- 1Beijing Key Laboratory for Forest Pest Control, Beijing Forestry University, Beijing, China
- 2Research Institute of Forestry New Technology, Chinese Academy of Forestry, Beijing, China
In China, the wood-boring weevil Eucryptorrhynchus scrobiculatus damages and eventually kills the tree of heaven Ailanthus altissima. To feed and digest the cell wall of A. altissima, E. scrobiculatus requires plant cell wall-degrading enzymes (PCWDEs). In the present study, we used next-generation sequencing to analyze the midgut transcriptome of E. scrobiculatus. Using three midgut transcriptomes, we assembled 21,491 unigenes from 167,714,100 clean reads. We identified 25 putative PCWDEs, including 11 cellulases and 14 pectinases. We constructed phylogenetic trees with a maximum likelihood algorithm to elucidate the relationships between sequences of the PCWDE protein families and speculate the functions of the PCWDE genes in E. scrobiculatus. The expression patterns of 17 enzymes in the midgut transcriptome were analyzed in various tissues by quantitative real-time PCR (RT-qPCR). The relative expression levels of 12 genes in the midgut and two genes in the proboscis were significantly higher than those in the other tissues. The proboscis and midgut are the digestive organs of insects, and the high expression level indirectly indicates that these genes are related to digestion. The present study has enabled us to understand the types and numbers of the PCWDEs of E. scrobiculatus and will be helpful for research regarding other weevils’ PCWDEs in the future.
Introduction
Plant cell walls are the most abundant and useful source of biomass on Earth. Coleoptera insects can spread into most habitats worldwide and have become the most species-rich insect order on Earth owing to their mostly herbivorous nature (digesting plant cell walls as nutrients) (Pauchet et al., 2010; Schuman and Baldwin, 2016; Antony et al., 2017). Herbivorous insects are thought to rely on gut microorganisms to digest plant cell walls until the endogenous cellulase genes from termites are identified (Watanabe et al., 1998; Calderón-Cortés et al., 2012). These endogenous insect genes that degrade plant cell walls are called plant cell wall degrading enzymes (PCWDEs). Studies have shown that herbivorous insects obtain a variety of PCWDE families through horizontal gene transfer (HGT) and then use plant cell walls more efficiently through gene replication and functional diversification (Calderón-Cortés et al., 2012; Kirsch et al., 2014, 2016; Busch et al., 2019). Since endogenous PCWDEs are thought to play important roles in the evolution of plant–insect interactions and insect diversification (Calderón-Cortés et al., 2012), a large number of reports on endogenous PCWDEs in herbivorous insects have been published in recent years (Watanabe and Tokuda, 2010; Pauchet et al., 2014; Kirsch et al., 2016; Antony et al., 2017). The advent of next-generation sequencing methods has enabled the identification of a growing number of endogenous PCWDEs in beetles, such as Rhynchophorus ferrugineus, Apriona japonica, and Phaedon cochleariae (Roy et al., 2012; Pauchet et al., 2014; Antony et al., 2017).
The degradation of plant cell walls by phytophagous insects is the primary requirement for utilizing host plants (Antony et al., 2017). Plant cell wall polysaccharides mainly consist of cellulose as well as hemicellulose and pectin (Cosgrove, 2005), and PCWDEs include cellulase, hemicellulase, and pectinase (Juge, 2006). Different hydrolase families of PCWDEs have been identified in many insect species (Watanabe et al., 1998; Calderón-Cortés et al., 2012), and these digestive enzymes play key roles in insect feeding diversity (Pauchet et al., 2010; Watanabe and Tokuda, 2010; Antony et al., 2017). Previous reports indicated that insect PCWDEs include three carbohydrate-active enzymes (CAZymes), namely glycoside hydrolase (GH), carbohydrate esterase (CE), and polysaccharide lyase (PL) (Cantarel et al., 2009; Pauchet et al., 2010; Watanabe and Tokuda, 2010; Calderón-Cortés et al., 2012). Endogenous insect cellulases include GH5, GH9, GH45, and GH48 (Pauchet et al., 2010; Watanabe and Tokuda, 2010; Calderón-Cortés et al., 2012). In addition to the GH48 family, cellulase activity has been detected in other families (Calderón-Cortés et al., 2012; Kirsch et al., 2014, 2016; Busch et al., 2018, 2019). However, only GH48 in insects is confirmed to have chitinase rather than cellulase activity (Fujita et al., 2006). The most common hemicellulases are xylanase and xyloglucanase (Calderón-Cortés et al., 2012). Only two endogenous genes encoding xylanases were found in Phaedon cochleariae, and functions have been verified (Girard and Jouanin, 1999; Calderón-Cortés et al., 2012; Pauchet and Heckel, 2013). Studies have shown that insects use the pectinases GH28 (polygalacturonase), CE8 (pectin methylesterase), and PL4 (rhamnogalacturonan lyase) to degrade the cell wall pectin network (Pauchet et al., 2010; Calderón-Cortés et al., 2012; Kirsch et al., 2016). The GH28 gene is ubiquitous in insects including the Curculionoidea. Weevils have both CE8 and GH28 genes (Pauchet et al., 2010; Calderón-Cortés et al., 2012; Kirsch et al., 2016). The functions of many CE8 and GH28 genes have been verified (Kirsch et al., 2014, 2016). The GH28 genes exhibit other enzyme activities in addition to pectinase activity, and some GH28 genes for which enzyme activities have not been detected may be pseudogenes (Kirsch et al., 2014). The PL4 genes have only been identified in weevils, such as Dendroctonus ponderosae and Hypothenemus hampei, and the function of these genes has not been identified (Pauchet et al., 2010; Keeling et al., 2013; Vega et al., 2015).
The weevil Eucryptorrhynchus scrobiculatus Motschulsky (Coleoptera: Curculionidae) is an important pest of the tree of heaven (Ailanthus altissima Swingle). It has an extensive geographical distribution across China (Yu et al., 2013; Liu and Wen, 2016; Yang et al., 2017) and can, therefore, cause serious damage (Ji et al., 2017a). The entire E. scrobiculatus life cycle occurs on the tree of heaven. The larvae and adults feed on the plant tissues and sap, generally feeding on weak and mechanically injured trees rather than healthy ones. As such, it is a secondary pest of A. altissima (McAvoy et al., 2013). E. scrobiculatus adults attack the trees while the larvae simultaneously attack the roots. If unchecked, this weevil may eventually kill its host (Ding et al., 2006). The adult insect is very harmful to A. altissima (Ji et al., 2017b), so the cellulase activity of the gut extract was tested (Chen, 2016). Chen’s study shows that the cellulase activity of the male E. scrobiculatus gut extract is significantly higher than that of female E. scrobiculatus gut extract (Chen, 2016). In the present study, we identified PCWDEs in E. scrobiculatus midgut by high-throughput sequencing and investigated their expression patterns. PCWDEs that have previously been functionally verified in insects were used to predict their function in E. scrobiculatus. Given the intrinsic nature of the wood boring weevil, our results are fundamental for studying the molecular mechanism of the digestion of plant cell walls in E. scrobiculatus. PCWDEs have the potential for pest management and biofuels.
Materials and Methods
Insect Rearing and Tissue Collection
Adult E. scrobiculatus were collected in Wutongshu Town, Pingluo County, Ningxia Hui Autonomous Region, China (38°16′86″N, 106°29′71″E) in June 2017. The insects were reared in a breathable plastic box (50 × 40 × 40 cm) containing perennial A. altissima branches. The plastic box was delivered to the Beijing Key Laboratory for Forest Pest Control in Beijing, China. There, the insects were frozen in liquid nitrogen, surface-sterilized with 75% (v/v) alcohol, and rinsed with sterile water. Dissection was performed by using an anatomical mirror (Leica, SE6) on ice on a clean bench. Various tissues (Head, leg, proboscis, foregut, midgut, and hindgut) were stored separately at -80°C. The midgut (the intestinal section between the cardiac valve and the base of the Malpighian tubule) was excised for RNA extraction.
Total RNA Extraction
Five midguts were used per RNA extraction. These were performed as three independent biological replicates with a RNeasy Plus kit (Qiagen, Hilden, Germany) following the manufacturer’s instructions. The total RNA was treated with DNase. RNA was quantitated with a Bioanalyzer 2100 (Agilent Technologies, Santa Clara, CA, United States) and monitored with a NanoDrop 8000 (Thermo Fisher Scientific, Waltham, MA, United States). Only high-quality RNA samples were used to construct the sequencing library, with optical density OD260//280 = 1.8 − 2.2, OD260/230 ≥ 2.0, RIN ≥ 6.5 and, 28S ribosomal RNA:18S ribosomal RNA ≥ 1.0. The RIN indicates the integrity of the RNA sample and is in the range of 1–10. RNA integrity increases with RIN.
Library Construction and Illumina Sequencing
Three RNA-seq transcriptome libraries were prepared using a TruSeqTM RNA sample preparation kit from Illumina (San Diego, CA, United States) and 5 μg RNA. Messenger RNA was isolated with oligo (dT) beads by the polyA selection method and fragmented in fragmentation buffer. Double-stranded cDNA was synthesized with a SuperScript double-stranded cDNA synthesis kit (Invitrogen, Carlsbad, CA, United States) and random hexamer primers (Illumina, San Diego, CA, United States). The cDNA was subjected to end-repair, phosphorylation, and ‘A’ base addition according to the Illumina library construction protocol. The libraries were assembled by selecting 200 − 300 bp cDNA fragments on a 2% low-range ultra-agarose gel, followed by PCR amplification with Phusion DNA polymerase (New England Biolabs (NEB), Ipswich, MA, United States) for 15 cycles. After quantitation with a TBS380 fluorometer (Turner BioSystems, Sunnyvale, CA, United States), the paired-end RNA-seq library was sequenced with an Illumina HiSeq 4000 (Illumina, San Diego, CA, United States). Read lengths of 150 bp and 6.6 Gb were targeted for sequencing. There were three biological replicates per experiment.
Assembly and Functional Annotation
Raw paired-end reads were trimmed and subjected to quality control with SeqPrep1 and Sickle2 using their default parameters. Clean reads were assembled with Trinity3. A 200-bp minimum contig length and k = 25 were used for the assembly. Normalization was performed in silico. The largest alternative splicing variant in the Trinity output for each unigene was selected as a unigene. The unigenes were searched against the NCBI non-redundant (NR) protein database using an e-value cutoff of 10–5. The open reading frame (ORF) of each unigene was determined with the NCBI ORF Finder tool4. The BLAST2GO program was used to obtain GO annotations for the unigenes and identify putative biological processes, molecular functions, and cellular components. Transcript expression levels were calculated by the fragments per kilobase of exon per million mapped reads (FRKM) method (Li and Dewey, 2011). The final FPKM is the average FPKM of three biological replicates.
