomotor activity in the sea cucumber to some extent.
In conclusion, PDP participated in the regulation of locomotor behavior in the sea cucumber A. japonicus, and more precisely, it could enhance the endurance of locomotion. This finding would provide evidence for the effect of PDP on the locomotor behavior of sea cucumbers.
In this study, the time point at which the significant differentiation of locomotor performance occurred between control and PDP administrated A. japonicus (the 7th h after injection) was selected to measure the changes of metabolite levels in the muscle tissues of these two groups. Our results revealed that the muscle metabolite profiles in sea cucumbers after PDP treatment were different from the control, and one metabolic pathway was disturbed significantly. PE and PC levels were significantly decreased in the muscle tissues of the PDP group, while the levels of LysoPE, LysoPC and ARA increased significantly. In addition, ARA was involved in the pathway of linoleic acid metabolism according to KEGG. These metabolites and linoleic acid metabolic pathways are likely to be potential mechanisms that underlying the effect of PDP on locomotor endurance and efficiency in A. japonicus.
In muscles, the composition of different phospholipids and phosphatidylglycerols types is closely related to cell membrane fluidity, lipid rafts, membrane protein dynamics and insulin receptor dynamics (Pilch et al., 1980; Nadiv et al., 1994; Gorski et al., 1999; Saha et al., 2016). Phosphatidylethanolamine (PE) and phosphatidylcholine (PC) are the major phospholipids in cell membrane, and PE accounts for 20–30% of the total phospholipid pool, while PC accounts for about 0.5% (Takagi, 1971). Knocking out PC and PE-related specific enzymes in model animal resulted in the decrease of PE synthesis, increase of PC:PE value, reduced skeletal muscle, declined activity of endoplasmic reticulum/sarcoplasmic reticulum (ER/SR) Ca2+ ATPase (SERCA), and finally decreased locomotor performance (Funai et al., 2013; Selathurai et al., 2015; Funai et al., 2016). Therefore, skeletal muscle growth, locomotor performance and glucose metabolism are likely to be related with the value of PC: PE. Phospholipid composition is biologically important for the functions that related to mitochondria, cell growth, muscle contraction, locomotor performance, and insulin sensitivity in skeletal muscle (Heden et al., 2016). In addition, acute and long-term physical exercise can reduce the value of PC:PE in human skeletal muscle, and mitochondrial function is involved in the potential molecular correlation between PC: PE ratio and insulin sensitivity in skeletal muscle (Lee et al., 2018). Thus, PC: PE value play a critical role in metabolism and insulin sensitivity of skeletal muscle (Wilson et al., 1981; Stuart et al., 1988; Goodyear and Kahn, 1998). The results of this study showed that phosphatidylethanolamine (PE) and phosphatidylcholine (PC) were decreased significantly in the muscle of sea cucumbers after PDP administration, although the change of PC: PE ratio was not clear according to our results, both PE and PC were degraded in the muscle cell membrane of sea cucumbers, and the fluidity and permeability of the cell membrane were changed, which will affect the physiology and function of muscle cells. This shift plays an important role in regulating animal behavioral plasticity (Wu et al., 2012). Considering that phospholipid composition is crucial for the transformation of muscle contraction and locomotor behavior in animals, and the PDP participates in the regulation of muscle contractility during the locomotion of animal (Hall and Lloyd, 1990), the decreases of phosphatidylethanolamine (PE) and phosphatidylcholine (PC) were likely to be a potential physiological mechanism that underlying the effect of PDPs on locomotor performance in A. japonicus.
Both lysophosphatidylethanolamine (LysoPE) and lysophosphatidylcholine (LysoPC) are part of lysophospholipids, and lysophosphatidylcholine (LysoPC) is also used as a biomarker for the diagnosis of diabetes (Oresic et al., 2008). Muscle metabolite profiles of sea cucumbers during reproductive period indicated that oxidative damage caused by elevated LysoPC was a potential mechanism for the decreased locomotor endurance of sea cucumbers (Ru et al., 2017). Although the level of lysophosphatidylcholine (LysoPC) was increased after PDP administration, the LysoPE was also up-regulated simultaneously, which is different from the previous results of muscle metabolite profiles of reproductive sea cucumbers. Therefore, the increases of both LysoPC and LysoPE were likely to be responsible for the increased locomotor endurance of A. japonicus after PDP treatment. LysoPC and LysoPE were converted from PE and PC by phospholipase A2; thus, the decreases of PE and PC might be the reasons for the increases of LysoPC and LysoPE in this study.
