- 1Department of Biological Sciences, Kongju National University, Kongju, South Korea
- 2Department of Applied Bioresource Science, National Marine Biodiversity Institute of Korea, Seocheon, South Korea
Cold weather is one of the biggest challenges in establishing a large-scale microalgae culture facility in temperate regions. In order to develop a strain that is resistant to low temperatures and still maintains high photosynthetic efficiency, transgenic studies have been conducted targeting many genes. Early light-inducible proteins (ELIPs) located in thylakoid membranes are known to protect photosynthetic machinery from various environmental stresses in higher plants. An ELIP homolog was identified from Chlamydomonas reinhardtii and named ELIP3. The role of the gene was analyzed in terms of photosynthetic CO2 assimilation under cold stress. Western blot results showed a significant accumulation of ELIP3 when the cells were exposed to cold stress (4°C). High light stress alone did not induce the accumulation of the protein. Enhanced expression of ELIP3 helped survival of the cell under photo-oxidative stress. The influx of CO2 to the photobioreactor induced strong accumulation of ELIP3, and enhanced survival of the cell under high light and cold stress. When the oxidative stress was reduced by adding a ROS quencher, TEMPOL, to the media the expression of ELIP3 was reduced. A knockdown mutant showed much lower photosynthetic efficiency than wild type in low temperature, and died rapidly when it was exposed to high light and cold stress. The overexpression mutant survived significantly longer in the same conditions. Interestingly, knockdown mutants showed negative phototaxis, while the overexpression mutant showed positive phototaxis. These results suggest that ELIP3 may be involved in the regulation of the redox state of the cell and takes important role in protecting the photosystem under photooxidative stress in low temperatures.
Introduction
Exposure to high light and cold stress causes the accumulation of reactive oxygen species (ROS) in plant cells, and plants have evolved various ROS scavenging machineries (Caverzan et al., 2012; Choudhury et al., 2013). Some plants also actively produce ROS and use it as a cell signaling molecule for development or defense against pathogen attack (Im et al., 2019). Most ROS in plant cells are generated by electrons originating from photosystem I (PSI), which generates superoxide anion (O2–) from molecular oxygen followed by the increase of hydrogen peroxide (H2O2) by superoxide dismutase (SOD) activity (Apel and Hirt, 2004; Asada, 2006). In low temperature, the functioning of the Calvin cycle declines, in part, due to a decrease in the activity of the CO2 assimilation enzyme ribulose-1,5-bisphosphate carboxylase/oxygenase (Rubisco) (Allen and Ort, 2001; Hirotsu et al., 2004; Zhou et al., 2004; Jiang et al., 2013; Gan et al., 2019). The limitation of photosynthetic CO2 fixation causes over-reduction of the photosynthetic electron transport chain and promotes singlet oxygen (1O2) generation by excited triplet state of chlorophyll in the antenna, as well as the reaction center of photosystem II (PSII) (Krieger-Liszkay et al., 2008). ROS generated in chloroplasts is usually scavenged by the water-water cycle (Asada, 1999), but ROS overproduction due to a combination of high light and low temperature can inhibit the turnover of the D1 protein of the PSII reaction center and lead to photoinhibition (Powles, 1984; Allen and Ort, 2001; Choi et al., 2002; Takahashi and Murata, 2006, 2008). In addition, overproduction of ROS in low temperature not only leads to oxidative inactivation of Calvin cycle enzymes, but also irreversible oxidative damage to chloroplast, and consequently severely inhibits photosynthesis and growth of plants (Kaiser, 1976; Tanaka et al., 1982).
Microalgae are biological resources that can produce not only renewable fuel, but also various products such as feedstock, food, materials, and useful chemicals (Zhu, 2015; Ruiz et al., 2016). Microalgae are considered as an optimal biomass producer as they can consume less water, and arable land, than land crops, but large-scale microalgal production is mostly carried out in tropical or sub-tropical locations where strains are grown at water temperatures between 15–30°C as growth is inhibited at low temperatures (Spolaore et al., 2006; Chisti, 2007). For microalgal production in cold climates, photobioreactor housed within greenhouses with additional heating systems are necessary (Baliga and Powers, 2010; Laamanen et al., 2014). Development of genetically engineered strains with reduced energy consumption has been extensively exploited for the optimal year-round production of various microalgae (Rosenberg et al., 2008; Radakovits et al., 2010; Apel and Weuster-Botz, 2015; Kotchoni et al., 2016). However, little is known of the genes involved in low temperature tolerance in microalgae.
Early light-inducible proteins (ELIPs) belong to the light-harvesting complex (LHC)-like proteins with chlorophyll-binding motifs, which has been extensively studied in land plants as a target gene to enhance tolerance against high light stress (Grimm and Kloppstech, 1987; Adamska, 2001; Heddad and Adamska, 2002; Engelken et al., 2012). In land plants, ELIPs were induced by light stress (high light, blue light, UV-A), and in a light intensity-dependent manner, controlled by the blue/UV light photoreceptor cryptochrome1 (CRY1) (Adamska et al., 1992a, b; Kleine et al., 2007). ELIP1 and ELIP2 of Arabidopsis thaliana are associated with the monomeric and trimeric major LHCb antenna of PSII and are considered to have a protective function for chloroplast from photooxidative stress under high light (Hutin et al., 2003; Heddad et al., 2006). However, in a study using elip1/elip2 mutants, the possibility that ELIPs modulate the xanthophyll cycle and have a photo-protective function only in severe stress conditions was proposed (Rossini et al., 2006). In the same vein, ELIPs were most highly expressed when photooxidative stress was aggravated due to combined stresses of high light and low temperature (Shimosaka et al., 1999; Fowler and Thomashow, 2002; Casazza et al., 2005; Han and Kim, 2013).
Chlamydomonas reinhardtii is one of the major target species for biomass production, but year-round production is limited in temperate climates as photosynthetic capacity decreases below 15°C and cell death occurs at 3°C (Hema et al., 2007; Kotchoni et al., 2016; Kwak et al., 2017). Among 10 ELIP homologs reported in C. reinhardtii, only ELIP3 has the conserved LHC motifs on the transmembrane domains and shows light-induced expression (Elrad and Grossman, 2004; Teramoto et al., 2004; Zones et al., 2015; Rochaix and Bassi, 2019). In this study, we characterized the function of ELIP3 in photooxidative stress conditions and evaluated the utility of the gene as a target for the development of low temperature-tolerant strains.
Materials and Methods
Algal Culture Conditions and Stress Treatment
Chlamydomonas reinhardtii CC-125 originating from the Chlamydomonas Resource Center1 was used as the wild type (WT). WT and transgenic strains were cultured in Tris–acetate-phosphate (TAP) medium and agar plates (Harris., 2009) at 23°C under 50 μmol photons m–2 s–1 (12 L: 12 D). White fluorescent lamps (Osram FL-60 SW, South Korea), high-light LED (CR-PAR30, CR-LED, China) and UV lamps (G15T8E; F15T8BL, Sankyo Denki, Japan) were used for light conditions. Culture chambers (Vision Biotech, South Korea) at each temperature (4, 10, 23°C) were used for temperature experiments. C. reinhardtii were exposed to various combinations of irradiance and temperature at a cell concentration of 5 × 106 cells/ml. CO2 experiments were performed using a custom-made photobioreactor with a water-jacket (Pyrex 500 ml, Scilab, South Korea) for low temperature. For CO2 treatments, high quality 5% CO2 was obtained from Special Gas Company (Daejeon, South Korea). CO2 experiments were performed following a previously described method (Han and Kim, 2013).
Western Blot Analysis
Western blotting was performed to analyze the accumulation of ELIP3 under stress conditions. C. reinhardtii (107 cells) was collected using centrifugation at 11,000 g for 10 min. The pellet was resuspended with 1× Laemmli buffer (S3401, Sigma-Aldrich, United States) and incubated at 100°C for 5 min, then centrifuged at 11,000 g for 10 min to obtain the supernatant. The supernatant was loaded onto 12% (w/v) SDS-polyacrylamide gels and transferred to polyvinylidene fluoride (PVDF) membranes (Bio-Rad, United States) using a Mini Trans–Blot® Cell (Bio-Rad, United States). The membrane was blocked with TBS buffer (Tris–buffered saline) with 5% skim milk and 0.1% Tween 20 with agitation. For the detection of ELIP3 protein, we developed rabbit polyclonal antibodies raised against the antigenic sequence, FGKSYTPEEWEKEVASGAF derived from the ELIP3 (XM_001694629) sequence (Ab Frontier, South Korea). The blots with expected protein size were incubated in primary Anti-ATPβ (loading control, 1:3000, Agrisera, Sweden), Anti-psbA (D1 protein) (1:5000, Agrisera, Sweden), or Anti-ELIP3 (1:2000) at 4°C for 12 h. We used less diluted Anti-ELIP3 (1:1500) when we perform western blotting at 10 and 23°C to enhance sensitivity. Then, the blots were incubated with horseradish peroxidase (HRP)-conjugated goat anti-rabbit secondary antibodies (Ab Frontier, South Korea) for 1 h. Protein levels were detected using a chemiluminescence kit (Amersham, United Kingdom). The relative protein levels were quantified using Quantity One software (Bio-Rad, United States) and normalized by ATPβ levels.
Generation of Transgenic Strains
For the overexpression strain of ELIP3, a cloned coding region of ELIP3 was inserted into the pChlamy_3/D-TOPO vector (Invitrogen, United States) (Supplementary Figure S1). The linearized plasmid, using the restriction enzyme ScaI, was transformed into the CC-125 strain by GenePulser Xcell electroporation system (Bio-Rad, United States). For the RNAi expression vector, the partial coding region (314 bp) of ELIP3 was amplified from cDNA using the specific primers ELIP3_RNAi-F and ELIP3_RNAi-R (Supplementary Table 1). The fragment of ELIP3 was added PstI and XbaI restriction enzyme sites in the 5′ end and BamHI and HindIII restriction enzyme sites in the 3′ end by the extra base pairs on the 5′ end of the primers. Using these enzyme restriction sites, fragment was ligated sequentially to Spacer (Rohr et al., 2004) and pCr102 vector (Kim et al., 2011), which generated a hairpin RNA construct from the 3′ UTR of aph7” (Supplementary Figure S1). The constructed vector was then transformed into CC-125 by electroporation after linearization using KpnI. Electroporated cells were recovered overnight in TAP medium containing 40 mM of sucrose, with agitation (120 rpm) under dim light (Shin et al., 2016). The transgenic strains were selected from TAP agar plates containing hygromycin B (15 mg/l).
Treatment of Redox Reagents
50 ml (5 × 106 cells/ml) of C. reinhardtii cells were centrifuged, and the pellets were resuspended in each reagent containing TAP media. Hydrogen peroxide (H2O2, Sigma-Aldrich, United States), norflurazon (NF, Sigma-Aldrich, United States) and 4-hydroxy-2, 2, 6, 6-tetramethylpiperidine 1-oxyl (TEMPOL, Sigma-Aldrich, United States) were used as the redox reagents.
Measurement of Chlorophyll Fluorescence Parameters
To measure the photosynthetic efficiency of WT and transgenic strains at different temperatures a previously described method was used (Han and Kim, 2013). Chlorophyll fluorescence measurements were made using a PHYTO-PAM (Walz, Germany). The C. reinhardtii cells (5 × 106 cells/ml) of each strain were incubated at 23 or 4°C in the light intensity of 50 μmol photons m–2 s–1 and 1 ml was harvested for calculation of fluorescence parameters. The effective and maximum quantum yields, and rapid light curves (RLC) were measured at designated times after exposure (Ralph and Gademann, 2005). RLCs were constructed based on nine actinic increasing light levels (0, 16, 64, 128, 192, 320, 512, 832, 1088, 1344 μmol photons m–2 s–1). The effective and maximum quantum yield of PSII were calculated as ΦPSII = ΔF/Fm′ = (Fm′-F)/Fm′ and Fv/Fm = (Fm-F0)/Fm, respectively. Fm′ and F values were measured in light incubated strains, and Fm and F values were measured by dark-adaptation for 10 min (Ralph and Gademann, 2005). The relative electron transport rate (rETR) was calculated as ETR = 0.84 × 0.5 × ΦPSII × light intensity for each light level. Due to the occurrence of Fm′ values higher than the Fm value measured after dark-adaptation, non-photochemical quenching (NPQ) values were calculated as NPQ = (Fm′max-Fm′)/Fm′ (Serôdio et al., 2007).