Identification of Endogenous PCWDEs
All candidate GH9, GH28, GH45, GH48, PL4, and CE8 enzymes were manually checked with the NCBI BLASTx tool (Altschul et al., 1990). Unigenes potentially encoding the PCWDEs were confirmed and categorized into glycoside hydrolase (GH) families with HmmSearch (Zhang et al., 2018). If genes have incomplete open reading frames in the transcriptome, the complete open reading frames were obtained with a SMARTer RACE 5’/3’ Kit (TaKaRa Bio Inc., Kusatsu, Shiga, Japan) according to the manufacturer’s instructions.
Phylogenetic Analysis of E. scrobiculatus PCWDEs
The sequence homology and evolutionarily conserved features of Eucryptorrhynchus scrobiculatus PCWDEs were analyzed by reconstructing phylogenetic trees with E. scrobiculatus protein sequences and homologous sequences from beetles and other insects. Closely related sequences from other insects were obtained from National Center for Biotechnology Information (NCBI) for each gene subfamily. A reference sequence whose structure was already elucidated was retrieved from the CAZy database. Multiple sequence alignment was performed with ClustalW (Price et al., 2010). Phylogenetic trees were constructed by the maximum-likelihood method in IQ-TREE (Minh et al., 2020). Phylogenetic trees were color-coded and arranged using FigTree v1.4.4 and TBtools v1.046 (Chen et al., 2020).
Tissue Expression Analysis of E. scrobiculatus PCWDEs
The expression profiles of PCWDE genes that were identified in the midgut transcriptome and whose FPKM was greater than 10 in various tissues (head, leg, proboscis, foregut, midgut, and hindgut) were analyzed by RT-PCR and RT-qPCR. Simultaneously, the only GH9 gene was also subjected to these analyses. The total RNA was processed as previously described. The first-strand cDNA was synthesized from the total RNA with a PrimeScript RT reagent kit and gDNA Eraser (TaKaRa Bio Inc., Kusatsu, Shiga, Japan) according to the manufacturer’s instructions. Two hundred nanograms cDNA was used for the RT-PCR and RT-qPCR templates. The RT-PCR specific primers were designed with PRIMER3 (Untergasser et al., 2012) using the following parameters: melting temperature (Tm) = 60°C; GC content = 40% − 60%; and product size = 100 − 200 bp. The primer sequences are listed in Supplementary Table S3. The PCR was performed using PrimeSTAR Max DNA Polymerase (TaKaRa Bio Inc., Kusatsu, Shiga, Japan) with an initial denaturation step at 98°C for 1 min followed by 35 cycles at 98°C for 20 s, 60°C for 15 s, 72°C for 1 min, and a final extension of 5 min at 72°C. The PCR products were electrophoretically separated on 2% agarose gels and verified by DNA sequencing.
The RT-qPCR analysis was conducted with an Applied Biosystems StepOnePlusTM (Thermo Fisher Scientific, Waltham, MA, United States) in 20-μL reaction volumes consisting of 10 μL SYBR Premix Ex Taq II, 0.8 μL of each primer (10 mM), 2 μL sample cDNA (2.5 ng RNA), 0.4 μL ROX, and 6 μL sterile distilled water. Thermal cycling was performed at 95°C for 30 s (pre-cycling) followed by 40 cycles at 95°C for 10 s, 57°C for 10 s, 60°C for 20 s, 95°C for 10 s, and a melting curve analysis at 65°C for 5 s. The annealing temperature was increased by 0.5°C per cycle to 95°C. All reactions were performed in triplicate. Gene expression levels in the adult gut tissues were compared with those in the other tissues and quantitated by the comparative 2–ΔΔCt method (Livak and Schmittgen, 2001) with Applied Biosystems StepOnePlusTM software (Applied Biosystems, Foster City, CA, United States). The E. scrobiculatus RPL13 gene was the endogenous control for normalizing PCWDE expression. The PCR amplifications was performed separately for each tissue using the same primers as those for RT-PCR amplification (Supplementary Table S1).
Statistical Analysis
ONE-way analysis of variance (ANOVA) with Tukey’s honestly significant difference (HSD) test was used to determine the differences in the expression of each PCWDE in the six tested tissues (head, leg, proboscis, foregut, midgut, and hindgut). The statistical analysis was performed using SPSS (version 20.0) and significant expression was considered for P < 0.05 (IBM Corp, Armonk, NY, United States). Cycle threshold (Ct) values are presented as mean ± SE.
Results
Transcriptome Sequencing and Unigene Assembly
The three midgut cDNA libraries (ESM1, ESM2, and ESM3) for E. scrobiculatus were sequenced in an Illumina HiSeq 4000 system (Illumina, San Diego, CA, United States). The raw reads and the Q20 and Q30 base call accuracies of the three ESM samples are shown in Supplementary Table S1. The adaptors were trimmed, low-quality sequences were removed, and the ESM1, ESM2, and ESM3 sequences were blended, spliced, and assembled. We obtained 21,491 unigenes with N50 = 2,736 bp and mean length = 1,520 bp (Supplementary Table S1). The assembled unigene lengths ranged from 201–29,088 bp (Supplementary Figure S1). The raw E. scrobiculatus reads were deposited in the NCBI SRA database under GenBank accession number SRP070604.
Homology Searching and Gene Functional Annotation
Unigenes were used as queries for BLASTx and BLASTp searches against protein databases. The E-value cutoff was 10–5. There were 4,269 (19.86%), 10,041 (46.72%), 6,127 (28.51%), 8,482 (39.47%), 3,540 (16.47%), 5,491(25.55%), 361 (1.68%), and 9,197 (42.79%) E. scrobiculatus unigenes. They were annotated with the Pfam, SwissProt, KEGG, String, COG, KOG, NOG, and GO databases, respectively (Supplementary Figure S2). A total of 17,747 (82.58%) of the 21,491 unigenes were annotated based on the homology and matches to the aforementioned databases. In addition, 14,925 (69.45%) of the 21,491 unigenes were annotated against the NCBI NR protein database. Homology searches revealed that the E. scrobiculatus midgut transcriptomes had the highest similarity (54.41%) with the sequences from D. ponderosae followed by Tribolium castaneum (17.83%; Supplementary Figure S3).
A Blast2GO search of the NR database assigned the GO terms ‘biological process’, ‘cellular component’ and ‘molecular function’ categories to 9,197 (15.61%) of the E. scrobiculatus unigenes. The cellular and metabolic process subcategories were the most highly represented within the biological process category. The cell and cell parts subcategories were highly abundant in the cellular component category. The most abundant unigenes in the molecular function category were associated with ‘binding’ and ‘catalytic activity’ (Figure 1).
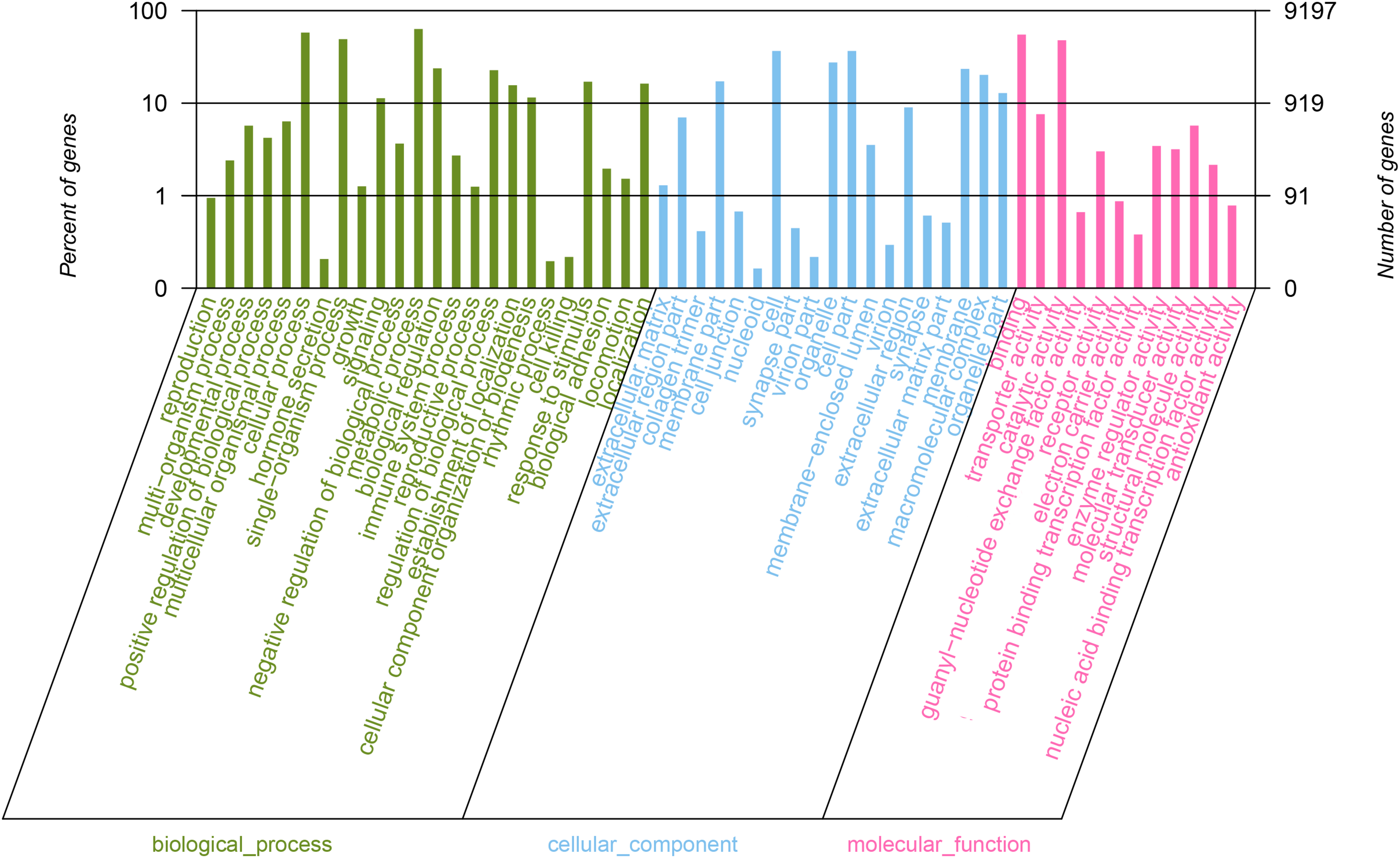
Figure 1. Functional annotation of all unigenes based on gene ontology (GO) categorization. GO analysis was performed at the level for three main categories (cellular component, molecular function, and biological process).