Arachidonic acid is a kind of polyunsaturated ω-6 fatty acid, which acts as precursor for many bioactive lipid mediators, and plays an important role in muscle anabolism. Few studies were focused on ARA in echinoderms. The composition of lipids and fatty acids in egg and body wall of sea urchin Diadema savignyi revealed that ARA accounts for the highest proportion (>50%) in the polyunsaturated fatty acids (Kim et al., 2018). ARA is likely to be an important component in the cells of echinoderm. In the process of muscle recovery after acute training in humans, the intake of ARA may enhance muscle adaptability (Mitchell et al., 2018). In addition, ARA can be oxidatively metabolized by cytochrome P450 epoxidase, and transformed into four regioisomeric epoxy eicosatrienoic acids (5,6-; 8,9-; 11,12-; 14,15-EET). They have the function of vasodilation, and cytochrome P450 metabolites of ARA can activate K+ ion channels of vascular smooth muscle (Hu and Kim, 1993). Besides, ARA and other fatty acids can directly activate K+ ion channels in smooth muscle cells (Ordway et al., 1989). It is well known that K+ ion channels are closely related to cell signal transduction. Besides, ARA can inhibit the activity of myosin phosphatase, which makes smooth muscle to be more sensitive to Ca2+ (Gong et al., 1992), and the release of Ca2+ will activate smooth muscle for contraction. The increase of ARA in the PDP administrated group might activate K+ ion channels in muscle cells, thereby affecting the signaling of muscle cells. At the same time, the increased level of ARA was likely to promote the sensitivity of muscle cells to Ca2+, and resulted in the contractility of longitudinal muscles in sea cucumbers. Thus, the elevated ARA in muscle tissues might be the potential physiological mechanism for the function of PDP in muscle contraction during locomotor behavior of A. japonicus.
This study showed that pedal peptide-type neuropeptide was involved in the regulation of locomotor behavior in A. japonicus. The prolonged duration of moving after PDP administration indicated that PDP enhanced the endurance of locomotion. The results of muscle metabolomic revealed that the decrease of PE and PC levels, and the increase of LysoPC, LysoPE, and ARA levels in muscle tissues after PDP treatment were the potential mechanisms that underlying the effects of PDP on locomotor behavior in A. japonicus.
The raw data supporting the conclusions of this article will be made available by the authors, without undue reservation.
KD designed and performed the research. LZ contributed new reagents and analytic tools. XF, XG, XL, and KD analyzed the data of locomotor behavior. KD wrote the manuscript. HY supervised the research. All authors contributed to the article and approved the submitted version.
This work was supported by the National Natural Science Foundation of China (41876157 and 41676136), the Key Deployment Project of Centre for Ocean Mega-Research of Science, Chinese Academy of Sciences (COMS2019Q15), the STS Program of Chinese Academy of Sciences (KFJ-STS-ZDTP-077), and the Taishan Scholars Program (Distinguished Taishan Scholars).
The authors declare that the research was conducted in the absence of any commercial or financial relationships that could be construed as a potential conflict of interest.
We thank Prof. Maurice Elphick and Prof. Muyan Chen for providing the sequence and structure of pedal peptide-type neuropeptide in this manuscript.
Chen, D., Taylor, K. P., Hall, Q., and Kaplan, J. M. (2016). The neuropeptides FLP-2 and PDF-1 act in concert to arouse Caenorhabditis elegans locomotion. Genetics 204, 1151–1159. doi: 10.1534/genetics.116.192898
Chen, M., Talarovicova, A., Zheng, Y., Storey, K. B., and Elphick, M. R. (2019). Neuropeptide precursors and neuropeptides in the sea cucumber Apostichopus japonicus: a genomic, transcriptomic and proteomic analysis. Sci. Rep. 9:8829.