Quantitative PCR and Northern Blot Analysis of ELIP3
Total RNA was isolated using the RNeasy Plant Mini Kit (Qiagen, Germany). The RNA concentration was determined using a spectrophotometer (MaestroNano, MaestroGen, Taiwan) and its integrity was assessed by 1.2% agarose-formaldehyde gel electrophoresis. First strand cDNAs were synthesized using Accuscript High Fidelity 1st strand cDNA kit (Agilent Technologies, United States). Real-time quantitative PCR was performed using QuantiSpeed SYBR Hi-Rox Kit (PhileKorea, South Korea) in a StepOnePlus Real-Time PCR System (Applied Biosystems, United States) as described previously (Lee and Kim, 2019). The primers used for qPCR are shown in Supplementary Table S1. The housekeeping gene 18S rRNA was used as an endogenous internal control to normalize gene expression. For northern blot analysis of ELIP3, the DNA probe was directly amplified and labeled with DIG-dUTP by PCR of the ELIP3 genes from cDNA using the DIG probe Synthesis Kit (Roche, Germany). The primers used for probe synthesis are shown in Supplementary Table S1. Northern blotting was performed as described previously (Han and Kim, 2013). The relative mRNA levels were quantified using Quantity One software (Bio-Rad, United States) and normalized by band intensities of rRNA.
Statistical Analysis
Statistical analysis was performed with GraphPad Prism 8 software (GraphPad Software, United States). The Shapiro-Wilk test was used to determine the distribution of the data. Statistical significance was determined by one-way Welch’s ANOVA with Dunnett’s T3 post hoc test or two-way ANOVA with Bonferroni’s post hoc test for multiple comparisons. In some cases, the two-tailed unpaired Student’s t-test (with Welch’s correction) was used for comparing two groups. Statistical significance was accepted when P < 0.05.
Results
Accumulation of ELIP3 in Response to Light and Low Temperature
Low temperature was found to enhance ELIP3 protein accumulation. For cells maintained in the dark, ELIP3 protein abundance was elevated after 12 h exposure to 4°C (Figures 1A,C). Further, ELIP3 protein abundance was elevated after 12 h exposure to light when the cells were incubated at both 10 and 4°C (Figures 1B,C). Northern blot analysis also showed a low temperature-dependent expression of ELIP3 (Figures 1D,E). There was a time delay between the expression of ELIP3 mRNA and accumulation of the protein. The expression of ELIP3 was detected at 3 h after incubation at 4°C and gradually increased over time (Figures 1E,F), while the accumulation of the protein was observed from 6 h in light and from 9 h in the dark (Figures 2A,B). Once ELIP3 accumulated in light for 12 h, the amount of protein did not change even after the cells were transferred to dark for 12 h (Figure 2C). The accumulation of ELIP3 significantly increased from 21 h after exposure to light (Figures 2C,D).
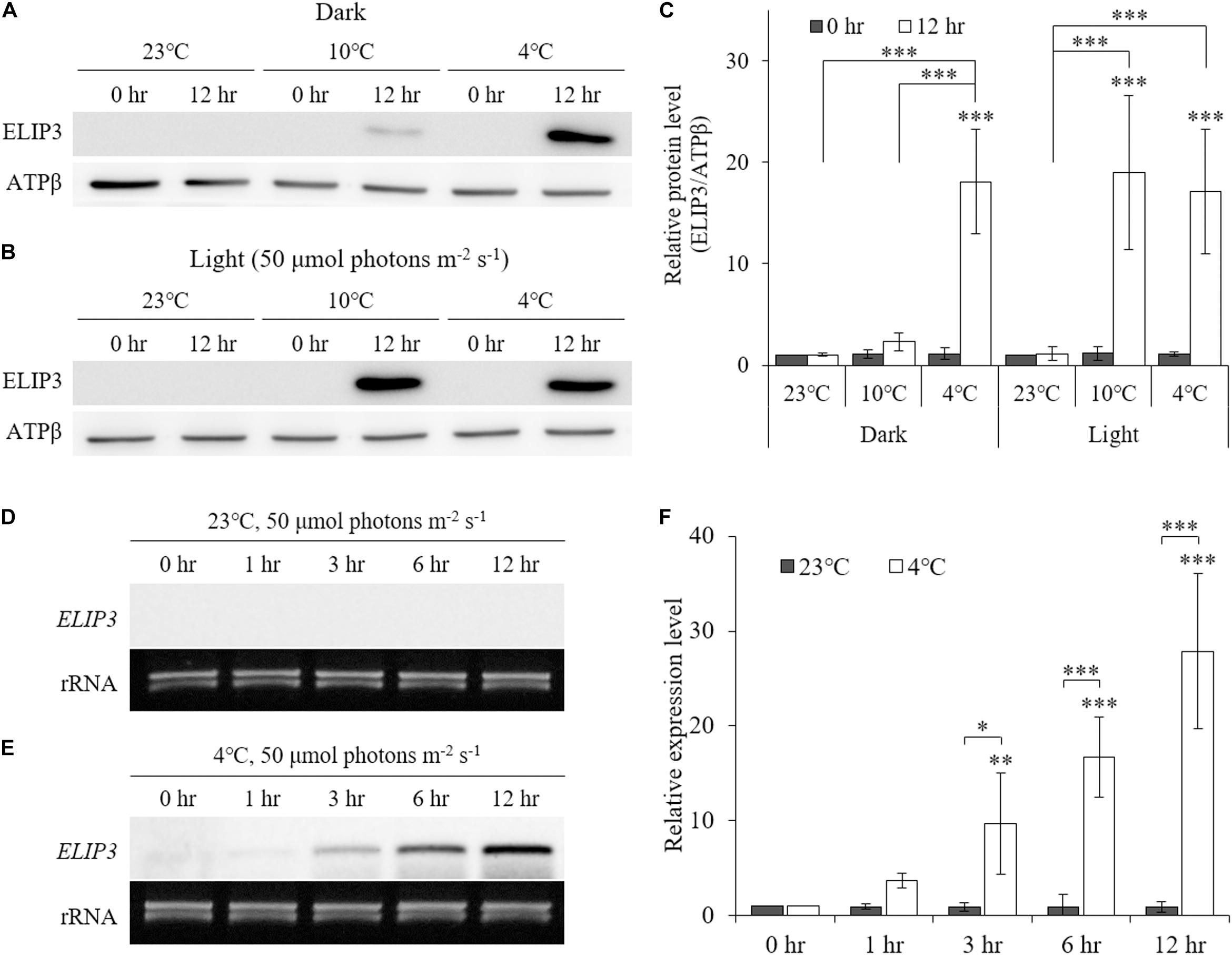
Figure 1. Western blot (A, B) and Northern blot (D, E) analysis showing accumulation of ELIP3 in different temperature regimes (23, 10, and 4°C). (A) Accumulation in the dark. (B) Enhanced accumulation in light (50 μmol photons m–2 s–1). (C) Densitometry of western blot results. Data are expressed as mean ± SD (two-way ANOVA with Bonferroni’s multiple comparisons test, n = 4). (D) Expression of ELIP3 at 23°C. (E) Expression of ELIP3 at 4°C. (F) Densitometry of northern blot results. Data are expressed as mean ± SD (two-way ANOVA with Bonferroni’s multiple comparisons test, n = 4). ATPβ was used as a loading control for western blotting, and rRNA for northern blotting. ∗P < 0.05, ∗∗P < 0.01, ∗∗∗P < 0.001.
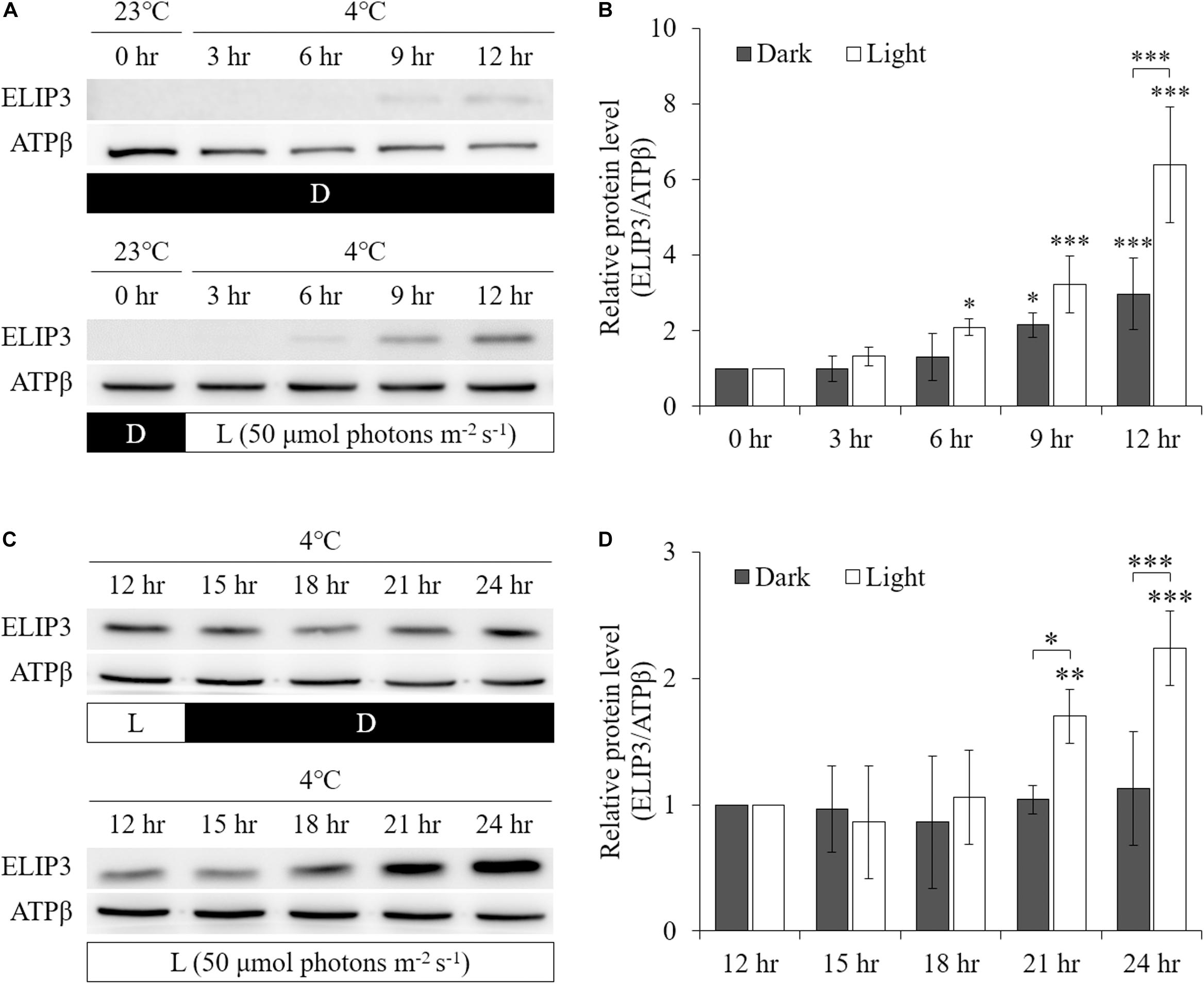
Figure 2. Western blot analysis showing accumulation of ELIP3 in response to low temperature (4°C) in dark and light (50 μmol photons m–2 s–1) conditions. (A) In dark, the accumulation started at 9 h. In light condition, the accumulation was stronger and started earlier at 6 h. (B) Densitometry of western blots in (A). Data are expressed as mean ± SD (two-way ANOVA with Bonferroni’s multiple comparisons test, n = 3). (C) Transfer from light to dark or continuous light after 12 h of preincubation in light. Enhanced accumulation of ELIP3 in continuous exposure to light. (D) Densitometry of western blots in (C). Data are expressed as mean ± SD (two-way ANOVA with Bonferroni’s multiple comparisons test, n = 3). ATPβ was used as a loading control. ∗P < 0.05, ∗∗P < 0.01, ∗∗∗P < 0.001.