Identification of Putative E. scrobiculatus PCWDE Candidates
We identified 25 unigenes encoding putative PCWDEs in the E. scrobiculatus midgut transcriptomes and the previous transcriptome (GenBank accession number: SRX719565). EscrGH45-1,EscrGH28-1, EscrGH28-2 and EscrGH28-3were identified in this previous transcriptome (SRX719565), and other genes were identified in the midgut transgene (SRP070604). These unigenes were verified by a BLASTx homology search. We selected the top matches for each unigene (Tables 1, 2 and Supplementary Table S3) and named them according to the Escr-enzyme-number format (named from 1–n, where n is the number of each enzyme family). Two genes (EscrGH48-3 and EscrCE8-3) obtained complete open reading frames by 3’RACE and the 3’RACE primers are shown in Supplementary Table S4.
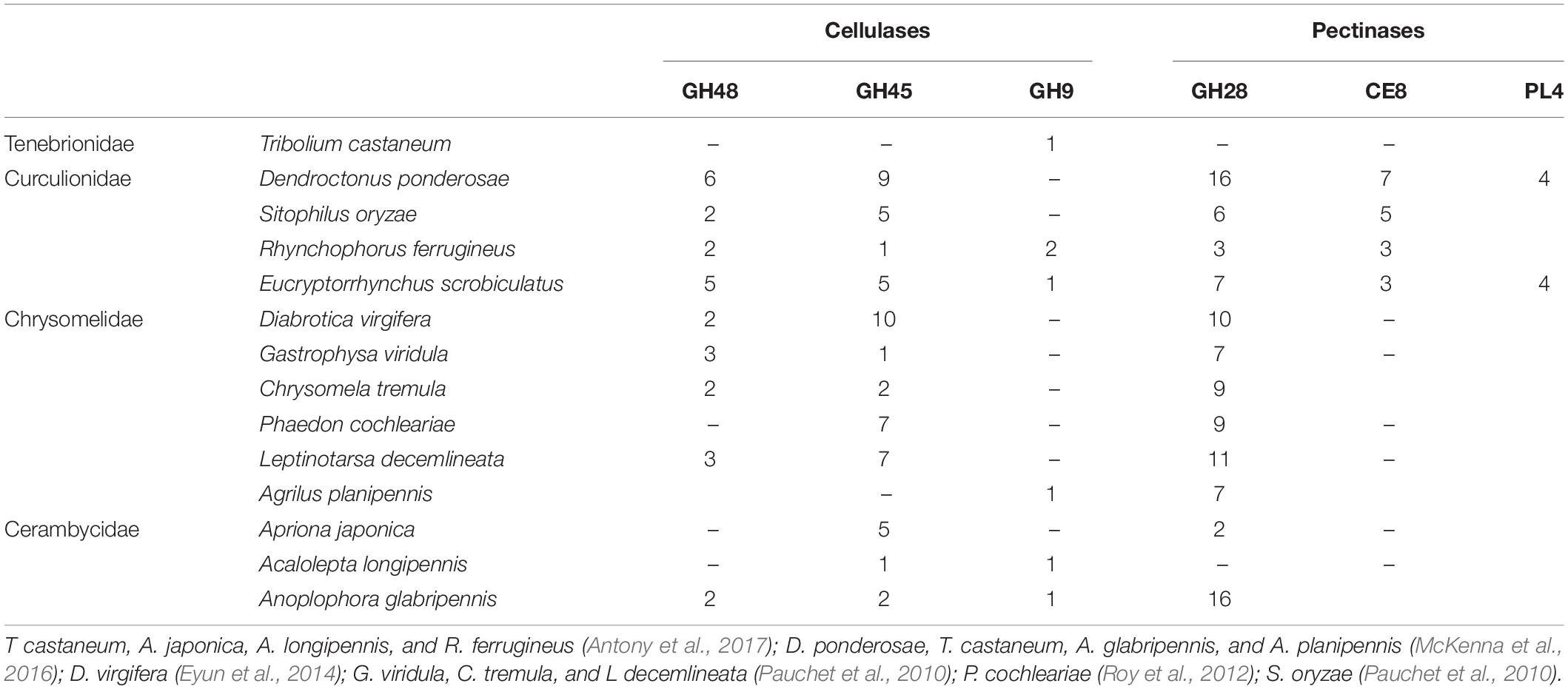
Table 2. A comparison of the number of plant cell wall-degrading enzymes identified from E. scrobiculatus and other beetles.
Of the 25 PCWDEs, 11 were grouped into cellulolytic enzymes (GH9, GH45, and GH48) and 14 were annotated as pectolytic enzymes (GH28, PL4, and CE8) (Table 1 and Supplementary Table S4). Cellulose digestion involves several enzymes in the GH superfamily. Our analysis of the midgut transcriptomes of E. scrobiculatus disclosed five GH48 family genes. All but one unigene (EscrGH48-4) had complete ORFs. Four unigenes comprised predicted signal peptide (SP) sequences. EscrGH48-5 matched Sitophilus oryzae GH48, EscrGH48-1 matched Otiorhynchus sulcatus GH48 (CAH25542.1), EscrGH48-2 and EscrGH48-4 matched Gastrophysa viridula GH48, and EscrGH48-4 shared 50% similarity with Leptinotarsa decemlineata GH48. The FPKM values for EscrGH48-5 and EscrGH48-1 were 5,676.389 and 345.209, respectively. Thus, they were highly expressed genes (Table 1). Members of the GH45 class of CAZy enzymes have endoglucanase activity and various uncharacterized functions (Busch et al., 2019). Five candidates of this class have been identified (EscrGH45-1–EscrGH45-5). All EscrGH45 sequences matched the GH45 enzymes of D. ponderosae with >70% similarity (Table 1). GH9 class enzymes have endoglucanase activity. Here, we identified EscrGH9-1 which shared 55% homology with Anoplophora glabripennis GH9 (XP_018568194.1 or AGLA010313; https://i5k.nal.usda.gov/).
Seven GH28 unigenes (EscrGH28-1–EscrGH28-7), encoding putative pectin-degrading polygalacturonases were identified in the InterPro program (Supplementary Table S4). Four GH28 unigenes were highly expressed in midgut transcription (FPKM > 10). In other insects, their homologs function as polygalacturonases. EscrGH28-6 resembled the GH28 reported for S. oryzae. The others were similar to GH28 from D. ponderosae (Supplementary Table S4). Enzymes of the CE8 class function as pectin methylesterases (PMEs) and degrade the pectin network. We putatively identified three CE8 unigenes. EscrCE8-1 and EscrCE8-2 shared high sequence similarity with S. oryzae PME. The EscrCE8-3 sequence most closely resembled the CE8 from S. oryzae (Supplementary Table S4). EscrCE8-2 shared 59% similarity with S. oryzae pectin methylesterase. The latter is the only beetle pectinase whose structure has been elucidated and reported (Teller et al., 2014). Four unigenes were assigned to PL4. EscrPL4-1 shares 68% similarity with D. ponderosae PL4. EscrPL4-2 sequences matched the PL4 enzymes in D. ponderosae with >72% homology. EscrPL4-3 and EscrPL4-4 shared high sequence similarity with D. ponderosae PL4 (Supplementary Table S4).
Phylogenetic Analysis of E. scrobiculatus PCWDEs
Candidate GH48 Genes
A phylogenetic tree was constructed from the catalytic domain sequences of five of the GH48 family genes and those of fungi, bacteria, and Coleoptera. The E. scrobiculatus GH48 sequences had high homology to the endoglucanases from D. ponderosae. The GH48 genes from the Coleoptera had only one GH48 domain. However, the GH48 genes from bacteria and fungi had other functional domains in addition to GH48 (Figure 2).
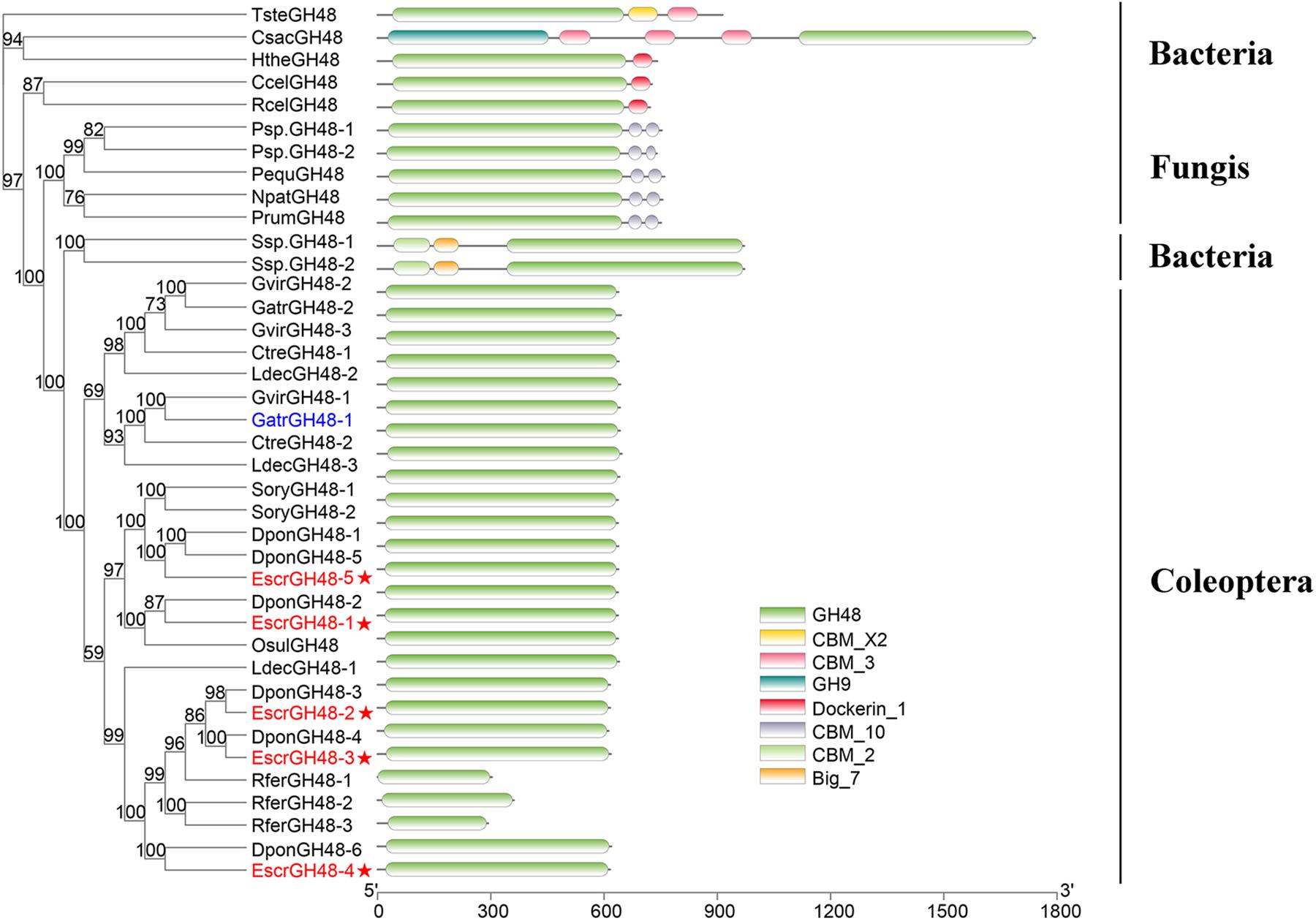
Figure 2. Comparison of amino acid sequences and domain structures in E. scrobiculatus and glycosyl hydrolase family 48 (Family GH48) members. The scale indicates distance (number of amino acid substitutions per site). The E. scrobiculatus genes are shown in red and marked with an asterisk. Members of fungi, bacteria, and insects were temporarily grouped. GH48, catalytic domain of glycosyl hydrolase family 48; GH9, catalytic domain of glycosyl hydrolase family 9; CBM 3, 10, cellulose binding domain; Big7, Bacterial Ig domain: CBM X2, Carbohydrate binding domain X2. Amino acid sequences used for the tree are listed in Supplementary Table S5.