Ding, K., Zhang, L. B., Zhang, T., Yang, H. S., and Brinkman, R. (2019). The effect of melatonin on locomotor behavior and muscle physiology in the sea cucumber Apostichopus japonicus. Front. Physiol. 10:221. doi: 10.3389/fphys.2019.00221
Florin, S., Suaudeau, C., Meunier, J., and Costentin, J. (1997). Orphan neuropeptide NocII, a putative pronociceptin maturation product, stimulates locomotion in mice. Neuroreport 8, 705–707. doi: 10.1097/00001756-199702100-00025
Funai, K., Lodhi, I. J., Spears, L. D., Yin, L., Song, H., Klein, S., et al. (2016). Skeletal muscle phospholipid metabolism regulates insulin sensitivity and contractile function. Diabetes 65, 358–370. doi: 10.2337/db15-0659
Funai, K., Song, H., Yin, L., Lodhi, I. J., Wei, X., Yoshino, J., et al. (2013). Muscle lipogenesis balances insulin sensitivity and strength through calcium signaling. J. Clin. Invest. 123, 1229–1240. doi: 10.1172/jci65726
Gong, M. C., Fuglsang, A., Alessi, D., Kobayashi, S., Cohen, P., Somlyo, A. V., et al. (1992). Arachidonic acid inhibits myosin light chain phosphatase and sensitizes smooth muscle to calcium. J. Biol. Chem. 267, 21492–21498.
Goodyear, L. J., and Kahn, B. B. (1998). Exercise, glucose transport, and insulin sensitivity. Annu. Rev. Med. 49, 235–261. doi: 10.1146/annurev.med.49.1.235
Gorski, J., Zendzian-Piotrowska, M., de Jong, Y. F., Niklinska, W., and Glatz, J. F. (1999). Effect of endurance training on the phospholipidcontent of skeletal muscles in the rat. Eur. J. Appl. Physiol. 79, 421–425. doi: 10.1007/s004210050532
Hall, J. D., and Lloyd, P. E. (1990). Involvement of pedal peptide in locomotion in Aplysia: modulation of foot muscle contractions. J. Neurobiol. 21, 858–868. doi: 10.1002/neu.480210604
He, C., Cong, X., Zhang, R., Wu, D., An, C., and Zhao, Z. (2013). Regulation of circadian locomotor rhythm by neuropeptide Y-like system in Drosophila melanogaster. Insect Mol. Biol. 22, 376–388. doi: 10.1111/imb.12027
Heden, T. D., Neufer, P. D., and Funai, K. (2016). Looking beyond structure: membrane phospholipids of skeletal muscle mitochondria. Trends Endocrinol. Metab. Trends Endocrin. Met. 27, 553–562. doi: 10.1016/j.tem.2016.05.007
Hofer, S., and Homberg, U. (2006). Evidence for a role of orcokinin-related peptides in the circadian clock controlling locomotor activity of the cockroach Leucophaea maderae. J. Exp. Biol. 209, 2794–2803. doi: 10.1242/jeb.02307
Hou, L., Yang, P., Jiang, F., Liu, Q., Wang, X., and Kang, L. (2017). The neuropeptide F/nitric oxide pathway is essential for shaping locomotor plasticity underlying locust phase transition. eLife 6:e22526.
Hu, S., and Kim, H. S. (1993). Activation of K+ channel in vascular smooth muscles by cytochrome P450 metabolites of arachidonic acid. Eur. J. Pharmacol. 230, 215–221. doi: 10.1016/0014-2999(93)90805-r
Jekely, G. (2013). Global view of the evolution and diversity of metazoan neuropeptide signaling. Proc. Natl. Acad. Sci. U.S.A. 110, 8702–8707. doi: 10.1073/pnas.1221833110
Ji, C., Wu, H., Wei, L., Zhao, J., Wang, Q., and Lu, H. (2013). Responses of Mytilus galloprovincialis to bacterial challenges by metabolomics and proteomics. Fish Shellfish Immunol. 35, 489–498. doi: 10.1016/j.fsi.2013.05.009
Kahsai, L., Martin, J. R., and Winther, A. M. E. (2010). Neuropeptides in the Drosophila central complex in modulation of locomotor behavior. J. Exp. Biol. 213, 2256–2265. doi: 10.1242/jeb.043190
Kato, S., Tsurumaru, S., Taga, M., Yamane, T., Shibata, Y., Ohno, K., et al. (2009). Neuronal peptides induce oocyte maturation and gamete spawning of sea cucumber, Apostichopus japonicus. Dev. Biol. 326, 169–176. doi: 10.1016/j.ydbio.2008.11.003
Kim, C. H., Kim, E. J., Go, H. J., Oh, H. Y., Lin, M., Elphick, M. R., et al. (2016). Identification of a novel starfish neuropeptide that acts as a muscle relaxant. J. Neurochem. 137, 33–45. doi: 10.1111/jnc.13543
Kim, H. D. T., Quoc, L. P., Nguyen, P. H., Lan, P. D., and Dinh, T. (2018). Research on the component of lipid classes, fatty acid from egg and body of sea urchin Diadema savignyi (Audouin, 1809). J. Pharmacogn. Phytochem. 7, 836–840.