UV–A irradiation increased ELIP3 protein abundance after 1 h at 23°C. Further, at 4°C, 3 h of UV–A irradiation markedly elevated ELIP3 protein abundance (Figures 3A,B). At warm temperature, the expression of D1, a reaction core protein of PSII, was decreased after 1 h of UV-A irradiation, while at 4°C, the expression of D1 was decreased after 5 h of UV-A irradiation (Figures 3A,B). UV-B irradiation did not induced accumulation of ELIP3 because of rapid cell death in this condition (Figures 3C,D).
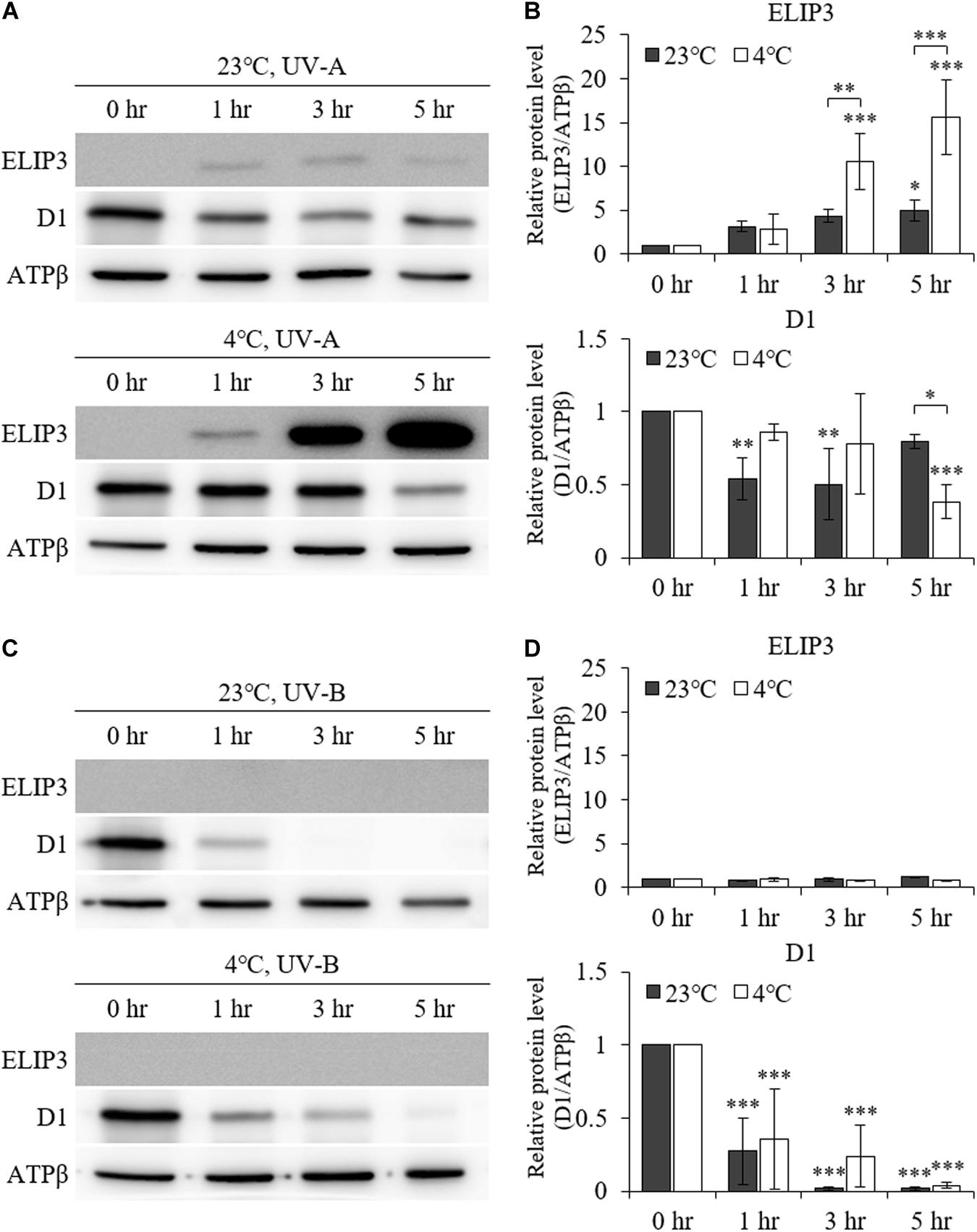
Figure 3. Western blot analysis under UV irradiation. (A) Accumulation of ELIP3 and D1 in UV–A irradiation. (B) Densitometry of western blots in (A). Data are expressed as mean ± SD (two-way ANOVA with Bonferroni’s multiple comparisons test, n = 3). (C) Accumulation of ELIP3 and D1 in UV-B irradiation. (D) Densitometry of western blots in (C). Data are expressed as mean ± SD (two-way ANOVA with Bonferroni’s multiple comparisons test, n = 3). ATPβ was used as a loading control. ∗P < 0.05, ∗∗P < 0.01, ∗∗∗P < 0.001.
The accumulation of ELIP3 was not induced in warm temperature even in very high light, 1000 μmol photons m–2 s–1, while accumulation of D1 was observed at the same condition (Figures 4A–C). When the cells were exposed to extreme high light (1,000 μmol photons m–2 s–1) at 4°C the cells lost color and began to die in 12 h (Figure 4D, left lane). ELIP3 and D1 protein disappeared at this time while the control protein ATPβ was still present (Figures 4A,C). When 5% CO2 gas was influxed to photobioreactor at the same condition cells survived much longer and chloroplasts managed to keep their color for 24 h (Figure 4D, right lane). Interestingly, the accumulation of ELIP3 was significantly enhanced with CO2 influx while the accumulation of D1 protein did not change much (Figures 4B,C). The effect of CO2 influx was not observed when the cells were exposed to moderate light, 50 μmol photons m–2 s–1, at 4°C (Supplementary Figure S2).
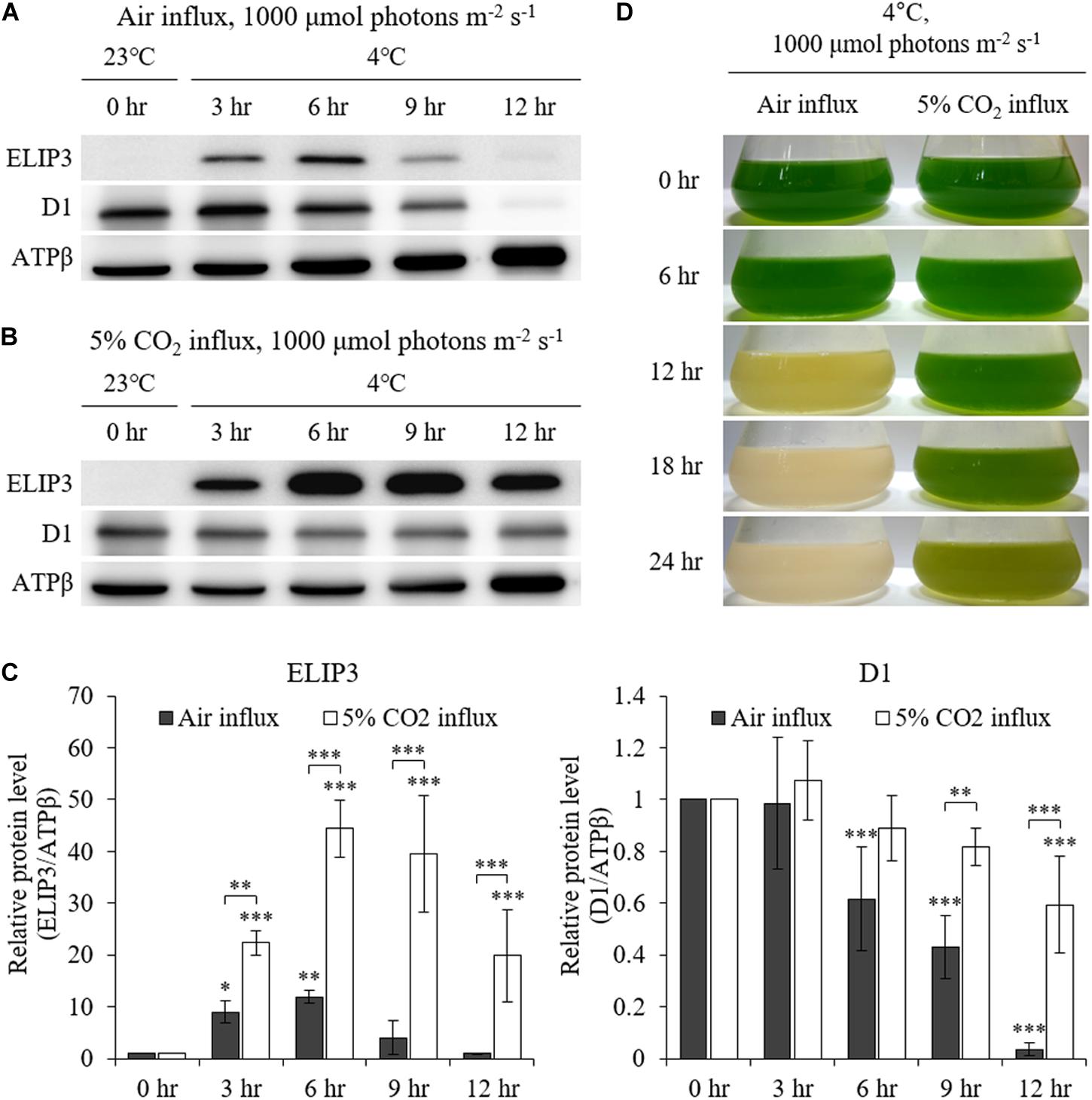
Figure 4. Western blot analysis of C. reinhardtii with 5% CO2 supply at low temperature (4°C) and high light (1,000 μmol photons m–2 s–1) using a photobioreactor. (A) Accumulation of ELIP3 and D1 in air flux condition. (B) Accumulation of ELIP3 and D1 with 5% CO2 supply. ATPβ was used as a loading control. (C) Densitometry of western blots in (A) and (B). Data are expressed as mean ± SD (two-way ANOVA with Bonferroni’s multiple comparisons test, n = 3). (D) The response of C. reinhardtii cells to low temperature and high light with or without added CO2 supply. ∗P < 0.05, ∗∗P < 0.01, ∗∗∗P < 0.001.
The accumulation of ELIP3 and D1 protein in low temperature was affected by the treatment of redox reagents. When oxidative stress was enforced by H2O2 and carotenoid biosynthesis inhibitor, norflurazon (NF) treatment the accumulation of ELIP3 was enhanced in low temperature at 15 h after the treatments, but treatment with the ROS quencher, TEMPOL, completely blocked accumulation of ELIP3 for 24 h (Figures 5A,B). The accumulation of D1 protein was not affected by H2O2 treatment, instead it reduced gradually after TEMPOL treatment (Figure 5B).