Candidate GH45 Genes
A phylogenetic tree was built using all GH45 families and those of the Coleoptera. The EscrGH45 family genes clustered with those of the Curculionidae. The GH45 family genes from Curculionidae were grouped into four clusters. The EscrGH45 family genes were distributed into four clusters (Figure 3). EscrGH45-2 clustered with the GH45 gene of S. oryzae, which has been verified to have endo-β-1,4-xyloglucanases activity. At the same time, EscrGH45-5 clustered with the GH45 gene of S. oryzae, which has been verified to have endo-β-1,4-glucanases activity.
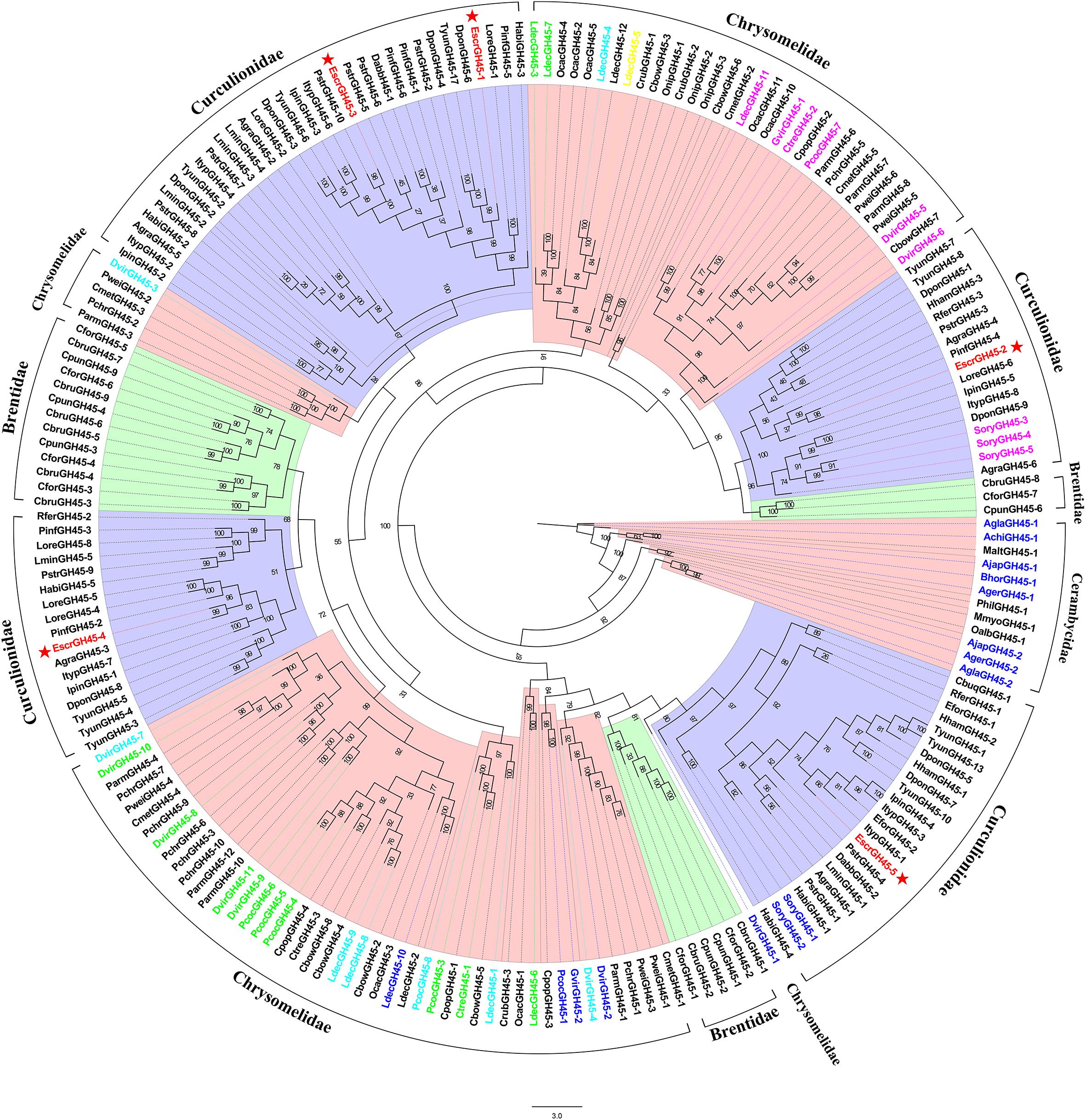
Figure 3. Comparison of amino acid sequences in E. scrobiculatus and glycosyl hydrolase family 45 (Family GH45) members. The E. scrobiculatus genes are shown in red and marked with an asterisk. Amino acid sequences used for the tree are listed in Supplementary Table S5. GH45s characterized to date are color-coded based on their activity; blue = endo-β-1,4-glucanases; green = endo-β-1,4-glucanases and (gluco)mannanases; yellow = endo-β-1,4-glucanases and endo-β-1,4-xyloglucanases; magenta = endo-β-1,4-xyloglucanases; cyan = no activity detected.
Candidate GH9 Genes
Comparatively few GH9 family genes were found in beetles. We used GH9 sequences from E. scrobiculatus and other insects (Coleoptera, Hymenoptera, Hemiptera, Phasmatodea, Blattodea, Embioptera, and Orthoptera) to build a phylogenetic tree. EscrGH9-1 had the highest homology with the A. glabripennis protein. The Coleoptera sequences were clustered into one group (Figure 4).
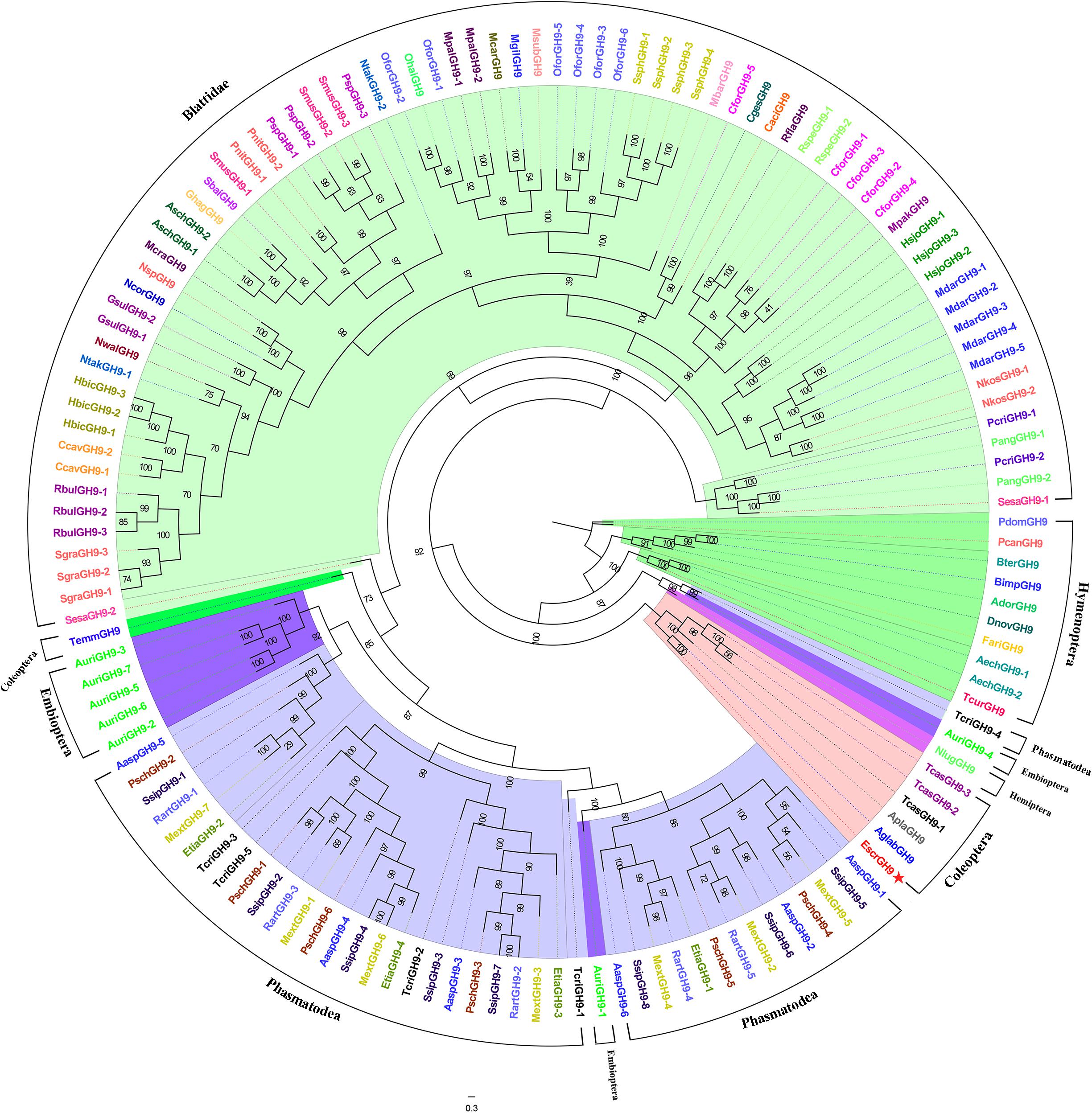
Figure 4. Comparison of amino acid sequences in E. scrobiculatus and glycosyl hydrolase family 9 (Family GH9) members. The scale indicates distance (number of amino acid substitutions per site). The E. scrobiculatus genes are shown in red and marked with an asterisk. Amino acid sequences used for the tree are listed in Supplementary Table S5.