Lee, S., Norheim, F., Gulseth, H. L., Langleite, T. M., Aker, A., Gundersen, T. E., et al. (2018). Skeletal muscle phosphatidylcholine and phosphatidylethanolamine respond to exercise and influence insulin sensitivity in men. Sci. Rep. 8:7885.
Lin, M., Egertova, M., Zampronio, C. G., Jones, A. M., and Elphick, M. R. (2017). Pedal peptide/orcokinin-type neuropeptide signaling in a deuterostome: the anatomy and pharmacology of starfish myorelaxant peptide in Asterias rubens. J. Comp. Neurol. 525, 3890–3917. doi: 10.1002/cne.24309
Lin, M., Egertová, M., Zampronio, C. G., Jones, A. M., and Elphick, M. R. (2018). Functional characterization of a second pedal peptide/orcokinin-type neuropeptide signaling system in the starfish Asterias rubens. J. Comp. Neurol. 526, 858–876. doi: 10.1002/cne.24371
Lloyd, P. E., and Connolly, C. M. (1989). Sequence of pedal peptide: a novel neuropeptide from the central nervous system of Aplysia. J. Neurosci. 9, 312–317. doi: 10.1523/jneurosci.09-01-00312.1989
Longley, R. D., and Peterman, M. (2013). Neuronal control of pedal sole cilia in the pond snail Lymnaea stagnalis appressa. J. Comp. Physiol. A 199, 71–86. doi: 10.1007/s00359-012-0770-x
Luo, B., Groenke, K., Takors, R., Wandrey, C., and Oldiges, M. (2007). Simultaneous determination of multiple intracellular metabolites in glycolysis, pentose phosphate pathway and tricarboxylic acid cycle by liquid chromatography-mass spectrometry. J. Chromatogr. A 1147, 153–164. doi: 10.1016/j.chroma.2007.02.034
Mitchell, C. J., D’Souza, R. F., Figueiredo, V. C., Chan, A., Aasen, K., Durainayagam, B., et al. (2018). The effect of dietary arachidonic acid supplementation on acute muscle adaptive responses to resistance exercise in trained men: a randomized controlled trial. J. Appl. Physiol. 124, 1080–1091. doi: 10.1152/japplphysiol.01100.2017
Nadiv, O., Shinitzky, M., Manu, H., Hecht, D., Roberts, C. T., LeRoith, D., et al. (1994). Elevated protein tyrosine phosphatase activity and increased membrane viscosity are associated with impaired activation of the insulin receptor kinase in old rats. Biochem. J. 298(Pt 2), 443–450. doi: 10.1042/bj2980443
Nicholson, J. K., Lindon, J. C., and Holmes, E. (1999). ‘Metabonomics’: understanding the metabolic responses of living systems to pathophysiological stimuli via multivariate statistical analysis of biological NMR spectroscopic data. Xenobiotica 29, 1181–1189. doi: 10.1080/004982599238047
O’Connor, D., and Mortishire-Smith, R. (2006). High-throughput bioanalysis with simultaneous acquisition of metabolic route data using ultra performance liquid chromatography coupled with time-of-flight mass spectrometry. Anal. Bioanal. Chem. 385, 114–121. doi: 10.1007/s00216-006-0353-1
Onchoi, C., Kongtip, P., Yoosook, W., and Chantanakul, S. (2008). High performance liquid charomatography for determination of urinary metabolites of toluene, xylene and styrene and its application. Southeast Asian J. Trop. Med. Public Health 39, 1164–1171.