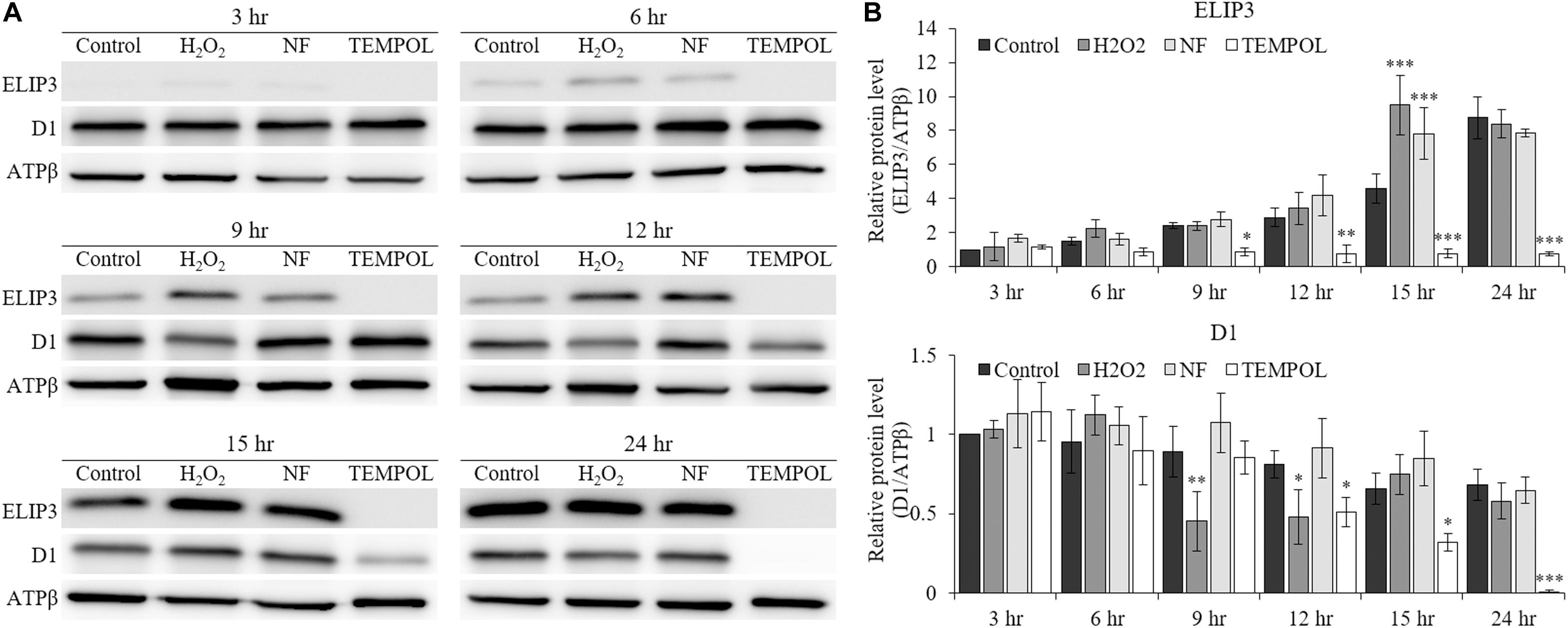
Figure 5. Western blot analysis showing effect of redox reagents on ELIP3 and D1 accumulation over time. (A) The cells were treated with H2O2 (50 μM), norflurazon (NF, 0.5 μM) and ROS quencher TEMPOL (20 mM) under low temperature (4°C, 50 μmol photons m–2 s–1). (B) Densitometry of western blots. Data are expressed as mean ± SD (two-way ANOVA vs control with Bonferroni’s multiple comparisons test, n = 3). ATPβ was used as a loading control. ∗P < 0.05, ∗∗P < 0.01, ∗∗∗P < 0.001.
Characterization of Transgenic Mutants
We produced overexpression (OX) and knockdown (RNAi) mutants of ELIP3. Real-time PCR results showed that the expression of ELIP3 was significantly higher in the OX mutant, in warm temperature, and much lower in RNAi strains than WT at 4°C (Figure 6A). Cell-blot assay of the mutants showed a significantly enhanced growth and viability in the OX strain compared to those in WT at low temperature (10°C), while the RNAi strains grew much slower and died earlier than WT in the same condition (Figure 6B).
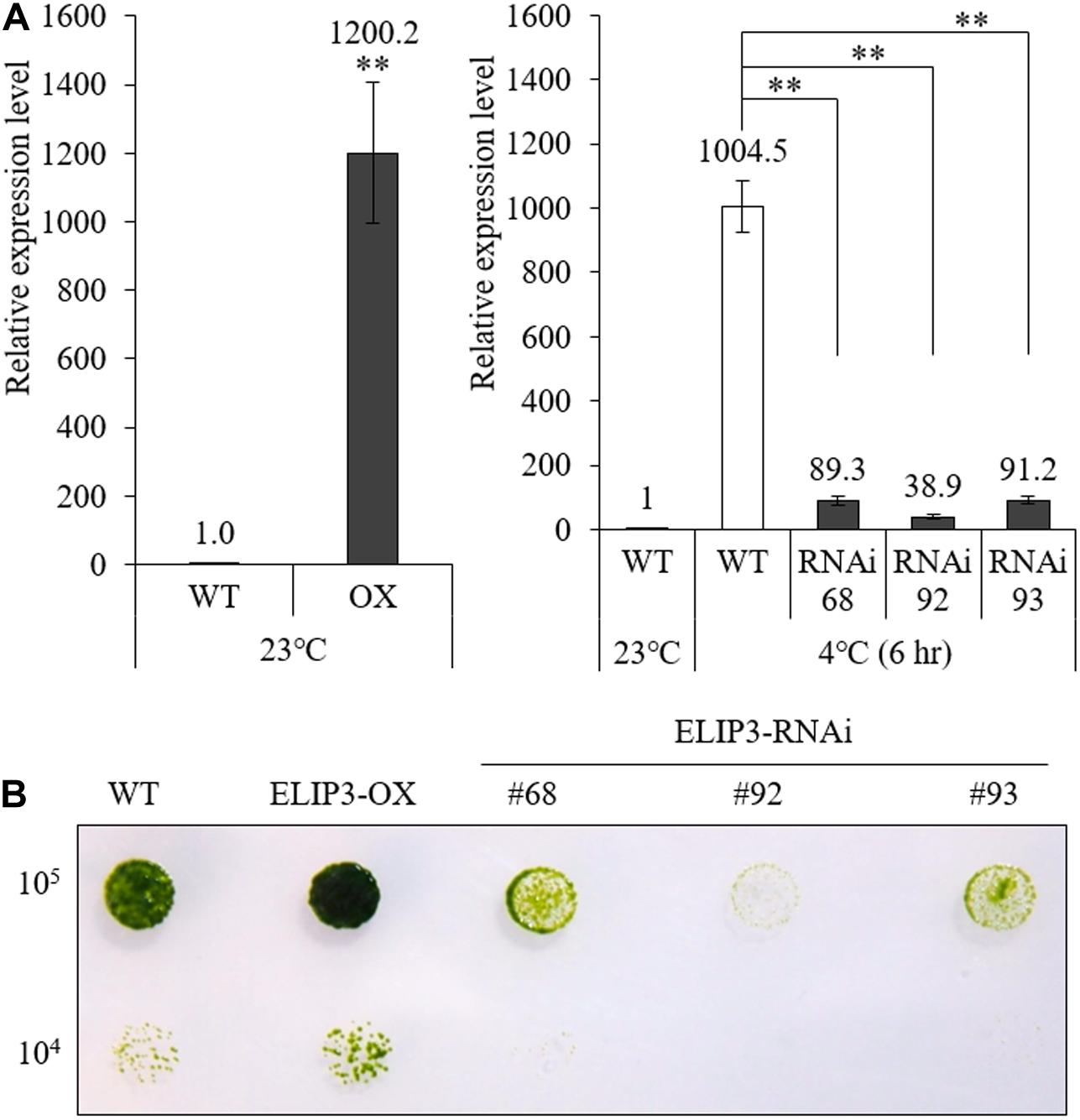
Figure 6. Expression of ELIP3 in transgenic strains. (A) Transcription levels in ELIP3 overexpression strain (OX) and knockdown strains (RNAi). Data are expressed as mean ± SD. (t-test and one-way ANOVA, ∗∗P < 0.01, n = 3). (B) Viability analysis using spot assay with TAP agar plate under low temperature. Cells of each strain (105 cells/ml, 104 cells/ml) were dispensed in the TAP agar plate and incubated at 10°C for 30 days.
Western blot analysis showed almost no accumulation of ELIP3 in the knockdown mutant, RNAi 92, at 4°C, 1000 μmol photons m–2 s–1 (Figures 7A,B). The quantitative densitometry showed a decrease of D1 levels in RNAi 92 compared to WT (Figure 7C). In static culture, the knockdown mutant began to lose pigment in less than 3 h at this condition and began to die in 9 h, while WT cultures were still green (Figure 7D).
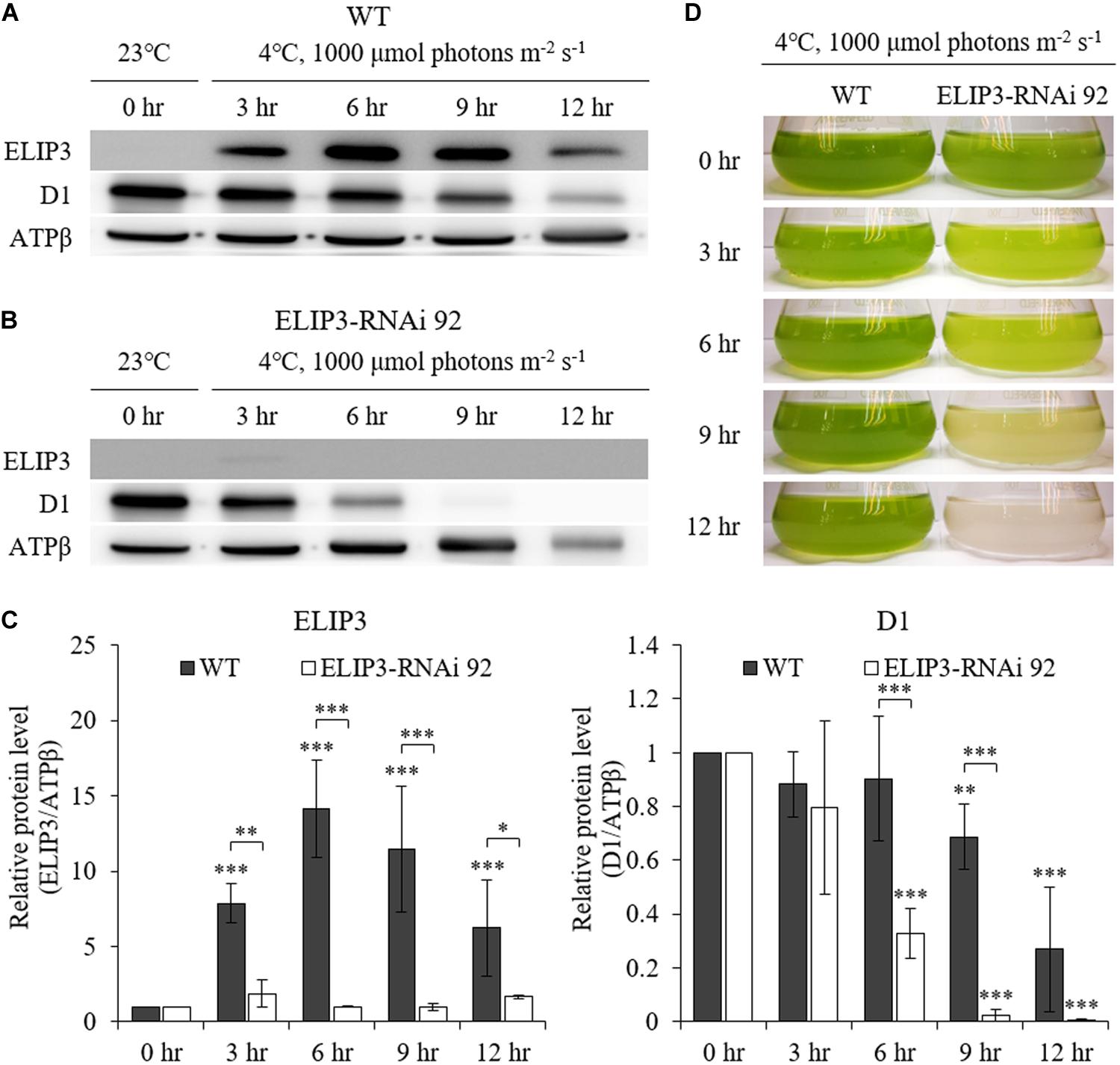
Figure 7. Characterization of ELIP3 knockdown strain RNAi 92 at low temperature (4°C) and high light (1,000 μmol photons m–2 s–1) conditions. (A) Western blot analysis of ELIP3 and D1 in WT and (B) RNAi 92. ATPβ was used as a loading control. (C) Densitometry of western blots in (A,B). Data are expressed as mean ± SD (two-way ANOVA with Bonferroni’s multiple comparisons test, n = 3). (D) The response of WT and RNAi 92 strain to low temperature and high light in static culture condition. ∗P < 0.05, ∗∗P < 0.01, ∗∗∗P < 0.001.