Candidate GH28 Genes
We performed a phylogenetic analysis on our candidate GH28 family genes and those from the phytophagous beetles (Curculionidae and Chrysomelidae) by a Maximum Likelihood analysis where two fungal GH28 genes from Aspergillaceae were taken as an outgroup. All GH28 genes of the Curculionidae specific clade were not characterized, and EcrGH28-4, EcrGH28-5, EcrGH28-6, and EcrGH28-7 belong to this clade. Remarkably, the Curculionidae and Chrysomelidae clade contained three distinct subclades: one with almost all Chrysomelidae GH28 genes and SoryGH28-1 active on pectin (active clade A), one with five genes active only on pectic trimers (active clade B), and the third including all Chrysomelidae GH28 genes (except LdecGH8-9) with non-active on pectin or pectic trimers (non-active clade). EcrGH28-1, EcrGH28-2, and EcrGH28-3 belong to the active clade A (Figure 5).
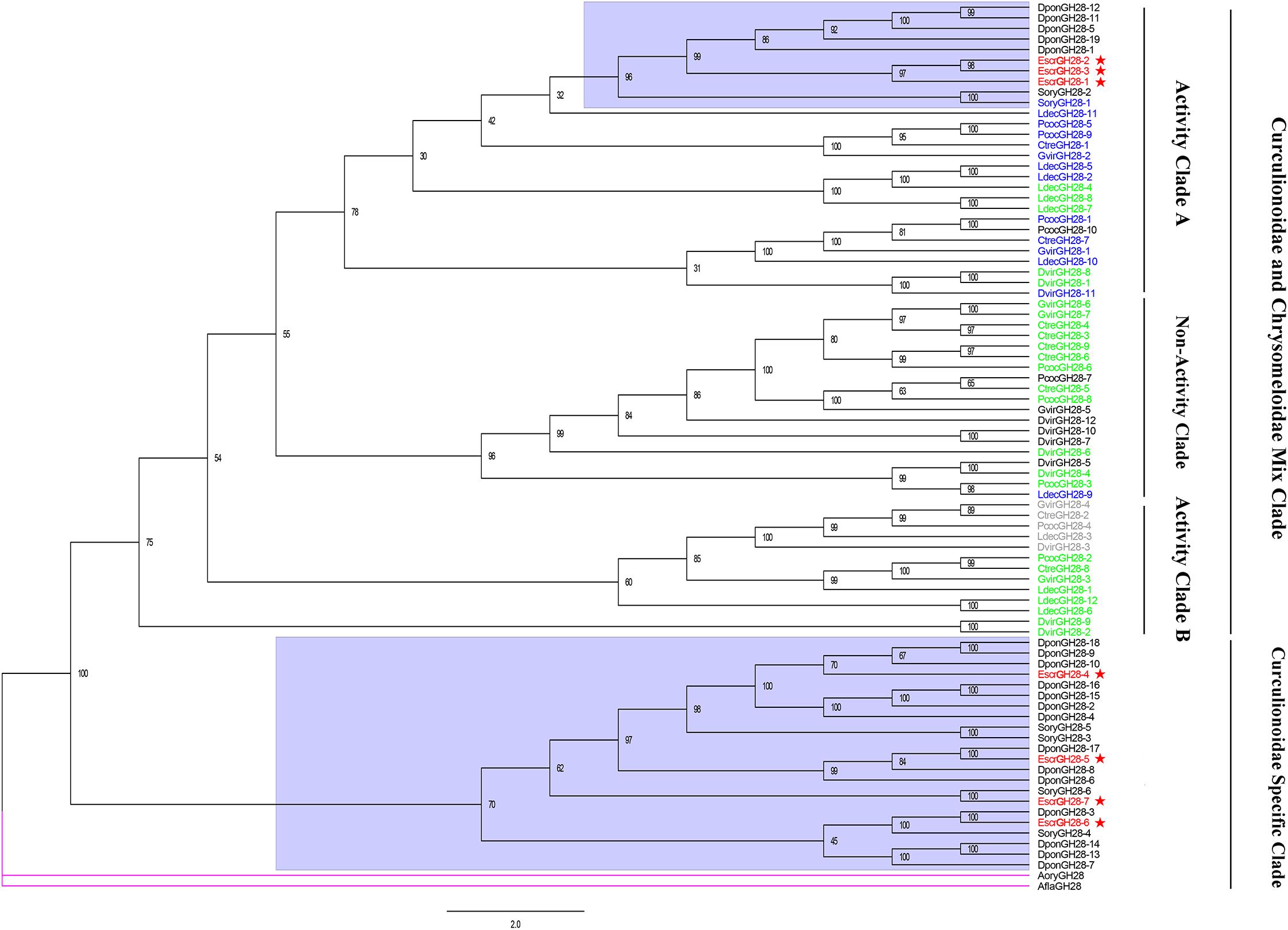
Figure 5. Comparison of amino acid sequences in E. scrobiculatus and glycosyl hydrolase family 28 (Family GH28) members. The E. scrobiculatus genes are shown in red and marked with an asterisk. Amino acid sequences used for the tree are listed in Supplementary Table S5. GH28 genes characterized to date are color-coded based on their activity; blue = activity on pectin; green = no activity detected; gray = activity toward tri-galacturonic acid.
Candidate CE8 Genes
We identified three putative CE8 unigenes. EscrCE8-1 and EscrCE8-2 shared high sequence similarity with S. oryzae PME (ADU33262.1). EscrCE8-3 was most similar to CE8 from S. oryzae (ADU33260.1; Table 3). EscrCE8-2 shared 59% similarity with S. oryzae pectin methylesterase. It is the only beetle pectinase whose structure has been elucidated and reported (Teller et al., 2014). The CE8 phylogenetic tree revealed that the CE8 genes of the Curculionidae shared similarity with those of bacteria (Figure 6).
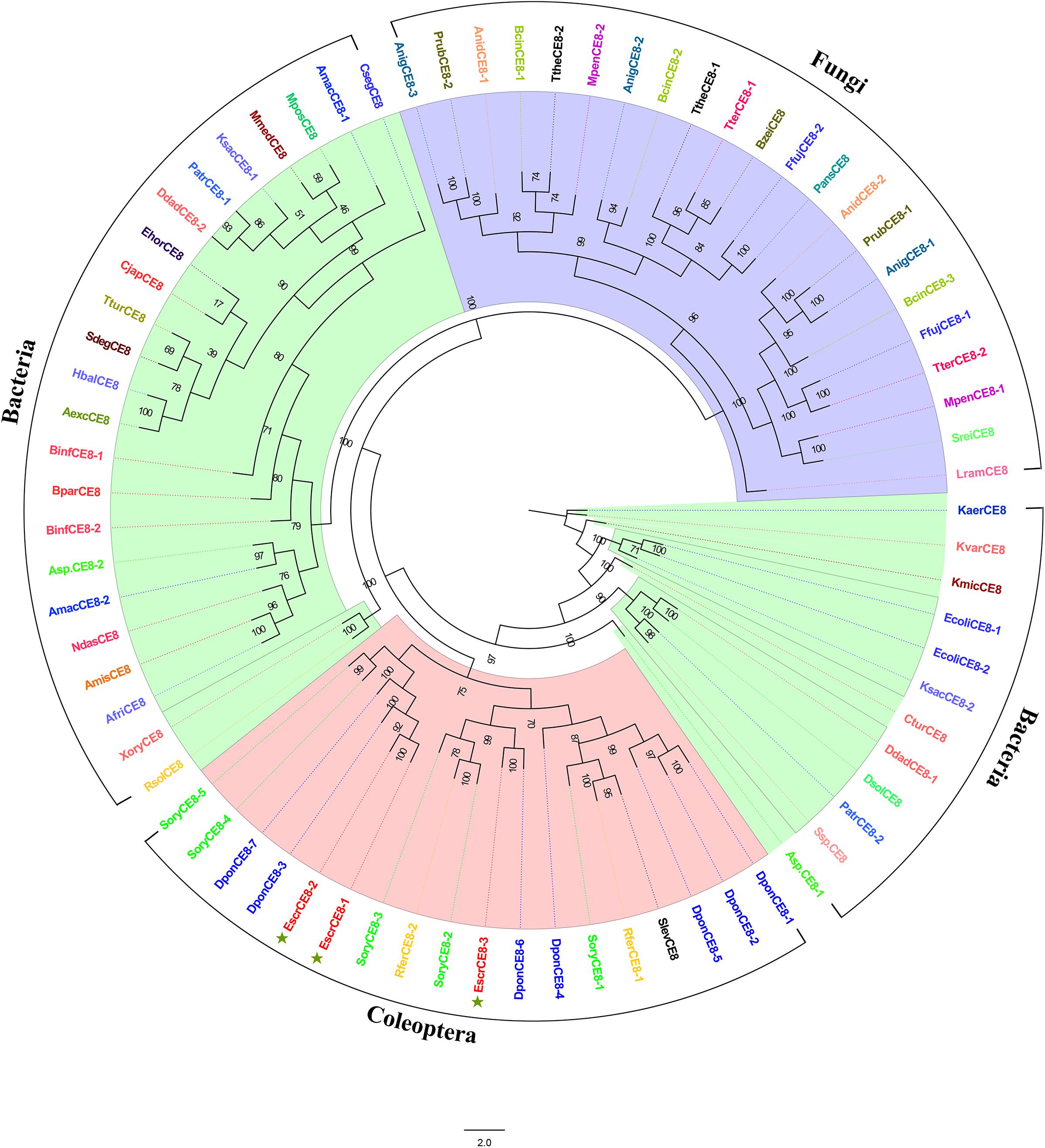
Figure 6. Comparison of amino acid sequences in E. scrobiculatus and glycosyl hydrolase family CE8 (Family GE8) members. The scale indicates distance (number of amino acid substitutions per site). The E. scrobiculatus genes are shown in red and marked with an asterisk. Amino acid sequences used for the tree are listed in Supplementary Table S5.
Tissue Expression Analysis of E. scrobiculatus PCWDEs
The specificity of the PCWDE primer was determined by RT-PCR. Fluorescence RT-qPCR was conducted to determine the expression patterns of the PCWDEs in various tissues. The expression levels of 13 genes were significantly higher in the midgut than the other tissues. These genes included three CHs (EscrGH45-2, EscrGH45-2, and EscrGH45-5) belonging to the cellulases, and eight genes (EscrCE8-1, EscrCE8-3, EscrGH28-4, EscrGH28-6, EscrGH28-7, EscrPL4-2, EscrPL4-3, and EscrPL4-4) belonging to the pectinases. EscrGH9-1, EscrGH45-4, EscrGH48-1, and EscrGH48-5 were highly expressed in the proboscis than the other tissues. EscrCE8-2 and EscrGH48-2 were upregulated in the midgut but their expression levels there did not significantly differ from those in the other tissues (Figure 7).