Ordway, R. W., Walsh, J. V., and Singer, J. J. (1989). Arachidonic acid and other fatty acids directly activate potassium channels in smooth muscle cells. Science 244, 1176–1179. doi: 10.1126/science.2471269
Oresic, M., Simell, S., Sysi-Aho, M., Näntö-Salonen, K., Seppänen-Laakso, T., Parikka, V., et al. (2008). Dysregulation of lipid and amino acid metabolism precedes islet autoimmunity in children who later progress to type 1 diabetes. J. Exp. Med. 205, 2975–2984. doi: 10.1084/jem.20081800
Pan, Y., Zhang, L., Lin, C., Sun, J. M., Kan, R. T., and Yang, H. S. (2015). Influence of flow velocity on motor behavior of sea cucumber Apostichopus japonicus. Physiol. Behav. 144, 52–59. doi: 10.1016/j.physbeh.2015.02.046
Pañeda, C., Huitron-Resendiz, S., Frago, L. M., Chowen, J. A., Picetti, R., de Lecea, L., et al. (2009). Neuropeptide S reinstates cocaine-seeking behavior and increases locomotor activity through corticotropin-releasing factor receptor 1 in mice. J. Neurosci. 29, 4155–4161. doi: 10.1523/jneurosci.5256-08.2009
Pauls, D., Hamarat, Y., Trufasu, L., Schendzielorz, T. M., Gramlich, G., Kahnt, J., et al. (2019). Drosophila carboxypeptidase D (SILVER) is a key enzyme in neuropeptide processing required to maintain locomotor activity levels and survival rate. 50, 3502–3519. doi: 10.1111/ejn.14516
Pilch, P. F., Tompson, P. A., and Czech, M. P. (1980). Coordinate modulation of D-glucose transport activity and bilayer fuidity in plasmamembranes derived from control and insulin-treated adipocytes. Proc. Natl. Acad. Sci. U.S.A. 77, 915–918. doi: 10.1073/pnas.77.2.915
Pinillos, M. L., Pedro, N. D., Alonso-Gómez, A. L., Alonso-Bedate, M., and Delgado, M. J. (2001). Food intake inhibition by melatonin in goldfish (Carassius auratus). Physiol. Behav. 72, 629–634. doi: 10.1016/s0031-9384(00)00399-1
Plumb, R. S., Stumpf, C. L., Granger, J. H., Castro-Perez, J. M., Haselden, J. N., and Dear, G. J. (2003). Use of liquid chromatography/time-of-flight mass spectrometry and multivariate statistical analysis shows promise for the detection of drug metabolites in biological fluids. Rapid Commun. Mass Spectrom 17, 2632–2638. doi: 10.1002/rcm.1250
Robertson, D. G., Reily, M. D., Sigler, R. E., Wells, D. F., Paterson, D. A., and Braden, T. K. (2000). Metabonomics: evaluation of nuclear magnetic resonance (NMR) and pattern recognition technology for rapid in vivo screening of liver and kidney toxicants. Toxicol. Sci. 57, 326–337. doi: 10.1093/toxsci/57.2.326
Rowe, M. L., Achhala, S., and Elphick, M. R. (2014). Neuropeptides and polypeptide hormones in echinoderms: new insights from analysis of the transcriptome of the sea cucumber Apostichopus japonicus. Gen. Comp. Endocr. 197, 43–55. doi: 10.1016/j.ygcen.2013.12.002
Rowe, M. L., and Elphick, M. R. (2012). The neuropeptide transcriptome of a model echinoderm, the sea urchin Strongylocentrotus purpuratus. Gen. Comp. Endocr. 179, 331–344. doi: 10.1016/j.ygcen.2012.09.009
Ru, X., Zhang, L., Liu, S., and Yang, H. (2017). Reproduction affects locomotor behaviour and muscle physiology in the sea cucumber, Apostichopus japonicus. Anim. Behav. 133, 223–228. doi: 10.1016/j.anbehav.2017.09.024
Saha, S., Anilkumar, A. A., and Mayor, S. (2016). GPI-anchored protein organization and dynamics at the cell surface. J. Lipid Res. 57, 159–175. doi: 10.1194/jlr.r062885
Selathurai, A., Kowalski, G. M., Burch, M. L., Sepulveda, P. V., Risis, S., Lee-Young, R. S., et al. (2015). The CDP-ethanolamine pathway regulates skeletal muscle diacylglycerol content and mitochondrial biogenesis without altering insulin sensitivity. Cell Metab. 21, 718–730. doi: 10.1016/j.cmet.2015.04.001
Soehler, S., Stengl, M., and Reischig, T. (2011). Circadian pacemaker coupling by multi-peptidergic neurons in the cockroach Leucophaea maderae. Cell Tissue Res. 343, 559–577. doi: 10.1007/s00441-010-1091-4
Stangier, J., Hilbich, C., Burdzik, S., and Keller, R. (1992). Orcokinin: a novel myotropic peptide from the nervous system of the crayfish, Orconectes limosus. Peptides 13, 859–864. doi: 10.1016/0196-9781(92)90041-z
Stuart, C. A., Shangraw, R. E., Prince, M. J., Peters, E. J., and Wolfe, R. R. (1988). Bed-rest-induced insulin resistance occurs primarily in muscle. Metabolism 37, 802–806. doi: 10.1016/0026-0495(88)90018-2
Sun, L., Sun, J., Xu, Q., Li, X., Zhang, L., and Yang, H. (2017). Metabolic responses to intestine regeneration in sea cucumbers Apostichopus japonicus. Comp. Biochem. Phys. D 22, 32–38. doi: 10.1016/j.cbd.2017.02.003
Takagi, A. (1971). Lipid composition of sarcoplasmic reticulum of human skeletal muscle. Biochim. Biophys. Acta 248, 12–20.