The knockdown mutant, RNAi 92, showed lower values of effective quantum yield and maximum quantum yield compared to WT and OX strains initially in warm temperature, but the difference was recovered over time (Figure 8A), and the difference became significantly larger at 4°C (Figure 8B). The OX mutant showed the highest values at 4°C followed by WT. The effective and maximum quantum yield of knockdown mutant decreased more rapidly compared to WT and OX only for first 3 h (Figure 8B). When the samples were transferred back to 23°C, after 12 h, both quantum yield recovered in all strains (Figure 8). ETR and NPQ in photosynthetically active radiation (PAR) showed similar increasing curves in all strains at 23°C (Figure 9A). ETR saturation was reached much faster at 4°C, especially in the RNAi 92 strain (Figure 9B).
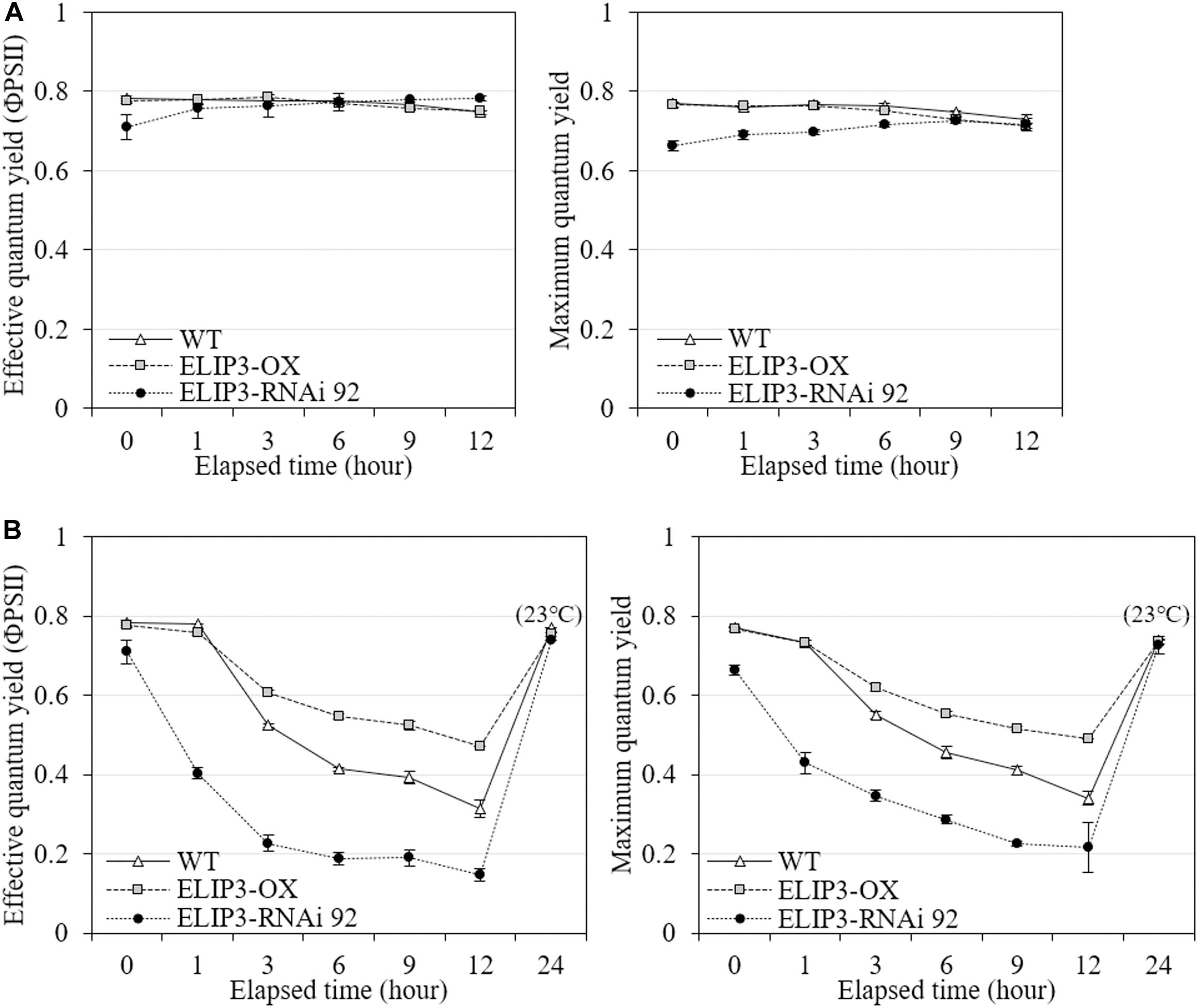
Figure 8. Effective quantum yield and maximum quantum yield measurements. Comparison of effective and maximum quantum yields from fluorescence measurement between WT, overexpression strain (OX) and knockdown strain (RNAi 92) at (A) 23°C and (B) 4°C. The strains measured at 4°C were incubated at 23°C under dark condition for 12 h, then recovery measured. Values represent the mean ± SD (n = 3).
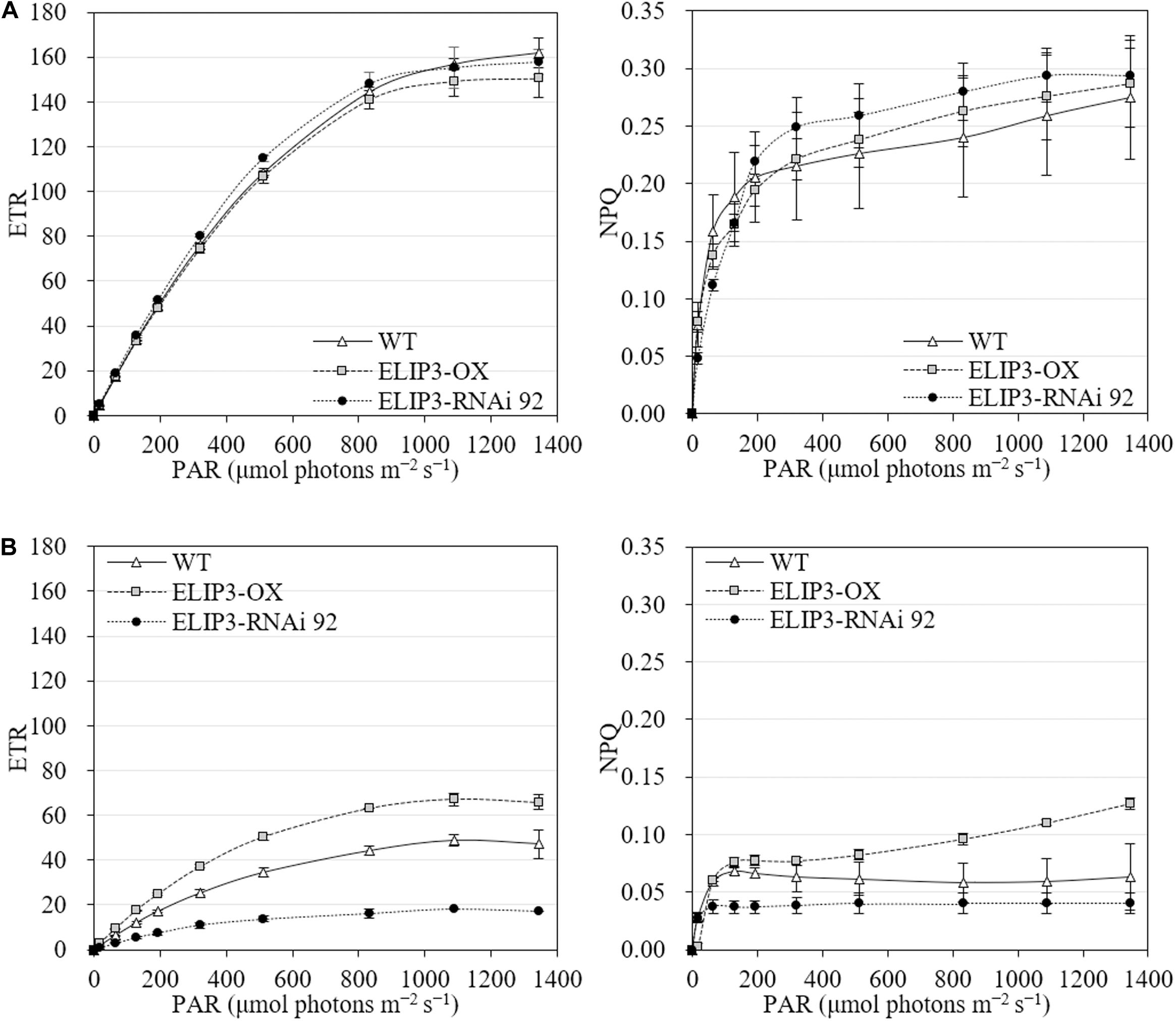
Figure 9. Electron transport rate (ETR) and non-photochemical quenching (NPQ) at different light levels for WT, overexpression strain (OX) and knockdown strain (RNAi 92). (A) 23°C. (B) 4°C. The values were measured after 12 h in each condition. Values represent the mean ± SD (n = 3).
The transgenic mutants of ELIP3 showed a different phototaxis pattern. When knockdown mutant, RNAi 92, were exposed to unidirectional light, 50 μmol photons m–2 s–1 at 23°C, the cells showed negative phototaxis (Supplementary Video S1), while OX cells showed positive phototaxis like WT cells (Supplementary Video S2).
Discussion
For optimal year-round production of microalgae in cold climates, development of a cold-tolerant strain is essential. The goal is to develop strains that grow well rather than simply survive in cold climates. In order to secure the growth rate of the cold-resistant strain, the genetic engineering of the strains has been extensively studied and some genes have been exploited as targets to maintain photosynthetic efficiency at low temperatures (e.g., Kotchoni et al., 2016). Our results suggest that ELIP3 in C. reinhardtii may be a useful target gene to achieve these goals. The results from transgenic mutants showed that the overexpression of ELIP3 not only enhances survival of cells, but also protects cells, to maintain photosynthetic efficiency in low temperature. Our results using redox reagents and CO2 influx suggested that cellular ROS generated in photooxidative stress may trigger accumulation of ELIP3. The expression of ELIP3 was significantly reduced when the oxidative stress was relieved with the treatment of antioxidant, TEMPOL, while the treatment with norflurazon or H2O2, the accumulation of ELIP3 was partially enhanced. CO2 influx to the media enhanced ELIP3 accumulation and relieved the limitation of CO2 assimilation in low temperature (Figure 10). Western blot analysis using antibody for the D1 protein suggested that ELIP3 is involved in mitigation of photooxidative stress in PSII also. These results suggest that ELIP3 of C. reinhardtii is involved in the mitigation of photooxidative stress at low temperature.