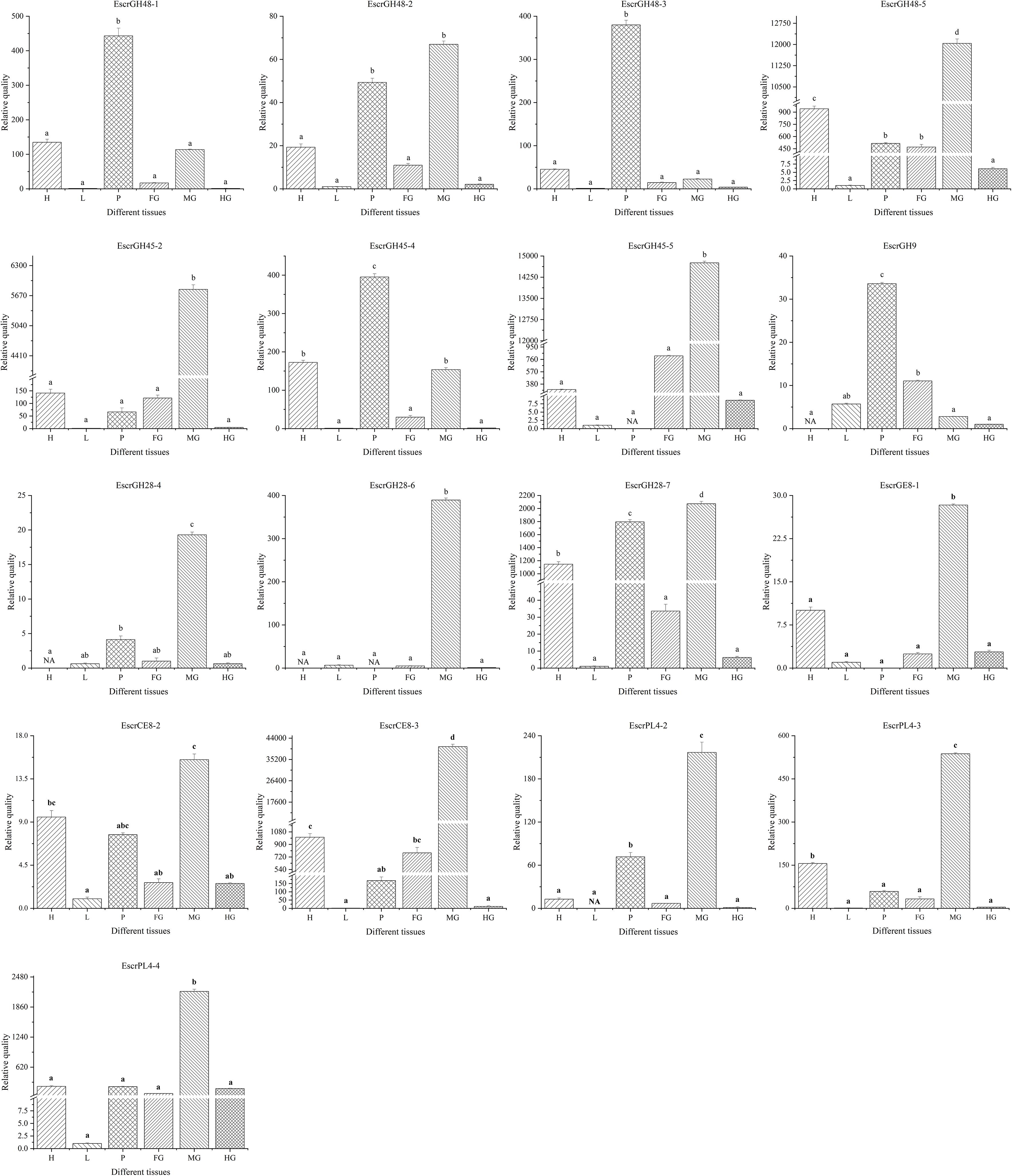
Figure 7. Plant cell wall-degrading enzymes (PCWDEs) transcript levels in different tissues of E. scrobiculatus. NA means the transcript level too low to measure.
Discussion
Twenty-five genes encoding PCWDEs and belonging to the glycoside hydrolases (GHs), polysaccharide lyases (PLs), and carbohydrate esterases (CEs) of CAZy enzymes were identified in the E. scrobiculatus midgut transcriptome (Table 2). A BLAST search in the NR and Swiss-Prot uniprot databases revealed that these genes were annotated as GH48, GH45, GH28, GH9, PL4, and CE8. Most of the candidate genes shared a high homology with those of D. ponderosae and S. oryzae. We performed statistics on the relevant PCWDE genes that had been functionally verified (Table 3). In order to predict the evolutionary relationship between these genes of E. scrobiculatus and related genes of other species, we conducted phylogenetic and domain analysis. A tissue-specific expression analysis of the gut, the main digestive organ in insects, disclosed relatively higher expression levels of 17 of the 25 identified PCWDEs. Our study determined the types and relative expression levels PCWDEs of E. scrobiculatus. These PCWDEs may be used for pest control of E. scrobiculatus and biofuels.
Previous studies have shown that endogenous cellulase of insects includes GH48, GH45, GH9, and GH5 (Pauchet et al., 2010; Calderón-Cortés et al., 2012; Fischer et al., 2013). Here, 11 genes belonging to GH48, GH45, and GH9 families were identified. However, no representatives of the GH5 family gene were detected (Table 1).
GH48 commonly occurs in cellulolytic bacteria and GH48 genes have cellulase activity (Berger et al., 2007). GH48 genes were identified in two fungal species (Piromyces equi and Piromyces sp.) (Steenbakkers et al., 2002). The GH48 family genes in Orpinomyces sp. and Neocallimastix patriciarum were also confirmed to have cellulase activity (Tzi-Yuan et al., 2011; Morrison et al., 2016). GH48 family genes have been identified in the superfamilies Curculionoidea and Chrysomeloidea of the Coleoptera (Fujita et al., 2006; Pauchet et al., 2010, 2014; Keeling et al., 2012; Eyun et al., 2014; McKenna et al., 2016; Antony et al., 2017). GH48 family genes have cellulase activity in bacteria and fungi. However, there is no evidence to indicate that insect GH48 family genes can break down cellulosic polysaccharides. GH48 family genes GatrGH48-1 and GatrGH48-2 (Figure 3) were isolated from Gastrophysa atrocyanea. Chitinase activity was associated with GatrGH48-1 (active phase-associated proteins (APAP) I). However, it has neither glucanase nor cellobiohydrolase activity (Fujita et al., 2006). No cellulase activity was found for the GH48 gene of Otiorhynchus sulcatus (Edwards et al., 2002). A domain structure analysis of the GH48 family genes by Pfam5 demonstrated that insect GH48 family genes have only one GH48 domain. The GH48 family genes of the Coleoptera share considerable amino acid sequence homology with those of bacteria and fungi (Figure 2). Nevertheless, the GH48 family genes in bacteria and fungi are constituents of complex proteins with multiple functional domains. The lack of other domains in GatrGH48-1 may account for the fact that it has no cellulase activity. EscrGH48 has the same domain structure as GatrGH48-1. Thus, we speculate that the EscrGH48 gene has chitinase activity and that the GH48 family of E. scrobiculatus does not have cellulase activity and does not degrade plant cells. The four genes of the GH48 family we quantitatively tested were highly expressed in the proboscis and midgut. The relative expression levels of EscrGH48-1 and EscrGH48-3 in the proboscis were significantly different from those in the other tissues. At the same time, the relative expression level of EscrGH48-5 in the midgut was significantly different from that in other tissues. We speculate that the proboscis and midgut are both organs that digest chitin.
Previous research showed that phytophagous beetles presumably obtained GH45 genes from fungi through an HGT event (Busch et al., 2019). The GH45 family genes were described for the Coleopteran superfamilies Chrysomeloidea and Curculionoidea. The GH45 family of Chrysomelidae gene function has been differentiated and now has cellulase, xyloglucanase, and glucomannanase activity (Busch et al., 2019). To study the evolutionary relationship of the beetle’s GH45 genes, we constructed a maximum-likelihood phylogenetic tree using the GH45 family genes of Coleoptera (Pauchet et al., 2010; Eyun et al., 2014; Antony et al., 2017; Busch et al., 2019). This phylogenetic tree showed that the EscrGH45 gene was localized to four distinct groups (Figure 3). In the cluster of GH45 genes including EscrGH45-5, SoryGH45-1, and SoryGH45-2 of S. oryzae of Curculionidae have endo-β-1,4-glucanase and endo-β-1,4-xyloglucanase activity (Busch et al., 2019). The FPKM value of GH45-5 in the midgut transcriptome was ≤ 8,000. We speculate that EscrGH45-5 has cellulase activity. SoryGH45-3, SoryGH45-4, and SoryGH45-5 can only break down xyloglucan rather than cellulose. EscrGH45-2 is highly homologous with these genes. The FPKM of EscrGH45-2 in the midgut transcriptome was 4,000. Thus, EscrGH45-2 may have xyloglucanase activity. As more GH45 genes in weevil are functionally validated, these GH45 genes may have three enzymatic activities (endo-β-1,4-glucanase, endo-β-1,4-xyloglucanase and mannanase). The RT-qPCR analysis revealed that the relative expression levels of EscrGH45-2 and EscrGH45-5 in the midgut were significantly higher than other tissues. The proboscis presented with the highest expression levels of EscrGH45-4. We speculate that cellulose is digested in the proboscis and midgut in E. scrobiculatus. Initially, the GH9 gene was thought to be absent in the chrysomelid and curculionid superfamilies (Watanabe and Tokuda, 2010; Calderón-Cortés et al., 2012; Fischer et al., 2013). The GH9 family genes were considered lost in the ancestors common to Chrysomelidae and Curculionidae (Eyun et al., 2014). Recently, however, the GH9 gene was discovered in the two aforementioned superfamilies (Antony et al., 2017; Busch et al., 2018). The GH9 genes were distributed in all insect orders examined thus far. A common ancestor of the insects may have borne GH9 cellulase genes that were vertically transferred to extant (descendant) insects (Fischer et al., 2013). In the phylogenetic tree, the GH9 genes of the Coleoptera were grouped into one cluster and EscrGH9-1 was highly homologous with AglaGH9. The latter harbored no endo-acting enzymatic activity for carboxymethylcellulose (McKenna et al., 2016). The FPKM for EscrGH9 in the midgut transcriptome was 3.151. The relative expression levels of EscrGH9-1 were low in all six insect tissues. Of the Curculionidae that have been studied, none presented with the GH9 gene. Therefore, the GH9 gene function has been lost or replaced in these insects. Thus, EscrGH9-1 may lack cellulase activity and may not be a plant cell-degrading enzyme.