Wei, H., and Stengl, M. (2011). Light affects the branching pattern of peptidergic circadian pacemaker neurons in the brain of the cockroach Leucophaea maderae. J. Biol. Rhythm 26, 507–517. doi: 10.1177/0748730411419968
Wilson, I. D., Nicholson, J. K., Castro-Perez, J., Granger, J. H., Johnson, K. A., Smith, B. W., et al. (2005). High resolution “ultra performance” liquid chromatography coupled to oa-TOF mass spectrometry as a tool for differential metabolic pathway profiling in functional genomic studies. J. Proteome Res. 4, 591–598. doi: 10.1021/pr049769r
Wilson, P. W., McGee, D. L., and Kannel, W. B. (1981). Obesity, very low density lipoproteins, and glucose intolerance over fourteen years: Te Framingham Study. Am. J. Epidemiol. 114, 697–704. doi: 10.1093/oxfordjournals.aje.a113240
Wu, R., Wu, Z., Wang, X., Yang, P., Yu, D., Zhao, C., et al. (2012). Metabolomic analysis reveals that carnitines are key regulatory metabolites in phase transition of the locusts. Proc. Natl. Acad. Sci. U.S.A. 109, 3259–3263. doi: 10.1073/pnas.1119155109
Yang, C. Y., Yu, K., Wang, Y., Chen, S.-A., Liu, D.-D., Wang, Z.-Y., et al. (2016). Aplysia locomotion: Network and behavioral actions of GdFFD, a D-amino acid-containing neuropeptide. PLoS one 11:e0147335. doi: 10.1371/journal.pone.0147335
Keywords: echinoderm, locomotor performance, muscle metabolite, pedal peptide, neuropeptide
Citation: Ding K, Zhang L, Fan X, Guo X, Liu X and Yang H (2020) The Effect of Pedal Peptide-Type Neuropeptide on Locomotor Behavior and Muscle Physiology in the Sea Cucumber Apostichopus japonicus. Front. Physiol. 11:559348. doi: 10.3389/fphys.2020.559348
Received: 06 May 2020; Accepted: 25 September 2020;
Published: 22 October 2020.
Edited by:
Enric Gisbert, Institute of Agrifood Research and Technology (IRTA), SpainReviewed by:
Yun-wei Dong, Xiamen University, ChinaCopyright © 2020 Ding, Zhang, Fan, Guo, Liu and Yang. This is an open-access article distributed under the terms of the Creative Commons Attribution License (CC BY). The use, distribution or reproduction in other forums is permitted, provided the original author(s) and the copyright owner(s) are credited and that the original publication in this journal is cited, in accordance with accepted academic practice. No use, distribution or reproduction is permitted which does not comply with these terms.
*Correspondence: Libin Zhang, emhhbmdsaWJpbkBxZGlvLmFjLmNu
Disclaimer: All claims expressed in this article are solely those of the authors and do not necessarily represent those of their affiliated organizations, or those of the publisher, the editors and the reviewers. Any product that may be evaluated in this article or claim that may be made by its manufacturer is not guaranteed or endorsed by the publisher.
Research integrity at Frontiers
Learn more about the work of our research integrity team to safeguard the quality of each article we publish.