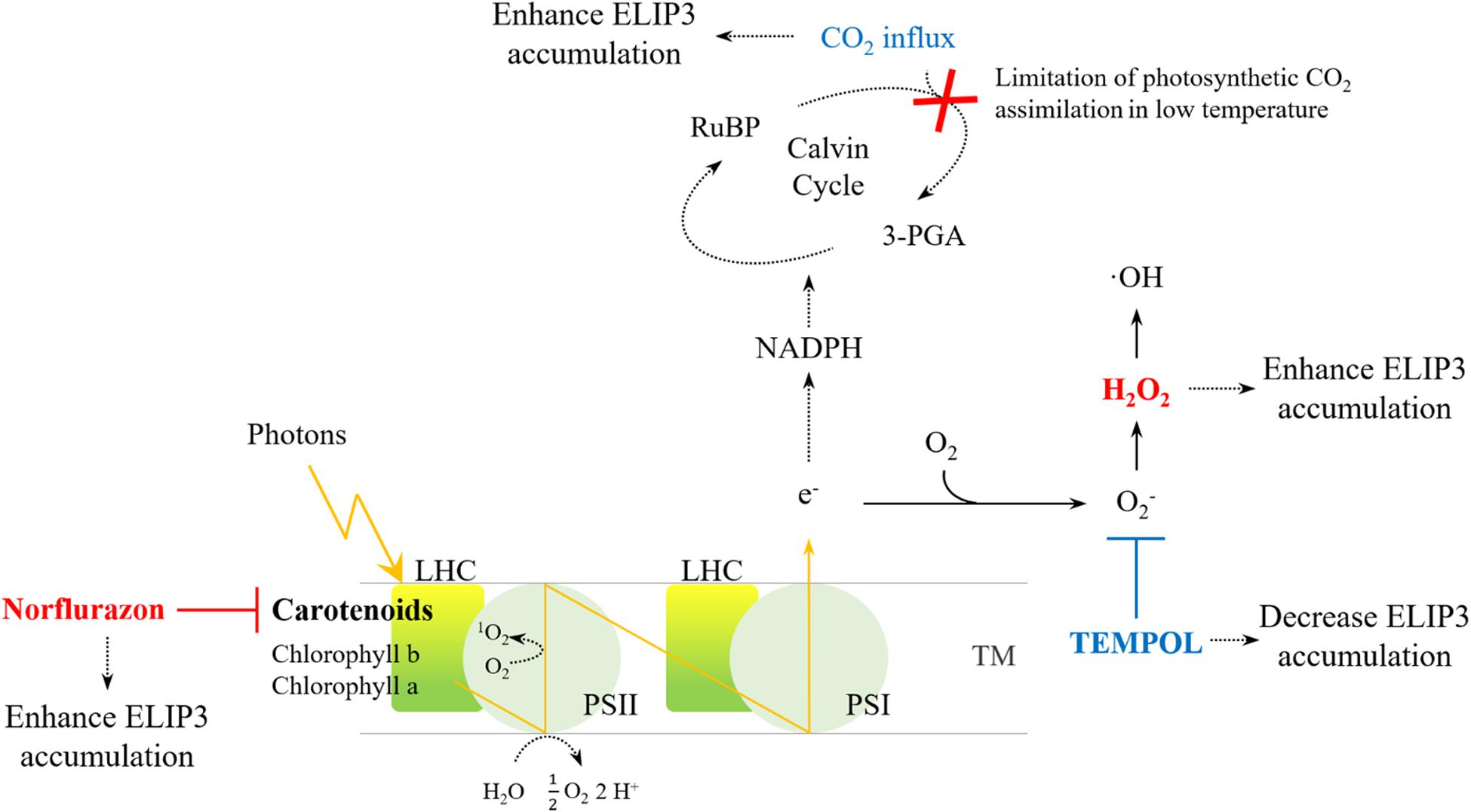
Figure 10. An overview of ROS generation in PSI and PSII and the effect of redox agents. The activity of the CO2 fixation enzyme, in the Cavin cycle, is limited under low temperatures, thereby leading to ROS production due to over-reduction of the photosystems. Increased CO2 may dissipate excess energy in the photosystems. The redox reagents were used to increase ROS (red) or to scavenge ROS (blue) in each photosystem. LHC, Light-harvesting complex; PS, photosystem; RuBP, Ribulose 1,5-bisphosphate; TM, thylakoid membrane; 3-PGA, 3-phosphoglyceric acid.
Algae appear to have higher thresholds for light stress than land plants. Microalgae grow optimally below 400 μmol photons m–2 s–1, and photoinhibition mostly occurs above 1,000 μmol photons m–2 s–1 (Singh and Singh, 2015). C. reinhardtii is usually cultured below 100 μmol photons m–2 s–1, but its best growth has been reported in much higher light intensity (Virtanen et al., 2019). Most land plants suffer from photo-oxidation around 400 μmol photons m–2 s–1 and the expression of ELIPs has been reported at even lower light intensity (Shimosaka et al., 1999). Most ELIPs reported in land plants accumulate in a high-light-dependent manner, and are not induced by low temperature alone, except for some ELIP homologs which can be induced also by other abiotic stresses (Adamska and Kloppstech, 1994; Ouvrard et al., 1996; Adamska, 2001). An ELIP homolog was reported in a multicellular green alga Spirogyra varians, which was also not induced by light but was induced by low temperature even in the dark (Han and Kim, 2013). It is not surprising that the accumulation of ELIP3 was induced by cold stress and not by light stress alone. Although the light intensity of 1,000 μmol photons m–2 s–1 was the highest level we can generate without raising the temperature in our apparatus, it might not be strong enough to generate photooxidative stress in C. reinhardtii. Cold stress especially when combined with high light may induce much stronger photooxidative stress to microalgae because cells are directly in contact with surrounding water body. ELIP3 in C. reinhardtii might have evolved to cope with the photooxidative stress in low temperature.
What is the signal that triggers accumulation of ELIP3 in C. reinhardtii? Our results from the experiments using redox agents showed that the accumulation of ELIP3 does not occur especially when cellular ROS level was scavenged with TEMPOL treatment. Cellular ROS are mostly generated in the photosystem. Under environmental stress, the increase of ROS by the photosystems acts as a major factor in photoinhibition (Murata et al., 2007; Lima-Melo et al., 2019). O2–, and subsequently H2O2, are generated by the Mehler reaction and water-water cycle from PSI, this reaction is an alternative electron sink that dissipated some excess excitation energy when CO2 availability is limited (Mehler, 1951; Asada, 1999; Takahashi and Murata, 2008). CO2-limitation promotes the production of singlet oxygen (1O2) in PSII, leading to the degradation of D1 and inhibition of the repair cycle in the reaction center along with H2O2, which is a major cause of photoinhibition (Nishiyama et al., 2001; Krieger-Liszkay, 2005; Takahashi and Murata, 2008). Although ROS production in environmental stress is genetically programmed for cellular signaling, high accumulation of ROS eventually leads to cell death (Shao et al., 2008; Choudhury et al., 2013; Mittler, 2017). It is well known that elevated CO2 supply can mitigate photooxidative stress in higher plants (Pérez-López et al., 2009; AbdElgawad et al., 2015; Xu et al., 2015). Previous studies in a multicellular green alga, Spirogyra varians, showed that CO2 influx may relieve photooxidative stress and reduce the expression of an ELIP homolog (Han and Kim, 2013). However, our results showed that CO2 influx in photooxidative conditions enhanced survival of the cell and induced greater accumulation of ELIP3.
Why does CO2 influx under photooxidative stress induce enhanced accumulation of ELIP3 in C. reinhardtii? It would have been more logical if the accumulation of ELIP3 is reduced as photooxidative stress was mitigated and cell survival was enhanced by CO2 influx, but the result was the opposite. It may be because we used mixotrophic TAP medium for the culture. It is known that these photomixotrophic conditions using TAP medium alleviate qE and photoinhibitory quenching (qI) of PSII in C. reinhardtii (Roach et al., 2013; Polukhina et al., 2016). The cells can use acetate contained in TAP medium to produce NADPH by catabolism and induces type II NADPH dehydrogenase (NDA2)-dependent cyclic electron flow (CEF) (Johnson and Alric, 2012; Burlacot et al., 2019), resulting in PSII limitation by over-reduction of the plastoquinone (PQ) pool in extreme photooxidative stress. This matches well with our PAM data showing very low NPQ value in overexpression mutant as well as knocked down strains. As a result, ELIP3 needs to be accumulated as the limitation was shifted back to PSII, however, qI was strongly induced by severe stress conditions despite the accumulation of ELIP3 in the absence of CO2 influx. The CO2 influx that could alleviates these limitations affected the accumulation of ELIP3 as well as D1 protein. These results suggest that the accumulation of ELIP3 affects more to qI than qE.
What is the role of ELIP3 in C. reinhardtii? Our results suggest that the accumulation of ELIP3 helps cell to alleviate photooxidative stress at low temperature. Then how? The function of ELIPs in higher plants is to disperse excess amounts of absorbed energy in the form of heat or fluorescence (Adamska et al., 1999; Montané and Kloppstech, 2000). ELIPs in higher plants are also known to inhibit the production of harmful 1O2 from 3Chl by neutralization and degradation of free chlorophyll in photosystem under stress conditions (Hutin et al., 2003; VanBuren et al., 2019). A reduction in zeaxanthin, a known NPQ agent, was observed in experiments using Arabidopsis elip1/elip2 mutant, suggesting that ELIPs are associated with xanthophyll regulation too (Rossini et al., 2006). There are other proteins involved in the protection of photosystem from photooxidative stress in C. reinhardtii. For example, light-harvesting complex stress-related protein 3 (LHCSR3) which has similar transmembrane structure to ELIP3 regulates qE in high light stress and is known to protect photosystems (Maruyama et al., 2014; Girolomoni et al., 2019; Roach et al., 2020). Difference lies in that only ELIP3 shows low-temperature dependent expression. Western blot analysis on D1 protein in PSII offered some clue about the function of ELIP3. D1 protein degraded when PSII is suffering from photooxidative stress in low temperature. When the accumulation of ELIP3 protein was reduced by TEMPOL treatment, D1 protein was degraded even though cellular oxidative stress was reduced. The accumulation of ELIP3 appears to be affected by the superoxide anion (O2–) generated from PSI because TEMPOL scavenges only O2– and does not remove other harmful 1O2 generated in PSII. Our results showed degradation of D1 proteins at low temperature even when cellular O2– was scavenged with the treatment of TEMPOL. These results may suggest that some ROS signaling is required for the maintenance of D1 in low temperature, or TEMPOL may cause damage to PSII although we could not find any reference on it. It is also possible that D1 protein in PSII requires ELIP3 for protection against photooxidative stress. Norflurazon treatment increases harmful 1O2 in PSII by inhibiting carotenoids production (Larkin, 2014). It is interesting that D1 protein was not degraded with the treatment of norflurazon, while ELIP3 accumulated. A knockdown mutant of ELIP3 showed faster degradation of D1 protein than WT under photooxidative condition supporting the idea that ELIP3 may be involved in the protection of other proteins in the photosystem. ELIP3 appears to have similar function to that of ELIPs in higher plants in protecting photosystem from oxidative stress. It is noteworthy that TAP medium that we used in this study provides mixotrophic condition to C. reinhardtii and makes it difficult to interpret our data in relation with ELIP3 function as qE. Further studies using various media will provide more information about the function of ELIP3 in maintaining NPQ under cold stress.
Cellular ROS is not the only signal that triggers accumulation of ELIP3 in C. reinhardtii. Strong accumulation of ELIP3 was induced by UV-A irradiation while UV-B irradiation did not induce any accumulation suggesting that a photoreceptor-mediated regulation may be involved in its expression. Cryptochrome (CRY), a blue/UV-A photoreceptor, has been proposed as a regulator for the expression of ELIPs in higher plants (Kleine et al., 2007). A transcription factor ELONGATED HYPOCOTYL 5 (HY5) that affects CRY1-dependent high light response has been identified in Arabidopsis ELIP2 promoter studies (Kleine et al., 2007; Hayami et al., 2015). However, not all ELIPs in higher plant are CRY-dependently induced (Kleine et al., 2007), nor regulated by HY5-type transcription factors (Hayami et al., 2015). Further functional studies using transgenic mutants of CRY and ELIP3 are necessary to elucidate the signals involved in this response in C. reinhardtii.
The direction of phototaxis in C. reinhardtii is regulated by the redox poise of the cytoplasm (Wakabayashi et al., 2011). Phosphorylation of channelrhodopsin-1 is affected by the redox state of the cytoplasm and changes with phototactic behavior in response to physiological stimuli in C. reinhardtii (Böhm et al., 2019). Our results showed that the knockdown mutant of ELIP3 showed negative phototaxis at room temperature suggesting that the mutant has different redox poise of the cytoplasm from that of WT or overexpression strain. C. reinhardtii has a homeostatic negative feedback mechanism to maintain a slightly reduced environment and to avoid oxidative damage in the cell via modulation of photosynthetic activity (Wakabayashi et al., 2011). The knockdown strain shows lower effective and maximum quantum yield than WT even in moderate light and warm temperature. These results suggest that decreased photosynthetic activity in the knock down mutant could fail to maintain a reduced environment in the cytoplasm which may result in altered direction of movement. Further studies on the signals for the phototaxis in relation with the expression of ELIP3 may reveal other roles of ELIP3 in the regulation of movement in C. reinhardtii.
Cold temperature is one of the most serious challenges in developing large-scale microalgal production facility outside of tropical or sub-tropical locations (Laamanen et al., 2014). Temperature often drops at night below 10°C even in temperate regions, which causes photooxidative damage to the cell. Our results show that ELIP3 is a promising target gene to develop cold-tolerant strains because it is involved in the protection of photosystems and helps to enhance photosynthetic efficiency at low temperature. Further studies using various combination of transgenic mutants are necessary to achieve the final goal in developing useful cold-tolerant strains of C. reinhardtii.
Data Availability Statement
The original contributions presented in the study are included in the article/Supplementary Material, further inquiries can be directed to the corresponding author.