Insect pectinases comprise rhamnogalacturonan lyases, pectin methylesterases, and polygalacturonases which belong to the multi-gene carbohydrate polysaccharide lyase family (PL4), esterase family 8 (CE8), and glycosyl hydrolase family 28 (GH28), respectively (Pauchet et al., 2010; Calderón-Cortés et al., 2012; Kirsch et al., 2016). Advances in sequencing technology and bioinformatic analysis have disclosed that endogenous GH28 is ubiquitous among insect taxa (Calderón-Cortés et al., 2012). Kirsch et al. (2014) indicated that three independent HGT events of GH28 genes occurred during the evolution of phytophagous beetles, one of which was retained by most descendent Curculionoidea (Kirsch et al., 2014). Phytophagous beetles acquired genes encoding active GH28 genes via HGTs, and these HGTs promoted their capacity to utilize plant material as a primary source of food (Kirsch et al., 2014). Most of the GH28 family genes identified in A. glabripennis of the Cerambycidae were active against at least one homogalacturonan polymer. As these gene functions are highly complementary, A. glabripennis efficiently decomposes pectineus homogalacturonan polymers. However, no other pectinase was identified in A. glabripennis (McKenna et al., 2016). Up to 19 GH28 family genes were identified in D. ponderosae (Eyun et al., 2014). At the same time, PL4 and CE8 were also identified in D. ponderosae (Pauchet et al., 2010). In addition to pectinase activity and tri galacturonase activity, the high number of catalytically inactive phytophagous GH28 proteins may act as “decoy” targets for GH28 inhibitory proteins, thus protecting the active GH28 proteins from inhibition (Kirsch et al., 2014). Here, seven GH28 genes were identified in E. scrobiculatus and four of them were localized to the midgut transcriptome. The FPKM for EscrGH28-4 and EscGH28-7 in the midgut transcriptome were 179.462 and 638.290, respectively. Phylogenetic analysis revealed that EscrGH28, DponGH28, and SoryGH28 genes were grouped into two distinct subclades based on the beetle’s GH28 protein (Figure 5). We speculate that the function of EscrGH28 genes may have differentiated. Kirsch et al. (2016) study indicated that the digestion of complex pectin polysaccharides in S. oryzae required CE8 family genes in addition to GH28 family genes (Kirsch et al., 2016). The function of these CE8 family genes has been verified, two of which have pectinase activity (Shen et al., 1999; Kirsch et al., 2016). Three CE8 genes were also identified E. scrobiculatus and the FPKM for EscCE8-1 was 13,453.841. To date, CE8 genes have only been found in weevils and in no other animals. The origin of weevil CE8 genes can be best explained by a HGT event from bacteria to weevils (Evangelista et al., 2015; Kirsch et al., 2016). In addition to the families of GH28 and CE8 genes, five PL4 family genes were described for D. ponderosae. They were putative rhamnogalacturonan lyases which constitute another class of pectolytic enzymes (Antony et al., 2017). Four PL4 family genes were identified in E. scrobiculatus (Supplementary Table S4). Except for EscPL4-3, the FPKMs of the PL4 family genes were ≥ 250. To date, the PL4 family of genes have only been identified in the Curculionidae. The RT-qPCR analysis was performed on the nine putative pectinase genes with comparatively high FPKMs (EscrPL4-2, EscrPL4-3, EscrPL4-4, EscrCE8-1, EscrCE8-2, EscrCE8-3, EscrGH28-4, EscrGH28-6, and EscrGH28-7). The relative expression level of those genes except for EscrGE8-2 in the midgut was significantly higher than that in other tissues. The midgut is the main digestive organ of insects, and high expression of these genes also indirectly indicates that these genes are related to digestion. In summary, we speculate that the GH28, CE8, and PL4 genes in E. scrobiculatus synergistically break down the complex polysaccharide of pectin. The functions of the PCWDEs examined in the present study were neither determined nor validated. In future research, we will verify the functionality of the PCWDEs. The potential of cellulase application in RNAi-medicated pest control strategies has been demonstrated by silencing a termite endogenous cellulase (Zhou et al., 2008). Plant cellulose can be digested by cellulase to produce the bioenergy source cellulosic ethanol (Willis et al., 2017). With the development of genetic engineering of bioenergy, elucidation of the functions of the major PCWDEs will be highly valuable.
Here, we identified 25 unigenes encoding the GH family enzymes GH48, GH45, GH38, GH28, and GH16), four unigenes encoding PL4 family enzymes, and three unigenes encoding CE family enzymes in the E. scrobiculatus transcriptome. Quantitative expression, phylogenetic analyses, and FPKMs identified the cellulases (EscrGH45-2 and EscrGH45-5) and pectinases (EscrGH28-7, EscrCE8-3, and EscrPL4-4) that were the most highly expressed in E. scrobiculatus. Thence, the enzymatic activities of the PCWDEs were predicted.
As E. scrobiculatus is a major pest of A. altissima, identification of the PCWDEs has practical applications in E. scrobiculatus pest management. We identified the PCWDEs, performed a phylogenetic analysis on them, determined their relative expression patterns and levels in various insect tissues. The present work has reference significance for the study of other plant cell wall degrading enzymes in weevils.
Data Availability Statement
The datasets transcriptome for this study can be found in in the NCBI SRA database under GenBank accession number SRP070604.
Author Contributions
PG and JW conceived and designed the experiments. PG, ZL, and JW participated in regular discussions regarding the design of the laboratory experiments and analysis of the sequencing data. PG performed the experiments and analyzed the data. JW obtained funding. PG contributed to the writing of this article. All authors read and approved the final manuscript.
Funding
This work was supported by National Natural Sciences Foundation of China (Grant No. 31770691) and National Key R&D Program of China (2018YFC1200400).
Conflict of Interest
The authors declare that the research was conducted in the absence of any commercial or financial relationships that could be construed as a potential conflict of interest.
Acknowledgments
We thank Kailang Yang and Xiaojian Wen (Beijing Forestry University, China) for help with insect collection.
Supplementary Material
The Supplementary Material for this article can be found online at: https://www.frontiersin.org/articles/10.3389/fphys.2020.01111/full#supplementary-material
Footnotes
- ^ https://github.com/jstjohn/SeqPrep
- ^ https://github.com/najoshi/sickle
- ^ http://trinityrnaseq.sourceforge.net/
- ^ http://www.ncbi.nlm.nih.gov/gorf/gorf.html
- ^ http://pfam.xfam.org/
References
Altschul, S. F., Gish, W., Miller, W., Myers, E. W., and Lipman, D. J. (1990). Basic local alignment search tool. J. Mol. Biol. 215, 403–410.
Antony, B., Johny, J., Aldosari, S. A., and Abdelazim, M. M. (2017). Identification and expression profiling of novel plant cell wall degrading enzymes from a destructive pest of palm trees, Rhynchophorus ferrugineus. Insect. Mol. Biol. 26, 469–484. doi: 10.1111/imb.12314
Berger, E., Zhang, D., Zverlov, V. V., and Schwarz, W. H. (2007). Two noncellulosomal cellulases of Clostridium thermocellum, Cel9I and Cel48Y, hydrolyse crystalline cellulose synergistically. FEMS Microbiol. Lett. 268, 194–201. doi: 10.1111/j.1574-6968.2006.00583.x
Busch, A., Danchin, E. G. J., and Pauchet, Y. (2019). Functional diversification of horizontally acquired glycoside hydrolase family 45 (GH45) proteins in Phytophaga beetles. BMC Evolut. Biol. 19:100. doi: 10.1186/s12862-019-1429-9
Busch, A., Kunert, G., Wielsch, N., and Pauchet, Y. (2018). Cellulose degradation in Gastrophysa viridula (Coleoptera: Chrysomelidae): functional characterization of two CAZymes belonging to glycoside hydrolase family 45 reveals a novel enzymatic activity. Insect. Mol. Biol. 27, 633–650. doi: 10.1111/imb.12500
Calderón-Cortés, N., Quesada, M., Watanabe, H., Cano-Camacho, H., and Oyama, K. (2012). Endogenous plant cell wall digestion: a key mechanism in insect evolution. Annu. Rev. Ecol. Evolut. Syst. 43, 45–71. doi: 10.1146/annurev-ecolsys-110411-160312
Cantarel, B. L., Coutinho, P. M., Rancurel, C., Bernard, T., Lombard, V., and Henrissat, B. (2009). The carbohydrate-active EnZymes database (CAZy): an expert resource for Glycogenomics. Nucleic Acids Res. 37, D233–D238.
Chen, C., Chen, H., Zhang, Y., Thomas, H. R., Frank, M. H., He, Y., et al. (2020). TBtools: an integrative toolkit developed for interactive analyses of big biological data. Mol. Plant 13, 1194–1202. doi: 10.1016/j.molp.2020.06.009
Chen, Y. (2016). The Comparative Study of Alimentary Canal Morphology and Cellulase in Eucryptorrhynchus scrobiculatus and E. brandti. Master thesis, Beijing Forestry University, Beijing.
Ding, J., Reardon, R., Wu, Y., Zheng, H., and Fu, W. (2006). Biological control of invasive plants through collaboration between China and the United States of America: a perspective. Biol. Invas. 8, 1439–1450. doi: 10.1007/s10530-005-5833-2
Edwards, M. G., Harrison, O., and Gatehouse, J. A. (2002). Digestive Enzymes of Vine Weevil (Otiorhynchus sulcatus) as Potential Targets for Insect Control Strategies. Ph. D. thesis, University of Durham, Durham.
Evangelista, D. E., de Paula, F. F., Rodrigues, A., and Henrique-Silva, F. (2015). Pectinases from Sphenophorus levis Vaurie, 1978 (Coleoptera: Curculionidae): putative accessory digestive enzymes. J. Insect. Sci. 15:168.
Eyun, S. I., Wang, H., Pauchet, Y., Ffrench-Constant, R. H., Benson, A. K., Valencia-Jimenez, A., et al. (2014). Molecular evolution of glycoside hydrolase genes in the Western corn rootworm (Diabrotica virgifera virgifera). PLoS One 9:e94052. doi: 10.1371/journal.pone.0094052
Fischer, R., Ostafe, R., and Twyman, R. M. (2013). Cellulases from insects. Adv. Biochem. Eng. Biotechnol. 136, 51–64. doi: 10.1007/10_2013_206
Fujita, K., Shimomura, K., Yamamoto, K., Yamashita, T., and Suzuki, K. (2006). A chitinase structurally related to the glycoside hydrolase family 48 is indispensable for the hormonally induced diapause termination in a beetle. Biochem. Biophys. Res. Commun. 345, 502–507. doi: 10.1016/j.bbrc.2006.04.126
Girard, C., and Jouanin, L. (1999). Molecular cloning of cDNAs encoding a range of digestive enzymes from a phytophagous beetle, Phaedon cochleariae. Insect. Biochem. Mol. Biol. 2, 1129–1142. doi: 10.1016/s0965-1748(99)00104-6
Ji, Y., Gao, P., Zhang, G.-Y., Wen, C., and Wen, J.-B. (2017a). Micro-habitat niche differentiation contributing to coexistence of Eucryptorrhynchus scrobiculatus Motschulsky and Eucryptorrhynchus brandti (Harold). Biocontrol. Sci. Technol. 27, 1180–1194. doi: 10.1080/09583157.2017.1390069
Ji, Y., Luo, W., Zhang, G., and Wen, J. (2017b). Projecting potential distribution of Eucryptorrhynchus scrobiculatus Motschulsky and E. brandti (Harold) under historical climate and RCP 8.5 scenario. Sci. Rep. 7:9163.