Author Contributions
JWL, SHL, and GHK conceived and designed research. JWL and SHL conducted experiments. JWL and JWH contributed research idea and analytical tools. JWL and GHK analyzed the data and wrote the manuscript. All authors read and approved the manuscript.
Funding
This work was supported by National Research Foundation of Korea grant (NRF-2019M3C1B7025093); and partly supported by Korea Institute of Planning and Evaluation for Technology in Food, Agriculture, and Forestry (IPET) through Golden Seed Project (213008-05-2-SB810), funded by Ministry of Agriculture, Food and Rural Affairs (MAFRA), Ministry of Oceans and Fisheries (MOF), Rural Development Administration (RDA), and Korea Forest Services (KFS).
Conflict of Interest
The authors declare that the research was conducted in the absence of any commercial or financial relationships that could be construed as a potential conflict of interest.
Acknowledgments
We thank Prof. Joe Zuccarello for the careful revision of the manuscript and useful comments. We also express sincere thanks to Dr. Won-Joong Jeong of Korea Research Institute of Bioscience and Biotechnology (KRIBB) and Prof. Ju-Hyoung Kim of Kunsan National University for technical assistance.
Supplementary Material
The Supplementary Material for this article can be found online at: https://www.frontiersin.org/articles/10.3389/fphys.2020.01083/full#supplementary-material
Footnotes
References
AbdElgawad, H., Farfan-Vignolo, E. R., de Vos, D., and Asard, H. (2015). Elevated CO2 mitigates drought and temperature-induced oxidative stress differently in grasses and legumes. Plant Sci. 231, 1–10. doi: 10.1016/j.plantsci.2014.11.001
Adamska, I. (2001). “The Elip family of stress proteins in the thylakoid membranes of pro- and eukaryota,” in Regulation of Photosynthesis, eds E.-M. Aro and B. Andersson (Dordrecht: Springer), 487–505. doi: 10.1007/0-306-48148-0_28
Adamska, I., and Kloppstech, K. (1994). Low temperature increases the abundance of early light-inducible transcript under light stress conditions. J. Biol. Chem. 269, 30221–30226.
Adamska, I., KloppstechS, K., and Ohad, I. (1992a). UV light stress induces the synthesis of the early light-inducible protein and prevents its degradation. J. Biol. Chem. 267, 24732–24737.
Adamska, I., Ohad, I., and Kloppstech, K. (1992b). Synthesis of the early light-inducible protein is controlled by blue light and related to light stress. Proc. Natl. Acad. Sci. U.S.A. 89, 2610–2613. doi: 10.1073/pnas.89.7.2610
Adamska, I., Roobol-Bóza, M., Lindahl, M., and Andersson, B. (1999). Isolation of pigment-binding early light-inducible proteins from pea: ELIPs, chlorophyll-binding proteins with transient appearance. Eur. J. Biochem. 260, 453–460. doi: 10.1046/j.1432-1327.1999.00178.x
Allen, D. J., and Ort, D. R. (2001). Impacts of chilling temperatures on photosynthesis in warm-climate plants. Trends Plant Sci. 6, 36–42. doi: 10.1016/S1360-1385(00)01808-2
Apel, A. C., and Weuster-Botz, D. (2015). Engineering solutions for open microalgae mass cultivation and realistic indoor simulation of outdoor environments. Bioproc. Biosyst. Eng. 38, 995–1008. doi: 10.1007/s00449-015-1363-1
Apel, K., and Hirt, H. (2004). Reactive oxygen species: metabolism, oxidative stress, and signal transduction. Annu. Rev. Plant Biol. 55, 373–399. doi: 10.1146/annurev.arplant.55.031903.141701
Asada, K. (1999). The water-water cycle in chloroplasts: scavenging of active oxygens and dissipation of excess photons. Annu. Rev. Plant. Physiol. Plant. Mol. Biol. 50, 601–639. doi: 10.1146/annurev.arplant.50.1.601
Asada, K. (2006). Production and scavenging of reactive oxygen species in chloroplasts and their functions. Plant Physiol. 141, 391–396. doi: 10.1104/pp.106.082040
Baliga, R., and Powers, S. E. (2010). Sustainable algae biodiesel production in cold climates. Int. J. Chem. Eng. 2010, 1–13. doi: 10.1155/2010/102179
Böhm, M., Boness, D., Fantisch, E., Erhard, H., Frauenholz, J., Kowalzyk, Z., et al. (2019). Channelrhodopsin-1 phosphorylation changes with phototactic behavior and responds to physiological stimuli in Chlamydomonas. Plant Cell 31, 886–910. doi: 10.1105/tpc.18.00936
Burlacot, A., Peltier, G., and Li-Beisson, Y. (2019). Subcellular energetics and carbon storage in Chlamydomonas. Cells 8:1154. doi: 10.3390/cells8101154
Casazza, A. P., Rossini, S., Rosso, M. G., and Soave, C. (2005). Mutational and expression analysis of ELIP1 and ELIP2 in Arabidopsis thaliana. Plant Mol. Biol. 58, 41–51. doi: 10.1007/s11103-005-4090-1
Caverzan, A., Passaia, G., Rosa, S. B., Ribeiro, C. W., Lazzarotto, F., and Margis-Pinheiro, M. (2012). Plant responses to stresses: role of ascorbate peroxidase in the antioxidant protection. Genet. Mol. Biol. 35, 1011–1019. doi: 10.1590/S1415-47572012000600016
Chisti, Y. (2007). Biodiesel from microalgae. Biotechnol. Adv. 25, 294–306. doi: 10.1016/j.biotechadv.2007.02.001
Choi, S., Jeong, S., Jeong, W., Kwon, S., Chow, W., and Park, Y. I. (2002). Chloroplast Cu/Zn-superoxide dismutase is a highly sensitive site in cucumber leaves chilled in the light. Planta 216, 315–324. doi: 10.1007/s00425-002-0852-z
Choudhury, S., Panda, P., Sahoo, L., and Panda, S. K. (2013). Reactive oxygen species signaling in plants under abiotic stress. Plant Signal. Behav. 8:e23681. doi: 10.4161/psb.23681
Elrad, D., and Grossman, A. R. (2004). A genome’s-eye view of the light-harvesting polypeptides of Chlamydomonas reinhardtii. Curr. Genet. 45, 61–75. doi: 10.1007/s00294-003-0460-x
Engelken, J., Funk, C., and Adamska, I. (2012). “The extended light-harvesting complex (LHC) protein superfamily: classification and evolutionary dynamics,” in Functional Genomics and Evolution of Photosynthetic Systems, eds R. Burnap and W. Vermaas (Dordrecht: Springer), 265–284. doi: 10.1007/978-94-007-1533-2_11
Fowler, S., and Thomashow, M. F. (2002). Arabidopsis transcriptome profiling indicates that multiple regulatory pathways are activated during cold acclimation in addition to the CBF cold response pathway. Plant Cell 14, 1675–1690. doi: 10.1105/tpc.003483
Gan, P., Liu, F., Li, R., Wang, S., and Luo, J. (2019). Chloroplasts— beyond energy capture and carbon fixation: tuning of photosynthesis in response to chilling stress. Int. J. Mol. Sci. 20:5046. doi: 10.3390/ijms20205046
Girolomoni, L., Cazzaniga, S., Pinnola, A., Perozeni, F., Ballottari, M., and Bassi, R. (2019). LHCSR3 is a nonphotochemical quencher of both photosystems in Chlamydomonas reinhardtii. Proc. Natl. Acad. Sci. U.S.A. 116, 4212–4217. doi: 10.1073/pnas.1809812116
Grimm, B., and Kloppstech, K. (1987). The early light-inducible proteins of barley: characterization of two families of 2-h-specific nuclear-coded chloroplast proteins. Eur. J. Biochem. 167, 493–499. doi: 10.1111/j.1432-1033.1987.tb13364.x
Han, J. W., and Kim, G. H. (2013). An ELIP-like gene in the freshwater green alga, Spirogyra varians (Zygnematales), is regulated by cold stress and CO2 influx. J. Appl. Phycol. 25, 1297–1307. doi: 10.1007/s10811-013-9975-9
Harris, E. H. ed. (2009). “Chlamydomonas in the laboratory,” in The Chlamydomonas Sourcebook: Introduction to Chlamydomonas and its Laboratory use, Vol. 1, (San Diego, CA: Academic press), 243.
Hayami, N., Sakai, Y., Kimura, M., Saito, T., Tokizawa, M., Iuchi, S., et al. (2015). The responses of Arabidopsis early light-induced protein2 to ultraviolet b, high light, and cold stress are regulated by a transcriptional regulatory unit composed of two elements. Plant Physiol. 169, 840–855. doi: 10.1104/pp.15.00398
Heddad, M., and Adamska, I. (2002). The evolution of light stress proteins in photosynthetic organisms. Int. J. Genomics 3, 504–510. doi: 10.1002/cfg.221
Heddad, M., Norén, H., Reiser, V., Dunaeva, M., Andersson, B., and Adamska, I. (2006). Differential expression and localization of early light-induced proteins in Arabidopsis. Plant Physiol. 142, 75–87. doi: 10.1104/pp.106.081489
Hema, R., Senthil-Kumar, M., Shivakumar, S., Chandrasekhara Reddy, P., and Udayakumar, M. (2007). Chlamydomonas reinhardtii, a model system for functional validation of abiotic stress responsive genes. Planta 226, 655–670. doi: 10.1007/s00425-007-0514-2
Hirotsu, N., Makino, A., Ushio, A., and Mae, T. (2004). Changes in the thermal dissipation and the electron flow in the water–water cycle in rice grown under conditions of physiologically low temperature. Plant Cell Physiol. 45, 635–644. doi: 10.1093/pcp/pch075
Hutin, C., Nussaume, L., Moise, N., Moya, I., Kloppstech, K., and Havaux, M. (2003). Early light-induced proteins protect Arabidopsis from photooxidative stress. Proc. Natl. Acad. Sci. U.S.A. 100, 4921–4926. doi: 10.1073/pnas.0736939100
Im, S. H., Klochkova, T. A., Lee, D. J., Gachon, C. M. M., and Kim, G. H. (2019). Genetic toolkits of the red alga Pyropia tenera against the three most common diseases in Pyropia farms. J. Phycol. 55, 801–815. doi: 10.1111/jpy.12857
Jiang, Y. P., Huang, L. F., Cheng, F., Zhou, Y. H., Xia, X.-J., Mao, W. H., et al. (2013). Brassinosteroids accelerate recovery of photosynthetic apparatus from cold stress by balancing the electron partitioning, carboxylation and redox homeostasis in cucumber. Physiol. Plantarum. 148, 133–145. doi: 10.1111/j.1399-3054.2012.01696.x
Johnson, X., and Alric, J. (2012). Interaction between starch breakdown, acetate assimilation, and photosynthetic cyclic electron flow in Chlamydomonas reinhardtii. J. Biol. Chem. 287, 26445–26452. doi: 10.1074/jbc.M112.370205
Kaiser, W. (1976). The effect of hydrogen peroxide on CO2 fixation of isolated intact chloroplasts. BBA Bioenerg. 440, 476–482. doi: 10.1016/0005-2728(76)90035-9
Kim, E., Park, H. S., Jung, Y., Choi, D. W., Jeong, W. J., Park, H. S., et al. (2011). Identification of the high-temperature response genes from Porphyra seriata (Rhodophyta) expression sequence tags and enhancement of heat tolerance of Chlamydomonas (Chlorophyta) by expression of the Porphyra HTR2 gene1: high-temperature stress in Porphyra. J. Phycol. 47, 821–828. doi: 10.1111/j.1529-8817.2011.01008.x
Kleine, T., Kindgren, P., Benedict, C., Hendrickson, L., and Strand, Å (2007). Genome-wide gene expression analysis reveals a critical role for Cryptochrome1 in the response of Arabidopsis to high irradiance. Plant Physiol. 144, 1391–1406. doi: 10.1104/pp.107.098293
Kotchoni, S. O., Gachomo, E. W., Slobodenko, K., and Shain, D. H. (2016). AMP deaminase suppression increases biomass, cold tolerance and oil content in green algae. Algal Res. 16, 473–480. doi: 10.1016/j.algal.2016.04.007