Juge, N. (2006). Plant protein inhibitors of cell wall degrading enzymes. Trends Plant Sci. 11, 359–367. doi: 10.1016/j.tplants.2006.05.006
Keeling, C. I., Henderson, H., Li, M., Yuen, M., Clark, E. L., Fraser, J. D., et al. (2012). Transcriptome and full-length cDNA resources for the mountain pine beetle, Dendroctonus ponderosae Hopkins, a major insect pest of pine forests. Insect. Biochem. Mol. Biol. 42, 525–536. doi: 10.1016/j.ibmb.2012.03.010
Keeling, C. I., Yuen, M. M. S., Liao, N. Y., Docking, T. R., Chan, S. K., Taylor, G. A., et al. (2013). Draft genome of the mountain pine beetle, Dendroctonus ponderosae Hopkins, a major forest pest. Genome Biol. 14:R27.
Kirsch, R., Gramzow, L., Theissen, G., Siegfried, B. D., Ffrench-Constant, R. H., Heckel, D. G., et al. (2014). Horizontal gene transfer and functional diversification of plant cell wall degrading polygalacturonases: key events in the evolution of herbivory in beetles. Insect. Biochem. Mol. Biol. 52, 33–50. doi: 10.1016/j.ibmb.2014.06.008
Kirsch, R., Heckel, D. G., and Pauchet, Y. (2016). How the rice weevil breaks down the pectin network: enzymatic synergism and sub-functionalization. Insect. Biochem. Mol. Biol. 71, 72–82. doi: 10.1016/j.ibmb.2016.02.007
Li, B., and Dewey, C. N. (2011). RSEM accurate transcript quantification from RNA-Seq data with or without a reference genome. BMC Bioinform. 12:323. doi: 10.1186/1471-2105-12-323
Liu, Z. K., and Wen, J. B. (2016). Transcriptomic analysis of Eucryptorrhynchus chinensis (Coleoptera: Curculionidae) using 454 pyrosequencing technology. J. Insect. Sci. 16:82. doi: 10.1093/jisesa/iew067
Livak, K. J., and Schmittgen, T. D. (2001). Analysis of relative gene expression data using real-time quantitative PCR and the 2(-Delta Delta C(T)) method. Methods 25, 402–408. doi: 10.1006/meth.2001.1262
McAvoy, T. J., Salom, S. M., Yu, B., Ji, H. L., Du, Y. Z., Johnson, N., et al. (2013). Occurrence and development of Eucryptorrhynchus brandti and E. chinensis (Coleoptera: Curculionidae) on Ailanthus altissimatrees subjected to different levels of mechanical damage. Biocontrol Sci. Technol. 24, 65–79. doi: 10.1080/09583157.2013.847902
McKenna, D. D., Scully, E. D., Pauchet, Y., Hoover, K., and Kirsch, R. (2016). Genome of the Asian longhorned beetle (Anoplophora glabripennis), a globally significant invasive species, reveals key functional and evolutionary innovations at the beetle-plant interface. Genome Biol. 17:227.
Minh, B. Q., Schmidt, H. A., Chernomor, O., Schrempf, D., Woodhams, M. D., von Haeseler, A., et al. (2020). IQ-TREE 2: new models and efficient methods for phylogenetic inference in the genomic era. Mol. Biol. Evol. 37, 1530–1534. doi: 10.1093/molbev/msaa015
Morrison, J. M., Elshahed, M. S., and Youssef, N. H. (2016). Defined enzyme cocktail from the anaerobic fungus Orpinomyces sp. strain C1A effectively releases sugars from pretreated corn stover and switchgrass. Sci. Rep. 6:29217.
Pauchet, Y., and Heckel, D. G. (2013). The genome of the mustard leaf beetle encodes two active xylanases originally acquired from bacteria through horizontal gene transfer. Proc. Biol. Sci. 280:20131021. doi: 10.1098/rspb.2013.1021
Pauchet, Y., Kirsch, R., Giraud, S., Vogel, H., and Heckel, D. G. (2014). Identification and characterization of plant cell wall degrading enzymes from three glycoside hydrolase families in the cerambycid beetle Apriona japonica. Insect. Biochem. Mol. Biol. 49, 1–13. doi: 10.1016/j.ibmb.2014.03.004
Pauchet, Y., Wilkinson, P., Chauhan, R., and Ffrench, R. H. (2010). Diversity of beetle genes encoding novel plant cell wall degrading enzymes. PLoS One 5:e15635. doi: 10.1371/journal.pone.0015635
Price, M. N., Dehal, P. S., and Arkin, A. P. (2010). FastTree 2–approximately maximum-likelihood trees for large alignments. PLoS One 5:e9490. doi: 10.1371/journal.pone.009490
Roy, K., Wielsch, N., Vogel, H., Svatoš, A., Heckel, D. G., and Pauchet, Y. (2012). Combining proteomics and transcriptome sequencing to identify active plant-cell-wall-degrading enzymes in a leaf beetle. BMC Genom. 13:587. doi: 10.1186/1471-2105-12-587
Schuman, M. C., and Baldwin, I. T. (2016). The layers of plant responses to insect herbivores. Annu. Rev. Entomol. 61, 373–394. doi: 10.1146/annurev-ento-010715-023851
Shen, Z., Pappan, K., Mutti, N. S., He, Q.-J., and Denton, M. (1999). Pectin methylesterase from the rice weevil Sitophilus oryzae (L.)(Coleoptera Curculionidae) Purification and characterization. Insect. Biochem. Mol. Biol. 29, 209–214. doi: 10.1016/s0965-1748(98)00127-1
Steenbakkers, P. J. M., Freelove, A., Van Cranenbroek, B., Sweegers, B. M. C., Harhangi, H. R., Vogels, G. D., et al. (2002). The major component of the cellulosomes of anaerobic fungi from the genus piromyces is a family 48 glycoside hydrolase. DNA Sequen. 13, 313–320. doi: 10.1080/1042517021000024191
Teller, D. C., Behnke, C. A., Pappan, K., Shen, Z., Reese, J. C., Reeck, G. R., et al. (2014). The structure of rice weevil pectin methylesterase. Acta Crystallogr. F Struct. Biol. Commun. 70, 1480–1484. doi: 10.1107/S2053230X14020433
Tzi-Yuan, W., Chen, H.-L., Lu, M.-Y. J., Chen, Y.-C., Sung, H.-M., Mao, C.-T., et al. (2011). Functional characterization of cellulases identified from the cow rumen fungus Neocallimastix patriciarum W5 by transcriptomic and secretomic analyses. Biotechnol. Biofuels 4:24. doi: 10.1186/1754-6834-4-24
Untergasser, A., Cutcutache, I., Koressaar, T., Ye, J., Faircloth, B. C., Remm, M., et al. (2012). Primer3–new capabilities and interfaces. Nucleic Acids Res. 40:e115. doi: 10.1093/nar/gks596
Vega, F. E., Brown, S. M., Chen, H., Shen, E., Nair, M. B., Ceja-Navarro, J. A., et al. (2015). Draft genome of the most devastating insect pest of coffee worldwide: the coffee berry borer, Hypothenemus hampei. Sci. Rep. 5:12525.
Watanabe, H., Noda, H., Tokuda, G., and Lo, N. (1998). A cellulase gene of termite origin. Nature 394, 330–331. doi: 10.1038/28527
Watanabe, H., and Tokuda, G. (2010). Cellulolytic systems in insects. Annu. Rev. Entomol. 55, 609–632. doi: 10.1146/annurev-ento-112408-085319
Willis, J. D., Grant, J. N., Mazarei, M., Kline, L. M., Rempe, C. S., Collins, A. G., et al. (2017). The TcEG1 beetle (Tribolium castaneum) cellulase produced in transgenic switchgrass is active at alkaline pH and auto-hydrolyzes biomass for increased cellobiose release. Biotechnol. Biofuels 10:230.
Yang, Y., Ren, L., Wang, T., Xu, L., and Zong, S. (2017). Comparative morphology of sensilla on antenna, maxillary palp and labial palp of larvae of Eucryptorrhynchus scrobiculatus(Olivier) and E. brandti (Harold) (Coleoptera: Curculionidae). Acta Zool. 98, 400–411. doi: 10.1111/azo.12185
Yu, Q. Q., Liu, Z. K., Chen, C., and Wen, J. (2013). Antennal sensilla of Eucryptorrhynchus chinensis (Olivier) and Eucryptorrhynchus brandti (Harold) (Coleoptera: Curculionidae). Microsc. Res. Tech. 76, 968–978. doi: 10.1002/jemt.22256
Zhang, H., Yohe, T., Huang, L., Entwistle, S., Wu, P., Yang, Z., et al. (2018). dbCAN2: a meta server for automated carbohydrate-active enzyme annotation. Nucleic Acids Res. 46, W95–W101.
Keywords: carbohydrate esterase, cellulase, glycoside hydrolase, hemicellulase, pectinase, polysaccharide lyase, transcriptome
Citation: Gao P, Liu Z and Wen J (2020) Expression Profiling of Plant Cell Wall-Degrading Enzyme Genes in Eucryptorrhynchus scrobiculatus Midgut. Front. Physiol. 11:1111. doi: 10.3389/fphys.2020.01111
Received: 28 May 2020; Accepted: 11 August 2020;
Published: 04 September 2020.
Edited by:
Jose Eduardo Serrão, Universidade Federal de Viçosa, BrazilReviewed by:
Matan Shelomi, National Taiwan University, TaiwanRatnasri Pothula, The University of Arizona, United States
Copyright © 2020 Gao, Liu and Wen. This is an open-access article distributed under the terms of the Creative Commons Attribution License (CC BY). The use, distribution or reproduction in other forums is permitted, provided the original author(s) and the copyright owner(s) are credited and that the original publication in this journal is cited, in accordance with accepted academic practice. No use, distribution or reproduction is permitted which does not comply with these terms.
*Correspondence: Junbao Wen, d2VuamJAYmpmdS5lZHUuY24=