Krieger-Liszkay, A. (2005). Singlet oxygen production in photosynthesis. J. Exp. Bot. 56, 337–346. doi: 10.1093/jxb/erh237
Krieger-Liszkay, A., Fufezan, C., and Trebst, A. (2008). Singlet oxygen production in photosystem II and related protection mechanism. Photosynth. Res. 98, 551–564. doi: 10.1007/s11120-008-9349-3
Kwak, M., Park, W. K., Shin, S. E., Koh, H. G., Lee, B., Jeong, B., et al. (2017). Improvement of biomass and lipid yield under stress conditions by using diploid strains of Chlamydomonas reinhardtii. Algal Res. 26, 180–189. doi: 10.1016/j.algal.2017.07.027
Laamanen, C. A., Shang, H., Ross, G. M., and Scott, J. A. (2014). A model for utilizing industrial off-gas to support microalgae cultivation for biodiesel in cold climates. Energ. Convers. Manage. 88, 476–483. doi: 10.1016/j.enconman.2014.08.047
Larkin, R. M. (2014). Influence of plastids on light signalling and development. Phil. Trans. R. Soc. B 369, 20130232. doi: 10.1098/rstb.2013.0232
Lee, J. W., and Kim, G. H. (2019). Red and far−red regulation of filament movement correlates with the expression of phytochrome and FHY 1 genes in Spirogyra varians (Zygnematales, Streptophyta). J. Phycol. 55, 688–699. doi: 10.1111/jpy.12849
Lima-Melo, Y., Alencar, V. T. C. B., Lobo, A. K. M., Sousa, R. H. V., Tikkanen, M., Aro, E. M., et al. (2019). Photoinhibition of photosystem I provides oxidative protection during imbalanced photosynthetic electron transport in Arabidopsis thaliana. Front. Plant Sci. 10:916. doi: 10.3389/fpls.2019.00916
Maruyama, S., Tokutsu, R., and Minagawa, J. (2014). Transcriptional regulation of the stress-responsive light harvesting complex genes in Chlamydomonas reinhardtii. Plant Cell Physiol. 55, 1304–1310. doi: 10.1093/pcp/pcu068
Mehler, A. H. (1951). Studies on reactions of illuminated chloroplasts: I. Mechanism of the reduction of oxygen and other hill reagents. Arch. Biochem. Biophys. 33, 65–77. doi: 10.1016/0003-9861(51)90082-3
Montané, M. H., and Kloppstech, K. (2000). The family of light-harvesting-related proteins (LHCs, ELIPs, HLIPs): was the harvesting of light their primary function? Gene 258, 1–8. doi: 10.1016/S0378-1119(00)00413-3
Murata, N., Takahashi, S., Nishiyama, Y., and Allakhverdiev, S. I. (2007). Photoinhibition of photosystem II under environmental stress. BBA Bioenerg. 1767, 414–421. doi: 10.1016/j.bbabio.2006.11.019
Nishiyama, Y., Yamamoto, H., Allakhverdiev, S. I., Inaba, M., Yokota, A., and Murata, N. (2001). Oxidative stress inhibits the repair of photodamage to the photosynthetic machinery. EMBO J. 20, 5587–5594. doi: 10.1093/emboj/20.20.5587
Ouvrard, O., Cellier, F., Ferrare, K., Tousch, D., Lamaze, T., Dupuis, J. M., et al. (1996). Identification and expression of water stress- and abscisic acid-regulated genes in a drought-tolerant sunflower genotype. Plant Mol. Biol. 31, 819–829. doi: 10.1007/BF00019469
Pérez-López, U., Robredo, A., Lacuesta, M., Sgherri, C., Muñoz-Rueda, A., Navari-Izzo, F., et al. (2009). The oxidative stress caused by salinity in two barley cultivars is mitigated by elevated CO2. Physiol. Plant. 135, 29–42. doi: 10.1111/j.1399-3054.2008.01174.x
Polukhina, I., Fristedt, R., Dinc, E., Cardol, P., and Croce, R. (2016). Carbon supply and photoacclimation cross talk in the green alga Chlamydomonas reinhardtii. Plant Physiol. 172, 1494–1505. doi: 10.1104/pp.16.01310
Powles, S. B. (1984). Photoinhibition of photosynthesis induced by visible light. Annu. Rev. Plant. Physiol. 35, 15–44. doi: 10.1146/annurev.pp.35.060184.000311
Radakovits, R., Jinkerson, R. E., Darzins, A., and Posewitz, M. C. (2010). Genetic engineering of algae for enhanced biofuel production. Eukaryot. Cell 9, 486–501. doi: 10.1128/EC.00364
Ralph, P. J., and Gademann, R. (2005). Rapid light curves: a powerful tool to assess photosynthetic activity. Aquat. Bot. 82, 222–237. doi: 10.1016/j.aquabot.2005.02.006
Roach, T., Na, C. S., Stöggl, W., and Krieger-Liszkay, A. (2020). The non-photochemical quenching protein LHCSR3 prevents oxygen-dependent photoinhibition in Chlamydomonas reinhardtii. J. Exp. Bot. 71, 2650–2660. doi: 10.1093/jxb/eraa022
Roach, T., Sedoud, A., and Krieger-Liszkay, A. (2013). Acetate in mixotrophic growth medium affects photosystem II in Chlamydomonas reinhardtii and protects against photoinhibition. BBA Bioenerg. 1827, 1183–1190. doi: 10.1016/j.bbabio.2013.06.004
Rochaix, J. D., and Bassi, R. (2019). LHC-like proteins involved in stress responses and biogenesis/repair of the photosynthetic apparatus. Biochemi. J. 476, 581–593. doi: 10.1042/BCJ20180718
Rohr, J., Sarkar, N., Balenger, S., Jeong, B., and Cerutti, H. (2004). Tandem inverted repeat system for selection of effective transgenic RNAi strains in Chlamydomonas: tandem inverted repeat system for efficient RNAi. Plant J. 40, 611–621. doi: 10.1111/j.1365-313X.2004.02227.x
Rosenberg, J. N., Oyler, G. A., Wilkinson, L., and Betenbaugh, M. J. (2008). A green light for engineered algae: redirecting metabolism to fuel a biotechnology revolution. Curr. Opin. Biotech. 19, 430–436. doi: 10.1016/j.copbio.2008.07.008
Rossini, S., Casazza, A. P., Engelmann, E. C. M., Havaux, M., Jennings, R. C., and Soave, C. (2006). Suppression of both ELIP1 and ELIP2 in Arabidopsis does not affect tolerance to photoinhibition and photooxidative stress. Plant Physiol. 141, 1264–1273. doi: 10.1104/pp.106.083055
Ruiz, J., Olivieri, G., de Vree, J., Bosma, R., Willems, P., Reith, J. H., et al. (2016). Towards industrial products from microalgae. Energy Environ. Sci. 9, 3036–3043. doi: 10.1039/C6EE01493C
Serôdio, J., Vieira, S., Cruz, S., and Coelho, H. (2007). Rapid light-response curves of chlorophyll fluorescence in microalgae: relationship to steady-state light curves and non-photochemical quenching in benthic diatom-dominated assemblages. Photosynth. Res. 90, 29–43. doi: 10.1007/s11120-006-9105-5
Shao, H. B., Chu, L. Y., Lu, Z. H., and Kang, C. M. (2008). Primary antioxidant free radical scavenging and redox signaling pathways in higher plant cells. Int. J. Biol. Sci. 4, 8–14. doi: 10.7150/ijbs.4.8
Shimosaka, E., Sasanuma, T., and Handa, H. (1999). A wheat cold-regulated cDNA encoding an early light-inducible protein (ELIP): its structure, expression and chromosomal location. Plant Cell Physiol. 40, 319–325. doi: 10.1093/oxfordjournals.pcp.a029544
Shin, S. E., Lim, J. M., Koh, H. G., Kim, E. K., Kang, N. K., Jeon, S., et al. (2016). CRISPR/Cas9-induced knockout and knock-in mutations in Chlamydomonas reinhardtii. Sci. Rep. 6:27810. doi: 10.1038/srep27810
Singh, S. P., and Singh, P. (2015). Effect of temperature and light on the growth of algae species: a review. Renew. Sust. Energ. Rev. 50, 431–444. doi: 10.1016/j.rser.2015.05.024
Spolaore, P., Joannis-Cassan, C., Duran, E., and Isambert, A. (2006). Commercial applications of microalgae. J. Biosci. Bioeng. 101, 87–96. doi: 10.1263/jbb.101.87
Takahashi, S., and Murata, N. (2006). Glycerate-3-phosphate, produced by CO2 fixation in the Calvin cycle, is critical for the synthesis of the D1 protein of photosystem II. BBA Bioenerg. 1757, 198–205. doi: 10.1016/j.bbabio.2006.02.002
Takahashi, S., and Murata, N. (2008). How do environmental stresses accelerate photoinhibition? Trends Plant Sci. 13, 178–182. doi: 10.1016/j.tplants.2008.01.005
Tanaka, K., Otsubo, T., and Kondo, N. (1982). Participation of hydrogen peroxide in the inactivation of Calvin-Cycle SH enzymes in SO2-fumigated spinach leaves. Plant Cell Physiol. 23, 1009–1018. doi: 10.1093/oxfordjournals.pcp.a076430
Teramoto, H., Itoh, T., and Ono, T. (2004). High-intensity-light-dependent and transient expression of new genes encoding distant relatives of light-harvesting chlorophyll-a/b proteins in Chlamydomonas reinhardtii. Plant Cell Physiol. 45, 1221–1232. doi: 10.1093/pcp/pch157
VanBuren, R., Pardo, J., Man Wai, C., Evans, S., and Bartels, D. (2019). Massive tandem proliferation of ELIPs supports convergent evolution of desiccation tolerance across land plants. Plant Physiol. 179, 1040–1049. doi: 10.1104/pp.18.01420
Virtanen, O., Valev, D., Kruse, O., Wobbe, L., and Tyystjarvi, E. (2019). Photoinhibition and continuous growth of the wild-type and a high-light tolerant strain of Chlamydomonas reinhardtii. Photosyntthetica 57, 617–626. doi: 10.32615/ps.2019.056
Wakabayashi, K. I., Misawa, Y., Mochiji, S., and Kamiya, R. (2011). Reduction-oxidation poise regulates the sign of phototaxis in Chlamydomonas reinhardtii. Proc. Natl. Acad. Sci. U.S.A. 108, 11280–11284. doi: 10.1073/pnas.1100592108
Xu, Z., Jiang, Y., and Zhou, G. (2015). Response and adaptation of photosynthesis, respiration, and antioxidant systems to elevated CO2 with environmental stress in plants. Front. Plant Sci. 6:701. doi: 10.3389/fpls.2015.00701
Zhou, Y. H., Yu, J. Q., Huang, L. F., and Nogues, S. (2004). The relationship between CO2 assimilation, photosynthetic electron transport and water-water cycle in chill-exposed cucumber leaves under low light and subsequent recovery. Plant Cell Environ. 27, 1503–1514. doi: 10.1111/j.1365-3040.2004.01255.x
Zhu, L. (2015). Biorefinery as a promising approach to promote microalgae industry: an innovative framework. Renew. Sust. Energ. Rev. 41, 1376–1384. doi: 10.1016/j.rser.2014.09.040
Keywords: ELIP, mutation, cold stress, ROS, CO2
Citation: Lee JW, Lee SH, Han JW and Kim GH (2020) Early Light-Inducible Protein (ELIP) Can Enhance Resistance to Cold-Induced Photooxidative Stress in Chlamydomonas reinhardtii. Front. Physiol. 11:1083. doi: 10.3389/fphys.2020.01083
Received: 24 March 2020; Accepted: 06 August 2020;
Published: 25 August 2020.
Edited by:
Koji Mikami, Hokkaido University, JapanReviewed by:
Xenie Johnson, Commissariat à l’Energie Atomique et aux Energies Alternatives (CEA), FranceWayne R. Fitzgibbon, Medical University of South Carolina, United States
Copyright © 2020 Lee, Lee, Han and Kim. This is an open-access article distributed under the terms of the Creative Commons Attribution License (CC BY). The use, distribution or reproduction in other forums is permitted, provided the original author(s) and the copyright owner(s) are credited and that the original publication in this journal is cited, in accordance with accepted academic practice. No use, distribution or reproduction is permitted which does not comply with these terms.
*Correspondence: Gwang Hoon Kim, Z2hraW1Aa29uZ2p1LmFjLmty